- 1Honeywell Labs, Honeywell Aerospace, Redmond, WA, United States
- 2Department of Neurology, Berenson-Allen Center for Non-Invasive Brain Stimulation, Beth Israel Deaconess Medical Center, Division for Cognitive Neurology, Harvard Medical School, Boston, MA, United States
- 3Department of Experimental Psychology, Medical Sciences Division, University of Oxford, Oxford, United Kingdom
- 4Department of Electrical and Computer Engineering, Northeastern University, Boston, MA, United States
The present study introduces a novel cognitive intervention aimed at improving fluid intelligence (Gf), based on a framework we refer to as FAST: Flexible, Adaptive, Synergistic Training. FAST leverages a combination of novel game-based executive function (EF) training—designed specifically to enhance the likelihood of transfer—and transcranial electrical stimulation (tES), with aims to synergistically activate and strengthen mechanisms of cognitive control critical to Gf. To test our intervention, we collected three Gf measures from 113 participants [the advanced short Bochumer Matrizen-Test (BOMAT), Raven’s Advanced Progressive Matrices (APM), and matrices similar to Raven’s generated by Sandia labs], prior to and following one of three interventions: (1) the FAST + tRNS intervention, a combination of 30 min of daily training with our novel training game, Robot Factory, and 20 min of concurrent transcranial random noise stimulation applied to bilateral dorsolateral prefrontal cortex (DLPFC); (2) an adaptively difficult Active Control intervention comprised of visuospatial tasks that specifically do not target Gf; or (3) a no-contact control condition. Analyses of changes in a Gf factor from pre- to post-test found numerical increases for the FAST + tRNS group compared to the two control conditions, with a 0.3 SD increase relative to Active Control (p = 0.07), and a 0.19 SD increase relative to a No-contact control condition (p = 0.26). This increase was found to be largely driven by significant differences in pre- and post-test Gf as measured on the BOMAT test. Progression through the FAST training game (Robot Factory) was significantly correlated with changes in Gf. This is in contrast with progress in the Active Control condition, as well as with changes in individual EFs during FAST training, which did not significantly correlate with changes in Gf. Taken together, this research represents a useful step forward in providing new insights into, and new methods for studying, the nature of Gf and its malleability. Though our results await replication and extension, they provide preliminary evidence that the crucial characteristic of Gf may, in fact, be the ability to combine EFs rapidly and adaptively according to changing demand, and that Gf may be susceptible to targeted training.
Introduction
Fluid intelligence (Gf) has been defined as the ability to flexibly apply one’s knowledge and skills to novel situations (Cattell, 1971; Carpenter et al., 1990). This ability is crucial in learning and adaptive behavior, and in navigating complex environments where one must maintain multiple competing goals (Gottfredson, 1997; Gray and Thompson, 2004; Deary et al., 2007). The question of whether focused training of specific cognitive skills can enhance Gf is currently a topic of intense debate (Shipstead et al., 2012; Melby-Lervåg and Hulme, 2013, 2015; Au et al., 2014; Karbach and Verhaeghen, 2014; von Bastian and Oberauer, 2014), and is one of substantial practical and theoretical importance: Gf is a strong predictor of academic success, lifetime earnings and other significant life outcomes (Gottfredson, 1997; Gray and Thompson, 2004; Deary et al., 2007), such that enhancement through training could confer valuable benefits. In terms of theory, identifying cognitive skills that can be trained to produce enhanced Gf would provide valuable evidence regarding the core component processes of Gf, which have proven elusive to date. With the stakes high, the literature is burgeoning with new training studies, including prominent examples of successful positive transfer from focused cognitive training with tests that load strongly on Gf (Jaeggi et al., 2008, 2014; Rudebeck et al., 2012; Salminen et al., 2012), as well as prominent failures to replicate transfer (Owen et al., 2010; Chooi and Thompson, 2012; Harrison et al., 2013; Thompson et al., 2013).
Skills acquired through cognitive training will only transfer to novel domains if the training tasks share component processes with the broader skills targeted for enhancement, and if the learned task encodings are general enough to be applied to novel contexts (Singley and Anderson, 1985; VanLehn, 1996; Jaeggi et al., 2008; Shipstead et al., 2010; Taatgen, 2013). Many studies to date have taken the approach of using working memory training tasks to enhance Gf, given that working memory has been proposed as a core executive function (EF) underlying Gf (Wiley et al., 2011; Diamond, 2013). Similar brain regions are activated during performance of working memory tasks (Burgess et al., 2011) and tasks thought to depend on Gf (Halford et al., 2007). Additionally, measures of working memory capacity have been shown to explain at least half of variance in Gf across individuals (Kane et al., 2005).
However, evidence of positive transfer from working memory training to Gf task performance remains mixed, with some analyses finding evidence of significant near and far-transfer (Schmiedek et al., 2010; Au et al., 2014; Karbach and Verhaeghen, 2014), and others finding limited or no transfer effects (Melby-Lervåg and Hulme, 2013; Rapport et al., 2013; Lampit et al., 2014). Inconsistent findings, combined with methodological issues such as lack of active control groups and small sample sizes, have complicated the larger picture of its effectiveness. Many studies to-date vary task difficulty while holding the task and stimuli fixed over training (e.g., strategy training approaches, reviewed in Verhaeghen et al., 2004; Jaeggi et al., 2008; Morrison and Chein, 2011), leading to repetitive practice that previous work on skill acquisition suggests may produce task-specific strategies rather than general skills that should transfer across contexts (Chase and Ericsson, 1982). Furthermore, studies have often lacked an appropriate active control condition (Shipstead et al., 2010; Dougherty et al., 2016), finding improvements only in comparison to no-contact controls and allowing for the possibility that Hawthorne or other placebo-like effects are the true source of improvement following training.
Considered together, prior research does not offer a consistent answer to the question of whether working memory training can enhance Gf. But, prior research does point to avenues for enhancing the effects of cognitive training on Gf. The first opportunity for improvement comes from EF research suggesting that a broader set of EF processes, besides working memory, contribute to Gf. As detailed in Diamond (2013), Gf represents a set of higher-order reasoning and problem-solving EFs, which are influenced by and share neural resources with three core EFs: working memory, cognitive flexibility and inhibition (Duncan and Owen, 2000; Duncan et al., 2012). These functions have been characterized as the core components of cognitive control, and the building-blocks of complex adaptive behaviors (Miyake et al., 2000; Friedman et al., 2008; Collins and Koechlin, 2012; Lunt et al., 2012). Therefore, rather than focusing on working memory training alone, all three core EFs should be considered. The second avenue for improving Gf-related cognitive training comes from the learning transfer literature, suggesting that the ability to encode generalizable knowledge crucially depends on the diversity of contexts in which skills are acquired and practiced.
These gaps have informed the FAST (Flexible, Adaptive, Synergistic Training) training framework. The FAST approach reflects a hypothesis that a training environment that supports broad contextual variation and broadly emphasizes all core EF processes will tap into networks relevant to Gf and will foster more effective skill development. The FAST approach is designed to engage all three core EFs [working memory, cognitive flexibility, and inhibitory control (Monsell, 1996; Miyake et al., 2000; Diamond, 2013)], and do so in various combinations across a rich variety of environments. EFs are exercised through variants of well-established task-types, including for working memory the n-back task (Verhaeghen et al., 2004; Owen et al., 2005; Verhaeghen and Basak, 2005), for cognitive flexibility the task switching paradigm (Rogers and Monsell, 1995), and for inhibition the go/no-go (Donders, 1969) and stop-signal tasks (Logan and Cowan, 1984).
A second empirically-grounded hypothesis of FAST is that the combination of the training with noninvasive brain stimulation (NIBS) may synergistically boost the activity of cortical areas thought to be critical to Gf, and promote modulations in neural activity (e.g., changes in cortical excitability, network connectivity, plasticity and/or increased signal-to-noise ratios) during training that can lead to enhanced cognitive function in healthy individuals (Krause and Cohen Kadosh, 2013; Cohen Kadosh, 2015; Santarnecchi et al., 2015; Looi et al., 2016). In this initial test of the combination of FAST and NIBS, we used transcranial random noise stimulation (tRNS). Researchers have suggested that tRNS can increase cortical excitability through mechanisms of stochastic resonance (Terney et al., 2008), which, combined with the appropriate task, can increase learning (Fertonani et al., 2011; Santarnecchi et al., 2015). Recent results have highlighted the particular benefits of tRNS applied to bilateral dorsolateral prefrontal cortex (DLPFC) in the acquisition and retention of high-level cognitive skill (Snowball et al., 2013), and the effect of tRNS has been shown to be more effective as task difficulty increases (Popescu et al., 2016), which may be beneficial in a training context. Work to-date exploring the impact of alternative stimulation approaches to enhancement of Gf has seen mixed results, with recent studies of transcranial alternating current in theta band (Pahor and Jaušovec, 2014) and gamma band frequencies (Santarnecchi et al., 2013) seeing positive offline results in terms of performance enhancement on Gf tests like Raven’s Advanced Progressive Matrices (APM), and a recent examination of the impact of transcranial direct current stimulation finding detrimental offline effects of stimulation on metrics of the Weschler adult intelligence scale IV (Sellers et al., 2015).
In light of the substantive methodological issues in the field reviewed above, and the considerable theoretical uncertainty about whether it is possible at all to enhance Gf relative to appropriately strict controls, we focused here on the combined intervention of FAST+tRNS, which we theorized would have the greatest likely efficacy. This approach left open—for the moment—the question of whether FAST, tRNS, or the combination thereof would critically underpin any observed effects. Thus, this study aimed to assess whether a compound intervention, consisting of a combination of FAST+tRNS, could lead to enhancement of Gf relative to both a no-contact and active control condition.
