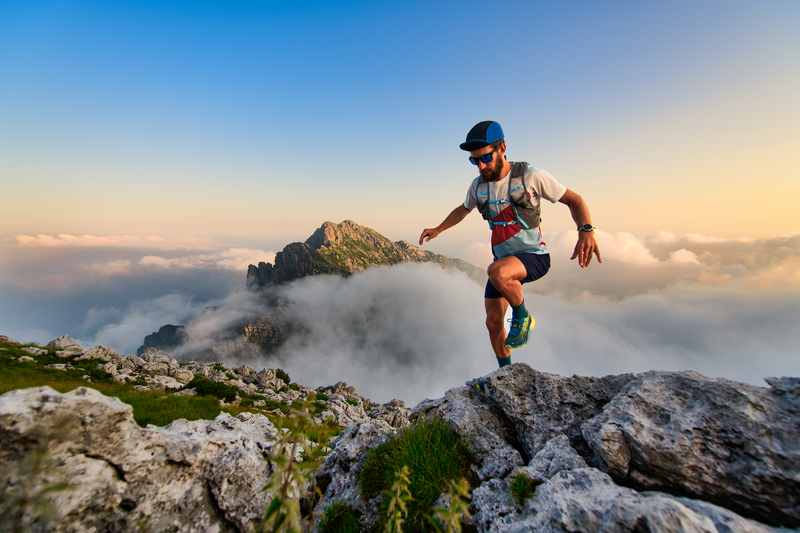
95% of researchers rate our articles as excellent or good
Learn more about the work of our research integrity team to safeguard the quality of each article we publish.
Find out more
MINI REVIEW article
Front. Neurosci. , 05 March 2019
Sec. Neuroendocrine Science
Volume 13 - 2019 | https://doi.org/10.3389/fnins.2019.00177
This article is part of the Research Topic Origins of Human Neuropathology: The Significance of Teneurin-Latrophilin Interaction View all 21 articles
Teneurins have well established roles in function and maintenance of the central nervous systems of vertebrates. In addition, teneurin c-terminal associated peptide (TCAP), a bioactive peptide found on the c-terminal portion of teneurins, has been shown to regulate glucose metabolism. Although, the majority of research conducted on the actions of teneurins and TCAPs has strictly focused on neurological systems in rodents, TCAP was first identified in rainbow trout after screening trout hypothalamic cDNA. This suggests a conserved functional role of TCAP across vertebrates, however, the current depth of literature on teneurins and TCAPs in fish is limited. In addition, the overall function of TCAP in regulating metabolism is unclear. This review will highlight work that has been conducted specifically in fish species in relation to the teneurin system and metabolism in order to identify areas of research that are needed for future work.
Teneurins, a family of highly conserved proteins, are large signaling molecules that act as type II transmembrane receptors at the cell surface, and intracellularly as transcriptional regulators when the intracellular domain is released (Tucker and Chiquet-Ehrismann, 2006; Tucker et al., 2007; Scholer et al., 2015). Originally identified in the fruit fly, Drosophila melanogaster, as ten-m and ten-a (Baumgartner and Chiquet-Ehrismann, 1993; Baumgartner et al., 1994; Levine et al., 1994), additional teneurin genes have been described in multiple species including chicken (Gallus gallus) (Minet et al., 1999; Rubin et al., 1999; Tucker et al., 2000, 2012), mouse (Mus musculus) (Oohashi et al., 1999; Tucker et al., 2012), rats (Rattus norvegicus) (Otaki and Firestein, 1999), human (Homo sapiens) (Minet and Chiquet-Ehrismann, 2000; Tucker et al., 2012), zebrafish (Danio rerio) (Mieda et al., 1999; Tucker et al., 2012), roundworm (Caenorhabditis elegans) (Drabikowski et al., 1999), and more recently, in the vase tunicate (Ciona intestinalis) (Colacci et al., 2005; Tucker et al., 2012). Four vertebrate teneurins and single C. elegans and C. intestinalis homologs have been identified (see review Tucker et al., 2007).
The teneurins contain a single transmembrane domain and large extracellular C-termini that contain domains important in protein-carbohydrate (YD-repeats) and protein-protein (EGF-repeats) interactions (Figure 1A; Tucker and Chiquet-Ehrismann, 2006). Recent evidence in rodents supports transcriptional regulation activity from the N-terminus (Scholer et al., 2015), and some teneurins contain Ca2+-dependent binding domains and other functional domains (Kawasaki and Kretsinger, 1994; Rubin et al., 1999). Much of teneurin biology and the role of the numerous functional domains and potential interactions across vertebrates remain unknown. Teneurins can homo- or hetero-dimerize (Figure 1B) and subsequently interact with other cells through hemophilic binding or teneurins can directly interact with the latrophilin receptor to elicit cellular responses (Li et al., 2018). Additionally, a small peptide released from the C-terminus, known as teneurin C-terminal associated peptide (TCAP; Figure 1A), can be cleaved and is known to exert action on several cellular functions independently of teneurin action (Wang et al., 2005).
