- 1Neuroscience Training Program, University of Wisconsin, Madison, WI, United States
- 2Department of Pathology and Laboratory Medicine, University of Wisconsin, Madison, WI, United States
Alzheimer's disease (AD) is the most prevalent form of late-onset dementia. AD affects the health of millions of people in the United States and worldwide. Currently, there are no approved therapies that can halt or reverse the clinical progression of AD. Traditionally, AD is characterized first by the appearance of amyloid-β (Aβ) plaques followed by the formation of intraneuronal neurofibrillary tangles (NFTs) composed of hyperphosphorylated tau (p-tau). These lesions are linked to synapse loss and eventual cognitive impairment. Additionally, microgliosis is consistently found in regions of the brain with AD pathology. The role of microglia in AD onset and progression remains unclear. Several recent reports indicate that the assembly of the multi-protein complex known as the NOD, LRR, and pyrin-domain containing 3 (Nlrp3) inflammasome by microglia results in apoptosis spec-like protein containing a CARD (Asc) spec formation, which then nucleates new Aβ plaques, thus amplifying Aβ-associated pathology. NFTs can also activate the Nlrp3 inflammasome leading to enhanced tau-associated pathology. Here, we will review the role of microglia and the activation of the inflammasome in the innate immune response to AD.
Introduction
Alzheimer's disease is the most common form of dementia. AD results in neuronal death likely caused by an accumulation of senile plaques primarily composed of amyloid-β (Aβ) peptides, first observed by Alois Alzheimer (1). Plaques promote an environment conducive to forming intraneuronal tau aggregates known as neuritic plaque tau, (NP) tau, and in more advanced stages of AD, neurofibrillary tangles (NFTs) (2, 3). Recent evidence suggests that neuroinflammation, mediated through increased levels of pro-inflammatory products released from innate immune cells, e.g., microglia, contribute to AD, and precedes Aβ plaque deposition and AD onset (4, 5). Microglial dysfunction caused by prolonged amyloid-induced microglial activation may also contribute to AD (6). Microglia are also crucial for maintenance and upholding homeostasis within the brain (7). Upon activation in numerous pathological conditions, including AD, microglial function, and morphology change dramatically (7). Monomeric and oligomeric forms of Aβ as well as tau aggregates such as NFTs activate microglia in AD (Figure 1). Additionally, activation of pattern recognition receptors (PRRs) expressed by microglia can influence AD pathology (8). Microglia and other innate immune cells express several toll-like receptors (TLRs), which when activated, subsequently result in the activation of NF-κB and the production of pro-inflammatory cytokines (9). Additionally, microglia also express several intracellular PRRs that are not membrane-bound such as nucleotide-binding domain and leucine-rich repeat-containing receptors (NLRs) family of receptors, absent in melanoma 2 (AIM2)-like receptors (ALRs) family of receptors and the tripartite motif-containing (TRIM) family member pyrin are known to initiate the assembly of the multi-protein complex inflammasome (10, 11). In most cases the inflammasome complex contains apoptosis spec like protein containing a caspase recruitment domain (Asc), and is also known as an Asc spec (12).
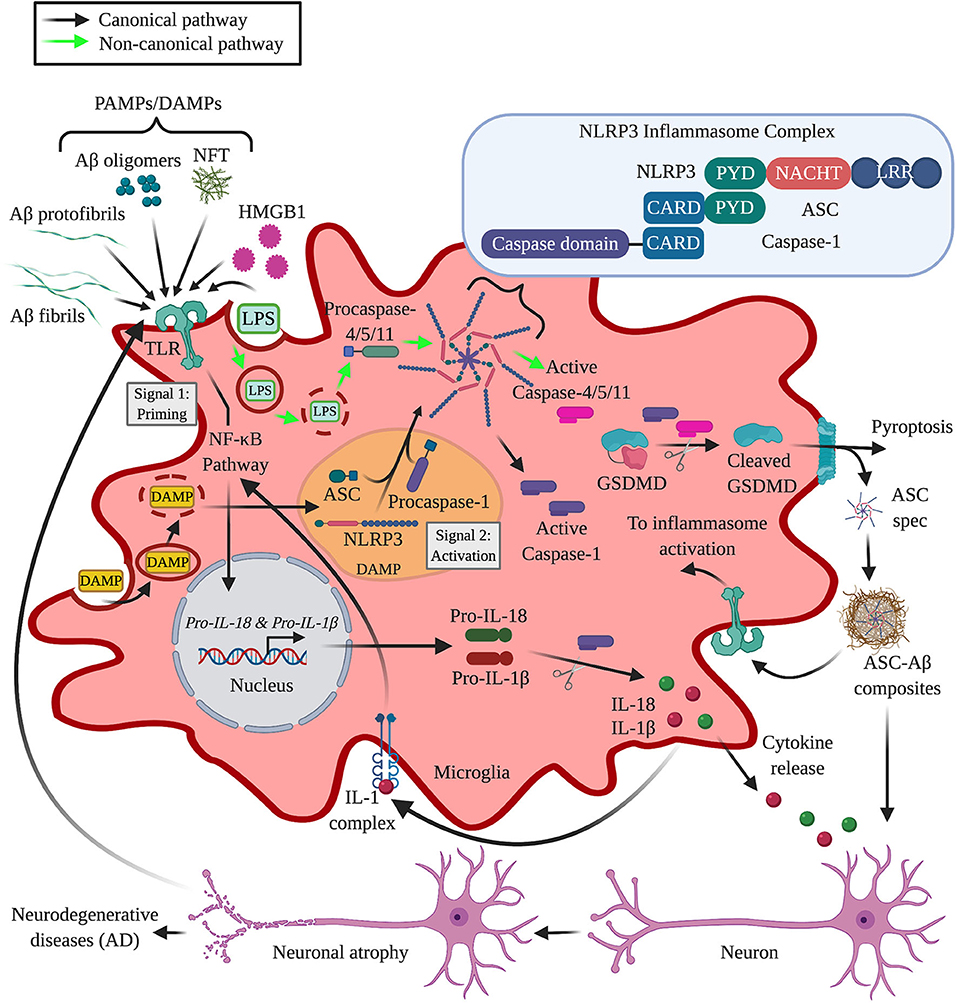
Figure 1. Role of microglia and canonical NLRP3 inflammasome in Alzheimer's disease. Cytokines, pathogen-associated molecular patterns (PAMPs), or danger-associated molecular patterns (DAMPs) bind cell surface receptors [e.g., toll-like receptors (TLRs)] on the microglia, leading to activation of the nuclear factor-κB (NF-κB) pathway (signal 1). The activation of the NF-κB pathway promotes a signaling cascade, resulting in the expression of cytokines such as pro-IL-1β and pro-IL-18 as well as NLR family pyrin domain containing 3 protein (Nlrp3). Through the canonical activation pathway, Nlrp3 oligomerizes in response to an internalized, cytosolic danger signal (DAMP) (signal 2) then recruits Asc and procaspase-1, resulting in inflammasome assembly (Asc-spec assembly) and caspase-1 autoactivation. Activated caspase-1 then cleaves pro-IL-1β or pro-IL-18 and gasdermin (GSDMD). GSDMD cleavage is also induced by caspases 4/5/11 through a non-canonical activation pathway that detects internalized, cytosolic LPS, and other PAMPs/DAMPs that bypass membrane-bound pattern recognition receptors such as TLRs. Subsequently, GSDMD induces pyroptosis, presumably releasing Asc-specs that cross-seed extracellular amyloid beta (Aβ) plaques and creates Asc-Aβ composites that induce a feed-forward cycle amplifying the proinflammatory response. In turn, proinflammatory cytokines including IL-1β and IL-18 induce neuronal damage and death, causing neurodegeneration. Degraded neurons can then trigger a feedback loop by activating microglia. IL-1R complexes are also activated, creating a positive feedback loop that drives additional pro-IL-1β production and primes local microglia for inflammasome activation. AD, Alzheimer's disease; IL, interleukin; NFT, neurofibrillary tangles; ASC, apoptosis-associated spec-like protein containing a CARD; CARD, caspase-activation and recruitment domain; LRR, leucine-rich repeat; NACHT, nucleotide-binding oligomerization domain; PYD, Pyrin domain; HMGB1, high mobility group box 1; LPS, lipopolysaccharide; NF-κB, nuclear factor kappa light chain enhancer of activated B cells created with BioRender.
