- 1Taipei Municipal Gan-Dau Hospital, Taipei, Taiwan
- 2Department of Neurology, Taipei Veterans General Hospital, Taipei, Taiwan
- 3Institute of Neuroscience, School of Life Sciences, National Yang-Ming University, Taipei, Taiwan
- 4Department of Biomedical Imaging and Radiological Sciences, National Yang-Ming University, Taipei, Taiwan
- 5Brain Research Center, National Yang-Ming University, Taipei, Taiwan
- 6Education Center for Humanities and Social Sciences, School of Humanities and Social Sciences, National Yang-Ming University, Taipei, Taiwan
The cerebellum has long been known to play an important role in motor and balance control, and accumulating evidence has revealed that it is also involved in multiple cognitive functions. However, the evidence from neuroimaging studies and clinical observations is not well-integrated at the anatomical or molecular level. The goal of this review is to summarize and link different aspects of the cerebellum, including molecular patterning, functional topography images, and clinical cerebellar disorders. More specifically, we explored the potential relationships between the cerebrocerebellar connections and the expression of particular molecules and, in particular, zebrin stripe (a Purkinje cell-specific antibody molecular marker, which is a glycolytic enzyme expressed in cerebellar Purkinje cells). We hypothesized that the zebrin patterns contribute to cerebellar functional maps—especially when cerebrocerebellar circuit changes exist in cerebellar-related diseases. The zebrin stripe receives input from climbing fibers and project to different parts of the cerebral cortex through its cerebrocerebellar connection. Since zebrin-positive cerebellar Purkinje cells are resistant to excitotoxicity and cell injury while zebrin-negative zones are more prone to damage, we suggest that motor control dysfunction symptoms such as ataxia and dysmetria present earlier and are easier to observe than non-ataxia symptoms due to zebrin-negative cell damage by cerebrocerebellar connections. In summary, we emphasize that the molecular zebrin patterns provide the basis for a new viewpoint from which to investigate cerebellar functions and clinico-neuroanatomic correlations.
Introduction
The cerebellum was historically regarded as a pure motor control system. However, in the past several decades studies from functional imaging (1–3) and clinical (4) studies have revealed that it is also involved in cognitive function. It remains unclear how the cerebellum relates to cognitive functions. Our interest is to integrate different cerebellar studies based on molecular strip patterns, especially the zebrin patterns (5), and we explored what was known about its connection to neuroanatomy, functional neuroimaging studies, and clinical cerebellar disorders. We would like to raise awareness of recent cerebellar studies and attempts to establish clinico-neuroanatomical correlations. Here, we reconsider the importance of molecular characteristics in cerebellar functions.
Gross Anatomy of Cerebellum
The cerebellum is located in the small posterior cranial fossa, a small space in which pons and medulla oblongata are also located. The cerebellum accounts for 10% of the total mass of the brain. However, this tiny structure contains a much greater number of neurons than the much larger cerebrum (6). The gross anatomy of the cerebellum is divided into two hemispheres and one vermis. Based on surface anatomic fissures, the cerebellum consists of the anterior lobe, the posterior lobe, and the flocculonodular lobe. The anterior lobe and posterior lobe are separated by the primary fissure. Several anatomical fissures divide the cerebellar lobes into 10 smaller cerebellar lobules, from lobule I to X [Figure 1; (7)].
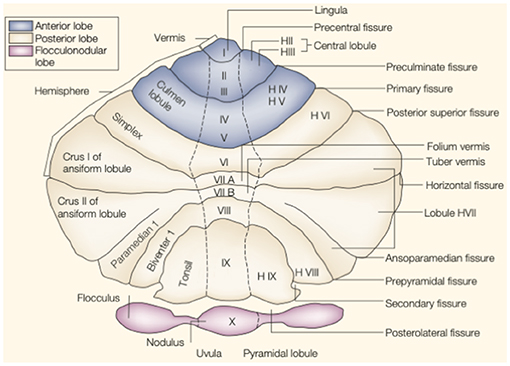
Figure 1. The anatomy of the cerebellum. This is an unfolded view of the cerebellar cortex showing fissures and lobules from I to X. Adapted from Manni and Petrosini (7).
Functional Subdivisions
The cerebellum can also be divided into 3 different parts based on its inputs from other brain regions: the vestibulocerebellum, the spinocerebellum, and the cerebrocerebellum. The vestibulocerebellum is phylogenetically the oldest part in the evolution of primates. The vestibulocerebellum (or flocculonodular lobe anatomically) mainly receives input from the vestibular nuclei, which are located in the medulla and pons, and projects back to the vestibular nuclei, controlling balance and ocular movements. The spinocerebellum, including the vermis, and paravermis of the hemispheres, receives sensory input mainly from the spinal cord (the spinocerebellar tract) and visual and auditory systems and projects back to deep cerebellar nuclei; based on interactions with the cerebral cortex (via the midbrain and thalamus) and the brain stem, it controls trunk and body movements. The cerebrocerebellum is the phylogenetically youngest part of the cerebellum and includes most parts of the cerebellar hemispheres. It receives input exclusively from the cerebral cortex based on cortico-ponto-cerebellar circuits and the olivocerebellar tract (via the climbing fibers). These climbing fibers project to the deep dentate nucleus and then the cerebral cortex (via the red nucleus and thalamus). The cerebrocerebellum modulates smooth and precise limb movements (7, 8).
Cellular Anatomy
At the microscopic level, the cytoarchitecture of the cerebellum is mainly divided into three layers: the molecular, Purkinje, and granular layers. The molecular layer contains GABAergic inhibitory interneurons that form synapses onto Purkinje cell dendrites. The Purkinje layer consists of Purkinje cells, the primary integrative neurons (inhibitory GABAergic neurons) and the only source of efferent fibers from the cerebellar cortex to the deep cerebellar nuclei (9). The granule cell layer, which includes granule cells, brush cells, and Golgi cells, contains most of the neurons in the cerebellum. The granule cells are the only excitatory (glutamatergic) cells in the cerebellar cortex and send parallel fibers into the superficial molecular layer.
