- 1Department of Psychiatry, University of Michigan, Ann Arbor, MI, United States
- 2Nemours/Alfred I. DuPont Hospital for Children, Wilmington, DE, United States
- 3Department of Communication Sciences and Disorders, University of Delaware, Newark, DE, United States
- 4Department of Communication Sciences and Disorders, Georgia State University, Atlanta, GA, United States
Stuttering is a neurodevelopmental disorder that manifests as frequent disruptions in the flow of speech, affecting 1% of adults. Treatments are limited to behavioral interventions with variable success and high relapse rates, particularly in adults. However, even in severe cases, fluency can be temporarily induced during conditions in which the speaker synchronizes his speech with external rhythmic cues, such as when reading in unison (choral speech) or with a metronome. Non-invasive neuromodulation techniques such as transcranial direct current stimulation (tDCS) have shown promise in augmenting the effects of behavioral treatment during motor and speech/language rehabilitation, but only one study to date has examined behavioral modulatory effects of tDCS in the context of stuttering. Using high-definition (HD)-tDCS electrodes, which improves focality of stimulation relative to conventional tDCS, we investigated the effects of tDCS on speech fluency and brain activation in 14 adults who stutter (AWS). Either anodal or sham stimulation was delivered on separate days over left supplementary motor area (SMA). During stimulation, participants read aloud in sync with a metronome. Measures of speech fluency and brain activity functional magnetic resonance imaging (fMRI) were collected before and after stimulation. No significant differences in brain activity or speech fluency were found when comparing active and sham stimulation. However, stuttering severity significantly modulated the effect of stimulation: active stimulation attenuated the atypically strong association between stuttering severity and right thalamocortical network activity, especially in more severe speakers. These preliminary results warrant additional research into potential application of HD-tDCS to modulate speech motor networks to enhance fluency in stuttering.
Introduction
Adults with persistent developmental stuttering report life-long struggles in speech communication, leading to detrimental effects on their social, emotional, and vocational well-being (Craig et al., 2009; Yaruss, 2010). Conventional behavioral speech therapy requires a considerable amount of effort (>100 h) and still over 70% of those that undergo therapy experience relapse of stuttering symptoms (Andrews et al., 1980; Craig and Hancock, 1995; Craig et al., 1996; Hancock et al., 1998). Success with electronic devices using delayed auditory feedback is variable and short-lived (Foundas et al., 2013), and pharmacological treatments have variable effects across individuals who stutter and are associated with unwanted side effects (Bothe et al., 2006; Maguire et al., 2010). Therefore, there is a critical need for developing therapeutic interventions that result in long-term enhancement of speech fluency in people who stutter (PWS). There is accumulating evidence of: (a) subtle neural differences in PWS; and (b) that the use of non-invasive brain stimulation improves motor and language performance in individuals with neurological speech and motor impairments and healthy individuals. The present study investigated the effects of brain stimulation (via high definition transcranial direct current stimulation; HD-tDCS) on neural activity and speech fluency in adults who stutter (AWS).
Corroborating predictions based on a neurobiologically plausible model of speech production [Gradient Order Directions into Velocities of Articulators (GODIVA); Bohland et al., 2010], accumulating empirical findings have suggested that the core deficit in stuttering may stem from disruption in the basal ganglia thalamocortical (BGTC) network, specifically in connections among the basal ganglia, supplementary motor area (SMA), and ventral premotor cortex (vPMC; Civier et al., 2013). The disruption in the BGTC network affects coordination of precisely timed movement initiation (SMA), activation of speech sounds (vPMC), and sequencing of sounds (putamen), all needed for speech fluency (Bohland et al., 2010; Civier et al., 2013). The SMA is critical in planning complex movement routines, including those that are required for fluent speech production, that are internally timed and initiated, rather than in response to external cues (Cunnington et al., 1996; Packman et al., 2007). PWS demonstrate difficulty in preparing and controlling precisely-timed complex movements including speech, poor performance on non-speech behavioral tasks that involve timing and rhythm and attenuated functional connectivity among BGTC regions (Packman and Onslow, 2002; Packman et al., 2007; Lu et al., 2009, 2010; Chang and Zhu, 2013; Wieland et al., 2015; Chang et al., 2016). Interestingly, most PWS exhibit temporarily increased fluency during externally paced conditions such as choral speech or metronome-paced speech (Park and Logan, 2015). They also show more “normalized” brain activation patterns during such conditions (i.e., similar to nonstuttering speakers; De Nil et al., 2003; Preibisch et al., 2003; Neumann et al., 2005; Giraud et al., 2008; Kell et al., 2009; Toyomura et al., 2011, 2015).
As a treatment, however, such techniques remain inadequate, as any improvements in speech fluency are short-lived (Kell et al., 2009) and the benefits often are outweighed by reduction in speech naturalness. To date, neuroscience-based treatments for stuttering have been limited. Techniques that exploit the principles of neuroplasticity, such as neuromodulation with tDCS, may offer new insights. This technique is safe, well-tolerated, non-invasive, inexpensive, and portable (Bikson et al., 2016) compared to other brain stimulation techniques such as transcranial magnetic stimulation (TMS). Additionally, tDCS readily lends itself to double-blinded, sham-controlled studies suitable for clinical trials (Woods et al., 2016). Conventional tDCS sponge electrodes are large (e.g., 35 cm2), resulting in diffuse current spread, but focality has recently been improved with high definition (HD) electrodes, which were used in the present research (Nitsche et al., 2007; Datta et al., 2008, 2009; Kuo et al., 2013). Stimulation via HD-tDCS can either increase or decrease brain activity using a weak constant electrical current (1–2 mA). Unlike TMS, which can induce neural firing, tDCS affects the resting membrane potential through the modulation of cellular-level changes, including sodium and calcium-dependent channels and NMDA receptor activity, thereby promoting long-term potentiation/depression (LTP/LTD) like effects (Stagg et al., 2009; Fritsch et al., 2010; Bolognini et al., 2011; Stagg and Nitsche, 2011).
Brain stimulation in conjunction with functional magnetic resonance imaging (fMRI) can enable researchers to examine any changes in brain activity in the targeted region and functionally connected regions as a result of the stimulation (Baudewig et al., 2001; Lang et al., 2005; Jang et al., 2009; Stagg et al., 2009; Keeser et al., 2011; Polanía et al., 2012; Pereira et al., 2013; Hampstead et al., 2014; Liew et al., 2014; Weber et al., 2014; Holland et al., 2016). HD-tDCS has been used in previous studies to provide functional improvement in language, motor skill learning, verbal fluency, speech reaction time, naming, numerical processing abilities, spelling, and learning an artificial grammar (Iyer et al., 2005; Sparing et al., 2008; Dockery et al., 2009; Reis et al., 2009; Baker et al., 2010 ; Fertonani et al., 2010; de Vries et al., 2010; Cattaneo et al., 2011; Fiori et al., 2011; Fridriksson et al., 2011; Madhavan and Shah, 2012; Richardson et al., 2014; Tsapkini et al., 2014; Vestito et al., 2014; Hartwigsen, 2015; Malyutina and den Ouden, 2015; Allman et al., 2016; Cappon et al., 2016; Hashemirad et al., 2016; Woods et al., 2016). Effects of stimulation are not always uniform across participants even in a single study, which suggests the existence of “good” vs. “poor” responders. Clinical measures of initial severity, baseline task performance, state and trait characteristics, and other individual differences often predict response to treatment (Berryhill and Jones, 2012; Tseng et al., 2012; Sarkar et al., 2014; Benwell et al., 2015; Learmonth et al., 2015; Hsu et al., 2016). Such effects may mask significant findings at an overall or group level, only to be revealed in more nuanced planned analyses.