In this review, we will primarily discuss the role of inflammasome activation by microglia in Alzheimer's disease with a special focus on Nlrp3 inflammasome activation.
Innate Immune Response in AD
Microglia originate from primitive macrophages that exit the yolk sac and colonize the neuroepithelium and are the primary immunocompetent cells found within the brain (13, 14). In a normal physiological state, microglia play a critical role in multiple developmental events within the central nervous system (CNS) such as the establishment of neural circuits, synaptic pruning and remodeling, and neurogenesis (15–21). Microglia are also responsible for clearing cellular debris and aggregate-prone proteins including Aβ as well as harmful bacteria and viruses through phagocytosis in diseased states (22). Microglia within a normal adult mouse brain are highly dense in gray matter areas including the hippocampus, basal ganglia, substantia nigra, and olfactory cortex (23). Throughout these areas, the number and role of microglia is highly regulated by the local microenvironment and their interactions with surrounding cells such as neurons, astrocytes, and oligodendrocytes (24).
Studying key Alzheimer's risk genes has provided critical insights into the function of microglia and how microglia modulate pathology in AD. It is known that several genes, expressed exclusively by microglia in the brain, such as CD33, a sialic-acid-binding immunoglobulin-like lectin (SIGLECs), and triggering receptor expressed on myeloid cells 2 (Trem2) carry single nucleotide polymorphisms (SNPs) that influence the risk for developing AD (7). CD33, a PRR on the cell's surface that recognizes sialylated glycoproteins and gangliosides, promotes Aβ deposition and plaque formation (25). Griciuc et al. (25) also found that CD33 expression impedes microglia's uptake and clearance of amyloid-β 42 (Aβ42), resulting in a larger plaque burden. Inhibition of CD33 using a subtype-selective sialic acid mimetic mitigates Aβ plaque-associated pathology by increasing Aβ plaque phagocytosis (26). Trem2 is another microglial surface receptor, it binds phospholipids and other polyanionic ligands and detects changes in the lipid microenvironment (27). Studies have shown that Trem2-deficient AD mouse models exhibit decreased clustering of microglia around plaques and increased neuritic damage, suggesting that this gene is crucial for containing early plaque diffusion (28, 29). Conversely, Trem2 expression in response to tau has been shown to enhance AD-like pathology (30, 31). So although it appears that Trem2 is important in enhancing microglial responses during AD-associated pathology it remains controversial whether Trem2 expression is overall beneficial or pathogenic in AD (29, 31–34). It is also important to note that many of these findings still require replication in patient samples to confirm the roles of these molecules in AD pathogenesis.
Microglia form a lattice throughout the brain and express an array of PRRs, which sense changes in the brain's environment through the detection of danger-associated molecular patterns (DAMPs) and pathogen-associated molecular patterns (PAMPs) among other stimuli (10). When a shift in the microenvironment is detected, these receptors send converging signals that promote a spectrum of microglial responses from surveillance to activation (35). As microglia survey their environment they rapidly extend and retract their filopodia-like processes within an area of the parenchyma, allowing them to survey that microenvironment (35). When activated, microglia adopt different morphologies, and produce various cytotoxic molecules including pro-inflammatory cytokines and inflammatory mediators consisting of nitric oxide (NO) and reactive oxygen species (ROS) (22, 36, 37). In summary, elucidating the links between innate immune activation and microglia's inflammatory responses concomitant with inflammasome activation, is becoming a crucial research area to better understand AD pathology and to find new therapeutic targets that could impede or slow its progression.
Nlrp3 Inflammasome Activation
Several inflammasomes have been implicated in neurodegenerative diseases, the NOD-, LRR-, and pyrin domain-containing 3 (NLRP3) inflammasome in particular has been shown to play a key role in the development and progression of Aβ-plaque formation as well as tau-induced pathology, which has been demonstrated in both post-mortem AD patient brain tissue and in vivo/in vitro transgenic mouse studies (37–42). The inflammasome is a multimeric protein complex that is most commonly composed of a sensor, an adaptor, and the downstream effector caspase-1 (12). Each inflammasome is named according to the sensor molecule that initiates activation and is activated through two signals that first prime and then activate the complex (43) (Figure 1). Upon activation, Nlrp3, and the majority of other structurally related receptors such as other NLRs, AIM2, or pyrin can form homotypic PYD-PYD or CARD-CARD interactions with the adaptor Asc (apoptosis-associated spec-like protein containing a caspase activating and recruiting domain) (44, 45). The interactions between these molecules are composed of an N-terminal pyrin domain (PYD) and C-terminal caspase-activation and recruitment domain (CARD) and result in the formation of a ring-like perinuclear complex called an Asc “spec,” a typical indicator of canonical inflammasome activation (12, 46). Following inflammasome activation, Asc recruits procaspase-1 through interactions with the CARD domain of caspase-1 (47). Procaspase-1 is then converted into its bioactive form through proximity-induced autocatalysis, producing mature caspase-1 that cleaves pro-IL-1β and pro-IL-18 into their respective secreted forms (10, 48). Caspase-1 also triggers the cleavage of pore-forming Gasdermin D (GSDMD), which induces a lytic, pro-inflammatory form of cell death called pyroptosis (49, 50). Generally, priming and activation of the inflammasome occurs in response to two different signals, however, it is possible that one molecule can deliver both signals. For example, LPS can initiate both the formation of the canonical and non-canonical Nlrp3 inflammasome involving human caspases 4/5 and mouse caspase-11 rather than caspase-1 (51–53). Non-canonical Nlrp3 inflammasome activation serves as another layer of defense for pathogens that have evolved to bypass membrane-bound PRRs such as TLR4 (54) (Figure 1). This form of activation is prompted by caspases' 4/5/11 detection of cytosolic lipopolysaccharide (LPS), which induces pyroptotic cell death through GSDMD cleavage (43). In AD, the Nlrp3 inflammasome is responsible for the maturation of caspase-1, which is in turn responsible for the maturation and secretion of pro-inflammatory cytokines such as IL-1β and IL-18 that can activate signaling pathways resulting in neuroinflammation and neuronal death (5, 12).