The afferent inputs to the cerebellar cortex consist of mossy and climbing fibers. Mossy fibers carry sensorimotor information from the ipsilateral vestibular nuclei, ipsilateral spinal cord, and contralateral pontine nuclei from cortico-pontocerebellar tracts and enter the granular layers. The climbing fibers carry sensorimotor inputs from the contralateral inferior olivary nucleus and project to and modulate the cell-firing activities of Purkinje cells. The Purkinje cell fiber projects to the deep nuclei, which is the only output from the cerebellum and controls the ultimate effect of cerebellar function. Each Purkinje cell receives input from one to seven climbing fibers, in contrast to the multiple inputs from parallel fibers and mossy fibers. The climbing fiber is a specific projection to a few Purkinje cells compared to mossy fiber, which has non-specific connections with multiple Purkinje cells (10, 11).
Zebrin Patterns
Interestingly, a striped pattern is formed by the Purkinje cell-specific antibody molecular marker zebrin (also called aldolase C) (12), which is a glycolytic enzyme expressed in cerebellar Purkinje cells (13, 14). This aldolase C enzyme expresses specifically in the hippocampus and Purkinje cells and plays an important role in ATP biosynthesis (15). Hawkes et al. demonstrated parasagittal stripe, a stereotyped array of transverse zones with zebrin immunostaining reactive and non-reactive patterns. The zebrin stripe constitute seven longitudinal bands identified by Professor Voogd, who used an acetylcholinesterase stain in the cerebellar cortex (16, 17). The zebrin-negative zones (C1, C3, and Y zones) are motor regions of the cerebellum that receive somatosensory input, and the zebrin-negative Purkinje cells fire at higher frequencies. On the other hand, the zebrin-positive zones (C2, D1, and D2 zones) are non-motor regions of the cerebellum, and the zebrin-positive Purkinje cells fire at lower frequencies (18). Each zone innervates different and specific olivo-cortico-nuclear pathways [Figure 2; (19)]. Larson et al. showed that electrical stimulation of nerves from different limbs have distinct climbing fiber responses in different longitudinal zones (20). In other words, the climbing fibers and those corticonuclear projections define these zebrin patterns, which may be the basic functional units of the cerebellum (21, 22). In addition, Richard et al.'s “one-map” hypothesis describes that climbing fibers have a closed-loop projection to specific zebrin patterns and unify the cerebellar map from the perspective of anatomy, embryology, and physiology (23). In sum, the zebrin-positive/-negative longitudinal patterns have different and specific connections and are related to distinct functions.
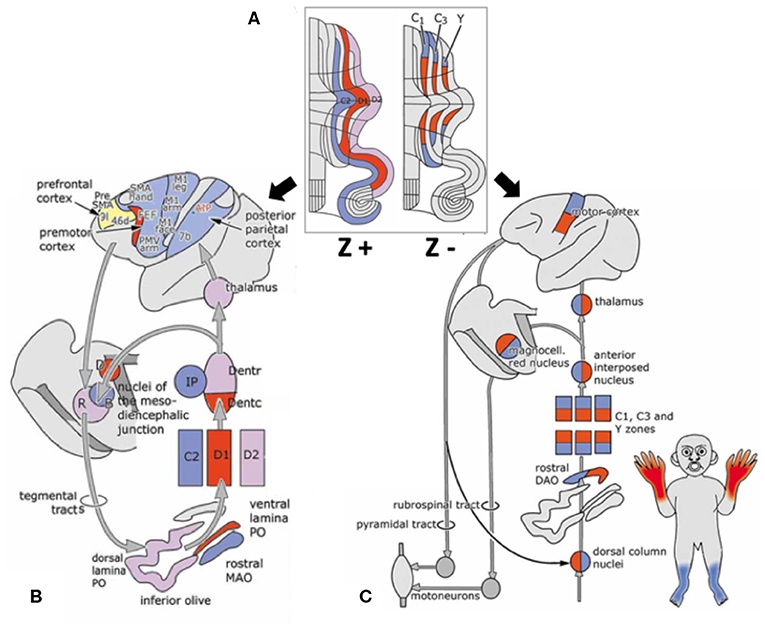
Figure 2. Longitudinal zebrin stripes and its cerebrocerebellar pathway. (A) This is a flattened cerebellar cortex showing zebrin stripes. Zebrin is a glycolytic enzyme expressed in cerebellar Purkinje cells. This figure shows the parasagittal stripes, a stereotyped array of transverse zones with an immunostaining reactive and non-reactive pattern. (B) The zebrin-positive zones (C2, D1, and D2 zones) are non-motor regions of the cerebellum. (C) The zebrin-negative zones (C1, C3, and Y zones) are motor regions of the cerebellum that receive somatosensory input. Each of the zones innervate different and specific olivo–cortico–nuclear pathways. Adapted from Voogd (17). A, A zone; B, B zone;C1–3, zones C1–3; D, dorsomedial cell column; D1,2, zones D1,2; IP, posterior interposed nucleus; MAO, medial accessory olive; PO, principal olive; VII–X, lobules VII–X of Larsell; X, X zone.
The functions of these zebrin patterns are potentially related to long-term potentiation (LTP) and long-term depression (LTD) (24). LTP produces a long-lasting increase in neuron activity based on the recent activation history of the cell, which is a well-characterized form of synaptic plasticity, especially in memory. In contrast, LTD produces a long-lasting decrease in synaptic strength, which is important for motor learning (25). LTD in zebrin-negative Purkinje cells is easier to induce than in zebrin-positive Purkinje cells (5, 25). This may represent the predominant form of plasticity in zebrin-positive zones (26), which is correlated with the non-motor function of the cerebellum. In summary, not only zebrin-positive/-negative cells and their specific connections, but also the physiological capacities of the zebrin cells may be a fundamental contributor to the cerebellar system. We consider that the zebrin patterns may be the cornerstone of the cerebellar functions and related to the clinical presentation of cerebellar disorders.
Cerebellar Functional Mapping from Neuroimaging Studies
It is difficult to trace the projection from the cerebral cortex to the cerebellum in the cortico–ponto–cerebellar pathway because the cerebrocerebellar connections are indirect and polysynaptic. Currently, the sole in vivo method to delineate neuronal pathways is tractography, based on diffusion-weighted imaging (27). However, the polysynaptic cerebrocerebellar pathways, which have contralateral connections, pass through the cerebellar deep nucleus and penetrate into the heavily folded cerebellar cortex; these factors make cerebrocerebellar circuits difficult to trace in vivo (28–31). Even so, ex vivo studies using retrograde transsynaptic tracers (rabies virus or herpes simplex virus) have identified a few cerebrocerebellar connections, including cerebello–thalamo–cortical and cortico–ponto–cerebellar pathways, and have shown that the primary motor cortex (M1) is linked with cerebellar lobules III–VI and VIII whereas dorsolateral prefrontal cortex area 46 is linked to crus II and lobule X (32, 33). This evidence suggests that cerebrocerebellar circuits are involved in sensorimotor control and higher cognitive functions such as attention, executive control, language, working memory, learning, pain, emotion, and addiction (34, 35).