Only two tDCS studies have been published to date relevant to the treatment of stuttering. A recent study (Chesters et al., 2018) paired traditional tDCS targeting left IFG with fluency-inducing speech (i.e., metronome-timed speech, choral speech) in a randomized, double-blind, controlled study. Thirty AWS received either sham or active tDCS for 20 min on five consecutive days. Speech fluency during reading and conversation was measured before, during, and at 1 and 6 weeks after treatment. The group who received active stimulation showed significant decrease in stuttering at 1 week after treatment, compared to the training-only (sham stimulation) group. This was maintained at 6 weeks, but only in the reading condition. These results provide initial support for using non-invasive brain stimulation to improve speech fluency in AWS when applied consecutively for several days. Yada et al. (2019) found that cathodal traditional tDCS applied to right Broca’s area decreased stuttering, although the study design involved multiple block of stimulation per day with only a short washout period between blocks. Nevertheless, the positive impact on fluency lends additional support for using non-invasive brain stimulation in stuttering treatment.
Similar to Chesters et al. (2018), we tested the potential augmentative effects of pairing tDCS with metronome-timed speech on speech fluency and brain activity in regions within and connected to the BGTC core timing network in AWS. Our study differed from Chesters et al. (2018) in several ways: (1) we targeted the left SMA compared to the left IFG that was targeted in their study; (2) we used HD electrodes, which improves focality of stimulation; (3) we stimulated at 1.5 mA compared to 1 mA in their study; (4) we used a within-subject rather than a between-subject design; (5) we collected fMRI before and after the tDCS sessions to examine any changes in brain function associated with brain stimulation; and (6) our study was preliminary in nature, only including one session each per subject of active and sham stimulation. We had two primary research questions. First, can speech-related brain activity in AWS be modulated by a single dose of anodal HD-tDCS targeting SMA? This is a crucial first step in developing an empirical basis for further study of the use of neuromodulation as an augmentative agent in stuttering therapy. We expected that compared to sham stimulation (i.e., training alone with the metronome-timed speech), anodal stimulation over left SMA would increase activity in the SMA, left vPMC/IFG, basal ganglia regions. Second, does stuttering severity modulate the effects of stimulation on brain activity? We hypothesized that stimulation would help reduce strong correlations between hyperactive brain areas and stuttering while enhancing activity in brain areas negatively correlated with stuttering severity.
Materials and Methods
Participants
We recruited 19 AWS to participate in this study. Of those 19, data from five were discarded from the final analyses due to: falling asleep during the MRI (n = 1), attrition related to time requirements of the study (n = 1), different MRI protocol used (n = 2), and incidental finding during MRI (n = 1). Thus, the final group included 14 AWS (three females) with a mean age of 22.6 years (range 18–46). All participants were monolingual, native English speakers with no history of psychiatric, developmental, cognitive, or speech and language disorders other than stuttering. All participants reported normal hearing and tested within normal limits on expressive and receptive language tests (Peabody Picture Vocabulary Test 4th edition; Expressive Vocabulary Test 2nd edition). Severity scores from the SSI-4 ranged from very mild (10) to very severe (41), while %SLD ranged from 2% to 32%. All participants reported receiving stuttering treatment, with duration of treatment ranging from a few days to a few years, primarily during their school years, but none received therapy within 1 year of study participation. The protocol was approved by the Institutional Review Boards of the University of Michigan Medical School (IRBMED). All subjects gave written informed consent in accordance with the Declaration of Helsinki.
Study Design
This study employed a within-subjects, counterbalanced, sham-controlled, double-blind design over three visits (Figure 1). Visit 1 included obtaining informed consent, administering speech and language tests, and practicing the experimental tasks for the subsequent visits. The protocol was identical for visits 2 and 3, which were exactly 1 week apart, only the stimulation condition changed. This design allowed each subject to be his or her own control. Participants were randomized into one of two groups: one group received active stimulation on visit 2 and sham stimulation visit 3, with the order reversed in the other group (see “HD-tDCS procedure” section below for details on active and sham stimulation). fMRI data were collected before and after the tDCS session to assess effects of stimulation on brain activity. Speech samples were also collected before and after stimulation to assess effects of stimulation on speech fluency. Stuttering severity was assessed off-line by a certified SLP using the Stuttering Severity Instrument [(SSI-4); Riley and Bakker, 2009] who was blind to visit number and before/after stimulation.
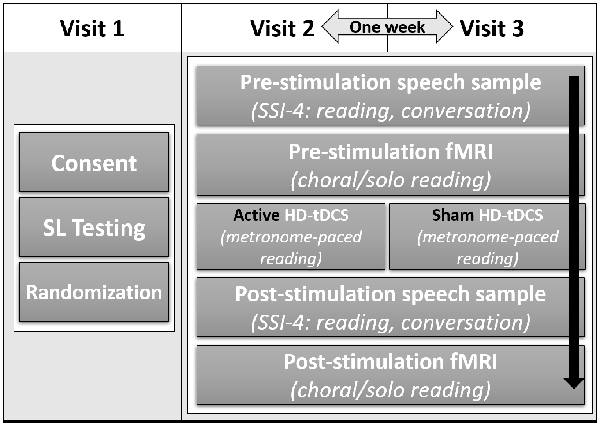
Figure 1. Study design showing group assignment, timeline, and task order for each visit. After consent and speech and language testing, participants were randomly assigned to receive active stimulation on visit 2 and sham stimulation on visit 3, or the reverse. All participants received active and sham stimulation on separate visits. Aside from the type of stimulation, visits 2 and 3 consisted of the same tasks in the same order for all participants.