Nlrp3 Inflammasome In Normal Aging
The concept of “inflamm-aging,” the low-grade chronic inflammatory state that accompanies aging, is a recent topic of interest (55). During aging, inflammasome activation can be triggered by local microenvironment changes associated with aging microglia (56). For example, aging microglia exhibit altered cytokine production, making microglial cells more susceptible to adopting a pro-inflammatory state that also primes the cells for inflammasome activation (56, 57). Aging microglial cells also have an increased accumulation of lipofuschin that has been associated with increased oxidative stress, which may cause microglia to lose their neuroprotective potential and contribute to age-related pathology (58, 59). Additional evidence suggests that components of the inflammasome including caspase-1, caspase-11, Asc, and IL-1β are increased in the cytosolic fraction of hippocampal lysates in aged mice, suggesting that inflammasome formation contributes to inflammation in aging (60). Consistent with the inflamm-aging hypothesis, Youm et al. found that reducing the Nlrp3 inflammasome-dependent pro-inflammatory cascade alleviated age-associated degenerative changes across multiple organs (61). This study also showed that Nlrp3 gene expression was lower in younger microglia compared to their senile counterparts (61). Thus, the status of inflamm-aging in the brain may be associated with changes in aging microglia prompted by local microenvironment and systemic environment states that induce inflammasome activation.
Nlrp3 Inflammasome and the Microbiome
Emerging evidence has highlighted cross-talk interactions between the gut microbiome and the brain (62, 63). In AD, the composition of gut microbiota can influence the development of or exacerbate the pathology associated with AD (64, 65). The role inflammasomes play within the gut-brain crosstalk is less clear. A newly published study that transplanted gut microbiota from AD patients to either APP/PS1 mice, a double transgenic mouse that carries chimeric mouse/human amyloid precursor protein (APP) and human presenilin 1 (PS1) mutations associated with familial AD, or wild type mice, demonstrated that the transplantation of the gut microbiome of an AD patient can influence AD pathology and Nlrp3 inflammasome activation. This study found that APP/PS1 mice receiving a transplant of the gut microbiome from an AD patient had increased expression of Nlrp3 in their intestinal tract and increased levels of inflammatory factors such as IL-1β and IL-6 in their peripheral blood (62). These mice also exhibited more severe cognitive impairment compared to those that did not receive the transplant. When the gut microbiome from an AD patient was transplanted into wild type mice, the intestinal expression of Nlrp3 was also upregulated but their cognitive abilities were not significantly altered (62). The microglia in the hippocampi of these mice, however, were still activated and there was still an up-regulated expression of inflammatory factors. Taken together, these studies indicate that gut microbiota modulate inflammatory responses through Nlrp3 inflammasome signaling.
Nlrp3 Inflammasome in AD
Both the accumulation and deposition of Aβ as well as NFT formation are detected by cytosolic PRRs, prompting Nlrp3 inflammasome activation in microglia (37, 38). The association between microglial Nlrp3 inflammasome activation and fibrillar Aβ was first demonstrated in vitro by Halle et al. (66). Their study found that exposing Aβ fibrils to primary mouse microglia induces IL-1β secretion in an Nlrp3-specific manner (66). Recently, soluble Aβ oligomers and protofibrils have also been shown to induce Asc spec formation in primary microglia cells collected from wild type mice (67). Nlrp3 activation has also been linked with tau aggregates in PS19 mice, a mouse model that overexpresses the human tau protein carrying the P301S mutation (39). The association between Nlrp3 inflammasome activation and tau exacerbates and drives tau pathology in AD mouse models (38, 39). Related work by Ising and colleagues was the first to suggest that the Nlrp3 inflammasome forms a link between Aβ plaques and NFTs (38). They showed that Tau22 mice receiving an intracerebral injection of fibrillar Aβ-containing APP/PS1 brain homogenates exhibited increased levels of tau hyperphosphorylation, cleaved caspase-1, IL-1β, and Asc in cerebral samples as well as significantly higher levels of extracellular Asc specs, which have been shown to seed Aβ plaques (37, 38). However, when they injected the same homogenate in Tau22/Asc−/− or Tau22/Nlrp3−/− mice, tau hyperphosphorylation did not occur and there were lower levels of cleaved caspase-1, IL-1β, and reduced Asc spec formation and release, further verifying that Nlrp3 activation is essential in the Aβ-tau cascade (38). These findings demonstrate a link between both tau and Aβ pathology and confirm Nlrp3 inflammasome activation in Tau22 mice. Additionally, Heneka et al. used Nlrp3-deficient and caspase-1-deficient APP/PS1 mice to show that mice unable to activate the inflammasome were spared from memory deficits and LTP suppression unlike the APP/PS1 mice that exhibited severe deficits in spatial memory formation (68). Their findings showed that Nlrp3 inflammasome activation restricts beneficial microglial clearance functions while Nlrp3- or caspase-1-deficiency increases microglial plaque phagocytosis (68). Interestingly, new findings support the notion that caspase-1 activation and pro-inflammatory cytokine secretion precede AD pathology, implying that Nlrp3 inflammasome activation is an early pathogenic event in AD (4, 69). Overall, these data indicate that targeting the Nlrp3 inflammasome in human clinical trials are warranted to determine whether inhibition of the inflammasome will hinder Aβ deposition and NFT formation and correlate positively with cognitive outcome measures.
Nlrp3 Inhibition As a Therapeutic Intervention for AD
As Nlrp3 inflammasome activation through both canonical and non-canonical pathways has been shown to play an important role in the pathology of AD, the Nlrp3 inflammasome has emerged as a possible target for future pharmacological therapies (67, 70, 71). Since Nlrp3 inflammasome activation is a multi-step process, inhibiting Nlrp3 inflammasome activation can be accomplished through several different means including: suppressing molecules that promote inflammasome activation or formation, silencing upstream signals, or by directly or indirectly inhibiting the inflammasome complex formation depending on the molecule targeted (10, 72). Some of the direct inhibitors, which specifically target Nlrp3-Nlrp3, Nlrp3-Asc, or NEK7-Nlrp3 interactions, are ginsenoside Rg3, oridonin, and tranilast (73–75). Ginsenoside Rg3, isolated from Panax ginseng, specifically blocks IL-1β secretion and caspase-1 activation by inhibiting LPS priming and Nlrp3 inflammasome assembly (73). In contrast, oridonin, derived from Rabdosia rubescens, blocks inflammasome assembly and activation by hindering the NEK7-Nlrp3 interaction, which is crucial for Nlrp3 oligomerization and Asc recruitment to Nlrp3 (74, 76). Tranilast, a historical anti-allergic drug used in the clinic, directly suppresses Nlrp3 inflammasome assembly by blocking Nlrp3 oligomerization (75). Examples of indirect inhibitors include β-hydroxybutyrate (BHB), MCC950, glyburide, and 16673-43-0, a glyburide analog that has no effect on insulin (77–80). BHB hinders inflammasome formation by inhibiting K+ efflux, which causes mitochondrial damage and exposure to a mitochondrial-specific phospholipid, cardiolipin, that leads to Nlrp3 activation (77, 81). MCC950, on the other hand, selectively blocks both the canonical and non-canonical Nlrp3 inflammasome activation pathways by impeding the ATP hydrolysis motif (80). Glyburide works by suppressing ATP-sensitive K+ channels and caspase-1 activation while its analog, 16673-43-0, achieves inhibition by inducing conformational changes in the inflammasome following Asc's activation or aggregation (79, 82).