Figure 1. (A) Teneurin peptides are highly conserved and contain an intracellular domain that contains polyproline cites and EH-hand-like Ca2+ binding cites, a transmembrane domain, and an extracellular domain containing 8 epidermal growth factor-like repeats, 17 cystein residues, 26 tyrosine-aspartic acid repeats, and a teneurin c-terminal associated peptide (TCAP). Figure rendered from Tucker and Chiquet-Ehrismann (2006) and Woelfle et al. (2015). (B) Teneurins can dimerize via EGF-like repeats (2 and 5), causing conformational changes that can lead to homophillic binding with neighboring cells. Following dimerization, the intracellular domain can anchor to the cytoskeleton (1) or can be cleaved (2) and translocate to the nucleus where it can interact with transcriptional regulators (Woelfle et al., 2015). (C) Outline of teneurins and TCAPs gene expression and functional analyses from teleost species.
Overall, the function of teneurins as signaling molecules is highly conserved, and consistent with their ancient origin, teneurins have essential mechanisms of action during development, and more specifically during the ontogeny of the nervous system. In C. elegans and D. melanogaster, teneurins have been shown to be required for fundamental developmental processes, like cell migration and axon pathfinding (Topf and Chiquet-Ehrismann, 2011; Beckmann et al., 2013). In fruit flies, teneurin-a is a dimeric receptor present in late stages of neuronal development and regulates eye and nervous system development and muscle attachment (Baumgartner and Chiquet-Ehrismann, 1993; Fascetti and Baumgartner, 2002); while teneurin-m/Odz pair rule genes regulate segmentation and body organization (Baumgartner et al., 1994; Levine et al., 1994). The overall orientation and formation of basement membranes has been shown to be regulated by teneurin-1 in C. elegans (Trzebiatowska et al., 2008). Additionally, teneurin-m/Odz is a homolog of mammalian teneurin-4 (Oohashi et al., 1999; Fascetti and Baumgartner, 2002), which is essential for gastrulation and the epithelial to mesenchymal transition in mice (Lossie et al., 2005). It is well understood that teneurins play a vital role in neuronal wiring, and recent evidence supports involvement in regulating synaptic connections and trans-synaptic signaling (see review Mosca, 2015).
Teneurin-1 occurs in a heterodimeric form via the EGF-like repeats, and is expressed in most tissues in the developing rats (Oohashi et al., 1999). Both teneurin-1 and teneurin-2 direct signaling pathways as their intracellular domains can be translocated to the nucleus (Nunes et al., 2005). Teneurin-1 can be found in the full length or alternatively spliced forms (with medium length or intracellular domain). Most commonly, the intracellular domain of teneurin-1 is found interacting with CAP/ponsin complex to function in cell adhesion to both the matrix and other cells. Additionally, teneurin-1 intracellular domain interacts with methyl-CpG-binding protein (MBD1) to possibly regulate gene transcription of cells within the nervous system (Nunes et al., 2005).
Outside of nervous system development and function, Ishii and co-workers recently demonstrated that teneurin-4 regulates postnatal muscle growth in mice (Ishii et al., 2015). Specifically, removal of teneurin-4 resulted in stunted postnatal growth accompanied by fewer satellite cells, suggesting a role of teneurin-4 in satellite cell proliferation. Interestingly, despite fewer satellite cells, muscle repair and renewal capacity was not affected by the absence of teneurin-4 (Ishii et al., 2015). Much of the work related to teneurin biology has focused heavily on nervous system development in rodent species, however, recent evidence suggests important roles of teneurins outside of this niche.
Teneurins were discovered in fish in 1999, when 2 homologs of tenm/odz were isolated while looking for factors regulated by LIM/homeodomain transcription factor Islet-3 in zebrafish (Mieda et al., 1999). Tenm/odz sequence alignments and identity comparisons confirmed the presence of teneurin homologs in zebrafish: ten-m3 (teneurin-3) and ten-m4 (teneurin-4) (Mieda et al., 1999). The expression profiles of ten-m3 and ten-m4 were shown to be consistent with reported profiles from rodent species, where expression is high during development in zebrafish embryos, particularly in the developing central nervous system (Mieda et al., 1999). More specifically, ten-m3 is expressed developmentally in somites, pharyngeal arches, notochord, and the brain, whereas ten-m4 appears to only be expressed in the developing brain of zebrafish embryos (Mieda et al., 1999).