Besides augmenting Gf training as noted above, we address methodological weaknesses that have been noted in working memory training research. Recent work has highlighted the need for comparison of cognitive interventions against active control conditions (Shipstead et al., 2012). We assessed the impact of the FAST+tRNS training across 9–11 daily sessions via changes in performance from pre- to post-training on a suite of established tasks that load strongly on Gf, and contrasted these results with those from an active control training of equivalent duration, as well as a no-contact control condition. The comparison of our intervention against an active control condition is particularly important, given recent prominent findings of null results in the literature when such a comparison is made (Chooi and Thompson, 2012; Redick et al., 2013). We also collected multiple measures of Gf in keeping with recommended best practices for determining the generalizability of results (Shipstead et al., 2010). With several measures, shared (non-task specific) variance can be established by means of factor analysis or composite score (Kim and Mueller, 1978). The active control condition was administered similarly to the FAST training and was designed to maintain participant motivation by including several tasks that were adaptive in their difficulty based on participant performance. Crucially, the active control training differed from FAST by targeting lower-level perceptual abilities, rather than high-level EFs. Additionally, a third group of participants served as passive (no-contact) controls, performing on the pre- and post-test measures with no intervening training.
Materials and Methods
Participants
A total of 113 participants across three data collection sites gave their informed consent to participate in the study: Beth Israel Deaconess Medical Center (BIDMC; n = 36), University of Oxford (n = 36) and Northeastern University (NEU; n = 41). Participant exclusion criteria included a history of health problems such as epilepsy, migraines, neurological and psychiatric disorders. Participants were required to have normal or corrected vision and hearing, and agree to abstain from alcohol throughout the study, and refrain from caffeine consumption within 2 h of daily training. Of the 113 participants from whom data was collected, 14 participants were excluded from the analysis due to either an error in the progression of FAST that stopped participants from reaching the highest level of our training (n = 10), or due to non-compliance with the test administration on at least two independent measures (n = 4). In addition to these 14, the initial seven participants of the study were administered a shorter version of the Bochumer Matrizen-Test (BOMAT), which had been used in a pilot study, and one participant was found to have been administered the BOMAT post-test at both the pre- and post-test sessions. Data from these participants were also excluded, and as a result final analyses consisted of pre-test and post-test performance for 91 participants across the three conditions (FAST+tRNS: n = 32, age 22.4 ± 3.4; active control: n = 30, age 24.57 ± 4.54; No-Contact: n = 29, age 23.4 ± 4.3; Harvard BIDMC: n = 27; NEU: n = 31; Oxford: n = 33).
Participants in the study were pseudorandomly assigned to one of three conditions: no-contact control (NC), active control (AC), or training (FAST+tRNS), such that group sizes and baseline characteristics were balanced as well as possible, One-way analysis of variance of the three groups found no significant differences in age (NC: μ= 23.38 years, SD = 4.25; AC: μ = 25.57, SD = 4.54; FAST+tRNS: μ = 22.4, SD = 3.42), years of education (NC: μ = 16.93, SD = 2.68; AC: μ = 17.23, SD = 3.13; FAST+tRNS: μ = 16.03, SD = 2.48), or Gf at pre-test (NC: μ = 0.09, SD = 1.09; AC: μ = 0.07, SD = 1.06; FAST+tRNS: μ = −0.14, SD = 0.87).
In the US, these studies were approved by the institutional review board at all participating institutions (Harvard BIDMC: Committee on Clinical Investigations/IRB, Protocol 2014P-000024; Northeastern: Human Subject Research Protection/IRB, Protocol 14-08-15) and in the UK by the Berkshire National Research Ethics Committee (REC reference 14/SC/0131). All participants gave written informed consent prior to training, according to the Declaration of Helsinki, and were remunerated for their participation (£12 and $11–20 per hour depending on site, in the UK and the US, respectively).
A Novel Approach: FAST
A fundamental aspect of the FAST framework is that it is designed to foster the development of general skill encoding through practice in highly variable contexts, in order to support transfer. This crucial flexibility of FAST comes from the wide array of tasks it includes, based on the use of unique (factorial) combinations of EFs, as well as the inclusion of an array of other critical cognitive elements throughout. For example, FAST tasks engage several domains of working memory through the use of different stimulus types and task contexts (visual: pictorial and spatial; symbolic: verbal and numeric). FAST also utilizes logical and relational cognitive operators in many of the tasks; for example some tasks require that participants sort stimuli based on whether they are both a certain color and shape (logical: and), or based on whether they come from the same semantic category (e.g., “animal"; relational: semantic). In the highest levels of training, FAST also includes tasks that require hypothesis-testing, where flexible task-model construction must occur.
Finally, a crucial feature of FAST is the requirement for participants to engage in rapid instructed task learning (Cole et al., 2013). The ability to generate new task models flexibly and reliably in response to changing instructions has been proposed to be central to the relationship between working memory and Gf (Salthouse and Pink, 2008) and could also be a crucial factor in the relationship of Gf with cognitive flexibility and inhibitory control. In particular, working memory tasks that involve instructed performance of this kind have been shown to very strongly activate the network of brain regions implicated in high-level reasoning and problem solving (Dumontheil et al., 2011) and correlate more strongly with Gf than standard working memory paradigms (Duncan et al., 2012). All components of FAST require participants to rapidly encode novel sets of instructions and use these to guide their action. This encode-perform cycle is repeated with entirely new task requirements every 2–3 min, with little or no repetition of specific tasks within or between training sessions, such that FAST emphasizes the general capacity to flexibly configure cognitive resources according to current requirements, rather than the specific ability to adopt any one configuration in particular.
Although FAST incorporates a very large task space, the particular progression of tasks encountered by a participant is adaptively controlled by measures of success, as well as an underlying structure of task “levels". Level 1 tasks involve only one EF (single-EF tasks), such as switching between categorizing object pictures as animal/non-animal or flying/non-flying, depending on the color of a light that appears with the stimulus (cognitive flexibility). Level 2 tasks combine two EFs (dual-EF tasks), such as tasks asking participants to both switch between categorizing object pictures as animal/non-animal or flying/non-flying depending on a cue light, and to withhold responses (inhibition) when a no-go stimulus cue is present (e.g., a yellow “stop” signal). Level 3 tasks combine one or two EF components with a cognitive operator of logical or relational contrasts, such as switching between categorizing object pictures as animal/non-animal or flying/non-flying, depending on whether the yellow “stop" signal or a cue light appears (not both; logical xOR). Level 4 tasks come in two varieties. Some tasks combine all three core EFs, such as switching between categorizations while withholding responses if the current stimulus matches the stimulus presented three trials previously (working memory). These Level 4 tasks incorporate an imbalance among the EF sub-components, such that a dual-EF task is performed for the majority of trials (primary task), and in the case of an intermittent cue, a single-EF task is performed (secondary task). This imbalance among the EF sub-tasks serves as a means of training against goal neglect (Duncan and Owen, 2000), a key component of Gf which, to our knowledge, has rarely if ever been explicitly incorporated into a training regimen. In addition, certain of these Level 4 tasks incorporate a dual n-back component, which has been tied to instances of transfer to improvement in Gf in well-known previous work (Jaeggi et al., 2008). Finally, the second type of Level 4 tasks requires the participant to engage in hypothesis-testing in order to infer the appropriate tasks to perform, such as inferring the correct categorization rule for object pictures based on the color of a light that appears with the stimulus, without explicit instruction to do so.
With the increasing number of components present in the levels of our game, we are able to iteratively expose participants to increasingly complex and diverse environments of EF practice. For example, a participant will encounter tasks targeting inhibition alone (Level 1 tasks) before they encounter inhibition tasks that also include either working memory (inhibition + working memory, Level 2) or cognitive flexibility (inhibition + cognitive flexibility, Level 2). All of this will precede tasks that also include a logical or relational operator (e.g., inhibition + working memory + xOR, Level 3), which are then followed by either those that include all three EFs (inhibition + working memory + cognitive flexibility, Level 4a), or those that include hypothesis-testing (Level 4b). While we predicted that our four levels would roughly contain tasks of increasing difficulty (e.g., Level 3 tasks would be harder than Level 2 tasks), tasks were further ordered according to relative difficulty, based on participant performance in previous pilot data. We used the resulting order to move participants through tasks of increasing difficulty (roughly sequentially through our four task levels), while maintaining approximately equal exposure to the three core EFs at all times. We also prioritized advancing participants into Level 2+ tasks fairly quickly, so participants spent the least amount of time on Level 1 (single-EF) tasks.
Procedure
Our study included three groups, to which participants were randomly assigned according to a stratified randomization process based on age, education, and gender. These groups included: individuals who received the FAST+tRNS intervention, individuals in an active control condition (AC), and individuals in a no-contact control condition (NC). Participants in the FAST+tRNS and active control groups were enrolled in the study for 13 days across 3 weeks, including an initial day of pre-tests, 9–11 days of training, and 1 day of post-tests. Participants were allowed to miss no more than two (total) days of training and remain enrolled in the study (minimum number of training days = 9). Participants were asked to refrain from drinking alcoholic beverages in the evenings before training sessions, and from drinking caffeinated beverages within 1 h of training. Participants were also instructed that compliance with the study protocol required at least 6 h of sleep each night during enrollment in the study. However, in this study sleep monitoring was not included.
Pre- and post-tests consisted of three tests of Gf as detailed below, and were completed in a single session lasting approximately 1 h. Parallel versions of the Gf tests were administered at the pre- and post-test sessions. On training days, participants in the FAST+tRNS and active control groups engaged in their respective training interventions for 30 min per day, during which time they engaged in a variety of either cognitive (FAST+tRNS) or visuospatial tasks (active control). In the case of the FAST+tRNS group, the final 9 days of training included 20 min of transcranial random noise stimulation (tRNS), applied bilaterally over dorsal prefrontal regions (days one and two had no stimulation). The onset of stimulation was aligned with the start of the training and included a 30-s ramp-up and ramp-down. Stimulation current (1 mA) was delivered via pi-electrodes (3.14 cm2) to channels F3 and F4 of the International 10-20 EEG system. Participants were informed of the possibility that they might receive stimulation during informed consent, and all participants were blinded as to the existence of multiple forms of training (AC and FAST+tRNS).
The total duration of each training session for the FAST+tRNS and active control groups was approximately 1 h, with 30 min of intervention, several minutes for breaks, and approximately 20 min for equipment set-up and paperwork. No-contact Control participants completed all pre-test and post-tests on the same schedule as the FAST+tRNS and active control groups, but received no training in-between.