HD-tDCS Procedure
A 4 × 1 HD-tDCS adapter connected to a 1 × 1 Clinical Trial system (Soterix Medical Inc., New York, NY, USA) was used to deliver active (anodal) and sham stimulation. Optimal electrode configuration for stimulation of left SMA was determined using HD-ExploreTM software (Soterix Medical Inc.,) modeling software that provides the predicted electrical current flow within the brain during stimulation (Figure 2). Ag/AgCl sintered ring electrodes were positioned within electrode holders filled with electrolyte gel in a head cap, which uses the International 10–20 System for EEG to label electrode positions. We centered position Cz on the head at the halfway point between the nasion and the inion longitudinally and left to right pre-auricular areas laterally. Stimulation condition delivery was controlled by a six-digit numeric code input into the stimulation device that either delivered active or sham stimulation. Further, the experimenter who entered the code into the device was blind with regard to which type of stimulation it initiated. During active stimulation, the current was ramped up over 30 s, maintained at 1.5 mA for the rest of the session, and automatically ramped down at the end of the 20-min session. The stimulation intensity in the present study is well within the typical safe and tolerable range used in human studies (Bikson et al., 2016; Woods et al., 2016). For sham stimulation, the current was automatically ramped up and down over the first 30 s to prevent actual stimulation of the target region. The current also was ramped up and back down 30 s prior to the end of the 20-min session, consistent with the literature, to assist with participant blinding (but see Richardson et al., 2014; Garnett and den Ouden, 2015; Woods et al., 2016; Fonteneau et al., 2019; for other methods of sham delivery). During stimulation participants read aloud while pacing each syllable to the beat of a metronome. The metronome speed was set at the maximum comfortable rate for each participant.
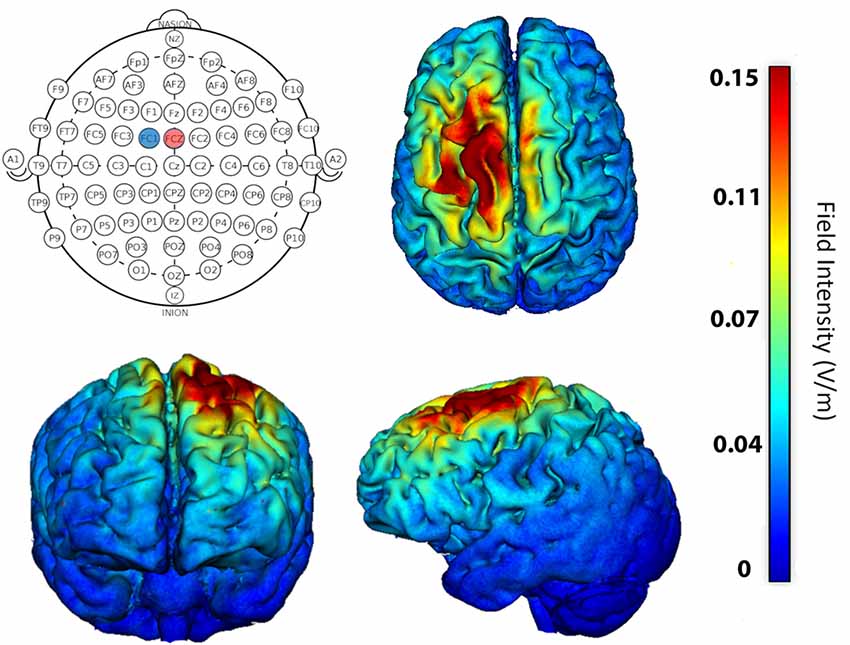
Figure 2. Stimulation location was determined using software that models current flow in the brain. For maximum focality stimulation of left supplementary motor area (SMA), the anode (+1.5 mA) was placed at FCZ (red circle) and cathode (−1.5 mA) at FC1 (blue circle). Resulting predicted field potentials and extent of current flow within the brain is shown on axial, coronal, and sagittal brains.
fMRI Procedure
The protocol for each fMRI session (i.e., before and after stimulation in both visits) was the same. Participants read aloud in two conditions: choral reading and solo reading. During choral reading, participants synchronized their reading with an audio recording (via earphones) of the same passage presented on the screen. During solo reading, participants read at their natural speech rate and backwards speech was presented in the earphones. This was designed to minimize differences in background auditory feedback between the conditions. In each of four 7-min functional runs, four 30-s blocks of solo reading and four blocks of choral reading were presented in alternating order. Scans were acquired using a 3T GE MRI scanner (MR 750). A standard echoplanar (EPI) pulse sequence was used, with the following parameters: recovery time (TR) = 2 s; voxel size = 3.4 × 3.4 × 4 mm; 37 interleaved sagittal slices; acceleration factor = 2. In addition, high-resolution structural images were acquired at the beginning of each scanning session using spoiled gradient-recalled acquisition in steady-state (SPGR) imaging (flip angle = 15°, FOV = 256 mm, 1 mm slice thickness).
fMRI Data Analysis
SPM121 was used for fMRI data preprocessing and statistical analysis unless specified otherwise. For each participant, functional images were corrected for differences in slice acquisition timings. Anatomical scans and functional volumes were co-registered to the first volume of the first scan using rigid body rotation. Functional scans were concatenated and de-noised using a strategy detailed in our previous publication (Xu et al., 2014). This de-noising strategy is based on spatial Independent Component Analysis (sICA), a signal un-mixing algorithm, and has been demonstrated to be able to remove fMRI artifacts associated with continuous speech production (AbdulSabur et al., 2014). De-noised functional scans were normalized to MNI space and spatially smoothed with a 6 mm FWHM kernel. Each participant’s preprocessed data were analyzed using general linear model (GLM) implemented in SPM12. Speaking conditions (choral and solo) at each stimulation condition (active and sham) were modeled with separate regressors. Individual beta estimates of each condition and participant’s SSI score as a covariate were entered into group-level analysis. Pairwise t-tests were used to compare between conditions. Family-wise errors were controlled using voxel-wise height threshold p < 0.001 and cluster-size threshold k > 12 voxels, which corresponded to a corrected p < 0.05. The cluster-size threshold was determined by AFNI 3dClustSim (version 17.2.13) with non-Gaussian auto-correlation function (-acf option; Cox et al., 2017).
Results
Effects of Anodal Stimulation on Brain Activity During Continuous Speaking
The first goal of this study was to examine the effects of HD-tDCS on brain activity during continuous speech. We contrasted the brain activity after active stimulation with that after sham stimulation, resulting in the (Active-Sham) contrast. For the purposes of this study, and due to lack of differential neural activity in the two speaking conditions, we report results with choral speech and solo speech combined: [active (choral+solo)] − [sham (choral+solo)]. Results for this analysis did not reveal any clusters that reached the pre-set significance threshold.
Modulatory Effects of Stuttering Severity
The second goal of the study was to determine if stuttering severity modulated the effects of stimulation on brain activity during speech. Given the range of severity in our initial 14 subjects, we excluded three subjects whose SSI scores were 10 or below (note: we also ran the analysis from “Effects of Anodal Stimulation on Brain Activity During Continuous Speaking” section excluding these same subjects, which did not change the results of that analysis). In this analysis of 11 subjects, the contrast involved subtracting the severity-modulated activation during sham from that during active. This leaves us with the change in relationship between severity and neural activation due to active stimulation. In other words, these are the regions that exhibited a differential modulatory effect of severity in speech-related brain regions. Table 1 and Figure 3 shows modulatory effects of stuttering severity on brain activity during speaking (natural and fluent conditions combined). Two clusters showed a differential modulatory effect of stuttering severity after active vs. sham stimulation for the speaking conditions combined: the right posterior central gyrus and the right thalamus.