Additional methods of inhibiting Nlrp3 inflammasome activation using autophagy-inducing treatments and microRNAs have recently become possible. Some studies have shown that autophagic proteins such as autophagy-related protein 7 (ATG7), microtubule-associated protein 1 light chain 3B (LC3B), and beclin-1 regulates Nlrp3 inflammasome activation by sustaining mitochondrial integrity (83, 84). This data demonstrated that deficiencies in autophagic proteins such as LC3B increases caspase-1 cleavage, Asc spec formation, and IL-1β release in macrophages (83, 84). Thus, by administering autophagy-inducing agents including resveratrol and cannabinoid receptor 2 (CB2R) agonists such as HU-308, Nlrp3 inflammasome activation can be inhibited (85, 86). MicroRNA based post-transcriptional Nlrp3 regulation also prevents inflammasome formation by reducing endogenous Nlrp3 protein levels (87, 88). MicroRNAs are small, conserved single stranded noncoding RNAs that post-transcriptionally regulate gene expression. They bind untranslated regions (UTRs) of transcripts and modify the stability and translation of the target mRNA, producing an inhibitory effect (89). One such example is miR-223, which targets a binding site in the Nlrp3 3′-UTR and was validated in vitro in macrophages (87). This study found that miR-223 overexpression inhibits Nlrp3 protein accumulation and IL-1β production from the inflammasome (87). Although each inhibitor has its own mechanism, they all have a similar effect resulting in decreased inflammasome formation, cytokine release, and systemic inflammation.
Within the context of Alzheimer's disease, some of these inhibitors have already been shown to reduce AD-associated pathology (Table 1). For instance, JC-124 and oridonin, two direct Nlrp3 inflammasome inhibitors, have been shown to reduce amyloid burden and microglial activation in AD mouse models (71, 91). Oridonin treatment also showed beneficial effects in attenuating disease pathology by decreasing inflammatory cytokine release in the hippocampus (91). Another study that elevated plasma ketone body levels through an oral dose of medium-chain triglycerides to individuals with AD or mild cognitive impairment reported a significant increase of BHB in serum levels and some subjects exhibited cognitive improvement (93).
While these findings seem promising, there are some limitations to consider when employing these agents as a potential therapeutic. For example, unintended immunosuppressive effects may result from the use of inhibitors that target IL-1β secretion or signaling (94, 95). Likewise, inhibitors focused on reducing cytokine secretion alone does not address Nlrp3-induced pyroptosis, which may contribute to additional pathology (72). Finally, compounds that inhibit cytokine secretion will not necessarily mitigate pathology driven by Asc spec formation. Continued Asc spec formation will result in further seeding of extracellular Aβ plaques, amplifying amyloid pathology, and the pro-inflammatory response (37). Overall, these studies suggest that hindering inflammasome assembly is a potential intervention method for attenuating AD pathology with the caveat that inhibition of the inflammasome can have severe unintended results.
Concluding Remarks
Traditionally, the cognitive decline associated with AD was attributed to the accumulation of amyloid and tau but emerging evidence suggests that neuroinflammation driven by and triggering additional Nlrp3 inflammasome activation is another critical contributor to AD pathology. Additional investigations are needed to gain insight into the contribution of other inflammasome pathways in neurodegeneration. Additional research is also needed to further clarify the complexity of microglia's signaling cascades and to determine how to modify the microglial response as a potential method for managing or treating AD.
While regulation of Nlrp3 inflammasome assembly and activation may be a potential therapeutic approach, it is currently unknown whether targeting inflammasome activation in AD will result in a beneficial or detrimental effect on clinical outcomes such as cognitive measures. It is also important to consider other therapeutic approaches aimed at modifying microglial function. It is possible that the pro-inflammatory response reproduced in many experimental studies and human genetic or RNA sequencing studies may be the product of a secondary pro-inflammatory response from tissue injury induced by neuronal death, the spreading of AD pathology, or glial dysfunction. Considering that there is microglial dysfunction in AD such as metabolic defects as well as decreased microglial motility and chemotaxis, promoting normal microglial function may prove an alternative therapeutic approach (6, 96, 97). Additional approaches may also include inhibiting microglial proteolytic cleavage of P2RY12, a G-coupled protein receptor located on glial cells, whose inhibition has been associated with neuroprotective effects and promoting other anti-inflammatory cytokines such as IL-4 or progranulin, which still remain to be tested and compared to inflammasome inhibitors (98–100).
Author Contributions
KH conceived the review. KH and TU discussed and contributed to the writing of this review. All authors contributed to the article and approved the submitted version.
Funding
TU was supported by startup funds from the University of Wisconsin Graduate School, the University of Wisconsin School of Medicine and Public Health, and the University of Wisconsin Department of Pathology and Laboratory Medicine. KH was supported by the Neuroscience Program T32 Training Grant (T32NS105602).
Conflict of Interest
The authors declare that the research was conducted in the absence of any commercial or financial relationships that could be construed as a potential conflict of interest.