Apparatus
All tests and training were administered on DELL PCs equipped with the Windows 7 operating system. All Gf tests (BOMAT, Raven’s, and Sandia) and active control tasks were implemented with Presentation software (Version 17.0; Neurobehavioral Systems, Inc., Albany, CA, USA). Transcranial electrical stimulation (tES) was applied using the Starstim™ system developed by Neuroelectrics (Barcelona, Spain). The Starstim™ wireless system offers flexible placement of up to eight electrodes according to the 10-20 system. Each electrode can be configured for either stimulation or recording, allowing for simultaneous delivery of electrical stimulation and EEG data acquisition. All EEG activity was recorded in real-time via an application programming interface developed by Neuroelectrics—analyses of the EEG data are not reported here.
Brain Stimulation
The stimulation protocol used in this study involved transcranial random noise stimulation (tRNS) over DLPFC (channels F3 and F4). While there is some debate as to whether the neurobiological substrate of intelligence is related to activity of a diffuse network vs. a restricted number of brain regions (Jung and Haier, 2007), the nature of the brain stimulation we were able to apply in the present investigation posed some constraints in terms of the number and location of available target regions (i.e., only two electrodes could be placed on the scalp). We, therefore, informed our decision of target location with results of previous studies of tES over DLPFC, which showed significant modulations of Gf (Santarnecchi et al., 2013, 2016) as well as with available neuroimaging literature addressing the localization of Gf-related processes in the brain (Cole et al., 2012). The final selection of montage also overlapped with those used in the vast majority of the available literature on cognitive enhancement and tES (Santarnecchi et al., 2015).
The frequency spectrum of transcranial random noise stimulation (tRNS) used in this study was 100–500 Hz. This could be referred to as “high-frequency random noise” stimulation (tRNS applied in a frequency band below 100 Hz is commonly reported as “low frequency” tRNS). As documented in the original publication by Terney et al. (2008) and Santarnecchi et al. (2015), random noise electrical stimulation above 100 Hz seems to elicit a significant modulation of both electrophysiological and behavioral indices, with changes in cortico-spinal excitability measured via single-pulse transcranial magnetic stimulation (TMS), and a reduction of response times during a serial response time task. While low-frequency tRNS did not show any significant results in the work by Terney et al. (2008), additional significant effects have been reported by several groups using high-frequency tRNS, showing effects on motor learning (Cappelletti et al., 2013), perceptual learning (Fertonani et al., 2011) and arithmetic training (Snowball et al., 2013). Due to a limitation in the sampling rate of the brain stimulation device used for the present investigation (500 Hz), random noise stimulation was delivered between 100–500 Hz instead of a 100–640 Hz window used in the aforementioned publications. Given the frequency unspecific effects of tRNS, and its mechanism of action based on the injection of white noise and corresponding increase in the signal-to-noise ratio of the targeted brain region via stochastic resonance (Chaieb et al., 2011; Fertonani et al., 2011), we expected 100–500 Hz tRNS to produce the same or very similar effects as the original 100–640 Hz version.
The current density selected in this study was within the safety guidelines for tES reported by Neuroelectrics, though they differ from the values one expects from a “canonical” 5*7 cm electrode. The pi-stim electrodes used in this study differ from other electrode solutions usually applied in similar investigations, as they are based on sintered Ag/AgCl pellets and require conductive gel, rather than the more typical saline solution. This arrangement, though relatively novel, provides a more uniform current delivery. As for the canonical formula Intensity/Area, as suggested by the manufacturer1, the resulting current density does not increase linearly with electrode size, making a comparison with other publications using 5*7 or 3*5 sponge electrodes not entirely accurate (Miranda et al., 2009). From a more practical point of view, pi-stim has been used in several publications to achieve more focal stimulation solutions, with no ill effects being reported (Minhas et al., 2010; Borckardt et al., 2012; Faria et al., 2012; Murray et al., 2015). The same applies to our experience based on more than 900 stimulation sessions in more than 100 participants.
Robot Factory
The behavioral portion of the FAST intervention is represented in the novel training game, Robot Factory (Figure 1; developed in collaboration with SimCoach Games2). Robot Factory was custom-designed based on the principles of the FAST framework and provides an engaging environment for completing a challenging and varied suite of EF tasks. In Robot Factory, participants are brought to a dystopian future in which they are employees of a factory producing robots. There they are asked to perform tasks related to building, sorting or programming ‘bots, according to instructions given every 2 min by their supervisor, Boss Bot. Task instructions are complex and reflect a “real world” application of one or more EF skills (e.g., programming a ‘bot by encoding a list of images as living beings/inanimate objects or flying/not-flying entities depending on cues presented in their work station during the shift). In Robot Factory, each 2-min “shift” represents a unique combination of EFs, logical or relational operators, stimulus domains, thematic context, stimuli and instructions. Progression through shifts is adaptive to a participant’s individual performance, such that task types and parameters for each session began at the level reached at the end of the previous session, keeping tasks challenging and requiring that participants maintain effortful performance.
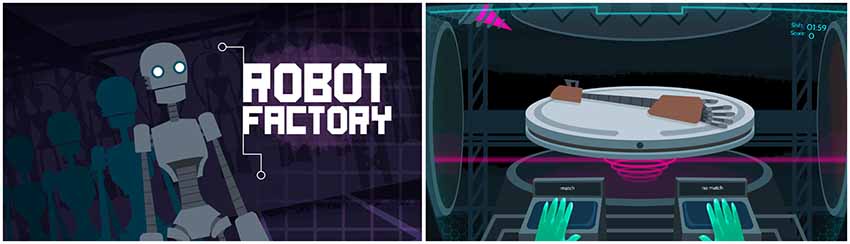
Figure 1. Robot Factory opening screen image (left), and example task screenshot from the Assembly Line scenario (right). In this task, participants are presented with robot arms one at a time on a moving platform, which rises from a portal at the bottom of the screen. Participants must decide which direction to sort the arm (indicated with the corresponding left or right “Shift” key), based on whether or not it is a match (in color and style) with the arm seen 2-previous. If the participant gives the correct response, the platform moves into the correct sorting tube and an icon appears indicating an increase in points. If the participant gives the incorrect response, the laser in the upper left corner shoots a beam onto the platform, dissolving the arm, and the platform recedes into the tube in the lower portion of the screen.
In Robot Factory, participants are presented with two-alternative forced choice tasks, where they are asked to respond with either the Left Shift or Right Shift key. Shifts are composed of a variable number of trials, the duration of which could last 1, 2, or 3 s, depending on recent participant performance. In a participant’s first exposure to a task, stimuli were presented for up to 2 s, or until the participant gave a response. If a participant performed well on the task, stimulus durations on related tasks would be reduced to 1 s (or until the participant responded), but if the participant performed poorly on the task, stimulus durations would instead be extended to 3 s (or until the participant responded). A similar algorithm was implemented for the n of the Update n-back tasks, which varied between 1-, 2- and 3-back tasks. Feedback for each response was offered to the participant in both visual and auditory forms, and throughout training thematic music was presented to participants via headphones.
Active Control
Our Active Control condition was created as a comparison group for our FAST intervention—one that is similarly challenging to FAST training, but which specifically does not target components of Gf. By including an active control condition we address a significant limitation in the WM training literature, in which the results of training protocols are often compared only against a no-contact control condition (Buschkuehl and Jaeggi, 2010; Shipstead et al., 2010; Dougherty et al., 2016), and our design specifically and deliberately includes tasks that have been proposed by critics of WM training as candidate active control tasks (Redick et al., 2013). With a carefully-designed active control, we can be confident that improvements seen following our intervention are not simply a reflection of non-Gf effects such as demand characteristics (Orne, 1962), low-level perceptual learning or Hawthorne effects (McCarney et al., 2007). Because interaction with experimenters has been shown to influence participant performance, our active control participants engaged in a similar overall experience to those in the intervention condition (same experimental setting, similar daily and overall training duration), which also controls for potential history effects (Shipstead et al., 2010).
In particular, our Active Control tasks were designed to recruit different cognitive functions and brain networks than those recruited by EFs or Gf tasks—namely sensory and perceptual networks. In our efforts to maintain engagement levels in the Active Control group relative to the FAST training group, we incorporated a range of difficulty levels in each of the Active Control tasks, with trials ranging from very easy to very difficult. We also created a large set of tasks in an effort to allow for variety in the training, as a countermeasure against the relatively monotonous nature of the tasks. We expected performance on the control tasks to improve with training, but that improvements on these tasks would not generalize to Gf task performance.
In the Active Control condition participants alternated between three, two-alternative forced choice tasks typically used to study low-level visual processing, implemented with adaptive difficulty (e.g., changes in the signal-to-noise ratio of stimuli; Figure 2): an Adaptive Visual Search task requiring the indication of whether a target letter “F" is facing to the left or the right [modeled after an active control condition recommended by critics of cognitive enhancement research (Redick et al., 2013)], a Silo Detection task requiring the identification of a triangular configuration of silos, and a Gabor “thumbprint" Detection task requiring the identification of a Gabor patch as being on the left or right half of a screen.
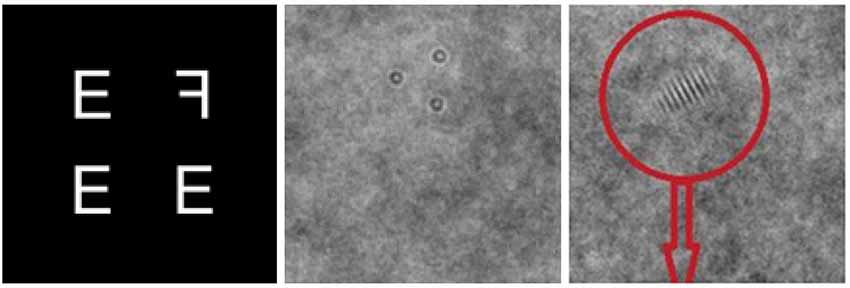
Figure 2. Example trials from the Adaptive Visual Search task (left), the Silo Detection task (middle) and the Gabor (“Fingerprint") detection task (right).