In 2012, additional teneurin genes were identified in zebrafish and stickleback (Gasterosteus aculeatus), including a complete teneurin-1 sequence and two teneurin-2 paralogues named teneurin-2a and teneurin-2b (Tucker et al., 2012). Further analysis of the teneurin sequences revealed that the teneurin sequences were mostly conserved, except for the potential absence of key processing sites, such as a furin cleavage site in teneurin-1 and nuclear localization sequences (NLS) in teneurin-1 and teneurin-2a (Tucker et al., 2012). Additionally, there is a predicted proline-rich SH3 binding domain in the N-terminal intracellular domain of teneurin-3 and a potential additional furin cleavage site on teneurin-2a. Five teneurin genes were also identified in stickleback, where stickleback have retained two teneurin-3 paralogues (teneurin-3a and teneurin-3b) and only a single teneurin-2 gene (Tucker et al., 2012). Additionally, the stickleback teneurin-1 gene contains a potential furin cleavage site and NLS in the N-terminal intracellular domain (Tucker et al., 2012). More recently, teneurin-2 expression was reported outside the developing fish embryo in the mature clownfish brain (Baraban et al., 2007) Thus, the discovery of teneurin genes in a few fish species has been fundamental in aiding in the understanding of teneurin conservation and evolution; however, little research has been performed to elucidate the roles of teneurins in fish.
Only a few publications have explored the roles of teneurins in fish (Figure 1C). A recent study demonstrated that teneurin-3 is expressed in retinal ganglion cells (RGCs), amacrine cells (ACs), and tectal neurons in zebrafish embryos, and evidence supports a role of teneurin-3 in shaping the morphological and functional connectivity of RGCs in developing zebrafish embryos (Antinucci et al., 2013). Additionally, teneurin-3 was shown to specify the correct development of functionally and morphologically defined subsets of ACs and RGCs, which is responsible for the formation of a circuit underlying retinal orientation selectivity. Outside of retinal developmental, teneurin-4 appears to be essential in regulating tremor disorders by modifying the intensity of myelin in the brain and the number of small-diameter neuronal axons along the notochord in developing zebrafish (Hor et al., 2015). This study also demonstrated that teneurin-4 regulates motor axon pathfinding, outgrowth, and branching in zebrafish embryos (Hor et al., 2015; Antinucci et al., 2016). Furthermore, reducing or removing functional teneurin-3 has been recently shown to play roles in seizure activity, as knockdown models appear to have enhanced seizure resistance (Baraban et al., 2007; Hortopan et al., 2010). Therefore, evidence supports a fundamental role of both teneurin-3 and teneurin-4 in the development and functionality of nervous system tissue in zebrafish.
It is well established that teneurins have conserved roles in neuronal development across taxa, but recent studies suggest that teneurins can affect the development and functions of non-neuronal tissues in fish. Recent work in clownfish (Amphiprion ocellaris) suggests that teneurin-2 might be important in maturing gonads, as teneurin-2 expression was upregulated in mature gonad tissue of both male and female clownfish (Casas et al., 2016). In fact, teneurin-2 (tenm-2) seems to play a role in the induction of adipocyte markers in humans, as tenm-2 expression is much higher in white adipose tissue compared to brown adipose tissue (Tews et al., 2014). After adipocyte differentiation occurs in humans, expression of tenm-2 drops sharply, however, loss-of-functions studies demonstrated that tenm-2 expression levels alone do not regulate differentiation of adipocytes (Tews et al., 2017). However, tenm-2 loss-of-function in human fat cells led to the expression of brown adipocyte markers, such as UCP1, within white adipocytes, which was corroborated by corresponding mitochondrial respiration rate increases (Tews et al., 2017), suggesting a functional role of teneurin-2 in regulating adipocyte metabolism. Further, removing tenm-2 function resulted in increased basal and cAMP-stimulated leak respiration leading to improved overall oxidative metabolism (Tews et al., 2017). Together, these data suggest that teneurins are likely to play regulatory roles in non-developing tissues and might aid in regulating metabolic functions across taxa. Furthermore, additional processing of teneurins can lead to biological activity of the TCAP that can also regulate metabolism. Little is known about the role teneurins might play in fish metabolism, leading to a wide open area awaiting investigation.
Around the same time that tenm-2 metabolic functions were being elucidated, another group demonstrated that the distal peptide portion of teneurins can also regulate metabolism. This distal portion, termed TCAP, was first discovered in 2004 while searching for corticotrophin-releasing factor (CRF) paralogs (Qian et al., 2004). The TCAP region was identified on the final 3′ exon of the teneurin-3 protein in rainbow trout hypothalamic cDNA and showed characteristics of a bioactive peptide (Qian et al., 2004). TCAP can be independently transcribed or be cleaved from teneurin as a peptide, suggesting it has functional independence from its pro-protein teneurin (Qian et al., 2004; Chand et al., 2013a). Several TCAP peptides homologs (TCAP-1-4) have been identified at the extracellular end of teneurins in a number of species (Qian et al., 2004; Colacci et al., 2005; Wang et al., 2005; Lovejoy et al., 2006; Tan et al., 2011; Chand et al., 2013a, 2014; D’Aquila et al., 2017). TCAP peptides are well conserved across animal taxa (Lovejoy et al., 2009), including teleost fishes (Figures 2A,B). Thus far, single TCAP-1 and -4 orthologs have been identified in zebrafish, stickleback, and pufferfish; while stickleback, pufferfish, and medaka each have known TCAP-3 paralogs (TCAP-3a and TCAP-3b) (Figure 2C). Zebrafish has maintained two TCAP-2 paralogs (TCAP-2a and TCAP-2b) (Figure 2C). Phylogenetic analysis suggests that TCAP-1 and TCAP-4 shara a common ancestor, as do TCAP-2 and TCAP-3 (Figure 2C), which is consistent with previously reported relationships for full-length teneurin proteins (Tucker et al., 2012). The significance and expression profiles of these fish TCAP homologs is currently not known.