References
1. Musiek ES, Holtzman DM. Three dimensions of the amyloid hypothesis: time, space and “wingmen.” Nat Neurosci. (2015) 18:800–6. doi: 10.1038/nn.4018
2. Cras P, Kawai M, Lowery D, Gonzalez-DeWhitt P, Greenberg B, Perry G. Senile plaque neurites in Alzheimer disease accumulate amyloid precursor protein. Proc Natl Acad Sci U S A. (1991) 88:7552–6. doi: 10.1073/pnas.88.17.7552
3. He Z, Guo JL, McBride JD, Narasimhan S, Kim H, Changolkar L, et al. Amyloid-β plaques enhance Alzheimer's brain tau-seeded pathologies by facilitating neuritic plaque tau aggregation. Nat Med. (2018) 24:29–38. doi: 10.1038/nm.4443
4. Tarkowski E. Intrathecal inflammation precedes development of Alzheimer's disease. J Neurol Neurosurg Psychiatry. (2003) 74:1200–5. doi: 10.1136/jnnp.74.9.1200
5. Wright AL, Zinn R, Hohensinn B, Konen LM, Beynon SB, Tan RP, et al. Neuroinflammation and neuronal loss precede Aβ plaque deposition in the hAPP-J20 mouse model of Alzheimer's disease. PLoS ONE. (2013) 8:e59586. doi: 10.1371/journal.pone.0059586
6. Baik SH, Kang S, Lee W, Choi H, Chung S, Kim J-I, et al. A breakdown in metabolic reprogramming causes microglia dysfunction in Alzheimer's disease. Cell Metab. (2019) 30:493–7.e6. doi: 10.1016/j.cmet.2019.06.005
7. Bachiller S, Jiménez-Ferrer I, Paulus A, Yang Y, Swanberg M, Deierborg T, et al. Microglia in neurological diseases: a road map to brain-disease dependent-inflammatory response. Front Cell Neurosci. (2018) 12:488. doi: 10.3389/fncel.2018.00488
8. Venegas C, Heneka MT. Danger-associated molecular patterns in Alzheimer's disease. J Leukoc Biol. (2017) 101:87–98. doi: 10.1189/jlb.3MR0416-204R
9. Kawai T, Akira S. Signaling to NF-κB by Toll-like receptors. Trends Mol Med. (2007) 13:460–9. doi: 10.1016/j.molmed.2007.09.002
10. Voet S, Srinivasan S, Lamkanfi M, Loo G. Inflammasomes in neuroinflammatory and neurodegenerative diseases. EMBO Mol Med. (2019) 11:e10248. doi: 10.15252/emmm.201810248
11. Gustin A, Kirchmeyer M, Koncina E, Felten P, Losciuto S, Heurtaux T, et al. NLRP3 inflammasome is expressed and functional in mouse brain microglia but not in astrocytes. PLoS One. (2015) 10:e0130624. doi: 10.1371/journal.pone.0130624
12. Malik A, Kanneganti T-D. Inflammasome activation and assembly at a glance. J Cell Sci. (2017) 130:3955–63. doi: 10.1242/jcs.207365
13. Ginhoux F, Greter M, Leboeuf M, Nandi S, See P, Gokhan S, et al. Fate mapping analysis reveals that adult microglia derive from primitive macrophages. Science. (2010) 330:841–5. doi: 10.1126/science.1194637
14. Alliot F, Godin I, Pessac B. Microglia derive from progenitors, originating from the yolk sac, and which proliferate in the brain. Dev Brain Res. (1999) 117:145–52. doi: 10.1016/S0165-3806(99)00113-3
15. Ji K, Akgul G, Wollmuth LP, Tsirka SE. Microglia actively regulate the number of functional synapses. PLoS One. (2013) 8:e56293. doi: 10.1371/journal.pone.0056293
16. Diaz-Aparicio I, Paris I, Sierra-Torre V, Plaza-Zabala A, Rodríguez-Iglesias N, Márquez-Ropero M, et al. Microglia actively remodel adult hippocampal neurogenesis through the phagocytosis secretome. J Neurosci. (2020) 40:1453–82. doi: 10.1523/JNEUROSCI.0993-19.2019
17. Ortega-Martinez S, Palla N, Zhang X, Lipman E, Sisodia SS. Deficits in enrichment-dependent neurogenesis and enhanced anxiety behaviors mediated by expression of Alzheimer's disease-linked Ps1 variants are rescued by microglial depletion. J Neurosci. (2019) 39:6766–80. doi: 10.1523/JNEUROSCI.0884-19.2019
18. Weinhard L, di Bartolomei G, Bolasco G, Machado P, Schieber NL, Neniskyte U, et al. Microglia remodel synapses by presynaptic trogocytosis and spine head filopodia induction. Nat Commun. (2018) 9:1228. doi: 10.1038/s41467-018-03566-5
19. Squarzoni P, Oller G, Hoeffel G, Pont-Lezica L, Rostaing P, Low D, et al. Microglia modulate wiring of the embryonic forebrain. Cell Rep. (2014) 8:1271–79. doi: 10.1016/j.celrep.2014.07.042
20. Hong S, Beja-Glasser VF, Nfonoyim BM, Frouin A, Li S, Ramakrishnan S, et al. Complement and microglia mediate early synapse loss in Alzheimer mouse models. Science. (2016) 352:712–6. doi: 10.1126/science.aad8373
21. Schafer DP, Lehrman EK, Kautzman AG, Koyama R, Mardinly AR, Yamasaki R, et al. Microglia sculpt postnatal neural circuits in an activity and complement-dependent manner. Neuron. (2012) 74:691–705. doi: 10.1016/j.neuron.2012.03.026
22. Heneka MT, Golenbock DT, Latz E. Innate immunity in Alzheimer's disease. Nat Immunol. (2015) 16:229–36. doi: 10.1038/ni.3102
23. Lawson LJ, Perry VH, Dri P, Gordon S. Heterogeneity in the distribution and morphology of microglia in the normal adult mouse brain. Neuroscience. (1990) 39:151–70. doi: 10.1016/0306-4522(90)90229-W
24. Lu Y, Guo Z, Zhang Y, Li C, Zhang Y, Guo Q, et al. Microenvironment remodeling micelles for Alzheimer's disease therapy by early modulation of activated microglia. Adv Sci. (2018) 6:1801586. doi: 10.1002/advs.201801586
25. Griciuc A, Serrano-Pozo A, Parrado AR, Lesinski AN, Asselin CN, Mullin K, et al. Alzheimer's disease risk gene CD33 inhibits microglial uptake of amyloid beta. Neuron. (2013) 78:631–43. doi: 10.1016/j.neuron.2013.04.014
26. Miles LA, Hermans SJ, Crespi GAN, Gooi JH, Doughty L, Nero TL, et al. Small molecule binding to Alzheimer risk factor CD33 promotes Aβ phagocytosis. iScience. (2019) 19:110–8. doi: 10.1016/j.isci.2019.07.023
27. Wang Y, Cella M, Mallinson K, Ulrich JD, Young KL, Robinette ML, et al. TREM2 lipid sensing sustains the microglial response in an Alzheimer's disease model. Cell. (2015) 160:1061–71. doi: 10.1016/j.cell.2015.01.049
28. Wang Y, Ulland TK, Ulrich JD, Song W, Tzaferis JA, Hole JT, et al. TREM2-mediated early microglial response limits diffusion and toxicity of amyloid plaques. J Exp Med. (2016) 213:667–75. doi: 10.1084/jem.20151948