Similar to Robot Factory, in each task participants were asked to respond with either the Left Shift or Right Shift key, and task difficulty was manipulated based on participant performance. In the Adaptive Visual Search task there were 12 levels of difficulty based on: (i) the size of the letter-array in which the target letter was presented (2×2 to 12×12, in steps of 2); and (ii) whether the distractor letters were homogenous or selected from a random array of four letters (heterogeneous). Participants were moved to a higher level of difficulty if they achieved 87.5% accuracy or higher, and were moved to a lower level of difficulty if their performance dropped to 75% or below. In both the Silo and Gabor Detection tasks, difficulty was manipulated by changes to the signal-to-noise ratio (starting point: 0.5), with an incorrect trial resulting in an increase in stimulus coherence of 0.02, and two consecutive correct trials resulting in a decrease of coherence of 0.02. In all cases, participants had up to 3 s to respond, and trials terminated upon participant response. Difficulty levels at each session were based on the last level achieved on the previous day, and feedback was provided following each task block (all tasks: percent accuracy and average response latency; Silo and Gabor Detection tasks: latest task difficulty level). Throughout training, participants heard the same thematic music (via headphones) as that presented to participants in Robot Factory.
Gf Tests
Three standardized Gf tests were used for our pre- and post-test assessment of Gf: (1) the “advanced-short” version of the BOMAT, (2) Raven’s APM (Raven’s), and (3) a third matrix reasoning test with a large corpus of problems of varying difficulty adapted from Raven’s, developed by Sandia National Laboratories (Albuquerque, NM, USA), which we will henceforth term the Sandia test. The tests were performed in this order at both pre- and post-test for all participants.
Each test is made up of visual analogy problems in which a single item is missing from a complex matrix containing patterns varying in object shape, size, fill and orientation. For each test problem, the task is to select which of six (BOMAT) or eight (Raven’s, Sandia) possible response fits within the matrix. In the BOMAT and Raven’s tests, items increase in difficulty successively, whereas items of differing difficulty are randomly intermixed in the Sandia. Given constraints on the duration of pre- and post-tests, performance time for each Gf test was limited to 15 min (45 min total for all three tests), an approach that has been taken in well-known previous work (Jaeggi et al., 2008). This duration is shorter than standard administration times for the BOMAT and Raven’s (typically 45 min each), thus further increasing the demands of the tests on our participants. Participants were informed of the time limit of each test, and were instructed to solve as many problems as they could during the duration, while also being accurate. Even with this constraint, our participants performed sufficiently well overall that some performed at or close to ceiling at pre-test for the Raven’s (with mean performance at pre-test lying 1.89 standard deviations below maximum score, as compared with 6.99 SDs for the BOMAT and 3.26 SDs for the Sandia).
Bochumer Matrizen-Test (BOMAT)
The “advanced-short” version of BOMAT (Hossiep et al., 2001) is a nonverbal test of inductive and deductive reasoning, similar in style and structure to the widely used Raven’s APM (Raven’s). Like Raven’s, the advanced-short BOMAT is designed to differentiate those on the higher end of the Gf scale and is particularly useful for testing highly intelligent individuals. Published A and B versions of the BOMAT were used for pre- and post-test, each of which consists of 29 items (one example and 28 test items of increasing difficulty). The BOMAT parallel forms were randomly counterbalanced across pre- and post-test sessions. BOMAT matrices are presented in a 3 × 5 format.
Raven’s Advanced Progressive Matrices (Raven’s)
Raven’s APM (Raven and Court, 1998) is the most common test of nonverbal abstract reasoning ability. It is a nonverbal assessment that can be used to assess intellectual efficiency, high-level observation skills, clear thinking ability, and intellectual capacity. We divided the test into two parallel versions, each containing 17 test items of increasing difficulty, by approximating an even-odd distribution. The resulting distribution of item difficulty was similar for both versions. Raven’s matrices are presented in a 3 × 3 format.
Sandia
Sandia matrices overcome the issue of a limited number of stimuli by providing the option to choose from a pool of approximately 3,000 matrices, obtained through the combination of different stimulus features like shape, color and orientation (Matzen et al., 2010). Experimental matrices belong to four different classes, based on the type and number of analogical operations required for a correct solution (1-, 2-, 3- relations, and logical matrices). Parallel versions of the test were created based on stimulus classes, which in our primary study contained 42 test items. Participants were limited to no more than 1 min on each test item given the relatively large n in the test. Sandia matrices are presented in a 3 × 3 format.
Data Analysis
The overarching goal of our analysis was to determine whether participants’ Gf ability improved as a result of our intervention (FAST+tRNS) compared to active (Active Control) and passive (No-contact) control conditions. Gf ability in this study was measured by performance on three Gf tests: BOMAT, Raven’s and Sandia. We calculated accuracy on these three tests at pre- and post-test (number of correct responses/total possible), and standardized those scores. We then created a measure of a single factor (Gf) from those scores via confirmatory factor analysis, with Varimax (orthogonal) rotation and Bartlett’s weighted least-squares scores. Our factor analysis was constrained to a single factor, and explained a total of 49% of the variance in scores at pre-test, and 61.7% of the variance in scores at post-test (Table 1).
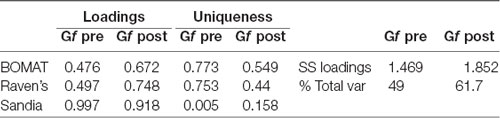
Table 1. Loadings and uniqueness (left), and sum of squared loadings and % total variance (right) for the Gf factor at pre- and post-test.
We then regressed the Gf factor at post-test on both the Gf factor at pre-test and Condition, based on the results of an Adjusted R2 model selection process (Table 2). The resulting β coefficients of our model represent the effect size of the FAST+tRNS training condition relative to each of the two control conditions.
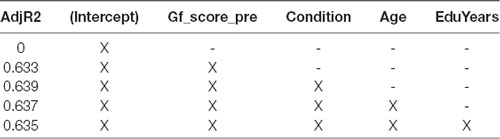
Table 2. Model selection by Adjusted R2, for regression of post-test Gf potentially controlling for Gf score at pre-test, age and years of education.
To better understand the drivers of change in Gf from pre- to post-test, we examined the correlation of progression in our training (FAST+tRNS) and Active Control conditions with changes in Gf, as well as the relationship of performance in individual EF tasks and changes in Gf.
Results
Examining Performance Across Suite of Gf Tests
Our first set of analyses focused on the critical question of whether participants in the FAST-tRNS condition showed greater Gf test performance at post-test relative to controls (Figure 3).
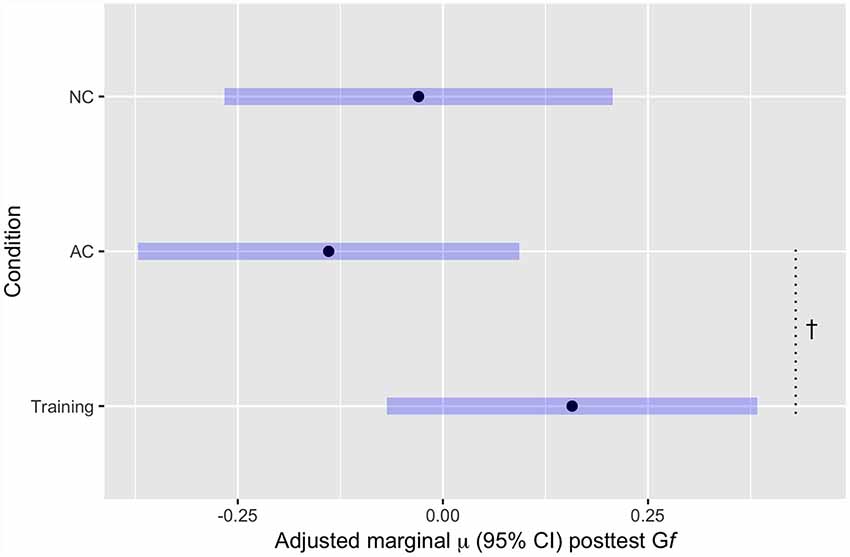
Figure 3. Mean Gf post-test scores, adjusted for estimated baseline Gf ability, by Condition. †p < 0.1.
These results show greater predicted Gf at post-test (controlling for pre-test Gf) for the training group (FAST+tRNS) compared to both control groups, but neither difference reached statistical significance [FAST+tRNS vs. No-contact control: β = 0.187, 95% CI (−0.14, 0.51), p = 0.26; FAST+tRNS vs. Active Control: β = 0.3, 95% CI (−0.03, 0.62), p = 0.07; Figure 3]. No significant difference was found between the control conditions [No-Contact vs. Active Control, β = 0.11, 95% CI (−0.22, 0.44), p = 0.51].
Examining Performance Within Each Gf Test
Our study utilized three tests of Gf as a means of better isolating a single latent factor (Gf), but the results of our factor analysis indicated relatively high uniqueness of both our BOMAT and Raven’s tests relative to the Sandia (Table 1). Given this, our next analyses examined whether changes from pre- to post-test were differentially represented across our three Gf tests. Here, we looked at standardized accuracy measures on each of the three Gf tests, controlling for pre-test Gf and Condition (Figure 4). A Bonferroni correction for multiple comparisons was used in these analyses.
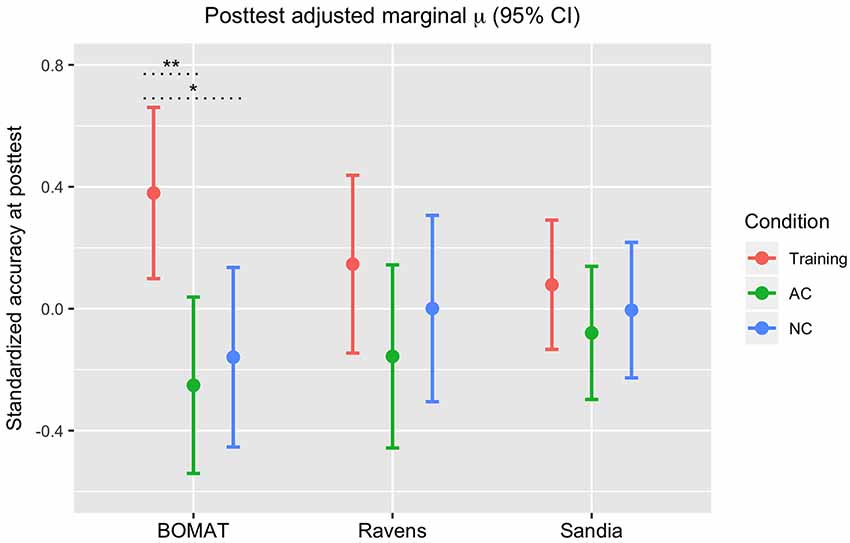
Figure 4. Standardized accuracy scores on each Gf post-test (BOMAT, Raven’s, Sandia), adjusted for estimated baseline Gf ability, by Condition. *p < 0.05, **p < 0.01, with Bonferroni correction.