Figure 2. Predicted peptide sequences of the TCAP family from fishes. Sequences predicted from predicted full length teneurin proteins on Ensemble. (A) Clustal Omega alignment of TCAP paralogs from the zebrafish (Danio rerio). (B) Alignment of TCAP orthologs from selected teleost species: zebrafish, D. rerio; stickleback, Gasterosteus aculeatus; spotted pufferfish, Tetraodon nigroviridis; clownfish, Amphiprion ocellaris; medaka, Oryzias latipes; rainbow trout, Oncorhynchus mykiss. (C) Predicted TCAP peptide sequences used to generate a Clustal Omega alignment and build this unrooted phylogenetic tree to predict evolutionary relationship of fish TCAP peptides.
Previous work has shown that TCAP shares ∼22% sequence similarity with the CRF superfamily and thus initial studies focused on targeting the roles of TCAP in relation to the stress axis (Chand et al., 2013b; Chen et al., 2013; Erb et al., 2014). Work has shown that TCAP-1 decreases stress-related behaviors in rodents and can block CRF stress-inducing effects (Al Chawaf et al., 2007a,b; Tan et al., 2008, 2009, 2011; Kupferschmidt et al., 2011). These studies established TCAP-1 as a potent anxiolytic in vivo, as rats treated with TCAP plus CRF displayed reduced stress-related behaviors, consistent with decreased anxiety (Al Chawaf et al., 2007b). Additionally, several studies have shown that TCAP-1 treatment can cause cytoskeletal reorganization of neurons in mammals (Bernstein and Bamburg, 2003; Al Chawaf et al., 2007a; Chand et al., 2013a), suggesting at least partial functional conservation in nervous system development and function between teneurins and TCAPs. Thus, further studies applied exogenous TCAP to elucidate its functions outside of stress and have since shown TCAP to be a novel growth and metabolic regulator. For example, exogenous rainbow trout TCAP-3 (rtTCAP-3) stimulated cellular proliferation and cAMP levels in Gn11 neuronal cells in vitro (Qian et al., 2004).
More recently, TCAP-1 was shown to exhibit metabolic effects in rats (Hogg et al., 2018), as TCAP-1 decreased blood glucose 40% in both Wistar rats and in the type II diabetic insulin-insensitive pathological model, Goto-Kakizaki rats (Hogg et al., 2018). Furthermore, TCAP-1 decreased insulin and increased serum glucagon levels, suggesting an effect on glucose metabolism systemically (Hogg et al., 2018). Similarly, TCAP-1 increases glucose uptake, similar to, but independent of, insulin in mouse mHypoE-38 hypothalamic cells, in vitro, with an accompanied decrease in cytosolic calcium levels and increased plasma membrane expression of GLUT3, the primary glucose transporter for neurons (Hogg et al., 2018). More importantly, TCAP-1 increased intracellular ATP concentrations in a dose dependent manner while simultaneously decreasing the levels of pyruvate and lactate, in vitro, suggesting that TCAP can enhance neuronal metabolism via oxidative energy production processes (Hogg et al., 2018).
Furthermore, evolutionary analysis of the TCAP peptide revealed that it predates insulin, which suggests its metabolic functions are highly conserved (Hogg et al., 2018). D’Aquila et al. (2017) showed that TCAP-1 treatment increased contractile behaviors in C. intestinalis, which is an energy dependent behavior. This suggests that TCAP can increase the energy production and metabolism in primitive species, further supporting the notion that TCAP has conserved functions in metabolism. Taken together, these recent studies provide critical insights for the conserved roles of teneurins and TCAPs in metabolism. Unfortunately, there is no data published on the effects of TCAP on metabolism in any fish species. However, it is expected that TCAP peptides likely regulate glucose uptake and enhance energetic efficiency.
It is clear that teneurins play a regulatory role in the developing nervous system, and recent evidence suggests regulatory roles of teneurins in metabolism. Additionally, when liberated, the 40-41-residue, TCAP, can regulate nervous system remodeling (Tan et al., 2012), the stress response of CRF signaling (Tan et al., 2012; Chen et al., 2013), and cellular metabolism. Recent evidence also suggests that TCAP likely regulates energy efficiency in cells allowing for physiological changes outside of the nervous system, such as in skeletal muscle and adipose tissue. The major focus of functional work related to teneurins and TCAPs has been conducted in rodent species and more specifically in nervous system development in rodents. However, as outlined here, more recent work has focused on the high conservation of teneurins and TCAPs functions in development, growth, and metabolism in non-rodent species, including several teleost fish species (Figure 1C). Teneurins, and presumably TCAPs, appears to function in nervous system development and cell morphology and migration in teleosts.