Because the cerebellum was initially considered to be responsible for only motor control and its complicated polysynaptic nature, only a few studies made connections between cognition and the cerebellum. For example, a notable exception was Petersen et al., who used positron emission tomography (PET) in 1988 to demonstrate that crus I and crus II in the right cerebellum are involved in the linguistic single-word processing of verbs when hearing some objects, such as “drink” water (36). More imaging studies about the latest neuroimaging techniques and findings related to the cerebellum are discussed next.
Resting-State fMRI Studies of Cerebellum
Resting-state functional magnetic resonance imaging (fMRI) is commonly used to study functional topography. In particular, resting-state functional connectivity (FC) fMRI has revealed a relationship between the cerebellum and several non-motor brain networks, including the somatomotor, frontoparietal, dorsal attention, ventral attention, limbic, salience, executive control, and default-mode networks (2). A unique cerebellar functional topography was demonstrated, with different regions being correlated with different non-motor networks [Figure 3A; (2, 39)]. The sensorimotor cerebellum involves in the anterior lobe, in lobule VIII and part of lobule VI, whereas the cognitive cerebellum involves in the posterior lobe (especially crus I and crus II) and vermis (40). In connectivity studies, the intrinsic connectivity networks (ICNs), the functional coupling between the distant brain cortex and the cerebellum, showed network mapping, including motor networks in the anterior lobe and lobule VIII and cognitive networks (dorsal attention, ventral attention, frontoparietal, default-mode, and salience networks) in the posterior lobe (2, 3, 39).
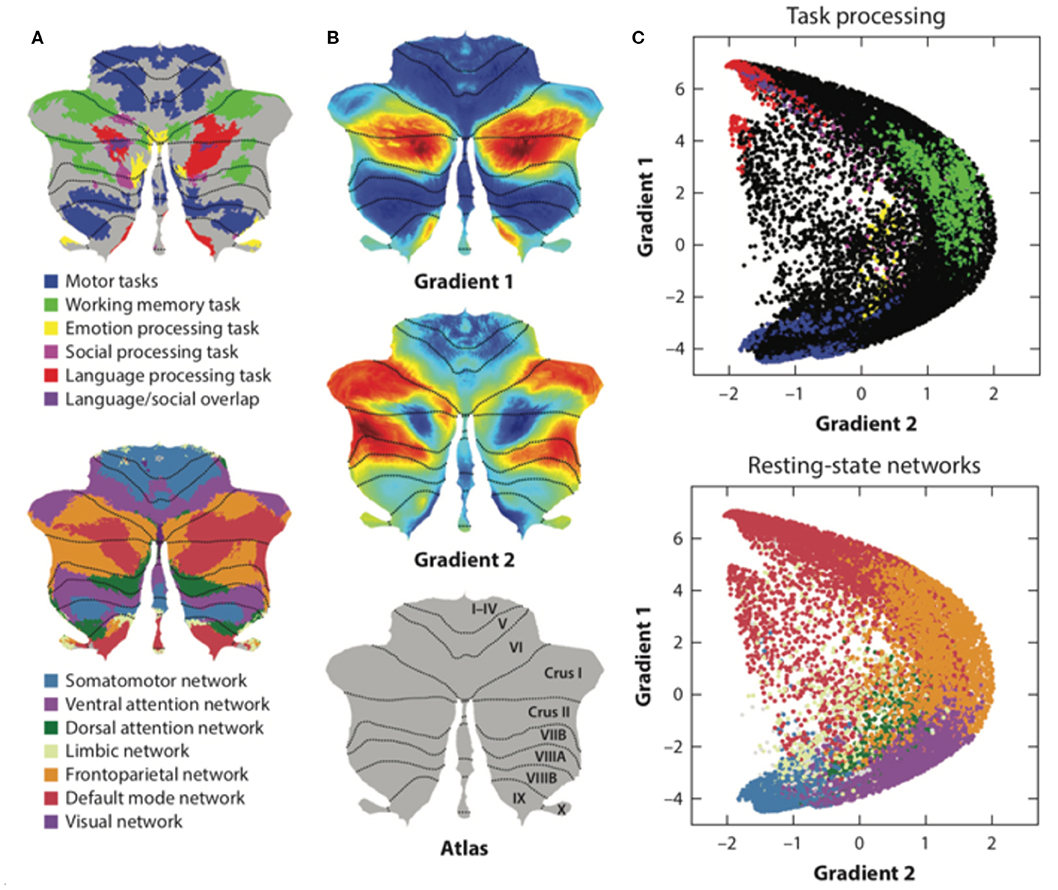
Figure 3. The functional maps and functional gradient of the cerebellum. (A) Resting-state functional fMRI shows cerebellar functional topography and is correlated with different non-motor networks, such as somatomotor, fronto-parietal, dorsal attention, ventral attention, limbic networks, salience network, executive control circuitry, and the default-mode network. Task-evoked fMRI research has shown that lobule V is activated for sensorimotor tasks; VIIIA/B for motor tasks; VIIIB for somatosensory activation; lobule VI and crus I for language and verbal working memory; lobule VI for spatial tasks; lobules VI, crus I, and VIIB for executive functions; and lobules VI, crus I, and medial VII for emotional processing. (B,C) Axis I extends from the primary motor to transmodal regions with the primary–unimodal–transmodal hierarchical principle. Axis II isolates the working memory/frontoparietal network areas and extends from task-unfocused to task-focused processing. The results follow a gradual organization of the well-established cerebellar distributions by using the functional gradient method. Adapted from Buckner et al. (2), Guell et al. (37), and Schmahmann et al. (38).