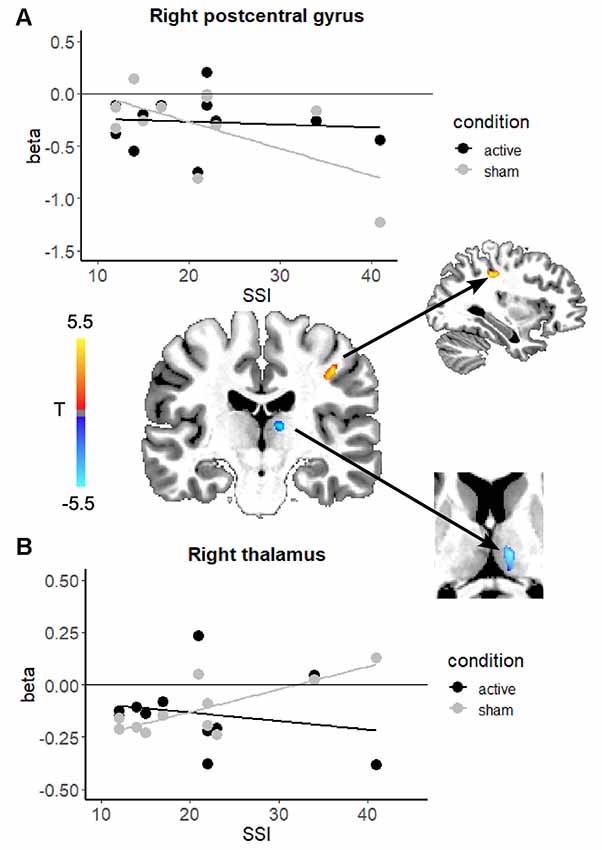
Figure 3. Scatterplots showing the relationship between brain activation and stuttering severity (SSI-4 scores) after active stimulation (black) and after sham stimulation (gray) the right postcentral gyrus (A) and the right thalamus (B). In the center, the change in the relationship between stuttering severity and brain activity in each region is overlaid on an example individual anatomical image. In both regions, active stimulation attenuated the relationship between severity and brain activation. Color blobs represent the overall direction of the change in correlation: in the right postcentral gyrus the relationship became less negative, while in the right thalamus the relationship became less positive.
Effects of Stimulation on Speech Fluency
During the reading portion, speech fluency significantly increased (%SLD decreased) following both active and sham stimulation. The %SLD before active stimulation (M = 7.52) decreased after stimulation (M = 3.97; p = 0.022). The %SLD before sham stimulation (M = 6.83) decreased after sham stimulation (M = 3.97; p = 0.027). Active stimulation had a greater effect on %SLD, resulting in a decrease of 3.55% compared to 2.86% after sham stimulation. There were no significant effects of stimulation or training alone (sham) on speech fluency during the conversation portion of the speech sample.
Discussion
As a preliminary window into the possible therapeutic use of brain stimulation to augment the effects of speech therapy, we investigated, for the first time, the effects of focal neuromodulation using HD-tDCS on speech fluency and brain activity during continuous speech in AWS. We expected that compared to sham stimulation, active stimulation would increase activity of areas functionally connected to SMA, such as BGTC network areas, in turn enhancing speech timing and thus fluency. Namely, that traditionally underactive regions (e.g., left IFG, basal ganglia) would show enhanced activity after anodal stimulation. Results from the main effect of active-sham stimulation analysis did not support our hypotheses. Any changes in brain activity or functional connectivity as a result of active stimulation of left SMA did not differ significantly from that observed in the sham condition.
There are at least three potential explanations for the lack of significant effects in this first analysis. First, due to the relatively small sample size, it is possible that we did not have enough statistical power to detect differences. However, even when examining the data at subthreshold there was very little difference between active and sham stimulation. Second, the SMA may not have been an ideal choice as a target for stimulation. Although SMA plays a critical role within the BGTC network in initiating complex motor sequences and has major connections with the putamen as well as the IFG, it is possible that the timing (as opposed to simply increased activity levels) of SMA activity in relation to movement onset is more important in augmenting fluent speech motor control. The current experimental design did not allow for such an examination. Third, there are considerable individual differences among PWS including severity levels, as well as possible SMA connectivity. In the current study, we used a modeling software that estimated spread of electric fields as a result of stimulating a specific target area, which was based on a single template brain that was applied to individual AWS to help localize and apply the electrodes to their scalp. In future studies, applying a subject-specific stimulation target localization based on the individual subjects’ brain structural MRI data might help mitigate these issues.
One variable that can differ considerably across speakers is stuttering severity. To account for the effects of stuttering severity, we examined the modulatory effects of stuttering severity on brain activity in a second analysis. Interestingly, we found two clusters in the right hemisphere that were differentially affected by active vs. sham stimulation in relation to severity. Active stimulation seems to deliver a stronger effect to severe AWS, changing the activation levels in regions that were abnormally heightened in severe AWS to be more similar to that in the mild AWS. Taken together, the two regions in which active stimulation modulated the relationship between activity and stuttering severity are part of the BGTC network that supports speech processing (Kotz and Schwartze, 2010). Within the BGTC network, the thalamus is suggested to play a critical role in interfacing the cerebellum with cortical motor areas (Kotz et al., 2016).
In the right postcentral gyrus, the negative correlation between stuttering severity and brain activity (gray line) was no longer present after active stimulation (black line). The right postcentral gyrus exhibited greater activity for more severe AWS at baseline (before active stimulation; Figure 2, gray line). This relationship, however, was no longer present after active stimulation. The results of the severity modulation somewhat support our hypotheses. These areas are known to mediate somatosensory processing, timing, and motor control. In a recent meta-analysis (Neef et al., 2015), the right postcentral gyrus was more active in PWS than fluent speakers (group effects, not severity). We found that although there was no main effect of stimulation in this region, active stimulation attenuated a negative relationship between activity in the right postcentral gyrus and stuttering severity.
In the right thalamus, we found that a positive relation between stuttering severity and activation in the right thalamus is no longer present after active stimulation. This finding may be related to findings from Giraud et al. (2008), who reported that stuttering severity was positively correlated with BG activity before, but not after therapy that focused on fluency-inducing techniques. Similar results have been found for the putamen (Neumann et al., 2003). In another study, Neef et al. (2018) used fMRI-based tractography to find that stuttering severity was positively correlated with connection strength between the right anterior thalamic radiation with seed placement in the right frontal pole. These results suggest that hyperactivity and connectivity involving the thalamus in more severe AWS could be alleviated with tDCS.
Finally, both active and sham stimulation significantly decreased the amount of stuttering during the reading portion of the speech sample, but not during conversation. This result is in agreement with the results of Chesters et al. (2018) who found that the effect of their tDCS treatment on reading (but not conversation) lasted up to 6 weeks. Although in the present study active stimulation led to a greater decrease (3.5%) in stuttering compared to sham stimulation (2.86%), this difference was not significant. This is not unexpected given that participants only received one session of active stimulation, which could be argued to be insufficient for effecting change in a complex motor movement like speech.