Teleosts are the largest vertebrate group and are dominant in freshwater and marine environments. Due to their worldwide distribution, they have amassed a vast amount of diversity in morphology, ecology, and behavior (Nelson et al., 2016). However, teleosts possess physiological features common to all vertebrates, as well as high genomic conservation, making them attractive models for the study of many biological questions, including the evolution of development, growth, and metabolic efficiency regulation. With this review, we wish to expand the interest in studying the functions of teneurins and TCAPs in relation to functions highlighted here, as well as related metabolic dysfunctions that are common to many human diseases. Areas of specific focus might include calcium transport, ATP production, mitochondrial function, and cell growth regulation.
PB conceived the concept and designed the outline for this mini review, as well as contributed to the writing, reviewing, and editing, and provided final editing and approval of mini review. RR contributed by assisted in designing the outline of the mini review and provided critical intellectual content. JM and KF provided critical intellectual content.
Research reported in this mini review was supported by the National Institute on Aging of the National Institutes of Health under award number P30 AG050886. The content is solely the responsibility of the authors and does not necessarily represent the official views of the National Institutes of Health.
The authors declare that the research was conducted in the absence of any commercial or financial relationships that could be construed as a potential conflict of interest.
Al Chawaf, A., St Amant, K., Belsham, D., and Lovejoy, D. A. (2007a). Regulation of neurite growth in immortalized mouse hypothalamic neurons and rat hippocampal primary cultures by teneurin C-terminal-associated peptide-1. Neuroscience 144, 1241–1254.
Al Chawaf, A., Xu, K., Tan, L., Vaccarino, F. J., Lovejoy, D. A., Rotzinger, S., et al. (2007b). Corticotropin-releasing factor (CRF)-induced behaviors are modulated by intravenous administration of teneurin C-terminal associated peptide-1 (TCAP-1). Peptides 28, 1406–1415.
Antinucci, P., Nikolaou, N., Meyer, M. P., and Hindges, R. (2013). Teneurin-3 specifies morphological and functional connectivity of retinal ganglion cells in the vertebrate visual system. Cell Rep. 5, 582–592. doi: 10.1016/j.celrep.2013.09.045
Antinucci, P., Suleyman, O., Monfries, C., and Hindges, R. (2016). Neural mechanisms generating orientation selectivity in the retina. Curr. Biol. 26, 1802–1815. doi: 10.1016/j.cub.2016.05.035
Baraban, S. C., Dinday, M. T., Castro, P. A., Chege, S., Guyenet, S., Taylor, M. R., et al. (2007). A large-scale mutagenesis screen to identify seizure-resistant zebrafish. Epilepsia 48, 1151–1157. doi: 10.1111/j.1528-1167.2007.01075.x
Baumgartner, S., and Chiquet-Ehrismann, R. (1993). Tena, a Drosophila gene related to tenascin, shows selective transcript localization. Mech. Dev. 40, 165–176. doi: 10.1016/0925-4773(93)90074-8
Baumgartner, S., Martin, D., Hagios, C., and Chiquet-Ehrismann, R. (1994). Tenm, a Drosophila gene related to tenascin, is a new pair-rule gene. EMBO J. 13, 3728–3740. doi: 10.1002/j.1460-2075.1994.tb06682.x
Beckmann, J., Schubert, R., Chiquet-Ehrismann, R., and Muller, D. J. (2013). Deciphering teneurin domains that facilitate cellular recognition, cell-cell adhesion, and neurite outgrowth using atomic force microscopy-based single-cell force spectroscopy. Nano Lett. 13, 2937–2946. doi: 10.1021/nl4013248
Bernstein, B. W., and Bamburg, J. R. (2003). Actin-ATP hydrolysis is a major energy drain for neurons. J. Neurosci. 23, 1–6. doi: 10.1523/JNEUROSCI.23-01-00002.2003
Casas, L., Saborido-Rey, F., Ryu, T., Michell, C., Ravasi, T., Irigoien, X., et al. (2016). Sex change in clownfish: molecular insights from transcriptome analysis. Sci. Rep. 6:35461. doi: 10.1038/srep35461