However, both cognitive and sensorimotor clusters are present within lobule VI. The sensorimotor network clusters are located more centrally and closer to the paramedian part of the cerebellum. The cognitive network clusters are located more laterally and closer to the post-erosuperior fissure (39, 40). More recent studies have demonstrated that lobule VI is a hub controlling sensorimotor and motivations. Lobule VI is an integrative interface between motor and cognitive/emotional circuits during a motor task with verbal encouragement. This hub controlling function may explain the overlap of both cognitive and sensorimotor clusters in lobule VI in fMRI studies.
Task-Evoked fMRI Studies of Cerebellum
Task-evoked fMRI detected blood-oxygen-level dependent (BOLD) signals changes in cerebellum when different tasks performed, such as sensorimotor tasks, language tasks, verbal working memory tasks, spatial tasks, executive function tasks, and emotional processing tasks. Task-evoked fMRI studies have shown that lobule V is activated during sensorimotor tasks; VIIIA/B during motor tasks; VIIIB during somatosensory tasks; lobule VI and crus I during language and verbal working memory tasks; lobule VI during spatial tasks; lobule VI, crus I, and VIIB during executive function tasks; and lobule VI, crus I, and medial VII during emotional processing (1). The functional lateralization of the cerebellum was noted in task-evoked fMRI studies (for example, the language task was linked to the right side of cerebellar lobule VI and crus I, and the spatial task was linked to the left side of cerebellar lobule VI), indicating that the information processing pathways crossed hemispheres between the cerebral cortex and cerebellum (1). However, the motor control system of the cerebellum is “double-crossed” (which is different from the single-crossed non-motor system in the cerebellum): The first crossing occurs in the decussation of the superior cerebellar peduncle while the second crossing occurs in the corticospinal or rubrospinal tracts descending into the spinal cord. Therefore, the cerebellar hemisphere modulates ipsilateral limb movements.
Moreover, social cognition tasks, including theory of mind (mirroring, event mentalizing, person mentalizing, abstraction) (41) and emotional affective processing (a painful experience of our own or from others), have also been examined in fMRI studies (40, 42). In these studies, a theory of mind task activated crus I in the bilateral cerebellum; an emotional affective processing task showed that the posterior cerebellar vermis was related to painful first-person experiences whereas the posterior cerebellar hemisphere (lobule VI) was related to empathetic pain on behalf of others (Figure 3A). In summary, cerebellar functional topography was revealed by multiple task-evoked MRIs with both cerebrum and cerebellum activation. This result emphasizes the role of the cerebellum in both motor and non-motor functions as well as its cerebrocerebellar connections. All these cerebrocerebellar connections project to different Purkinje cells. Further studies should focus on these cerebrocerebellar pathways and their relationships to zebrin patterns.
Functional Gradient Neuroimaging Studies of Cerebellum
Functional and anatomical structure matches are crucial for the definition of cerebellar functional neuroanatomy, which means that anatomy reflects a functional hierarchy from primary to transmodal processing (43). Recently, Guell et al. demonstrated a novel functional imaging technique. This new method applies diffusion maps embedded into the brain's resting-state imaging results followed by two principal gradients of resting-state FC in the cerebral cortex. Axis I extends from the primary motor to transmodal regions with the primary–unimodal–transmodal hierarchical principle (43). Axis II isolates working memory/frontoparietal network areas and extends from task-unfocused to task-focused processing. The results follow a gradual organization of the well-established cerebellar distributions by using the functional gradient method [Figures 3B,C; (37)]. The gradients were interpreted by analyzing their relationship to resting-state and task-evoked fMRI cerebellar maps (37). When each cerebellar anatomical voxel was arranged along the two functional axes, a gradual pattern of organization emerged.
Even so, the functional maps (including resting fMRI and task-evoked fMRI) do not necessarily align with the anatomical lobules. Recently, King et al. compared the similarity of paired voxels within a region across a anatomical boundary using a multidomain task battery in fMRI and revealed that, although functional boundaries existed, they were not aligned with either anatomic lobules or zebrin stripe (44). Bernard et al. also demonstrated that the lobular boundaries were not necessarily indicative of functional boundaries by comparing the anatomical and self-organizing map approaches in the resting-state cortico-cerebellar FC networks (3). These neuroimaging studies also support the “one-map” hypothesis by unifying the cerebellar map not only anatomically but also functionally (23).
The Role of Cerebellum in Neurological Diseases
Next, we review studies of diseases related to cerebellar dysfunction, with a particular focus on clinical ataxiology and its contributing clinical expression of cerebellar pathology: cerebellar motor syndrome, vestibular cerebellar syndrome, and cerebellar cognitive affective syndrome (CCAS) (45). CCAS, also called Schmahmann's syndrome, was first described in 1998; it presents with neuropsychiatric symptoms, such as the blunting of affect, lack of initiation, apathy, depression, and loss of empathy or disinhibited, irritable, and inappropriate behavior (4, 46). These studies provide evidence that underlies the complexity of cerebellar symptoms despite the undergoing disease and our understanding of cerebellar function, through the various forms of pathophysiology they encompass.
Cerebellar Lesions
Cerebellar stroke patients typically present with dizziness, ataxia, dysmetria, and postural imbalance. However, atypical cerebellar stroke patients may present with only dizziness or CCAS and without obvious cerebellar symptoms or signs by neurological examinations, which are difficult to diagnose. Moreover, these cerebellar strokes lead to notably poor outcomes (47). Following cerebellar stroke, cerebellar motor syndrome was shown to be associated with the anterior lobe, while CCAS was associated with the posterior lobe in a voxel-based lesion symptom mapping study analyzing relationships between behavioral deficits in neurological populations and lesion sites associated with those deficits (48). Cerebellar mutism syndrome (CMS) was also noted in children after surgery for tumors in the posterior fossa, which presented as mutism, emotional lability, hypotonia, and ataxia (49, 50). Patients with tumor compression to the cerebellum, such as posterior fossa arachnoid cyst or Chiari malformation, also presented with neurodevelopmental and psychiatric symptoms (developmental delay, intellectual disability, autistic, and obsessive-compulsive symptoms) (51).
In summary, cerebellar lesions located in the anterior lobe and parts of lobule VI interrupted cerebellar communication with cerebral and spinal motor systems and caused cerebellar motor syndrome. Cerebellar lesions located in the posterior lobe (lobules VI and VII) interrupted the cerebellar modulation of cerebrocerebellar cognitive loops and caused cognitive impairments. Finally, cerebellar lesions located in the vermis interrupted cerebrocerebellar limbic loops and caused neuropsychiatric symptoms (40).