In conclusion, we report preliminary findings from the first HD-tDCS study of AWS. We found that anodal stimulation to the left SMA modulated the relationship between brain activity and stuttering severity in key regions of the right thalamocortical network. This is an important first step in paving the way for future use of HD-tDCS in the treatment of stuttering, which may have greater efficacy for treatment of severe stuttering cases. However, these results should be interpreted with caution due to some limitations, including the small sample size and wide range of stuttering severity represented in the current subject sample. Stuttering severity was measured using the SSI, and like all measures of behavioral attributes of stuttering, the score is influenced by many factors, including fatigue, task demands (e.g., reading vs. conversation), and treatment history, among others. All participants in this study reported receiving at least some formal stuttering therapy during their school years, with the exception of one who sought treatment in college, but none within 1 year of study participation. Because each person was his or her own control, differences in the individual before and after stimulation, accordingly, confounds (e.g., treatment history and severity) across individuals were minimized.
It is also possible that the use of metronome-paced reading during stimulation but choral reading during fMRI may have affected the results. During stimulation, we sought to use a condition that maximized chances of enhancing fluency in speakers who stutter, and metronome-paced speech is such a condition, even compared to choral speech (Toyomura et al., 2011). We paired stimulation with this condition to heighten the chances of augmenting the effects of the fluency inducing condition, and thus increase chances of finding differences between active and sham stimulation. There were also practical reasons behind using choral reading during fMRI. The auditory beats of the metronome could interact with the rhythmic beats and sounds of the scanner, and lead to additional auditory stimulation. Instead, we chose to use choral speech, which elicits similar neural activity (albeit weaker) than metronome-paced speech (Toyomura et al., 2011). Choral reading is also somewhat more naturalistic because it involves human voice rather than beats. Still, the lack of significant findings could be related to the use of different tasks, i.e., the effect could not transfer from one task to another.
Finally, this study involved a single active tDCS session, which likely provided insufficient intensity to precipitate significant changes in brain activity and speech fluency in all our participants. Chesters et al. (2018) have preliminary evidence showing that intensive treatment that involves delivering tDCS on five consecutive days was needed to effect behavioral change. This is consistent with other studies that have used similar multiple-day treatment designs that led to significant and prolonged motor enhancement (e.g., Reis et al., 2009). Future studies with larger sample sizes and more intensive delivery of stimulation are needed to further our understanding of effects of brain stimulation on brain activity and speech fluency. Other stimulation target regions should also be explored, guided by previous neuroimaging findings in stuttering.
Data Availability Statement
The raw data supporting the conclusions of this manuscript will be made available by the authors to any qualified researcher.
Ethics Statement
The studies involving human participants were reviewed and approved by Institutional Review Boards of the University of Michigan Medical School (IRBMED) which oversees human subjects research conducted at the Medical School and Michigan Medicine. The participants provided their written informed consent to participate in this study.
Author Contributions
EG, HC, AC, and S-EC contributed to the conception and design of the study. EG and AC recruited participants and collected the data. EG and HC performed data analysis. EG wrote the first draft of the manuscript. All authors contributed to manuscript revisions and read and approved the submitted version.
Funding
This project was funded by the American Speech-Language-Hearing Foundation Clinical Research Grant and the Matthew K. Smith Stuttering Research Fund, both awarded to S-EC.
Conflict of Interest
The authors declare that the research was conducted in the absence of any commercial or financial relationships that could be construed as a potential conflict of interest.
Acknowledgments
We would like to thank the participants for their time, Ben Hampstead for use of the modeling software, Saralyn Rubsam for her help in identifying and coding disfluencies, and Abhinaya Krishnaraj for her assistance with data collection.
Footnotes
References
AbdulSabur, N. Y., Xu, Y., Liu, S., Chow, H. M., Baxter, M., Carson, J., et al. (2014). Neural correlates and network connectivity underlying narrative production and comprehension: a combined fMRI and PET study. Cortex 57, 107–127. doi: 10.1016/j.cortex.2014.01.017
Allman, C., Amadi, U., Winkler, A. M., Wilkins, L., Filippini, N., Kischka, U., et al. (2016). Ipsilesional anodal tDCS enhances the functional benefits of rehabilitation in patients after stroke. Sci. Transl. Med. 8:330re1. doi: 10.1126/scitranslmed.aad5651
Andrews, G., Guitar, B., and Howie, P. (1980). Meta-analysis of the effects of stuttering treatment. J. Speech Hear. Disord. 45, 287–307. doi: 10.1044/jshd.4503.287
Baker, J. M., Rorden, C., and Fridriksson, J. (2010). Using transcranial direct-current stimulation to treat stroke patients with aphasia. Stroke 41, 1229–1236. doi: 10.1161/strokeaha.109.576785
Baudewig, J., Nitsche, M. A., Paulus, W., and Frahm, J. (2001). Regional modulation of BOLD MRI responses to human sensorimotor activation by transcranial direct current stimulation. Magn. Reson. Med. 45, 196–201. doi: 10.1002/1522-2594(200102)45:2<196::aid-mrm1026>3.0.co;2-1
Benwell, C. S. Y., Learmonth, G., Miniussi, C., Harvey, M., and Thut, G. (2015). Non-linear effects of transcranial direct current stimulation as a function of individual baseline performance: evidence from biparietal tDCS influence on lateralized attention bias. Cortex 69, 152–165. doi: 10.1016/j.cortex.2015.05.007