Chand, D., Casatti, C. A., de Lannoy, L., Song, L., Kollara, A., Barsyte-Lovejoy, D., et al. (2013a). C-terminal processing of the teneurin proteins: independent actions of a teneurin C-terminal associated peptide in hippocampal cells. Mol. Cell. Neurosci. 52, 38–50. doi: 10.1016/j.mcn.2012.09.006
Chand, D., de Lannoy, L., Tucker, R., and Lovejoy, D. A. (2013b). Origin of chordate peptides by horizontal protozoan gene transfer in early metazoans and protists: evolution of the teneurin C-terminal associated peptides (TCAP). Gen. Comp. Endocrinol. 188, 144–150. doi: 10.1016/j.ygcen.2013.02.006
Chand, D., Colacci, M., Dixon, K., Kollara, A., Brown, T. J., Lovejoy, D. A., et al. (2014). C-terminal region of teneurin-1 co-localizes with the dystroglycan complex in adult mouse testes and regulates testicular size and testosterone production. Histochem. Cell Biol. 141, 191–211. doi: 10.1007/s00418-013-1154-1
Chen, Y., Xu, M., De Almeida, R., and Lovejoy, D. A. (2013). Teneurin C-terminal associated peptides (TCAP): modulators of corticotropin-releasing factor (CRF) physiology and behavior. Front. Neurosci. 7:166. doi: 10.3389/fnins.2013.00166
Colacci, M., De Almeida, R., Chand, D., Lovejoy, S. R., Sephton, D., Vercaemer, B., et al. (2005). Characterization of the teneurin C-terminal associated peptide (TCAP) in the vase tunicate, ciona intestinalis: a novel peptide system associated with energy metabolism and reproduction. Gen. Comp. Endocrinol. 216, 161–170. doi: 10.1016/j.ygcen.2015.01.021
D’Aquila, A. L., Hsieh, A. H., Hsieh, A. H., De Almeida, R., Lovejoy, S. R., Lovejoy, D. A., et al. (2017). Expression and actions of corticotropin-releasing factor/diuretic hormone-like peptide (CDLP) and teneurin C-terminal associated peptide (TCAP) in the vase tunicate, Ciona intestinalis: antagonism of the feeding response. Gen. Comp. Endocrinol. 246, 105–115. doi: 10.1016/j.ygcen.2016.06.015
Drabikowski, K., Trzebiatowska, A., and Chiquet-Ehrismann, R. (1999). ten-1, an essential gene for germ cell development, epidermal morphogenesis, gonad migration, and neuronal pathfinding in Caenorhabditis elegans. Dev. Biol. 282, 27–38. doi: 10.1016/j.ydbio.2005.02.017
Erb, S., McPhee, M., Brown, Z. J., Kupferschmidt, D. A., Song, L., Lovejoy, D. A., et al. (2014). Repeated intravenous administrations of teneurin-C terminal associated peptide (TCAP)-1 attenuates reinstatement of cocaine seeking by corticotropin-releasing factor (CRF) in rats. Behav. Brain Res. 269, 1–5. doi: 10.1016/j.bbr.2014.04.013
Fascetti, N., and Baumgartner, S. (2002). Expression of Drosophila Ten-a, a dimeric receptor during embryonic development. Mech. Dev. 114, 197–200. doi: 10.1016/S0925-4773(02)00055-2
Hogg, D. W., Chen, Y., D’Aquila, A. L., Xu, M., Husic, M., Tan, L. A., et al. (2018). A novel role of the corticotrophin-releasing hormone regulating peptide, teneurin C-terminal associated peptide 1, on glucose uptake into the brain. J. Neuroendocrinol. 30:e12579. doi: 10.1111/jne.12579
Hor, H., Francescatto, L., Bartesaghi, L., Ortega-Cubero, S., Kousi, M., Lorenzo-Betancor, O., et al. (2015). Missense mutations in TENM4, a regulator of axon guidance and central myelination, cause essential tremor. Hum. Mol. Genet. 24, 5677–5686. doi: 10.1093/hmg/ddv281
Hortopan, G. A., Dinday, M. T., and Baraban, S. C. (2010). Zebrafish as a model for studying genetic aspects of epilepsy. Dis. Model Mech. 3, 144–148. doi: 10.1242/dmm.002139
Ishii, K., Suzuki, N., Mabuchi, Y., Ito, N., Kikura, N., Fukada, S., et al. (2015). Muscle satellite cell protein teneurin-4 regulates differentiation during muscle regeneration. Stem Cells 33, 3017–3027. doi: 10.1002/stem.2058
Kawasaki, H., and Kretsinger, R. H. (1994). Calcium-binding proteins. 1: ef-hands. Protein Profile 1, 343–517.