Spinocerebellar Ataxias
Spinocerebellar ataxias (SCAs) are rare inherited neurodegenerative cerebellar disorders with clinical and genetic heterogeneities. The most clinically significant symptoms of SCAs are ataxia, dysmetria, dysarthria, and oculomotor signs (52, 53). The prevalence of SCA subtypes varies across populations (54–56). SCA1, SCA2, and SCA3 are the most common subtypes in Caucasians, while SCA2, SCA3, and SCA6 are more frequently encountered in Japanese and Chinese people (57, 58). Nonataxia symptoms, including cognitive impairment, neuropsychiatric symptoms, and social cognition deficits, have been found in SCA patients (59–62). Cerebellar volume decreases have been noted in SCA patients. SCA1, SCA2, and SCA3 patients presented with atrophy in the cerebellar hemispheres and vermis as well as the brainstem. Atrophy in the cerebellar hemispheres was less severe in SCA3 patients than in SCA1 and SCA6 patients. However, atrophy in those with SCA6 was restricted to the pure cerebellar cortex without vermis and brainstem (63, 64). Functional MRI connectivity studies in SCA2 and SCA3 patients showed decreased connectivity between the sensorimotor area in the cerebral cortex and the cerebellum (65, 66). In SCA6 patients, cerebellar lobules V and VI and the dentate nuclei were more active compared to controls when performing a hand-movement task (67). DTI studies in SCA6 patients also showed increased connectivity between the cerebral cortex (especially the occipital cortex) and cerebellum in moderate cases and decreased connectivity in severe cases (68). This result may indicate a compensatory phenomenon in the cerebrocerebellum circuit in response to spinocerebellum dysfunction in SCA6 patients (69). Indeed, patients with SCA6 progress slower than those with SCA1, SCA2, SCA3, and SCA17 and have longer disease durations (70). The chronic nature of SCA6 may be related to compensatory phenomena involving increased cerebrocerebellar connections during cerebellar degeneration.
Recently, Hashimoto and Honda et al. demonstrated different internal model disruptions in aging people and spinocerebellar ataxia patients (SCA6 and SCA31) by using prism adaptation tasks (71, 72). These studies also established links between the internal models and the clinical presentation of cerebellar disorders. More studies are needed to investigate the relationship between clinical presentation and internal models.
Neurodegenerative Disease and the Cerebellum
Alzheimer's disease (AD) is the most common form of dementia. The deposits of amyloid plaques and ubiquitin-immunoreactive dystrophic neurites are found in not only the cerebral cortex, but also the cerebellum (73, 74). Decreased volumes of cerebellar gray matter have been found in patients with early-onset AD (75). However, whether there are changes in the FC of the cerebrocerebellar connections in patients with AD and mild cognitive impairment (MCI) remains a controversial question. Some resting-state fMRI studies have shown decreased FC of the cerebrocerebellar connections in MCI and AD patients (76, 77). However, in another study, decreased FC of the cerebrocerebellar connections in resting-state networks was found in the AD group whereas a significant increase in FC was noted in the MCI group (78). This connectivity difference indicated a compensatory phenomenon in the cerebrocerebellum connection in the early stages of AD; in other words, these controversial results may have been due to connectivity changes during the clinical progression of AD. Future studies could focus on subgroup analyses based on the clinical cognitive impairment severity to investigate the cerebrocerebellum connectivity differences at each of the different stages of disease progression (69, 78). In addition, a syndrome with cognitive impairment and dynapenia, called physio-cognitive decline syndrome (PCDS), is considered to be correlated with the cerebellum (79, 80). Chen et al. demonstrated reduced gray matter volume (GMV) in the cerebellum, hippocampi, middle frontal gyri, and several other cerebral regions in the prefrail and frail groups compared to the robust group (81).
Parkinson's disease (PD) is also a common neurodegenerative disorder associated with motor and cognitive impairments. The main pathophysiology of PD is the degeneration of dopaminergic neurons in the striato–thalamo–cortical pathways. The anatomical connections between the basal ganglia and cerebellum have been elucidated (82). Cerebellar atrophy has been noted in PD and Parkinson plus syndromes [including multiple system atrophy (MSA) and progressive supranuclear palsy (PSP)] (83–86). Connectivity DTI studies have shown decreased FA in the cerebellar hemispheres in PD patients (87). However, increased connectivity in the cerebellum, primary sensorimotor cortex, and premotor area was found when using a regional homogeneity (ReHo) method in fMRI (88). In addition, enhanced connectivity between the dentate nucleus and cerebellum in PD patients was noted in resting-state fMRI (89). The reasons for the increased connectivity observed in these studies are still unknown, but the findings suggest that cerebellar involvement in PD is compensatory (90).
Multiple Sclerosis and the Cerebellum
Multiple sclerosis (MS) is an inflammatory disease with clinical mono- or polysymptomatic presentations depending on the location of the demyelinating lesion. In some cases, there are asymptomatic lesions as well-symptoms without corresponding lesions. The most common presentations are optic neuritis, cerebellum, brainstem, and spinal cord syndromes (91). The cerebellum is one of the most commonly involved brain regions in MS patients, with cerebellar lesions being described in approximately half of all cases in clinically defined MS (92). Cerebellar structural atrophy in MRI studies has been noted in MS patients (93). D'Ambrosio et al. demonstrated that cerebellar volumetric abnormalities were correlated with the clinical symptoms and motor and cognitive performance impairments in MS patients. Lower anterior cerebellar volume and brain T2 lesion volume predicted worse motor performance, whereas lower posterior cerebellar volume and brain T2 lesion volume predicted poor cognitive performance (94), which maps into the previously described functional cerebellar topography (40). In addition, resting-state fMRI in progressive MS showed reduced FC between crus II and the right frontal pole and increased FC between lobule VIIb and the right precentral gyrus after controlling for structural damage (95). Sbardella et al. also showed enhanced dentate FC to frontal and parietal cortical areas in MS patients compared to healthy controls, and the increased connectivity was related to better cognitive performance (96). These studies indicate the plasticity of cerebrocerebellar circuits and functional compensation when damage occurs (69, 97).