Berryhill, M. E., and Jones, K. T. (2012). tDCS selectively improves working memory in older adults with more education. Neurosci. Lett. 521, 148–151. doi: 10.1016/j.neulet.2012.05.074
Bikson, M., Grossman, P., Thomas, C., Zannou, A. L., Jiang, J., Adnan, T., et al. (2016). Safety of transcranial direct current stimulation: evidence based update 2016. Brain Stimul. 9, 641–661. doi: 10.1016/j.brs.2016.06.004
Bohland, J. W., Bullock, D., and Guenther, F. H. (2010). Neural representations and mechanisms for the performance of simple speech sequences. J. Cogn. Neurosci. 22, 1504–1529. doi: 10.1162/jocn.2009.21306
Bolognini, N., Vallar, G., Casati, C., Latif, L. A., El-Nazer, R., Williams, J., et al. (2011). Neurophysiological and behavioral effects of tDCS combined with constraint-induced movement therapy in poststroke patients. Neurorehabil. Neural Repair 25, 819–829. doi: 10.1177/1545968311411056
Bothe, A. K., Davidow, J. H., Bramlett, R. E., Franic, D. M., and Ingham, R. J. (2006). Stuttering treatment research 1970–2005: II. Systematic review incorporating trial quality assessment of pharmacological approaches. Am. J. Speech Lang. Pathol. 15, 342–352. doi: 10.1044/1058-0360(2006/032)
Cappon, D., Jahanshahi, M., and Bisiacchi, P. (2016). Value and efficacy of transcranial direct current stimulation in the cognitive rehabilitation: a critical review since 2000. Front. Neurosci. 10:157. doi: 10.3389/fnins.2016.00157
Cattaneo, Z., Pisoni, A., and Papagno, C. (2011). Transcranial direct current stimulation over Broca’s region improves phonemic and semantic fluency in healthy individuals. Neuroscience 183, 64–70. doi: 10.1016/j.neuroscience.2011.03.058
Chang, S.-E., Chow, H. M., Wieland, E. A., and McAuley, J. D. (2016). Relation between functional connectivity and rhythm discrimination in children who do and do not stutter. Neuroimage Clin. 12, 442–450. doi: 10.1016/j.nicl.2016.08.021
Chang, S.-E., and Zhu, D. C. (2013). Neural network connectivity differences in children who stutter. Brain 136, 3709–3726. doi: 10.1093/brain/awt275
Chesters, J., Möttönen, R., and Watkins, K. E. (2018). Transcranial direct current stimulation over left inferior frontal cortex improves speech fluency in adults who stutter. Brain 141, 1161–1171. doi: 10.1093/brain/awy011
Civier, O., Bullock, D., Max, L., and Guenther, F. H. (2013). Computational modeling of stuttering caused by impairments in a basal ganglia thalamo-cortical circuit involved in syllable selection and initiation. Brain Lang. 126, 263–278. doi: 10.1016/j.bandl.2013.05.016
Cox, R. W., Chen, G., Glen, D. R., Reynolds, R. C., and Taylor, P. A. (2017). FMRI clustering in AFNI: false-positive rates redux. Brain connect. 7, 152–171. doi: 10.1089/brain.2016.0475
Craig, A., Blumgart, E., and Tran, Y. (2009). The impact of stuttering on the quality of life in adults who stutter. J. Fluency Disord. 34, 61–71. doi: 10.1016/j.jfludis.2009.05.002
Craig, A., Hancock, K., Chang, E., McCready, C., Shepley, A., McCaul, A., et al. (1996). A controlled clinical trial for stuttering in persons aged 9 to 14 years. J. Speech Hear. Res. 39, 808–826. doi: 10.1044/jshr.3904.808
Craig, A. R., and Hancock, K. (1995). Self-reported factors related to relapse following treatment for stuttering. Aust. J. Hum. Commun. Disord. 23, 48–60. doi: 10.3109/asl2.1995.23.issue-1.04
Cunnington, R., Iansek, R., Thickbroom, G. W., Laing, B. A., Mastaglia, F. L., Bradshaw, J. L., et al. (1996). Effects of magnetic stimulation over supplementary motor area on movement in Parkinson’s disease. Brain 119, 815–822. doi: 10.1093/brain/119.3.815
Datta, A., Bansal, V., Diaz, J., Patel, J., Reato, D., and Bikson, M. (2009). Gyri-precise head model of transcranial direct current stimulation: improved spatial focality using a ring electrode versus conventional rectangular pad. Brain Stimul. 2, 201.e1–207.e1. doi: 10.1016/j.brs.2009.03.005
Datta, A., Elwassif, M., Battaglia, F., and Bikson, M. (2008). Transcranial current stimulation focality using disc and ring electrode configurations: FEM analysis. J. Neural Eng. 5, 163–174. doi: 10.1088/1741-2560/5/2/007
De Nil, L. F., Kroll, R. M., Lafaille, S. J., and Houle, S. (2003). A positron emission tomography study of short- and long-term treatment effects on functional brain activation in adults who stutter. J. Fluency Disord. 28, 357–380. doi: 10.1016/j.jfludis.2003.07.002
de Vries, M. H., Barth, A. C. R., Maiworm, S., Knecht, S., Zwitserlood, P., and Flöel, A. (2010). Electrical stimulation of broca’s area enhances implicit learning of an artificial grammar. J. Cogn. Neurosci. 22, 2427–2436. doi: 10.1162/jocn.2009.21385
Dockery, C. A., Hueckel-Weng, R., Birbaumer, N., and Plewnia, C. (2009). Enhancement of planning ability by transcranial direct current stimulation. J. Neurosci. 29, 7271–7277. doi: 10.1523/JNEUROSCI.0065-09.2009
Fertonani, A., Rosini, S., Cotelli, M., Rossini, P. M., and Miniussi, C. (2010). Naming facilitation induced by transcranial direct current stimulation. Behav. Brain Res. 208, 311–318. doi: 10.1016/j.bbr.2009.10.030
Fiori, V., Coccia, M., Marinelli, C. V., Vecchi, V., Bonifazi, S., Ceravolo, M. G., et al. (2011). Transcranial direct current stimulation improves word retrieval in healthy and nonfluent aphasic subjects. J. Cogn. Neurosci. 23, 2309–2323. doi: 10.1162/jocn.2010.21579
Fonteneau, C., Mondino, M., Arns, M., Baeken, C., Bikson, C., Brunoni, A. R., et al. (2019). Sham tDCS: A hidden source of variability? Reflections for further blinded, controlled trials. Brain Stimul. 12, 668–673. doi: 10.1016/j.brs.2018.12.977
Foundas, A. L., Mock, J. R., Cindass, R. Jr., and Corey, D. M. (2013). Atypical caudate anatomy in children who stutter. Percept. Mot. Skills 116, 528–543. doi: 10.2466/15.10.pms.116.2.528-543
Fridriksson, J., Richardson, J. D., Baker, J. M., and Rorden, C. (2011). Transcranial direct current stimulation improves naming reaction time in fluent aphasia. Stroke 42, 819–821. doi: 10.1161/strokeaha.110.600288
Fritsch, B., Reis, J., Martinowich, K., Schambra, H. M., Ji, Y., Cohen, L. G., et al. (2010). Direct current stimulation promotes BDNF-dependent synaptic plasticity: potential implications for motor learning. Neuron 66, 198–204. doi: 10.1016/j.neuron.2010.03.035
Garnett, E. O., and den Ouden, D.-B. (2015). Validating a sham condition for use in high definition transcranial direct current stimulation. Brain Stimul. 8, 551–554. doi: 10.1016/j.brs.2015.01.399