29. Jay TR, Hirsch AM, Broihier ML, Miller CM, Neilson LE, Ransohoff RM, et al. Disease progression-dependent effects of TREM2 deficiency in a mouse model of Alzheimer's disease. J Neurosci. (2017) 37:637–47. doi: 10.1523/JNEUROSCI.2110-16.2017
30. Leyns CEG, Ulrich JD, Finn MB, Stewart FR, Koscal LJ, Remolina Serrano J, et al. TREM2 deficiency attenuates neuroinflammation and protects against neurodegeneration in a mouse model of tauopathy. Proc Natl Acad Sci U S A. (2017) 114:11524–9. doi: 10.1073/pnas.1710311114
31. Leyns CEG, Gratuze M, Narasimhan S, Jain N, Koscal LJ, Jiang H, et al. TREM2 function impedes tau seeding in neuritic plaques. Nat Neurosci. (2019) 22:1217–22. doi: 10.1038/s41593-019-0433-0
32. Ulland TK, Song WM, Huang SC-C, Ulrich JD, Sergushichev A, Beatty WL, et al. TREM2 maintains microglial metabolic fitness in Alzheimer's disease. Cell. (2017) 170:649–63.e13. doi: 10.1016/j.cell.2017.07.023
33. Yuan P, Condello C, Keene CD, Wang Y, Bird TD, Paul SM, et al. TREM2 haplodeficiency in mice and humans impairs the microglia barrier function leading to decreased amyloid compaction and severe axonal dystrophy. Neuron. (2016) 92:252–64. doi: 10.1016/j.neuron.2016.09.016
34. Jay TR, Miller CM, Cheng PJ, Graham LC, Bemiller S, Broihier ML, et al. TREM2 deficiency eliminates TREM2+ inflammatory macrophages and ameliorates pathology in Alzheimer's disease mouse models. J Exp Med. (2015) 212:287–95. doi: 10.1084/jem.20142322
35. Benarroch EE. Multiple roles in surveillance, circuit shaping, and response to injury. Neurology. (2013) 81:1079–88. doi: 10.1212/WNL.0b013e3182a4a577
36. Wang Q. Amyloid-mediated inhibition of NMDA receptor-dependent long-term potentiation induction involves activation of microglia and stimulation of inducible nitric oxide synthase and superoxide. J Neurosci. (2004) 24:6049–56. doi: 10.1523/JNEUROSCI.0233-04.2004
37. Venegas C, Kumar S, Franklin BS, Dierkes T, Brinkschulte R, Tejera D, et al. Microglia-derived ASC specks cross-seed amyloid-β in Alzheimer's disease. Nature. (2017) 552:355–61. doi: 10.1038/nature25158
38. Ising C, Venegas C, Zhang S, Scheiblich H, Schmidt SV, Vieira-Saecker A, et al. NLRP3 inflammasome activation drives tau pathology. Nature. (2019) 575:669–73. doi: 10.1038/s41586-019-1769-z
39. Stancu I-C, Cremers N, Vanrusselt H, Couturier J, Vanoosthuyse A, Kessels S, et al. Aggregated Tau activates NLRP3-ASC inflammasome exacerbating exogenously seeded and non-exogenously seeded Tau pathology in vivo. Acta Neuropathol. (2019) 137:599–617. doi: 10.1007/s00401-018-01957-y
40. Tan M-S, Tan L, Jiang T, Zhu X-C, Wang H-F, Jia C-D, et al. Amyloid-β induces NLRP1-dependent neuronal pyroptosis in models of Alzheimer's disease. Cell Death Dis. (2014) 5:e1382. doi: 10.1038/cddis.2014.348
41. Denes A, Coutts G, Lénárt N, Cruickshank SM, Pelegrin P, Skinner J, et al. AIM2 and NLRC4 inflammasomes contribute with ASC to acute brain injury independently of NLRP3. Proc Natl Acad Sci U S A. (2015) 112:4050–5. doi: 10.1073/pnas.1419090112
42. Liu L, Chan C. IPAF inflammasome is involved in interleukin-1β production from astrocytes, induced by palmitate; implications for Alzheimer's disease. Neurobiol Aging. (2014) 35:309–21. doi: 10.1016/j.neurobiolaging.2013.08.016
43. Kelley N, Jeltema D, Duan Y, He Y. The NLRP3 inflammasome: an overview of mechanisms of activation and regulation. IJMS. (2019) 20:3328. doi: 10.3390/ijms20133328
44. Cai X, Chen J, Xu H, Liu S, Jiang Q-X, Halfmann R, et al. Prion-like polymerization underlies signal transduction in antiviral immune defense and inflammasome activation. Cell. (2014) 156:1207–22. doi: 10.1016/j.cell.2014.01.063
45. Sborgi L, Ravotti F, Dandey VP, Dick MS, Mazur A, Reckel S, et al. Structure and assembly of the mouse ASC inflammasome by combined NMR spectroscopy and cryo-electron microscopy. Proc Natl Acad Sci U S A. (2015) 112:13237–42. doi: 10.1073/pnas.1507579112
46. Nambayan RJT, Sandin SI, Quint DA, Satyadi DM, de Alba E. The inflammasome adapter ASC assembles into filaments with integral participation of its two Death Domains, PYD and CARD. J Biol Chem. (2019) 294:439–52. doi: 10.1074/jbc.RA118.004407
47. Lu A, Magupalli VG, Ruan J, Yin Q, Atianand MK, Vos MR, et al. Unified polymerization mechanism for the assembly of ASC-dependent inflammasomes. Cell. (2014) 156:1193–206. doi: 10.1016/j.cell.2014.02.008
48. Ghayur T, Banerjee S, Hugunin M, Butler D, Herzog L, Carter A, et al. Caspase-1 processes IFN-gamma-Inducing factor and regulates LPS-induced IFN–y production. Nature. (1997) 386:619–23. doi: 10.1038/386619a0
49. Heneka MT, McManus RM, Latz E. Inflammasome signalling in brain function and neurodegenerative disease. Nat Rev Neurosci. (2018) 19:610–21. doi: 10.1038/s41583-018-0055-7
50. He W, Wan H, Hu L, Chen P, Wang X, Huang Z, et al. Gasdermin D is an executor of pyroptosis and required for interleukin-1β secretion. Cell Res. (2015) 25:1285–98. doi: 10.1038/cr.2015.139
51. Ross C, Chan AH, Von Pein J, Boucher D, Schroder K. Dimerization and auto-processing induce caspase-11 protease activation within the non-canonical inflammasome. Life Sci Alliance. (2018) 1:e201800237. doi: 10.26508/lsa.201800237
52. Lee BL, Stowe IB, Gupta A, Kornfeld OS, Roose-Girma M, Anderson K, et al. Caspase-11 auto-proteolysis is crucial for noncanonical inflammasome activation. J Exp Med. (2018) 215:2279–88. doi: 10.1084/jem.20180589
53. Bauernfeind FG, Horvath G, Stutz A, Alnemri ES, MacDonald K, Speert D, et al. Cutting edge: NF-κB activating pattern recognition and cytokine receptors license NLRP3 inflammasome activation by regulating NLRP3 expression. J Immunol. (2009) 183:787–91. doi: 10.4049/jimmunol.0901363