In summary, the clinical presentation of ataxia is typically taken as the first sign of cerebellar disease, but neuropsychiatric impairments are often not diagnosed at that stage—perhaps in part because the cerebellum is still perceived by some physicians as being responsible purely for motor control, meaning the relevance of non-motor symptoms to the cerebellum might be overlooked. A second reason for the lack of early diagnosis may be the lack of neuropsychiatric assessment tools for CCAS. Recently, Schmahmann developed a scale for evaluating the clinical neuropsychiatric symptoms in patients with cerebellar disorders, including patients with Mini-Mental State Examination (MMSE) scores >28 (i.e., normal cognitive function). The Schmahmann's syndrome scale includes tests of executive function, language, visual spatial function, and affect regulation with sensitivity and selectivity for detecting patients with CCAS of 85%/74% in exploratory cohorts and 95%/78% in validation cohort studies (98). By using this CCAS scale, we can clinically identify patients with cerebellar non-motor symptoms. The third reason could be that ataxia and dysmetria symptoms present earlier than non-ataxia symptoms. Interestingly, neuroimaging studies in AD, PD, MS, and SCA patients showed compensatory phenomena (69, 88, 89, 97). We hypothesized that these compensatory phenomena are related to zebrin patterning based on the evidence from molecular, functional imaging, and clinical studies (see next).
Combination of Multiple Neuroimaging Studies of the Cerebellum and Zebrin
Compensatory Phenomena and Zebrin
We hypothesized that zebrin-positive cells contribute to the compensatory phenomena observed in cerebellar-related chronic neurodegenerative diseases. In particular, zebrin-positive cerebellar Purkinje cells are resistant to excitotoxicity, cell injury, and degenerate slowly (99), the brain has time to compensate for disrupted cerebral function by increasing the cerebrocerebellar connectivity. Indeed, several neuroimaging studies have shown enhanced cerebrocerebellar connectivity in chronic neurodegenerative diseases (such as SCAs, AD, PD, and MS) (69, 88, 89, 97). The compensatory phenomena in the cerebrocerebellar circuit indicate the potential role of plasticity in the cerebellum.
This hypothesis can also explain why the ataxia and dysmetria symptoms present earlier than non-ataxia symptoms and the neuropsychiatric impairment symptoms of CCAS are less severe than the ataxic symptoms. Because zebrin-positive cells have been associated with the non-motor cortical regions and zebrin-negative cells have been associated with motor cortical regions (17). We suggest that motor control dysfunction symptoms such as ataxia and dysmetria present earlier and are easier to observe than non-ataxia symptoms due to zebrin-negative cell damage. Cerebellar zebrin-positive cells are resistant to cell injury (99) while zebrin-negative zones are more prone to damage (12, 18), suggesting that zebrin-positive Purkinje cells and its cerebrocerebellar connection are preserved with normal non-motor functions when brain damage occurs. We believe that the clinical presentations of these diseases and neuroimaging studies might be connected with cerebellar anatomical zebrin patterns, suggesting a new way to investigate these cerebellar disorders.
Triple Functional Representation of the Cerebellum
Triple functional representation topography of the cerebellum was demonstrated by Guell et al.—namely, primary, secondary, and tertiary representation (Figure 4). The primary and secondary representations showed a mirror-symmetry order of the functional topographic maps of language, working memory, and social and emotion processing by task-evoked and seed-based resting-state fMRI (37, 101). The symmetrical patterns of primary and secondary representation are similar to up-and-down symmetrical molecular zebrin patterns, which may indicate that those cerebellar areas with the same functions (with the same color in Figure 4) have similar zebrin cell properties. Furthermore, these symmetrical cerebellar areas carry identical inputs from cerebrocerebellar pathways with specific correspondence. For example, the symmetrical motor function representations carry inputs from the motor cortex. Interestingly, the tertiary representation was located in the vermis and para-vermis region, which are not symmetrical to primary and secondary representation. We hypothesized that three representations of the cerebellum maybe corelated to its cerebrocerebellar pathways. The functional synaptic organization of cerebrocerebellar fibers in vermis were conserved in a study with mice compared to 85% synaptic silence in the cerebellar hemisphere (102, 103). Therefore, the triple functional representation topography may be explained by an up-and-down symmetrical molecular zebrin pattern with specific cerebrocerebellar pathways as well as one vermis/paravermis region that preserves multiple functional pathways. The function of the vermis also supports its multiple pathways, which is responsible for not only body posture, locomotion, and eye movement (the so-called spinocerebellum), but also emotional behavioral changes and autonomic functions (the so-called limbic-connected cerebellum) (8, 42). This symmetrical observation implies the importance of the zebrin patterns and their correlation to functional maps. Further research might be critical for making the connection among molecular, anatomical, and functional representations.
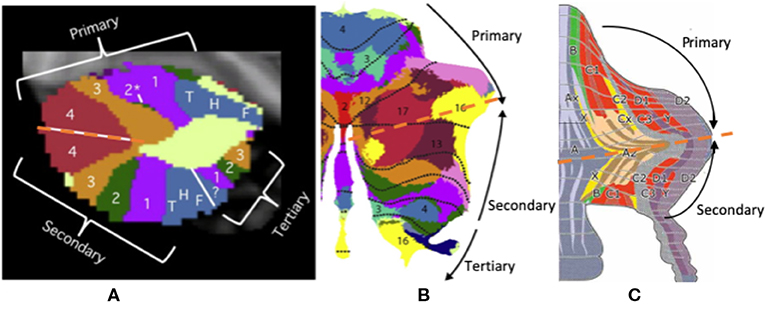
Figure 4. The triple presentation of cerebellar functional topography. (A,B) This figure shows the triple functional representation topography in the cerebellum: primary, secondary, and tertiary representation. The primary and secondary representations show a mirror-symmetry order of the functional topographic maps of language, working memory, and social and emotional processing by task-evoked and seed-based resting-state fMRI. (C) The symmetrical pattern of primary and secondary representation is similar to up-and-down symmetrical molecular zebrin patterns. Adapted from Voogd (17), Buckner et al. (2), and Diedrichsen et al. (100).