Giraud, A. L., Neumann, K., Bachoud-Levi, A. C., von Gudenberg, A. W., Euler, H. A., Lanfermann, H., et al. (2008). Severity of dysfluency correlates with basal ganglia activity in persistent developmental stuttering. Brain Lang. 104, 190–199. doi: 10.1016/j.bandl.2007.04.005
Hampstead, B. M., Brown, G. S., and Hartley, J. F. (2014). Transcranial direct current stimulation modulates activation and effective connectivity during spatial navigation. Brain Stimul. 7, 314–324. doi: 10.1016/j.brs.2013.12.006
Hancock, K., Craig, A., McCready, C., McCaul, A., Costello, D., Campbell, K., et al. (1998). Two- to six-year controlled-trial stuttering outcomes for children and adolescents. J. Speech Lang. Hear. Res. 41, 1242–1252. doi: 10.1044/jslhr.4106.1242
Hartwigsen, G. (2015). The neurophysiology of language: insights from non-invasive brain stimulation in the healthy human brain. Brain Lang. 148, 81–94. doi: 10.1016/j.bandl.2014.10.007
Hashemirad, F., Zoghi, M., Fitzgerald, P. B., and Jaberzadeh, S. (2016). The effect of anodal transcranial direct current stimulation on motor sequence learning in healthy individuals: a systematic review and meta-analysis. Brain Cogn. 102, 1–12. doi: 10.1016/j.bandc.2015.11.005
Holland, R., Leff, A. P., Penny, W. D., Rothwell, J. C., and Crinion, J. (2016). Modulation of frontal effective connectivity during speech. Neuroimage 140, 126–133. doi: 10.1016/j.neuroimage.2016.01.037
Hsu, T.-Y., Juan, C.-H., and Tseng, P. (2016). Individual differences and state-dependent responses in transcranial direct current stimulation. Front. Hum. Neurosci. 10:643. doi: 10.3389/fnhum.2016.00643
Iyer, M. B., Mattu, U., Grafman, J., Lomarev, M., Sato, S., and Wassermann, E. M. (2005). Safety and cognitive effect of frontal DC brain polarization in healthy individuals. Neurology 64, 872–875. doi: 10.1212/01.wnl.0000152986.07469.e9
Jang, S. H., Ahn, S. H., Byun, W. M., Kim, C. S., Lee, M. Y., and Kwon, Y. H. (2009). The effect of transcranial direct current stimulation on the cortical activation by motor task in the human brain: an fMRI study. Neurosci. Lett. 460, 117–120. doi: 10.1016/j.neulet.2009.05.037
Keeser, D., Meindl, T., Bor, J., Palm, U., Pogarell, O., Mulert, C., et al. (2011). Prefrontal transcranial direct current stimulation changes connectivity of resting-state networks during fMRI. J. Neurosci. 31, 15284–15293. doi: 10.1523/JNEUROSCI.0542-11.2011
Kell, C. A., Neumann, K., von Kriegstein, K., Posenenske, C., von Gudenberg, A. W., Euler, H., et al. (2009). How the brain repairs stuttering. Brain 132, 2747–2760. doi: 10.1093/brain/awp185
Kotz, S. A., Brown, R. M., and Schwartze, M. (2016). Cortico-striatal circuits and the timing of action and perception. Curr. Opin. Behav. Sci. 8, 42–45. doi: 10.1016/j.cobeha.2016.01.010
Kotz, S. A., and Schwartze, M. (2010). Cortical speech processing unplugged: a timely subcortico-cortical framework. Trends Cogn. Sci. 14, 392–399. doi: 10.1016/j.tics.2010.06.005
Kuo, H.-I., Bikson, M., Datta, A., Minhas, P., Paulus, W., Kuo, M.-F., et al. (2013). Comparing cortical plasticity induced by conventional and high-definition 4 × 1 ring tDCS: a neurophysiological study. Brain Stimul. 6, 644–648. doi: 10.1016/j.brs.2012.09.010
Lang, N., Siebner, H. R., Ward, N. S., Lee, L., Nitsche, M. A., Paulus, W., et al. (2005). How does transcranial DC stimulation of the primary motor cortex alter regional neuronal activity in the human brain? Eur. J. Neurosci. 22, 495–504. doi: 10.1111/j.1460-9568.2005.04233.x
Learmonth, G., Thut, G., Benwell, C. S. Y., and Harvey, M. (2015). The implications of state-dependent tDCS effects in aging: behavioural response is determined by baseline performance. Neuropsychologia 74, 108–119. doi: 10.1016/j.neuropsychologia.2015.01.037
Liew, S.-L., Santarnecchi, E., Buch, E. R., and Cohen, L. G. (2014). Non-invasive brain stimulation in neurorehabilitation: local and distant effects for motor recovery. Front. Hum. Neurosci. 8:378. doi: 10.3389/fnhum.2014.00378
Lu, C., Ning, N., Peng, D., Ding, G., Li, K., Yang, Y., et al. (2009). The role of large-scale neural interactions for developmental stuttering. Neuroscience 161, 1008–1026. doi: 10.1016/j.neuroscience.2009.04.020
Lu, C., Peng, D., Chen, C., Ning, N., Ding, G., Li, K., et al. (2010). Altered effective connectivity and anomalous anatomy in the basal ganglia-thalamocortical circuit of stuttering speakers. Cortex 46, 49–67. doi: 10.1016/j.cortex.2009.02.017
Madhavan, S., and Shah, B. (2012). Enhancing motor skill learning with transcranial direct current stimulation—a concise review with applications to stroke. Front. Psychiatry 3:66. doi: 10.3389/fpsyt.2012.00066
Maguire, G., Franklin, D., Vatakis, N. G., Morgenshtern, E., Denko, T., Yaruss, J. S., et al. (2010). Exploratory randomized clinical study of pagoclone in persistent developmental stuttering: the examining pagoclone for peRsistent dEvelopmental stuttering study. J. Clin. Psychopharmacol. 30, 48–56. doi: 10.1097/jcp.0b013e3181caebbe
Malyutina, S., and den Ouden, D.-B. (2015). High-definition tDCS of noun and verb retrieval in naming and lexical decision. Neuroregulation 2, 111–125. doi: 10.15540/nr.2.3.111
Neef, N. E., Anwander, A., Bütfering, C., Schmidt-Samoa, C., Friederici, A. D., Paulus, W., et al. (2018). Structural connectivity of right frontal hyperactive areas scales with stuttering severity. Brain 141, 191–204. doi: 10.1093/brain/awx316
Neef, N. E., Anwander, A., and Friederici, A. D. (2015). The neurobiological grounding of persistent stuttering: from structure to function. Curr. Neurol. Neurosci. Rep. 15:63. doi: 10.1007/s11910-015-0579-4
Neumann, K., Euler, H. A., von Gudenberg, A. W., Giraud, A.-L., Lanfermann, H., Gall, V., et al. (2003). The nature and treatment of stuttering as revealed by fMRI: a within- and between-group comparison. J. Fluency Disord. 28, 381–410. doi: 10.1016/j.jfludis.2003.07.003
Neumann, K., Preibisch, C., Euler, H. A., von Gudenberg, A. W., Lanfermann, H., Gall, V., et al. (2005). Cortical plasticity associated with stuttering therapy. J. Fluen. Disord. 30, 23–39. doi: 10.1016/j.jfludis.2004.12.002
Nitsche, M. A., Doemkes, S., Karaköse, T., Antal, A., Liebetanz, D., Lang, N., et al. (2007). Shaping the effects of transcranial direct current stimulation of the human motor cortex. J. Neurophysiol. 97, 3109–3117. doi: 10.1152/jn.01312.2006