BOMAT: we found significant differences in performance (standardized accuracy) between the FAST+tRNS training condition and both the No-contact [β = 0.54, 95% CI (0.13 0.946), p < 0.05] and Active Control conditions (β = 0.63, 95% CI (0.23, 1.03), p < 0.01), and no significant difference between the control conditions [β = 0.09, 95% CI (−0.32, 0.5), p = 0.66]. Ravens: we found no significant differences in performance (standardized accuracy) between the FAST+tRNS training condition and the No-contact [β = 0.15, 95% CI (−0.28, 0.57), p = 0.5] or Active Control conditions [β = 0.3, 95% CI (−0.12, 0.72), p = 0.16], and no significant difference between the control conditions [β = 0.16, 95% CI (−0.27, 0.59), p = 0.47]. Sandia: we found no significant differences in performance (standardized accuracy) between the FAST+tRNS training condition and the No-contact [β = 0.08, 95% CI (−0.22, 0.39), p = 0.6] or Active Control conditions [β = 0.16, 95% CI (−0.15, 0.46), p = 0.31], and no significant difference between the control conditions [β = 0.07, 95% CI (−0.24, 0.39), p = 0.64]. Altogether, we found numeric increases in post-test performance for the FAST+tRNS group in all three tests, but significant differences in performance only in the BOMAT.
The Positive Impact of Training on Performance
Our next analysis focused on the FAST+tRNS group alone. If our FAST+tRNS training is effective at improving Gf, then we can hypothesize that an individual’s degree of progression through the Robot Factory game should be predictive of the level of improvement in Gf task performance from pre- to post-test. This is a strong prediction, since we might expect variation across participants in their level of motivation or effort to create a positive correlation with both pre-test score and FAST progression, acting against the predicted increase in post-test Gf performance with training. To test this prediction, we ran a regression analysis of FAST+tRNS participants’ Gf at post-test, as a function of progress in our training game, Robot Factory. Depending on whether FAST+tRNS participants completed 9, 10 or 11 days of training, they engaged in 135, 150 or 165 2-min “shifts" of Robot Factory tasks (15 shifts per day). Progress in Robot Factory was therefore defined as the proportion of those shifts “passed” by a participant, with accuracy >80%. Because Robot Factory was designed to be increasingly challenging, with more complex tasks occurring only after participants had mastered simpler tasks, a greater proportion of shifts passed meant faster progress through Robot Factory, to more difficult task types.
Here, we modeled post-test Gf as a function of progress in Robot Factory (RF) and estimated baseline Gf ability. Results of these analyses found a significant positive correlation between progress in Robot Factory and Gf at post-test [β = 4.63, 95% CI (1.74 7.53), p < 0.001], with participants who progressed further through the FAST+tRNS training showing greater pre- to post-test change in Gf (Figure 5).
Changes in Executive Function Performance During Training
Given that Robot Factory is comprised of a series of tasks targeting EF practice, which serve as part of the foundation for improvements in Gf, our final set of FAST+tRNS analyses examined changes in EF performance during training in Robot Factory. Because progression through Robot Factory (and corresponding task difficulty) was controlled by individual participant performance, a direct comparison of performance by day could not be made, as tasks on Day n for some participants might be significantly harder than tasks on the same day for other participants. Rather than comparing by training time point, our approach was to compare changes within individual participant performance, from the first of their trials in single executive function (single-EF) tasks to the last of their single-EF trials. With this metric, we can examine improvements in EF performance for each individual participant, which are relative to each individual’s progress.
For this analysis, we examined performance in single-EF tasks (excluding those tasks/shifts which combined multiple EFs) and compared the average of the first of trials for each participant with the average of the last of trials for each participant (Figure 6). We measured change in terms of improvement in n-back (update) task accuracy, reduction in switch costs, and decrease in estimated stop-signal reaction time (SSRT) in the stop-signal (inhibit) task (Logan and Cowan, 1984), such that positive values indicated improvement with training for all three measures. The results of this analysis found only a numerical increase in accuracy in the update task (t(69) = 1.00, p = 0.16), and significant decreases in switch cost RT (t(70) = 2.90, p < 0.01) and SSRT (t(70) = 6.12, p < 0.0001).
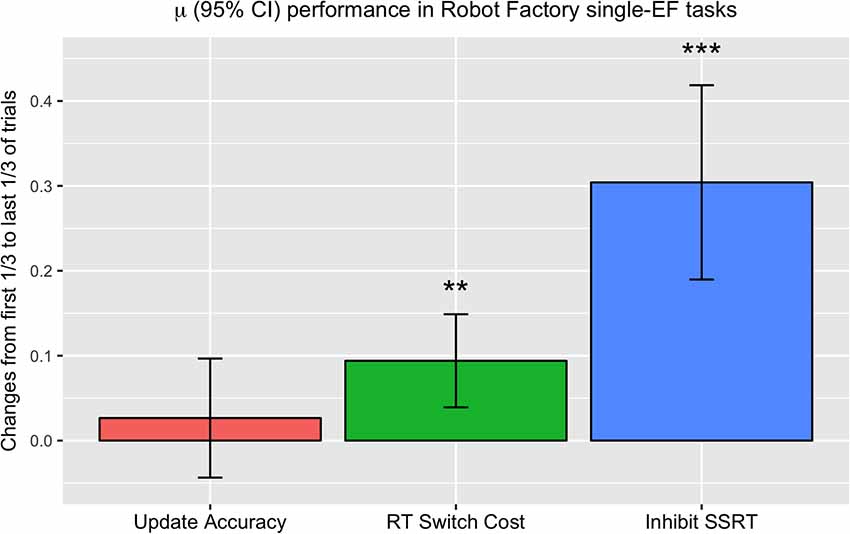
Figure 6. Changes in performance in the first of each participant’s trials in single-EF tasks to their last of trials in single-EF tasks. Measures are plotted so that positive values indicate improvement with training, giving the degree of accuracy improvement in Update trials (left), degree of decrease in average RT switch cost in Switch trials (middle), and degree of reduction in average stop-signal reaction time (SSRT) in Inhibit trials (right). **p < 0.01, ***p < 0.001.
If improvements in EF performance are part of the underlying mechanisms of Gf improvement, then we would expect the changes we see in EF metrics to correlate with Gf performance. In our final analyses, we examined Gf at post-test, as a function of baseline Gf and improvement in EF performance during training (individually added to separate models). From this we found no significant correlations among the individual tasks [n-back accuracy, β = 0.71, 95% CI (−0.41, 1.84), p = 0.21; decrease in switch cost RT, β = 0.3, 95% CI (−1.2, 1.78), p = 0.69; decrease in SSRT, β = 0.29, 95% CI (−0.41, 1.0), p = 0.4]. Overall, our analyses found that EF task performance within Robot Factory improved during training, but individual improvement in EFs did not significantly correlate with changes in Gf.
Does Progress in Active Control Tasks Also Lead to Improvements?
Finally, given that progress in Robot Factory strongly predicted Gf gains from pre- to post-test, a reasonable question to ask is whether there was a similar impact of progress in our Active Control tasks on Gf test performance. If so, it might suggest that the positive correlation between Robot Factory progress and Gf gain reflects non-specific effects of adaptive training such as change in motivation. To answer this, we conducted a final analysis of only those participants who engaged in AC training, modeling their post-test Gf as a function of progress in Active Control tasks, controlling for Gf at pre-test. Here, progress in AC tasks was represented by the average difficulty level achieved on each of the three AC tasks on the last day of training (difficulty levels ranged throughout each training session), summed into a single score per participant. In these analyses, two participants had to be excluded based on recording errors in two or more of their AC tasks (final AC group, n = 28). Importantly, no significant correlation was found between progress in the Active Control tasks and Gf post-test [β = −0.003, 95% CI (−0.06, 0.05), p = 0.91]: this negative-signed and very weak correlation between AC training progression and Gf gain contrasts with the strong, positive correlation involving Robot Factory progression, providing reassurance that the latter reflects a meaningful, specific effect of this form of training on Gf test performance.
Discussion
The present study introduces a novel cognitive training intervention—Flexible, Adaptive, Synergistic Training (FAST)—that is designed to enhance Gf through targeted, varied training of core EFs combined with brain stimulation. In the new Robot Factory game, EFs of working memory, cognitive flexibility, and inhibition are exercised in increasingly complex configurations and diverse task environments, reliant on dynamic task instructions. The FAST+tRNS intervention pairs this EF training with bifocal tRNS over DLPFC, a brain region that is a core component of a distributed fronto-parietal network strongly implicated in EF and Gf (Duncan and Owen, 2000; Duncan et al., 2012). Together, this combination of EF training and targeted stimulation represents an approach that aims to target Gf networks through synergistic activation by brain stimulation and EF practice, increasing activity during a critical state of enhanced plasticity. Our results suggest that the FAST+tRNS intervention may be effective in improving Gf task performance compared against both no-contact controls, and, critically, against an active control group that engages in a similarly extended and challenging training regime targeting lower-level cognitive functions. Such improvements relative to an active control are fairly rare in the literature (Buschkuehl and Jaeggi, 2010; Shipstead et al., 2010; Dougherty et al., 2016), highlighting the importance of the comparison presented in this work. It should be noted that the central aim of the experiment described here was a feasibility study of a compound intervention consisting of a game and cortical stimulation—hence, the relative contribution of the individual elements of the intervention are difficult to establish and will have to be assessed in future confirmatory research.