Taking the many neuroimaging and disease studies of the cerebellum together, we noticed that the traditional human cerebellar anatomical lobules are very different from the molecular zebrin patterns and cerebellar functional topographic maps. By unifying the anatomical, molecular, and functional topography, we can explain how the cerebellum works through cerebrocerebellum circuits, affecting the various networks. Many neuroimaging studies have revealed cerebellar functional topographical features that are correlated with different brain networks related to motor and non-motor functions. These functional areas cross anatomical lobule borders (3, 44), indicating that functional maps are different from anatomical topography. Considering the similarity of three functional representations and zebrin patterns of cerebellum, the zebrin-positive/-negative cells' properties may be more crucial to cerebellar functions. Due to the current limitations of neuroimaging techniques, we cannot demonstrate zebrin stripe in conventional MRI studies. However, Boillat et al. showed similar longitudinal stripe-like patterns by using quantitative T1 and T2* mapping at ultrahigh field (7T) MRI (104). Solodkin et al. also demonstrated the visualization of zebrin-like patterns in SCA1patients by fMRI connectivity and this zebrin patterns diminished during the disease progression of SCA1 (105). Therefore, by separating the zebrin-like signals in neuroimaging studies, we might be able to study the alteration and function of zebrin stripe and its relationship to clinical disease.
Conclusions
In this article, we reviewed cerebellum-related studies from multiple viewpoints, including aspects of molecular function, structural features, functional imaging, and clinical diseases. Due to the complexity and incomplete nature of the field being reviewed, the overall picture of the mechanisms underlying cerebellar functions remains confusing and challenging. We emphasized in particular the roles of zebrin stripe in the cerebellum, which have been found to be related to specific climbing fibers and their olivo–cortico–nuclear pathways. Evidence from several neuroimaging studies has demonstrated the presentations of cerebellar functional topography, which is similar to the zebrin pattern. The cerebellar functional maps cross the borders of the conventional anatomical cerebellar lobules. Functional imaging studies have also shown compensatory phenomena in cerebellum-related diseases, such as AD, PD, MS, and SCA. We hypothesized that these compensatory phenomena are related to zebrin patterning and offered a new perspective on cerebellar functions based on the evidence from molecular, functional imaging, and clinical studies.
This review has some limitations. The precise relationships among the anatomical cerebellum, molecular stripe patterns, and functional topography are unknown. The neuroimaging studies showed zebrin-like patterns, but we need more studies to confirm the zebrin-like patterns in imaging studies are identical to the molecular zebrin patterns. The functional imaging of molecular zebrin pattern is still being developed. Furthermore, a computational model of cerebrocerebellar connections that addresses how the cerebellum processes information and regulates cerebrocerebellar network circuits is still unavailable.
Several fundamental theories of cerebellar function exist. One of the most famous theories regarding cerebellum functionality is the dysmetria of thought theory based on the universal cerebellar transform (UCT) (4, 38, 46, 106, 107). This model implies a general function for regulating multiple circuits. In this framework, impairments in UCT function cause the dysregulation of these subcircuits that leads to functional loss, which has been called “the dysmetria of thought theory” (108). However, multiple functionality theory suggests unique and diverse functions for the different circuits (100). The electrophysiology of the zebrin pattern is the key that holds the answer regarding which fundamental theory is preferable. If zebrin-positive and zebrin-negative cells have similar electrophysiological cell activity but different functions due to distinct and specific olivo–cortico–nuclear pathways, the universal cerebellar transform is supported. However, if zebrin-positive and zebrin-negative cells have different electrophysiological activities coupled with different pathways, the multiple functionality theory is more likely. We need more studies to investigate these fundamental theories.
Furthermore, the compensatory phenomena resulting in enhanced cerebrocerebellar circuits are important for plasticity and learning. Cerebellar stimulation with techniques such as rTMS or TDCS and the application of other forms of cerebrocerebellar circuit neuromodulation may be effective for the control of motor symptoms or CCAS in patients with cerebellar lesions. At present, cerebellar plasticity produced by cerebellar regulation is still a new research topic. More research is required to understand the neural mechanisms, their relationship to zebrin-positive and zebrin-negative cells (5), and their association with the neuromodulation of the cerebellum based on this knowledge with potential therapeutic applications for different cerebellar disorders (6).
Author Contributions
Y-CL, P-NW, C-PL, and L-HC: conceptualization of the study. Y-CL, C-CH and L-HC: manuscript.
Funding
This work was supported by the Ministry of Science and Technology, Taiwan (108-2410-H-010-007-MY3, 108-2321-B-010-010-MY2, and 107-2410-H-010-010-001), Veterans General Hospitals and University System of Taiwan Joint Research Program (VGHUST109-V1-2-2), UST X108UST05, and Aging and Health Research Center, National Yang-Ming University, Taiwan.
Conflict of Interest
The authors declare that the research was conducted in the absence of any commercial or financial relationships that could be construed as a potential conflict of interest.