Kupferschmidt, D. A., Lovejoy, D. A., Rotzinger, S., and Erb, S. (2011). Teneurin C-terminal associated peptide-1 blocks the effects of corticotropin-releasing factor on reinstatement of cocaine seeking and on cocaine-induced behavioural sensitization. Br. J. Pharmacol. 162, 574–583. doi: 10.1111/j.1476-5381.2010.01055.x
Levine, A., Bashan-Ahrend, A., Budai-Hadrian, O., Gartenberg, D., Menasherow, S., Wides, R., et al. (1994). Odd Oz: a novel Drosophila pair rule gene. Cell 77, 587–598. doi: 10.1016/0092-8674(94)90220-8
Li, J., Shalev-Benami, M., Sando, R., Jiang, X., Kibrom, A., Wang, J., et al. (2018). Structural basis for teneurin function in circuit-wiring: a toxin motif at the synapse. Cell 173, 735–748.e15. doi: 10.1016/j.cell.2018.03.036
Lossie, A. C., Nakamura, H., Thomas, S. E., and Justice, M. J. (2005). Mutation of l7Rn3 shows that Odz4 is required for mouse gastrulation. Genetics 169, 285–299. doi: 10.1534/genetics.104.034967
Lovejoy, D. A., Al Chawaf, A., and Cadinouche, M. Z. (2006). Teneurin C-terminal associated peptides: an enigmatic family of neuropeptides with structural similarity to the corticotropin-releasing factor and calcitonin families of peptides. Gen. Comp. Endocrinol. 148, 299–305. doi: 10.1016/j.ygcen.2006.01.012
Lovejoy, D. A., Rotzinger, S., and Barsyte-Lovejoy, D. (2009). Evolution of complementary peptide systems: teneurin C-terminal-associated peptides and corticotropin-releasing factor superfamilies. Ann. N. Y. Acad. Sci. 1163, 215–220. doi: 10.1111/j.1749-6632.2008.03629.x
Mieda, M., Kikuchi, Y., Hirate, Y., Aoki, M., and Okamoto, H. (1999). Compartmentalized expression of zebrafish ten-m3 and ten-m4, homologues of the Drosophila ten(m)/odd Oz gene, in the central nervous system. Mech. Dev. 87, 223–227. doi: 10.1016/S0925-4773(99)00155-0
Minet, A. D., and Chiquet-Ehrismann, R. (2000). Phylogenetic analysis of teneurin genes and comparison to the rearrangement hot spot elements of E. coli. Gene 257, 87–97. doi: 10.1016/S0378-1119(00)00388-7
Minet, A. D., Rubin, B. P., Tucker, R. P., Baumgartner, S., and Chiquet-Ehrismann, R. (1999). Teneurin-1, a vertebrate homologue of the Drosophila pair-rule gene ten-m, is a neuronal protein with a novel type of heparin-binding domain. J. Cell Sci. 112( Pt 12), 2019–2032.
Mosca, T. J. (2015). On the Teneurin track: a new synaptic organization molecule emerges. Front. Cell Neurosci. 9:204. doi: 10.3389/fncel.2015.00204
Nelson, J. S., Grande, T., and Wilson, M. V. H. (2016). Fishes of the World. Hoboken, N J: John Wiley & Sons. doi: 10.1002/9781119174844
Nunes, S. M., Ferralli, J., Choi, K., Brown-Luedi, M., Minet, A. D., Chiquet-Ehrismann, R., et al. (2005). The intracellular domain of teneurin-1 interacts with MBD1 and CAP/ponsin resulting in subcellular codistribution and translocation to the nuclear matrix. Exp. Cell Res. 305, 122–132. doi: 10.1016/j.yexcr.2004.12.020
Oohashi, T., Zhou, X. H., Feng, K., Richter, B., Morgelin, M., Perez, M. T., et al. (1999). Mouse ten-m/Odz is a new family of dimeric type II transmembrane proteins expressed in many tissues. J. Cell Biol. 145, 563–577. doi: 10.1083/jcb.145.3.563
Otaki, J. M., and Firestein, S. (1999). Neurestin: putative transmembrane molecule implicated in neuronal development. Dev. Biol. 212, 165–181. doi: 10.1006/dbio.1999.9310
Qian, X., Barsyte-Lovejoy, D., Wang, L., Chewpoy, B., Gautam, N., Al Chawaf, A., et al. (2004). Cloning and characterization of teneurin C-terminus associated peptide (TCAP)-3 from the hypothalamus of an adult rainbow trout (Oncorhynchus mykiss). Gen. Comp. Endocrinol. 137, 205–216. doi: 10.1016/j.ygcen.2004.02.007
Rubin, B. P., Tucker, R. P., Martin, D., and Chiquet-Ehrismann, R. (1999). Teneurins: a novel family of neuronal cell surface proteins in vertebrates, homologous to the Drosophila pair-rule gene product Ten-m. Dev. Biol. 216, 195–209. doi: 10.1006/dbio.1999.9503
Scholer, J., Ferralli, J., Thiry, S., and Chiquet-Ehrismann, R. (2015). The intracellular domain of teneurin-1 induces the activity of microphthalmia-associated transcription factor (MITF) by binding to transcriptional repressor HINT1. J. Biol. Chem. 290, 8154–8165. doi: 10.1074/jbc.M114.615922
Tan, L. A., Al Chawaf, A., Vaccarino, F. J., Boutros, P. C., and Lovejoy, D. A. (2011). Teneurin C-terminal associated peptide (TCAP)-1 modulates dendritic morphology in hippocampal neurons and decreases anxiety-like behaviors in rats. Physiol. Behav. 104, 199–204. doi: 10.1016/j.physbeh.2011.03.015
Tan, L. A., Chand, D., De Almeida, R., Xu, M., De Lannoy, L., Lovejoy, D. A., et al. (2012). Modulation of neuroplastic changes and corticotropin-releasing factor-associated behavior by a phylogenetically ancient and conserved peptide family. Gen. Comp. Endocrinol. 176, 309–313. doi: 10.1016/j.ygcen.2011.11.011