The results of the study suggest transfer from the EF skills exercised in Robot Factory to matrix-reasoning of the Gf tests. Our analyses showed improvements in EF task performance during training, as well as significant correlations of game progression and Gf post-test performance. Our results also found numerically greater gains in Gf for our FAST+tRNS training condition compared to both controls (Active Control, No-Contact Control), with a marginally significant difference between training and the Active Control Condition (no significant difference between training and No-Contact). Analysis of the individual Gf tests found that these numerical gains were seen in all tests, but were driven most notably by significant increases in performance of the FAST+tRNS training group in the BOMAT, relative to both controls.
By comparing our FAST+tRNS intervention to a similarly-challenging training provided by the Active Control condition, we provide evidence against the interpretation that our training gains are the result of demand characteristics or a Hawthorne effect (Roethlisberger and Dickson, 1939; Sommer, 1968; Parsons, 1974). Furthermore, because our Active Control condition was carefully designed to emphasize low-level visual-spatial processing, the comparison of the FAST training-only and AC conditions effectively isolates the impact of our training on cognitive control processes (vs. increases in processing speed or visual search). These results offer initial evidence that visuospatial processing is not the main driver of improvements we see following our FAST intervention, though spatial working memory confounds resulting from the different matrix-sizes among the Gf tests remain (Moody, 2009).
While correlation analyses indicate that improvements in Gf task performance from pre- to post-test were strongly predicted by participants’ degree of progression through the FAST game (Robot Factory), progression in Active Control tasks was not correlated with such gains. This association is unlikely to reflect confounding effects of underlying Gf ability or level of motivation, both of which could be expected to affect pre-test Gf performance as much as post-test. Instead, the association can be understood in terms of the design of the Robot Factory training game, in which participants are given successively more complex combinations of EF training the further they progress. Progression through the game is determined by performance, so individuals who do well in simpler initial tasks are moved more quickly to more complex, higher-level tasks. This leaves these individuals more time to practice dual and even triple-EF tasks, as well as tasks with a dual n-back or hypothesis-testing component. These more difficult Robot Factory tasks require the most complex and structured organizations of component EFs and require them to be assembled rapidly with minimal instruction. We hypothesize that it is at these highest and most challenging levels of the game that participants are acquiring cognitive skills most relevant to Gf task performance.
As this study served as an initial proof-of-concept for our training approach, additional work in this research program is currently underway tackling several important issues not specifically addressed in this study3. First, it is critical that the relative contributions of the FAST game (Robot Factory) and tES (here, tRNS) be more thoroughly explored. In this work, we cannot definitively say whether the gains we see are specifically the result of our training game alone, its combination with our stimulation protocol (or expectation effects induced by NIBS), or some combination thereof. A key aim of our future research will be to compare our FAST intervention with NIBS against a condition with Robot Factory training and sham stimulation, as well as to contemplate the effect of different forms and doses of NIBS. Nevertheless, these results do offer initial evidence for the possibility of enhancing Gf through a short but intensive cognitive training regimen.
Regarding our training game, Robot Factory, a natural question is which of its many constituent components might be crucial to producing the observed Gf gains: the requirement to combine EFs, to combine them in novel ways repeatedly over the course of training sessions adapted to participants’ abilities, to do so rapidly and with minimal verbal instruction, or to engage in hypothesis-testing to derive complex tasks from feedback. This issue can be addressed in future research by leveraging the complexity and richness of our training intervention to support an examination of relative contributions of the various elements of the training, provided the study has enough power (McKanna et al., under preparation).
Overall, the present research represents a potentially useful step forward in providing new insights into, and new methods for studying, the nature of Gf and its malleability. Though our results await replication and extension, they provide preliminary evidence that while Gf depends, as previously suggested, on core EFs of working memory, cognitive flexibility and inhibition, the crucial characteristic of Gf may in fact be the ability to combine these functions rapidly and adaptively according to changing demand. More intriguing still, the ability to do so may be susceptible to targeted training that can lead to improvements in Gf, with consequent implications for our understanding of the nature of intelligence as well as practical implications in light of the known predictive relationship between Gf and a host of significant life outcomes.
Concluding Remarks
While there has been widespread research interest in both characterizing the neural mechanisms underlying Gf, and in developing effective and practical interventions, researchers have called for careful scrutiny and appropriate skepticism in assessing claims regarding the efficacy of interventions aimed at enhancing Gf. These cautions are also relevant in the interpretation of the results presented here. The present study represents a preliminary test of a specific, theoretically-motivated hypothesis about activities that are likely to enhance Gf. While results show promise, and reveal directions for future research, they do not form a sufficient basis for using the interventions described in clinical, educational, or personal enhancement contexts.
The study presented here reflects a limited set of performance measures, from a limited number of training conditions. The authors make no claims beyond the population of this study, but cite these preliminary results as evidence of the potential of enhancing Gf through cognitive training. Future work will further explore the efficacy of FAST, comparing the impact of synergistic behavioral training + NIBS against training + sham stimulation, to determine the effects of expectation. Currently no plans exist for marketing of FAST or related trainings.
Ethics Statement
In the US, these studies were approved by the institutional review board at all participating institutions (Harvard BIDMC: Committee on Clinical Investigations/IRB, Protocol 2014P-000024; Northeastern: Human Subject Research Protection/IRB, Protocol 14-08-15) and in the UK by the Berkshire National Research Ethics Committee (REC reference 14/SC/0131). All participants gave written informed consent prior to training, according to the Declaration of Helsinki, and were remunerated for their participation (£163; 12 and $11–20 per hour depending on site, in the UK and the US, respectively).
Author Contributions
SM was the Principal Investigator and Program Manager of the SHARP Project. SM, AP-L, RC, MP and NY were responsible for the conceptualization of the SHARP Project, its funding acquisition and supervision. JA was responsible for the formal analysis, visualization and original draft writing of this manuscript. Review and editing of this manuscript was done by SM, JA, A-KB, FP, JM, ES, RC and NY. Investigations underlying this work were conducted by SM, JA, A-KB, FP, JM, ES, AP-L, RC, MP and NY, and the methodology employed here was co-determined by SM, JA, MP and NY.
Funding
This research was supported by the Intelligence Advanced Research Projects Activity (IARPA) via contract #2014413121700007 to Honeywell. The United States Government is authorized to reproduce and distribute reprints for Governmental purposes notwithstanding any copyright annotation thereon. The funder provided support in the form of salaries for all authors, but did not have any additional role in the study design, data collection and analysis, decision to publish, or preparation of the manuscript. The specific roles of these authors are articulated in the author contributions section. The views and conclusions contained herein are those of the authors and should not be interpreted as necessarily representing the official policies or endorsements, either expressed or implied, of IARPA, the Office of the Director of National Intelligence, or the U.S. Government.
Conflict of Interest Statement
Prof. AP-L and Prof. RC serve on the scientific advisory board of Neuroelectrics Inc. Prof. AP-L also serves on the scientific advisory boards for Nexstim, Neuronix, Starlab Neuroscience, Axilum Robotics, Magstim Inc. and Neosync. These affiliations do not alter our adherence to Frontiers’ policies on sharing data and materials. Prof. AP-L is listed as an inventor on several issued and pending patents on the use of transcranial magnetic stimulation, and the real-time integration of transcranial magnetic stimulation with electroencephalography and magnetic resonance imaging: Transcranial magnetic stimulation (tms) methods and apparatus (US 13/744,869; PCT/US2007/024694), Method and apparatus for recording an electroencephalogram during transcranial magnetic stimulation (US 09/067,111; US 09/746,055; PCT/US1999/008489), A method and a system for optimizing the configuration of multisite transcranial current stimulation and a computer readable medium and a computer program (US 14/058,517; PCT/IB2014/002180; EP20140793891), Identifying individual target sites for transcranial magnetic stimulation applications (US 14/401,296; PCT/US2013/032673), Method and apparatus for monitoring a magnetic resonance image during transcranial magnetic stimulation (US 09/096,725; PCT/US1999/013051). Prof. RC also led a patent entitled Apparatus for improving and/or maintaining numerical ability (US 13/578,125; PCT/GB2011/050211; EP20110702868). These patents do not alter our adherence to Frontiers’ policies on sharing data and materials. Listed authors SM and JA, and Honeywell SHARP Team authors Michael Dillard, and Umut Orhan are or were employed by the Honeywell Corporation at the time of authorship. Honeywell SHARP Team authors Garrett Kimball and Eben Myers are employed by the company Simcoach games. These commercial affiliations do not alter our adherence to Frontiers’ policies on sharing data and materials.
The remaining authors declare that the research was conducted in the absence of any commercial or financial relationships that could be construed as a potential conflict of interest.
Acknowledgments
We would like to thank the members of the larger Honeywell SHARP team for their valuable contributions to this work, including the SHARP Team authors: (Harvard Medical School) Ann Connor, FP, ES, AP-L; (Honeywell) JA, Michael Dillard, Umut Orhan, Dennis Cornhill, SM; (Northeastern University) JM, Deniz Erdogmus, MP; (Oxford) A-KB, RC, Karen Mansfield, NY; (SimCoach Games) Garrett Kimball, Eben Myers, and other key contributors: (Oxford) Giulia Maistrello, Amar Sarkar, Ruairidh Battleday, Kalaiyashni Puvanendran, Morio Hamada; (Northeastern University) Sadegh Salehi, Yeganeh Marghi, and Asieh Ahani.
Footnotes
- ^ http://www.neuroelectrics.com/download/NE_UM_Part02_Electrode.pdf
- ^ www.simcoachgames.com
- ^ https://osf.io/26aun/
References
Au, J., Sheehan, E., Tsai, N., Duncan, G. J., Buschkuehl, M., and Jaeggi, S. M. (2014). Improving fluid intelligence with training on working memory: a meta-analysis. Psychon. Bull. Rev. 22, 366–377. doi: 10.3758/s13423-014-0699-x
Borckardt, J. J., Bikson, M., Frohman, H., Reeves, S. T., Datta, A., Bansal, V., et al. (2012). A pilot study of the tolerability and effects of high-definition transcranial direct current stimulation (HD-tDCS) on pain perception. J. Pain 13, 112–120. doi: 10.1016/j.jpain.2011.07.001
Burgess, G. C., Gray, J. R., Conway, A. R., and Braver, T. S. (2011). Neural mechanisms of interference control underlie the relationship between fluid intelligence and working memory span. J. Exp. Psychol. Gen. 140, 674–692. doi: 10.1037/a0024695
Buschkuehl, M., and Jaeggi, S. M. (2010). Improving intelligence: a literature review. Swiss Med. Wkly. 140, 266–272.