54. Kayagaki N, Wong MT, Stowe IB, Ramani SR, Gonzalez LC, Akashi-Takamura S, et al. Noncanonical inflammasome activation by intracellular LPS independent of TLR4. Science. (2013) 341:1246–9. doi: 10.1126/science.1240248
55. Franceschi C, Bonafè M, Valensin S, Olivieri F, De Luca M, Ottaviani E, et al. Inflamm-aging: an evolutionary perspective on immunosenescence. Ann N Y Acad Sci. (2006) 908:244–54. doi: 10.1111/j.1749-6632.2000.tb06651.x
56. Hu M, Lin Y, Zhang B, Lu D, Lu Z, Cai W. Update of inflammasome activation in microglia/macrophage in aging and aging-related disease. CNS Neurosci Ther. (2019) 25:1299–307. doi: 10.1111/cns.13262
57. O'Neil SM, Witcher KG, McKim DB, Godbout JP. Forced turnover of aged microglia induces an intermediate phenotype but does not rebalance CNS environmental cues driving priming to immune challenge. Acta Neuropathol Commun. (2018) 6:129. doi: 10.1186/s40478-018-0636-8
58. Singh Kushwaha S, Patro N, Kumar Patro I. A sequential study of age-related lipofuscin accumulation in hippocampus and striate cortex of rats. Ann Neurosci. (2018) 25:223–33. doi: 10.1159/000490908
59. Brown DR. Role of microglia in age-related changes to the nervous system. Sci World J. (2009) 9:1061–71. doi: 10.1100/tsw.2009.111
60. Mejias NH, Martinez CC, Stephens ME, de Rivero Vaccari JP. Contribution of the inflammasome to inflammaging. J Inflamm. (2018) 15:23. doi: 10.1186/s12950-018-0198-3
61. Youm Y-H, Grant RW, McCabe LR, Albarado DC, Nguyen KY, Ravussin A, et al. Canonical Nlrp3 inflammasome links systemic low-grade inflammation to functional decline in aging. Cell Metab. (2013) 18:519–32. doi: 10.1016/j.cmet.2013.09.010
62. Shen H, Guan Q, Zhang X, Yuan C, Tan Z, Zhai L, et al. New mechanism of neuroinflammation in Alzheimer's disease: the activation of NLRP3 inflammasome mediated by gut microbiota. Prog Neuro-Psychopharmacol Biol Psychiatry. (2020) 100:109884. doi: 10.1016/j.pnpbp.2020.109884
63. Ma Q, Xing C, Long W, Wang HY, Liu Q, Wang R-F. Impact of microbiota on central nervous system and neurological diseases: the gut-brain axis. J Neuroinflammation. (2019) 16:53. doi: 10.1186/s12974-019-1434-3
64. Vogt NM, Kerby RL, Dill-McFarland KA, Harding SJ, Merluzzi AP, Johnson SC, et al. Gut microbiome alterations in Alzheimer's disease. Sci Rep. (2017) 7:13537. doi: 10.1038/s41598-017-13601-y
65. Harach T, Marungruang N, Duthilleul N, Cheatham V, Mc Coy KD, Frisoni G, et al. Reduction of Abeta amyloid pathology in APPPS1 transgenic mice in the absence of gut microbiota. Sci Rep. (2017) 7:41802. doi: 10.1038/srep46856
66. Halle A, Hornung V, Petzold GC, Stewart CR, Monks BG, Reinheckel T, et al. The NALP3 inflammasome is involved in the innate immune response to amyloid-β. Nat Immunol. (2008) 9:857–65. doi: 10.1038/ni.1636
67. Lučiunaite A, McManus RM, Jankunec M, Rácz I, Dansokho C, Dalgediene I, et al. Soluble Aβ oligomers and protofibrils induce NLRP3 inflammasome activation in microglia. J Neurochem. (2020) e14945. doi: 10.1111/jnc.14945
68. Heneka MT, Kummer MP, Stutz A, Delekate A, Schwartz S, Vieira-Saecker A, et al. NLRP3 is activated in Alzheimer's disease and contributes to pathology in APP/PS1 mice. Nature. (2013) 493:674–8. doi: 10.1038/nature11729
69. de Calignon A, Fox LM, Pitstick R, Carlson GA, Bacskai BJ, Spires-Jones TL, et al. Caspase activation precedes and leads to tangles. Nature. (2010) 464:1201–4. doi: 10.1038/nature08890
70. Nakanishi A, Kaneko N, Takeda H, Sawasaki T, Morikawa S, Zhou W, et al. Amyloid β directly interacts with NLRP3 to initiate inflammasome activation: identification of an intrinsic NLRP3 ligand in a cell-free system. Inflamm Regener. (2018) 38:27. doi: 10.1186/s41232-018-0085-6
71. Yin J, Zhao F, Chojnacki JE, Fulp J, Klein WL, Zhang S, et al. NLRP3 Inflammasome inhibitor ameliorates amyloid pathology in a mouse model of Alzheimer's disease. Mol Neurobiol. (2018) 55:1977–87. doi: 10.1007/s12035-017-0467-9
72. Zahid A, Li B, Kombe AJK, Jin T, Tao J. Pharmacological Inhibitors of the NLRP3 Inflammasome. Front Immunol. (2019) 10:2538. doi: 10.3389/fimmu.2019.02538
73. Shi Y, Wang H, Zheng M, Xu W, Yang Y, Shi F. Ginsenoside Rg3 suppresses the NLRP3 inflammasome activation through inhibition of its assembly. FASEB J. (2020) 34:208–21. doi: 10.1096/fj.201901537R
74. He H, Jiang H, Chen Y, Ye J, Wang A, Wang C, et al. Oridonin is a covalent NLRP3 inhibitor with strong anti-inflammasome activity. Nat Commun. (2018) 9:2550. doi: 10.1038/s41467-018-04947-6
75. Huang Y, Jiang H, Chen Y, Wang X, Yang Y, Tao J, et al. Tranilast directly targets NLRP 3 to treat inflammasome-driven diseases. EMBO Mol Med. (2018) 10:e8689. doi: 10.15252/emmm.201708689
76. He Y, Zeng MY, Yang D, Motro B, Núñez G. NEK7 is an essential mediator of NLRP3 activation downstream of potassium efflux. Nature. (2016) 530:354–57. doi: 10.1038/nature16959
77. Youm Y-H, Nguyen KY, Grant RW, Goldberg EL, Bodogai M, Kim D, et al. The ketone metabolite β-hydroxybutyrate blocks NLRP3 inflammasome-mediated inflammatory disease. Nat Med. (2015) 21:263–9. doi: 10.1038/nm.3804
78. Liu L, Dong Y, Ye M, Jin S, Yang J, Joosse ME, et al. The pathogenic role of NLRP3 inflammasome activation in inflammatory bowel diseases of both mice and humans. ECCOJC. (2016) 11:737–50. doi: 10.1093/ecco-jcc/jjw219
79. Marchetti C, Chojnacki J, Toldo S, Mezzaroma E, Tranchida N, Rose SW, et al. A Novel pharmacologic inhibitor of the NLRP3 inflammasome limits myocardial injury after ischemia-reperfusion in the mouse. J Cardiovasc Pharmacol. (2014) 63:316–22. doi: 10.1097/FJC.0000000000000053
80. Coll RC, Hill JR, Day CJ, Zamoshnikova A, Boucher D, Massey NL, et al. MCC950 directly targets the NLRP3 ATP-hydrolysis motif for inflammasome inhibition. Nat Chem Biol. (2019) 15:556–9. doi: 10.1038/s41589-019-0277-7