Tan, L. A., Xu, K., Vaccarino, F. J., Lovejoy, D. A., and Rotzinger, S. (2008). Repeated intracerebral teneurin C-terminal associated peptide (TCAP)-1 injections produce enduring changes in behavioral responses to corticotropin-releasing factor (CRF) in rat models of anxiety. Behav. Brain Res. 188, 195–200. doi: 10.1016/j.bbr.2007.10.032
Tan, L. A., Xu, K., Vaccarino, F. J., Lovejoy, D. A., and Rotzinger, S. (2009). Teneurin C-terminal associated peptide (TCAP)-1 attenuates corticotropin-releasing factor (CRF)-induced c-Fos expression in the limbic system and modulates anxiety behavior in male Wistar rats. Behav. Brain Res. 201, 198–206. doi: 10.1016/j.bbr.2009.02.013
Tews, D., Fromme, T., Keuper, M., Hofmann, S. M., Debatin, K. M., Klingenspor, M., et al. (2017). Teneurin-2 (TENM2) deficiency induces UCP1 expression in differentiating human fat cells. Mol. Cell. Endocrinol. 443, 106–113. doi: 10.1016/j.mce.2017.01.015
Tews, D., Schwar, V., Scheithauer, M., Weber, T., Fromme, T., Klingenspor, M., et al. (2014). Comparative gene array analysis of progenitor cells from human paired deep neck and subcutaneous adipose tissue. Mol. Cell. Endocrinol. 395, 41–50. doi: 10.1016/j.mce.2014.07.011
Topf, U., and Chiquet-Ehrismann, R. (2011). Genetic interaction between Caenorhabditis elegans teneurin ten-1 and prolyl 4-hydroxylase phy-1 and their function in collagen IV-mediated basement membrane integrity during late elongation of the embryo. Mol. Biol. Cell 22, 3331–3343. doi: 10.1091/mbc.E10-10-0853
Trzebiatowska, A., Topf, U., Sauder, U., Drabikowski, K., and Chiquet-Ehrismann, R. (2008). Caenorhabditis elegans teneurin, ten-1, is required for gonadal and pharyngeal basement membrane integrity and acts redundantly with integrin ina-1 and dystroglycan dgn-1. Mol. Biol. Cell 19, 3898–3908. doi: 10.1091/mbc.E08-01-0028
Tucker, R. P., Beckmann, J., Leachman, N. T., Scholer, J., and Chiquet-Ehrismann, R. (2012). Phylogenetic analysis of the teneurins: conserved features and premetazoan ancestry. Mol. Biol. Evol. 29, 1019–1029. doi: 10.1093/molbev/msr271
Tucker, R. P., and Chiquet-Ehrismann, R. (2006). Teneurins: a conserved family of transmembrane proteins involved in intercellular signaling during development. Dev. Biol. 290, 237–245. doi: 10.1016/j.ydbio.2005.11.038
Tucker, R. P., Kenzelmann, D., Trzebiatowska, A., and Chiquet-Ehrismann, R. (2007). Teneurins: transmembrane proteins with fundamental roles in development. Int. J. Biochem. Cell Biol. 39, 292–297. doi: 10.1016/j.biocel.2006.09.012
Tucker, R. P., Martin, D., Kos, R., and Chiquet-Ehrismann, R. (2000). The expression of teneurin-4 in the avian embryo. Mech. Dev. 98, 187–191. doi: 10.1016/S0925-4773(00)00444-5
Wang, L., Rotzinger, S., Al Chawaf, A., Elias, C. F., Barsyte-Lovejoy, D., Qian, X., et al. (2005). Teneurin proteins possess a carboxy terminal sequence with neuromodulatory activity. Brain Res. Mol. Brain Res. 133, 253–265. doi: 10.1016/j.molbrainres.2004.10.019
Keywords: teneurin, teneurin C-terminal associated peptide, teneurin C-terminal associated peptides, fish, metabolism
Citation: Reid RM, Freij KW, Maples JC and Biga PR (2019) Teneurins and Teneurin C-Terminal Associated Peptide (TCAP) in Metabolism: What’s Known in Fish? Front. Neurosci. 13:177. doi: 10.3389/fnins.2019.00177
Received: 01 October 2018; Accepted: 14 February 2019;
Published: 05 March 2019.
Edited by:
Richard P. Tucker, University of California, Davis, United StatesReviewed by:
Hélène Volkoff, Memorial University of Newfoundland, CanadaCopyright © 2019 Reid, Freij, Maples and Biga. This is an open-access article distributed under the terms of the Creative Commons Attribution License (CC BY). The use, distribution or reproduction in other forums is permitted, provided the original author(s) and the copyright owner(s) are credited and that the original publication in this journal is cited, in accordance with accepted academic practice. No use, distribution or reproduction is permitted which does not comply with these terms.
*Correspondence: Peggy R. Biga, cGVnYmlnYUB1YWIuZWR1
Disclaimer: All claims expressed in this article are solely those of the authors and do not necessarily represent those of their affiliated organizations, or those of the publisher, the editors and the reviewers. Any product that may be evaluated in this article or claim that may be made by its manufacturer is not guaranteed or endorsed by the publisher.
Research integrity at Frontiers
Learn more about the work of our research integrity team to safeguard the quality of each article we publish.