Cappelletti, M., Gessaroli, E., Hithersay, R., Mitolo, M., Didino, D., Kanai, R., et al. (2013). Transfer of cognitive training across magnitude dimensions achieved with concurrent brain stimulation of the parietal lobe. J. Neurosci. 33, 14899–14907. doi: 10.1523/JNEUROSCI.1692-13.2013
Carpenter, P. A., Just, M. A., and Shell, P. (1990). What one intelligence test measures: a theoretical account of the processing in the Raven Progressive Matrices Test. Psychol. Rev. 97, 404–431. doi: 10.1037/0033-295x.97.3.404
Cattell, R. B. (1971). Abilities: Their Structure, Growth, and Action. New York, NY: Houghton Mifflin.
Chaieb, L., Paulus, W., and Antal, A. (2011). Evaluating aftereffects of short-duration transcranial random noise stimulation on cortical excitability. Neural Plast. 2011:105927. doi: 10.1155/2011/105927
Chase, W. G., and Ericsson, K. A. (1982). Skill and working memory. Psychol. Learn. Motiv. 16, 1–58. doi: 10.1016/S0079-7421(08)60546-0
Chooi, W. T., and Thompson, L. A. (2012). Working memory training does not improve intelligence in healthy young adults. Intelligence 40, 531–542. doi: 10.1016/j.intell.2012.07.004
Cohen Kadosh, R. (2015). Modulating and enhancing cognition using brain stimulation: science and fiction. J. Cogn. Psychol. 27, 141–163. doi: 10.1080/20445911.2014.996569
Cole, M. W., Laurent, P., and Stocco, A. (2013). Rapid instructed task learning: a new window into the human brain’s unique capacity for flexible cognitive control. Cogn. Affect. Behav. Neurosci. 13, 1–22. doi: 10.3758/s13415-012-0125-7
Cole, M. W., Yarkoni, T., Repovs, G., Anticevic, A., and Braver, T. S. (2012). Global connectivity of prefrontal cortex predicts cognitive control and intelligence. J. Neurosci. 32, 8988–8999. doi: 10.1523/JNEUROSCI.0536-12.2012
Collins, A., and Koechlin, E. (2012). Reasoning, learning, and creativity: frontal lobe function and human decision-making. PLoS Biol. 10:e1001293. doi: 10.1371/journal.pbio.1001293
Deary, I. J., Strand, S., Smith, P., and Fernandes, C. (2007). Intelligence and educational achievement. Intelligence 35, 13–21. doi: 10.1016/j.intell.2006.02.001
Diamond, A. (2013). Executive functions. Annu. Rev. Psychol. 64, 135–168. doi: 10.1146/annurev-psych-113011-143750
Donders, F. C. (1969). On the speed of mental processes. Acta Psychol. 30, 412–431. doi: 10.1016/0001-6918(69)90065-1
Dougherty, M. R., Hamovitz, T., and Tidwell, J. W. (2016). Reevaluating the effectiveness of n-back training on transfer through the Bayesian lens: support for the null. Psychon. Bull. Rev. 23, 306–316. doi: 10.3758/s13423-015-0865-9
Dumontheil, I., Thompson, R., and Duncan, J. (2011). Assembly and use of new task rules in fronto-parietal cortex. J. Cogn. Neurosci. 23, 168–182. doi: 10.1162/jocn.2010.21439
Duncan, J., and Owen, A. M. (2000). Common regions of the human frontal lobe recruited by diverse cognitive demands. Trends Neurosci. 23, 475–483. doi: 10.1016/s0166-2236(00)01633-7
Duncan, J., Schramm, M., Thompson, R., and Dumontheil, I. (2012). Task rules, working memory, and fluid intelligence. Psychon. Bull. Rev. 19, 864–870. doi: 10.3758/s13423-012-0225-y
Faria, P., Fregni, F., Sebastião, F., Dias, A. I., and Leal, A. (2012). Feasibility of focal transcranial DC polarization with simultaneous EEG recording: preliminary assessment in healthy subjects and human epilepsy. Epilepsy Behav. 25, 417–425. doi: 10.1016/j.yebeh.2012.06.027
Fertonani, A., Pirulli, C., and Miniussi, C. (2011). Random noise stimulation improves neuroplasticity in perceptual learning. J. Neurosci. 31, 15416–15423. doi: 10.1523/JNEUROSCI.2002-11.2011
Friedman, N. P., Miyake, A., Young, S. E., DeFries, J. C., Corley, R. P., and Hewitt, J. K. (2008). Individual differences in executive functions are almost entirely genetic in origin. J. Exp. Psychol. Gen. 137, 201–225. doi: 10.1037/0096-3445.137.2.201
Gottfredson, L. S. (1997). Why g matters: the complexity of everyday life. Intelligence 24, 79–132. doi: 10.1016/s0160-2896(97)90014-3
Gray, J. R., and Thompson, P. M. (2004). Neurobiology of intelligence: science and ethics. Nat. Rev. Neurosci. 5, 471–482. doi: 10.1038/nrn1405
Halford, G. S., Cowan, N., and Andrews, G. (2007). Separating cognitive capacity from knowledge: a new hypothesis. Trends Cogn. Sci. 11, 236–242. doi: 10.1016/j.tics.2007.04.001
Harrison, T. L., Shipstead, Z., Hicks, K. L., Hambrick, D. Z., Redick, T. S., and Engle, R. W. (2013). Working memory training may increase working memory capacity but not fluid intelligence. Psychol. Sci. 24, 2409–2419. doi: 10.1177/0956797613492984
Hossiep, R., Turck, D., and Hasella, M. (2001). BOMAT-Advanced-Short Version: Bochumer Matrizentest. Göttingen, Germany: Hogrefe Verlag fur Psychologie.
Jaeggi, S. M., Buschkuehl, M., Jonides, J., and Perrig, W. J. (2008). Improving fluid intelligence with training on working memory. Proc. Natl. Acad. Sci. U S A 105, 6829–6833. doi: 10.1073/pnas.0801268105
Jaeggi, S. M., Buschkuehl, M., Shah, P., and Jonides, J. (2014). The role of individual differences in cognitive training and transfer. Mem. Cognit. 42, 464–480. doi: 10.3758/s13421-013-0364-z
Jung, R. E., and Haier, R. J. (2007). The Parieto-Frontal Integration Theory (P-FIT) of intelligence: converging neuroimaging evidence. Behav. Brain Sci. 30, 135–154. doi: 10.1017/s0140525x07001185
Kane, M. J., Hambrick, D. Z., and Conway, A. R. (2005). Working memory capacity and fluid intelligence are strongly related constructs: comment on Ackerman, Beier, and Boyle. Psychol. Bull. 131, 66–71. doi: 10.1037/0033-2909.131.1.66
Karbach, J., and Verhaeghen, P. (2014). Making working memory work a meta-analysis of executive-control and working memory training in older adults. Psychol. Sci. 25, 2027–2037. doi: 10.1177/0956797614548725
Kim, J., and Mueller, C. W. (1978). Introduction to Factor Analysis: What it is and How to do it. Beverly Hills, CA: SAGE Publications, Inc.
Krause, B., and Cohen Kadosh, R. (2013). Can transcranial electrical stimulation improve learning difficulties in atypical brain development? A future possibility for cognitive training. Dev. Cogn. Neurosci. 6, 176–194. doi: 10.1016/j.dcn.2013.04.001
Lampit, A., Hallock, H., and Valenzuela, M. (2014). Computerized cognitive training in cognitively healthy older adults: a systematic review and meta-analysis of effect modifiers. PLOS Med. 11:e1001756. doi: 10.1371/journal.pmed.1001756
Logan, G. D., and Cowan, W. B. (1984). On the ability to inhibit thought and action: a theory of an act of control. Psychol. Rev. 91, 295–327. doi: 10.1037/0033-295x.91.3.295
Looi, C. Y., Duta, M., Brem, A. K., Huber, S., Nuerk, H. C., and Cohen Kadosh, R. (2016). Combining brain stimulation and video game to promote long-term transfer of learning and cognitive enhancement. Sci. Rep. 6:22003. doi: 10.1038/srep22003
Lunt, L., Bramham, J., Morris, R. G., Bullock, P. R., Selway, R. P., Xenitidis, K., et al. (2012). Prefrontal cortex dysfunction and ‘jumping to conclusions’: bias or deficit? J. Neuropsychol. 6, 65–78. doi: 10.1111/j.1748-6653.2011.02005.x
Matzen, L. E., Benz, Z. O., Dixon, K. R., Posey, J., Kroger, J. K., and Speed, A. E. (2010). Recreating Raven’s: software for systematically generating large numbers of Raven-like matrix problems with normed properties. Behav. Res. Methods 42, 525–541. doi: 10.3758/brm.42.2.525
McCarney, R., Warner, J., Iliffe, S., van Haselen, R., Griffin, M., and Fisher, P. (2007). The Hawthorne Effect: a randomised, controlled trial. BMC Med. Res. Methodol. 7:30. doi: 10.1186/1471-2288-7-30
Melby-Lervåg, M., and Hulme, C. (2013). Is working memory training effective? A meta-analytic review. Dev. Psychol. 49, 270–291. doi: 10.1037/a0028228
Melby-Lervåg, M., and Hulme, C. (2015). There is no convincing evidence that working memory training is effective: a reply to Au et al. (2014) and Karbach and Verhaeghen (2014). Psychon. Bull. Rev. 17, 324–330. doi: 10.3758/s13423-015-0862-z
Minhas, P., Bansal, V., Patel, J., Ho, J. S., Diaz, J., Datta, A., et al. (2010). Electrodes for high-definition transcutaneous DC stimulation for applications in drug delivery and electrotherapy, including tDCS. J. Neurosci. Methods 190, 188–197. doi: 10.1016/j.jneumeth.2010.05.007
Miranda, P. C., Faria, P., and Hallett, M. (2009). What does the ratio of injected current to electrode area tell us about current density in the brain during tDCS? Clin. Neurophysiol. 120, 1183–1187. doi: 10.1016/j.clinph.2009.03.023
Miyake, A., Friedman, N. P., Emerson, M. J., Witzki, A. H., Howerter, A., and Wager, T. D. (2000). The unity and diversity of executive functions and their contributions to complex “frontal lobe” tasks: a latent variable analysis. Cogn. Psychol. 41, 49–100. doi: 10.1006/cogp.1999.0734
Monsell, S. (1996). “Control of mental processes,” in Unsolved mysteries of the mind: Tutorial essays in cognition, ed. V. Bruce (Oxford: Taylor & Francis), 93–148.