81. Iyer SS, He Q, Janczy JR, Elliott EI, Zhong Z, Olivier AK, et al. Mitochondrial cardiolipin is required for Nlrp3 inflammasome activation. Immunity. (2013) 39:311–23. doi: 10.1016/j.immuni.2013.08.001
82. Lamkanfi M, Mueller JL, Vitari AC, Misaghi S, Fedorova A, Deshayes K, et al. Glyburide inhibits the cryopyrin/Nalp3 inflammasome. J Cell Biol. (2009) 187:61–70. doi: 10.1083/jcb.200903124
83. Cho M-H, Cho K, Kang H-J, Jeon E-Y, Kim H-S, Kwon H-J, et al. Autophagy in microglia degrades extracellular β-amyloid fibrils and regulates the NLRP3 inflammasome. Autophagy. (2014) 10:1761–75. doi: 10.4161/auto.29647
84. Nakahira K, Haspel JA, Rathinam VAK, Lee S-J, Dolinay T, Lam HC, et al. Autophagy proteins regulate innate immune responses by inhibiting the release of mitochondrial DNA mediated by the NALP3 inflammasome. Nat Immunol. (2011) 12:222–30. doi: 10.1038/ni.1980
85. Shao B-Z, Wei W, Ke P, Xu Z-Q, Zhou J-X, Liu C. Activating cannabinoid receptor 2 alleviates pathogenesis of experimental autoimmune encephalomyelitis via activation of autophagy and inhibiting NLRP3 inflammasome. CNS Neurosci Ther. (2014) 20:1021–8. doi: 10.1111/cns.12349
86. Chang Y-P, Ka S-M, Hsu W-H, Chen A, Chao LK, Lin C-C, et al. Resveratrol inhibits NLRP3 inflammasome activation by preserving mitochondrial integrity and augmenting autophagy. J Cell Physiol. (2015) 230:1567–79. doi: 10.1002/jcp.24903
87. Haneklaus M, Gerlic M, Kurowska-Stolarska M, Rainey A-A, Pich D, McInnes IB, et al. Cutting edge: miR-223 and EBV miR-BART15 regulate the NLRP3 inflammasome and IL-1β production. J Immunol. (2012) 189:3795–9. doi: 10.4049/jimmunol.1200312
88. Wang Y, Han Z, Fan Y, Zhang J, Chen K, Gao L, et al. MicroRNA-9 inhibits NLRP3 inflammasome activation in human atherosclerosis inflammation cell models through the JAK1/STAT signaling pathway. Cell Physiol Biochem. (2017) 41:1555–71. doi: 10.1159/000470822
89. Tezcan G, Martynova EV, Gilazieva ZE, McIntyre A, Rizvanov AA, Khaiboullina SF. MicroRNA post-transcriptional regulation of the NLRP3 inflammasome in immunopathologies. Front Pharmacol. (2019) 10:451. doi: 10.3389/fphar.2019.00451
90. Yoon S-J, Park J-Y, Choi S, Lee J-B, Jung H, Kim T-D, et al. Ginsenoside Rg3 regulates S-nitrosylation of the NLRP3 inflammasome via suppression of iNOS. Biochem Biophys Res Commun. (2015) 463:1184–9. doi: 10.1016/j.bbrc.2015.06.080
91. Wang S, Yang H, Yu L, Jin J, Qian L, Zhao H, et al. Oridonin attenuates Aβ1-42-induced neuroinflammation and inhibits NF-κB pathway. PLoS One. (2014) 9:e104745. doi: 10.1371/journal.pone.0104745
92. Kashiwaya Y, Bergman C, Lee J-H, Wan R, King MT, Mughal MR, et al. A ketone ester diet exhibits anxiolytic and cognition-sparing properties, and lessens amyloid and tau pathologies in a mouse model of Alzheimer's disease. Neurobiol Aging. (2013) 34:1530–9. doi: 10.1016/j.neurobiolaging.2012.11.023
93. Reger MA, Henderson ST, Hale C, Cholerton B, Baker LD, Watson GS, et al. Effects of β-hydroxybutyrate on cognition in memory-impaired adults. Neurobiol Aging. (2004) 25:311–4. doi: 10.1016/S0197-4580(03)00087-3
94. Salliot C, Dougados M, Gossec L. Risk of serious infections during rituximab, abatacept and anakinra treatments for rheumatoid arthritis: meta-analyses of randomised placebo-controlled trials. Ann Rheum Dis. (2009) 68:25–32. doi: 10.1136/ard.2007.083188
95. Kullenberg T, Löfqvist M, Leinonen M, Goldbach-Mansky R, Olivecrona H. Long-term safety profile of anakinra in patients with severe cryopyrin-associated periodic syndromes. Rheumatology. (2016) 55:1499–506. doi: 10.1093/rheumatology/kew208
96. Streit WJ, Braak H, Xue Q-S, Bechmann I. Dystrophic (senescent) rather than activated microglial cells are associated with tau pathology and likely precede neurodegeneration in Alzheimer's disease. Acta Neuropathol. (2009) 118:475–85. doi: 10.1007/s00401-009-0556-6
97. Mildner A, Huang H, Radke J, Stenzel W, Priller J. P2Y 12 receptor is expressed on human microglia under physiological conditions throughout development and is sensitive to neuroinflammatory diseases: P2Y 12 Expression on Human Microglia. Glia. (2017) 65:375–87. doi: 10.1002/glia.23097
98. Gadani SP, Cronk JC, Norris GT, Kipnis J. IL-4 in the brain: a cytokine to remember. J Immunol. (2012) 189:4213–9. doi: 10.4049/jimmunol.1202246
99. Walker DG, Tang TM, Mendsaikhan A, Tooyama I, Serrano GE, Sue LI, et al. Patterns of expression of purinergic receptor P2RY12, a putative marker for non-activated microglia, in aged and Alzheimer's disease brains. IJMS. (2020) 21:678. doi: 10.3390/ijms21020678
Keywords: Alzheimer's disease, microglia, Nlrp3 inflammasome, neuroinflammation, neurodegeneration
Citation: Hanslik KL and Ulland TK (2020) The Role of Microglia and the Nlrp3 Inflammasome in Alzheimer's Disease. Front. Neurol. 11:570711. doi: 10.3389/fneur.2020.570711
Received: 08 June 2020; Accepted: 13 August 2020;
Published: 18 September 2020.
Edited by:
Tuan Leng Tay, University of Freiburg, GermanyReviewed by:
Shanu Faerch Roemer, Mayo Clinic Florida, United StatesJui-Heng Tseng, University of North Carolina at Chapel Hill, United States
Constanza Cortes, University of Alabama at Birmingham, United States
Copyright © 2020 Hanslik and Ulland. This is an open-access article distributed under the terms of the Creative Commons Attribution License (CC BY). The use, distribution or reproduction in other forums is permitted, provided the original author(s) and the copyright owner(s) are credited and that the original publication in this journal is cited, in accordance with accepted academic practice. No use, distribution or reproduction is permitted which does not comply with these terms.
*Correspondence: Tyler K. Ulland, dHVsbGFuZCYjeDAwMDQwO3dpc2MuZWR1