Packman, A., Code, C., and Onslow, M. (2007). On the cause of stuttering: integrating theory with brain and behavioral research. J. Neurolinguistics 20, 353–362. doi: 10.1016/j.jneuroling.2006.11.001
Packman, A., and Onslow, M. (2002). Searching for the cause of stuttering. Lancet 360, 655–656. doi: 10.1016/s0140-6736(02)09880-x
Park, J., and Logan, K. J. (2015). The role of temporal speech cues in facilitating the fluency of adults who stutter. J. Fluency Disord. 46, 41–55. doi: 10.1016/j.jfludis.2015.07.001
Pereira, J. B., Junqué, C., Bartrés-Faz, D., Martí, M. J., Sala-Llonch, R., Compta, Y., et al. (2013). Modulation of verbal fluency networks by transcranial direct current stimulation (tDCS) in Parkinson’s disease. Brain Stimul. 6, 16–24. doi: 10.1016/j.brs.2012.01.006
Polanía, R., Paulus, W., and Nitsche, M. A. (2012). Modulating cortico-striatal and thalamo-cortical functional connectivity with transcranial direct current stimulation. Hum. Brain Mapp. 33, 2499–2508. doi: 10.1002/hbm.21380
Preibisch, C., Neumann, K., Raab, P., Euler, H. A., von Gudenberg, A. W., Lanfermann, H., et al. (2003). Evidence for compensation for stuttering by the right frontal operculum. Neuroimage 20, 1356–1364. doi: 10.1016/s1053-8119(03)00376-8
Reis, J., Schambra, H. M., Cohen, L. G., Buch, E. R., Fritsch, B., Zarahn, E., et al. (2009). Noninvasive cortical stimulation enhances motor skill acquisition over multiple days through an effect on consolidation. Proc. Natl. Acad. Sci. U S A 106, 1590–1595. doi: 10.1073/pnas.0805413106
Richardson, J. D., Fillmore, P., Datta, A., Truong, D., Bikson, M., and Fridriksson, J. (2014). Toward development of sham protocols for high-definition transcranial direct current stimulation (HD-tDCS). Neuroregulation 1, 62–72. doi: 10.15540/nr.1.1.62
Sarkar, A., Dowker, A., and Cohen Kadosh, R. (2014). Cognitive enhancement or cognitive cost: trait-specific outcomes of brain stimulation in the case of mathematics anxiety. J. Neurosci. 34, 16605–16610. doi: 10.1523/JNEUROSCI.3129-14.2014
Sparing, R., Dafotakis, M., Meister, I. G., Thirugnanasambandam, N., and Fink, G. R. (2008). Enhancing language performance with non-invasive brain stimulation—a transcranial direct current stimulation study in healthy humans. Neuropsychologia 46, 261–268. doi: 10.1016/j.neuropsychologia.2007.07.009
Stagg, C. J., Best, J. G., Stephenson, M. C., O’Shea, J., Wylezinska, M., Kincses, Z. T., et al. (2009). Polarity-sensitive modulation of cortical neurotransmitters by transcranial stimulation. J. Neurosci. 29, 5202–5206. doi: 10.1523/JNEUROSCI.4432-08.2009
Stagg, C. J., and Nitsche, M. A. (2011). Physiological basis of transcranial direct current stimulation. Neuroscientist 17, 37–53. doi: 10.1177/1073858410386614
Toyomura, A., Fujii, T., and Kuriki, S. (2011). Effect of external auditory pacing on the neural activity of stuttering speakers. Neuroimage 57, 1507–1516. doi: 10.1016/j.neuroimage.2011.05.039
Toyomura, A., Fujii, T., and Kuriki, S. (2015). Effect of an 8-week practice of externally triggered speech on basal ganglia activity of stuttering and fluent speakers. Neuroimage 109, 458–468. doi: 10.1016/j.neuroimage.2015.01.024
Tsapkini, K., Frangakis, C., Gomez, Y., Davis, C., and Hillis, A. E. (2014). Augmentation of spelling therapy with transcranial direct current stimulation in primary progressive aphasia: preliminary results and challenges. Aphasiology 28, 1112–1130. doi: 10.1080/02687038.2014.930410
Tseng, P., Hsu, T.-Y., Chang, C.-F., Tzeng, O. J. L., Hung, D. L., Muggleton, N. G., et al. (2012). Unleashing potential: transcranial direct current stimulation over the right posterior parietal cortex improves change detection in low-performing individuals. J. Neurosci. 32, 10554–10561. doi: 10.1523/JNEUROSCI.0362-12.2012
Vestito, L., Rosellini, S., Mantero, M., and Bandini, F. (2014). Long-term effects of transcranial direct-current stimulation in chronic post-stroke aphasia: a pilot study. Front. Hum. Neurosci. 8:785. doi: 10.3389/fnhum.2014.00785
Weber, M. J., Messing, S. B., Rao, H., Detre, J. A., and Thompson-Schill, S. L. (2014). Prefrontal transcranial direct current stimulation alters activation and connectivity in cortical and subcortical reward systems: a tDCS-fMRI study. Hum. Brain Mapp. 35, 3673–3686. doi: 10.1002/hbm.22429
Wieland, E. A., McAuley, J. D., Dilley, L. C., and Chang, S.-E. (2015). Evidence for a rhythm perception deficit in children who stutter. Brain Lang. 144, 26–34. doi: 10.1016/j.bandl.2015.03.008
Woods, A. J., Antal, A., Bikson, M., Boggio, P. S., Brunoni, A. R., Celnik, P., et al. (2016). A technical guide to tDCS and related non-invasive brain stimulation tools. Clin. Neurophysiol. 127, 1031–1048. doi: 10.1016/j.clinph.2015.11.012
Xu, Y., Tong, Y., Liu, S., Chow, H. M., AbdulSabur, N. Y., Mattay, G. S., et al. (2014). Denoising the speaking brain: toward a robust technique for correcting artifact-contaminated fMRI data under severe motion. Neuroimage 103, 33–47. doi: 10.1016/j.neuroimage.2014.09.013
Yada, Y., Tomisato, S., and Hashimoto, R. I. (2019). Online cathodal transcranial direct current stimulation to the right homologue of Broca’s area improves speech fluency in people who stutter. Psychiatry Clin. Neurosci. 73, 63–69. doi: 10.1111/pcn.12796
Keywords: speech, stuttering, fMRI, tDCS, neuromodulation, fluency, neuroimaging
Citation: Garnett EO, Chow HM, Choo AL and Chang S-E (2019) Stuttering Severity Modulates Effects of Non-invasive Brain Stimulation in Adults Who Stutter. Front. Hum. Neurosci. 13:411. doi: 10.3389/fnhum.2019.00411
Received: 11 July 2019; Accepted: 07 November 2019;
Published: 21 November 2019.
Edited by:
Xing Tian, New York University Shanghai, ChinaReviewed by:
Luc De Nil, University of Toronto, CanadaMartin Sommer, University of Göttingen, Germany
Copyright © 2019 Garnett, Chow, Choo and Chang. This is an open-access article distributed under the terms of the Creative Commons Attribution License (CC BY). The use, distribution or reproduction in other forums is permitted, provided the original author(s) and the copyright owner(s) are credited and that the original publication in this journal is cited, in accordance with accepted academic practice. No use, distribution or reproduction is permitted which does not comply with these terms.
*Correspondence: Emily O’Dell Garnett, ZW1pbHlvZ0BtZWQudW1pY2guZWR1