Moody, D. E. (2009). Can intelligence be increased by training on a task of working memory? Intelligence 37, 327–328. doi: 10.1016/j.intell.2009.04.005
Morrison, A. B., and Chein, J. M. (2011). Does working memory training work? The promise and challenges of enhancing cognition by training working memory. Psychon. Bull. Rev. 18, 46–60. doi: 10.3758/s13423-010-0034-0
Murray, L. M., Edwards, D. J., Ruffini, G., Labar, D., Stampas, A., Pascual-Leone, A., et al. (2015). Intensity dependent effects of transcranial direct current stimulation on corticospinal excitability in chronic spinal cord injury. Arch. Phys. Med. Rehabil. 96, S114–S121. doi: 10.1016/j.apmr.2014.11.004
Orne, M. T. (1962). On the social psychology of the psychological experiment: with particular reference to demand characteristics and their implications. Am. Psychol. 17, 776–783. doi: 10.1037/h0043424
Owen, A. M., Hampshire, A., Grahn, J. A., Stenton, R., Dajani, S., Burns, A. S., et al. (2010). Putting brain training to the test. Nature 465, 775–778. doi: 10.1038/nature09042
Owen, A. M., McMillan, K. M., Laird, A. R., and Bullmore, E. (2005). N-back working memory paradigm: a meta-analysis of normative functional neuroimaging studies. Hum. Brain Mapp. 25, 46–59. doi: 10.1002/hbm.20131
Pahor, A., and Jaušovec, N. (2014). The effects of theta transcranial alternating current stimulation (tACS) on fluid intelligence. Int. J. Psychophysiol. 93, 322–331. doi: 10.1016/j.ijpsycho.2014.06.015
Parsons, H. M. (1974). What happened at Hawthorne? New evidence suggests the Hawthorne effect resulted from operant reinforcement contingencies. Science 183, 922–932. doi: 10.1126/science.183.4128.922
Popescu, T., Krause, B., Terhune, D. B., Twose, O., Page, T., Humphreys, G., et al. (2016). Transcranial random noise stimulation mitigates increased difficulty in an arithmetic learning task. Neuropsychologia 81, 255–264. doi: 10.1016/j.neuropsychologia.2015.12.028
Rapport, M. D., Orban, S. A., Kofler, M. J., and Friedman, L. M. (2013). Do programs designed to train working memory, other executive functions and attention benefit children with ADHD? A meta-analytic review of cognitive, academic, and behavioral outcomes. Clin. Psychol. Rev. 33, 1237–1252. doi: 10.1016/j.cpr.2013.08.005
Raven, J. C., and Court, J. H. (1998). Raven’s Progressive Matrices and Vocabulary Scales. Oxford: Oxford Psychologists Press.
Redick, T. S., Shipstead, Z., Harrison, T. L., Hicks, K. L., Fried, D. E., Hambrick, D. Z., et al. (2013). No evidence of intelligence improvement after working memory training: a randomized, placebo-controlled study. J. Exp. Psychol. Gen. 142, 359–379. doi: 10.1037/a0029082
Roethlisberger, F. J., and Dickson, W. J. (1939). Management and the Worker. Cambridge, MA: Harvard University Press.
Rogers, R. D., and Monsell, S. (1995). Costs of a predictable switch between simple cognitive tasks. J. Exp. Psychol. Gen. 124, 207–231. doi: 10.1037/0096-3445.124.2.207
Rudebeck, S. R., Bor, D., Ormond, A., O’Reilly, J. X., and Lee, A. C. (2012). A potential spatial working memory training task to improve both episodic memory and fluid intelligence. PLoS One 7:e50431. doi: 10.1371/journal.pone.0050431
Salminen, T., Strobach, T., and Schubert, T. (2012). On the impacts of working memory training on executive functioning. Front. Hum. Neurosci. 6:166. doi: 10.3389/fnhum.2012.00166
Salthouse, T. A., and Pink, J. E. (2008). Why is working memory related to fluid intelligence? Psychon. Bull. Rev. 15, 364–371. doi: 10.3758/pbr.15.2.364
Santarnecchi, E., Brem, A.-K., Levenbaum, E., Thompson, T., Cohen Kadosh, R., and Pascual-Leone, A. (2015). Enhancing cognition using transcranial electrical stimulation. Curr. Opin. Behav. Sci. 4, 171–178. doi: 10.1016/j.cobeha.2015.06.003
Santarnecchi, E., Muller, T., Rossi, S., Sarkar, A., Polizzotto, N. R., Rossi, A., et al. (2016). Individual differences and specificity of prefrontal gamma frequency-tACS on fluid intelligence capabilities. Cortex 75, 33–43. doi: 10.1016/j.cortex.2015.11.003
Santarnecchi, E., Polizzotto, N. R., Godone, M., Giovannelli, F., Feurra, M., Matzen, L., et al. (2013). Frequency-dependent enhancement of fluid intelligence induced by transcranial oscillatory potentials. Curr. Biol. 23, 1449–1453. doi: 10.1016/j.cub.2013.06.022
Schmiedek, F., Lövden, M., and Lindenberger, U. (2010). Hundred days of cognitive training enhance broad cognitive abilities in adulthood: findings from the COGITO study. Front. Aging Neurosci. 2:27. doi: 10.3389/fnagi.2010.00027
Sellers, K. K., Mellin, J. M., Lustenberger, C. M., Boyle, M. R., Lee, W. H., Peterchev, A. V., et al. (2015). Transcranial direct current stimulation (tDCS) of frontal cortex decreases performance on the WAIS-IV intelligence test. Behav. Brain Res. 290, 32–44. doi: 10.1016/j.bbr.2015.04.031
Shipstead, Z., Redick, T. S., and Engle, R. W. (2010). Does working memory training generalize. Psychol. Belg. 50, 245–276. doi: 10.5334/pb-50-3-4-245
Shipstead, Z., Redick, T. S., and Engle, R. W. (2012). Is working memory training effective? Psychol. Bull. 138, 628–654. doi: 10.1037/a0027473
Singley, M. K., and Anderson, J. R. (1985). The transfer of text-editing skill. Int. J. Man Mach. Stud. 22, 403–423. doi: 10.1016/s0020-7373(85)80047-x
Snowball, A., Tachtsidis, I., Popescu, T., Thompson, J., Delazer, M., Zamarian, L., et al. (2013). Long-term enhancement of brain function and cognition using cognitive training and brain stimulation. Curr. Biol. 23, 987–992. doi: 10.1016/j.cub.2013.04.045
Taatgen, N. A. (2013). The nature and transfer of cognitive skills. Psychol. Rev. 120, 439–471. doi: 10.1037/a0033138
Terney, D., Chaieb, L., Moliadze, V., Antal, A., and Paulus, W. (2008). Increasing human brain excitability by transcranial high-frequency random noise stimulation. J. Neurosci. 28, 14147–14155. doi: 10.1523/JNEUROSCI.4248-08.2008
Thompson, T. W., Waskom, M. L., Garel, K. L., Cardenas-Iniguez, C., Reynolds, G. O., Winter, R., et al. (2013). Failure of working memory training to enhance cognition or intelligence. PLoS One 8:e63614. doi: 10.1371/journal.pone.0063614
VanLehn, K. (1996). Cognitive skill acquisition. Annu. Rev. Psychol. 47, 513–539. doi: 10.1146/annurev.psych.47.1.513
Verhaeghen, P., and Basak, C. (2005). Ageing and switching of the focus of attention in working memory: results from a modified N-Back task. Q. J. Exp. Psychol. A 58, 134–154. doi: 10.1080/02724980443000241
Verhaeghen, P., Cerella, J., and Basak, C. (2004). A working memory workout: how to expand the focus of serial attention from one to four items in 10 hours or less. J. Exp. Psychol. Learn. Mem. Cogn. 30, 1322–1337. doi: 10.1037/0278-7393.30.6.1322
von Bastian, C. C., and Oberauer, K. (2014). Effects and mechanisms of working memory training: a review. Psychol. Res. 78, 803–820. doi: 10.1007/s00426-013-0524-6
Keywords: cognitive training, cognitive enhancement, fluid intelligence, executive function, transcranial electrical stimulation (tES), FAST
Citation: Almquist JN-F, Mathan S, Brem A-K, Plessow F, McKanna J, Santarnecchi E, Pascual-Leone A, Cohen Kadosh R, Pavel M and Yeung N (2019) FAST: A Novel, Executive Function-Based Approach to Cognitive Enhancement. Front. Hum. Neurosci. 13:235. doi: 10.3389/fnhum.2019.00235
Received: 09 January 2019; Accepted: 25 June 2019;
Published: 02 August 2019.
Edited by:
Ashok Hegde, Georgia College and State University, United StatesReviewed by:
Theodore Zanto, University of California, San Francisco, United StatesTorkel Klingberg, Karolinska Institute (KI), Sweden
Moussa Antoine Chalah, Hôpitaux Universitaires Henri Mondor, France
Copyright © 2019 Almquist, Mathan, Brem, Plessow, McKanna, Santarnecchi, Pascual-Leone, Cohen Kadosh, Pavel and Yeung. This is an open-access article distributed under the terms of the Creative Commons Attribution License (CC BY). The use, distribution or reproduction in other forums is permitted, provided the original author(s) and the copyright owner(s) are credited and that the original publication in this journal is cited, in accordance with accepted academic practice. No use, distribution or reproduction is permitted which does not comply with these terms.
*Correspondence: Santosh Mathan, c2FudG9zaC5tYXRoYW5AaG9uZXl3ZWxsLmNvbQ==