References
1. Stoodley CJ, Schmahmann JD. Functional topography in the human cerebellum: a meta-analysis of neuroimaging studies. Neuroimage. (2009) 44:489–501. doi: 10.1016/j.neuroimage.2008.08.039
2. Buckner RL, Krienen FM, Castellanos A, Diaz JC, Yeo BT. The organization of the human cerebellum estimated by intrinsic functional connectivity. J Neurophysiol. (2011) 106:2322–45. doi: 10.1152/jn.00339.2011
3. Bernard JA, Seidler RD, Hassevoort KM, Benson BL, Welsh RC, Wiggins JL, et al. Resting state cortico-cerebellar functional connectivity networks: a comparison of anatomical and self-organizing map approaches. Front Neuroanat. (2012) 6:31. doi: 10.3389/fnana.2012.00031
4. Schmahmann JD, Sherman JC. The cerebellar cognitive affective syndrome. Brain. (1998) 121(Pt 4):561–79. doi: 10.1093/brain/121.4.561
5. Hawkes R. Purkinje cell stripes and long-term depression at the parallel fiber-Purkinje cell synapse. Front Syst Neurosci. (2014) 8:41. doi: 10.3389/fnsys.2014.00041
6. Azevedo FA, Carvalho LR, Grinberg LT, Farfel JM, Ferretti RE, Leite RE, et al. Equal numbers of neuronal and nonneuronal cells make the human brain an isometrically scaled-up primate brain. J Comp Neurol. (2009) 513:532–41. doi: 10.1002/cne.21974
7. Manni E, Petrosini L. A century of cerebellar somatotopy: a debated representation. Nat Rev Neurosci. (2004) 5:241–9. doi: 10.1038/nrn1347
8. Apps R, Hawkes R, Aoki S, Bengtsson F, Brown AM, Chen G, et al. Cerebellar modules and their role as operational cerebellar processing units: a consensus paper [corrected]. Cerebellum. (2018) 17:654–82. doi: 10.1007/s12311-018-0959-9
9. Ito M, Yoshida M. The cerebellar-evoked monosynaptic inhibition of Deiters' neurones. Experientia. (1964) 20:515–6. doi: 10.1007/BF02154085
10. Lang EJ, Sugihara I, Welsh JP, Llinas R. Patterns of spontaneous purkinje cell complex spike activity in the awake rat. J Neurosci. (1999) 19:2728–39. doi: 10.1523/JNEUROSCI.19-07-02728.1999
11. Bayer HM, Glimcher PW. Midbrain dopamine neurons encode a quantitative reward prediction error signal. Neuron. (2005) 47:129–41. doi: 10.1016/j.neuron.2005.05.020
12. Hawkes R, Leclerc N. Purkinje cell axon collateral distributions reflect the chemical compartmentation of the rat cerebellar cortex. Brain Res. (1989) 476:279–90. doi: 10.1016/0006-8993(89)91248-1
13. Rocchi M, Vitale E, Covone A, Romeo G, Santamaria R, Buono P, et al. Assignment of human aldolase C gene to chromosome 17, region cen–q21. Hum Genet. (1989) 82:279–82. doi: 10.1007/BF00291170
14. Graham DJ, Wylie DR. Zebrin-immunopositive and -immunonegative stripe pairs represent functional units in the pigeon vestibulocerebellum. J Neurosci. (2012) 32:12769–79. doi: 10.1523/JNEUROSCI.0197-12.2012
15. Arakaki TL, Pezza JA, Cronin MA, Hopkins CE, Zimmer DB, Tolan DR, et al. Structure of human brain fructose 1,6-(bis)phosphate aldolase: linking isozyme structure with function. Protein Sci. (2004) 13:3077–84. doi: 10.1110/ps.04915904
16. Voogd J, Glickstein M. The anatomy of the cerebellum. Trends Neurosci. (1998) 21:370–5. doi: 10.1016/S0166-2236(98)01318-6
17. Voogd J. What we do not know about cerebellar systems neuroscience. Front Syst Neurosci. (2014) 8:227. doi: 10.3389/fnsys.2014.00227
18. Zhou H, Lin Z, Voges K, Ju C, Gao Z, Bosman LW, et al. Cerebellar modules operate at different frequencies. Elife. (2014) 3:e02536. doi: 10.7554/eLife.02536.018
19. Jorntell H, Ekerot C, Garwicz M, Luo XL. Functional organization of climbing fibre projection to the cerebellar anterior lobe of the rat. J Physiol. (2000) 522 (Pt 2):297–309. doi: 10.1111/j.1469-7793.2000.00297.x
20. Larson B, Miller S, Oscarsson O. Termination and functional organization of the dorsolateral spino-olivocerebellar path. J Physiol. (1969) 203:611–40. doi: 10.1113/jphysiol.1969.sp008882
21. Andersson G, Oscarsson O. Projections to lateral vestibular nucleus from cerebellar climbing fiber zones. Exp Brain Res. (1978) 32:549–64. doi: 10.1007/BF00239552
22. Voogd J, Ruigrok TJ. The organization of the corticonuclear and olivocerebellar climbing fiber projections to the rat cerebellar vermis: the congruence of projection zones and the zebrin pattern. J Neurocytol. (2004) 33:5–21. doi: 10.1023/B:NEUR.0000029645.72074.2b
23. Apps R, Hawkes R. Cerebellar cortical organization: a one-map hypothesis. Nat Rev Neurosci. (2009) 10:670–81. doi: 10.1038/nrn2698
24. De Zeeuw CI, Ten Brinke MM. Motor learning and the cerebellum. Cold Spring Harb Perspect Biol. (2015) 7:a021683. doi: 10.1101/cshperspect.a021683
25. Wadiche JI, Jahr CE. Multivesicular release at climbing fiber-Purkinje cell synapses. Neuron. (2001) 32:301–13. doi: 10.1016/S0896-6273(01)00488-3
26. Wadiche JI, Jahr CE. Patterned expression of Purkinje cell glutamate transporters controls synaptic plasticity. Nat Neurosci. (2005) 8:1329–34. doi: 10.1038/nn1539
27. Basser PJ, Pajevic S, Pierpaoli C, Duda J, Aldroubi A. In vivo fiber tractography using DT-MRI data. Magn Reson Med. (2000) 44:625–32. doi: 10.1002/1522-2594(200010)44:4<625::AID-MRM17>3.0.CO
28. Kamali A, Kramer LA, Frye RE, Butler IJ, Hasan KM. Diffusion tensor tractography of the human brain cortico-ponto-cerebellar pathways: a quantitative preliminary study. J Magn Reson Imaging. (2010) 32:809–17. doi: 10.1002/jmri.22330
29. Keser Z, Hasan KM, Mwangi BI, Kamali A, Ucisik-Keser FE, Riascos RF, et al. Diffusion tensor imaging of the human cerebellar pathways and their interplay with cerebral macrostructure. Front Neuroanat. (2015) 9:41. doi: 10.3389/fnana.2015.00041
30. Palesi F, Tournier JD, Calamante F, Muhlert N, Castellazzi G, Chard D, et al. Reconstructing contralateral fiber tracts: methodological aspects of cerebello-thalamocortical pathway reconstruction. Funct Neurol. (2016) 31:229–38. doi: 10.11138/FNeur/2016.31.4.229
31. Palesi F, De Rinaldis A, Castellazzi G, Calamante F, Muhlert N, Chard D, et al. Contralateral cortico-ponto-cerebellar pathways reconstruction in humans in vivo: implications for reciprocal cerebro-cerebellar structural connectivity in motor and non-motor areas. Sci Rep. (2017) 7:12841. doi: 10.1038/s41598-017-13079-8
32. Middleton FA, Strick PL. Anatomical evidence for cerebellar and basal ganglia involvement in higher cognitive function. Science. (1994) 266:458–61. doi: 10.1126/science.7939688
33. Kelly RM, Strick PL. Cerebellar loops with motor cortex and prefrontal cortex of a nonhuman primate. J Neurosci. (2003) 23:8432–44. doi: 10.1523/JNEUROSCI.23-23-08432.2003
34. Ramnani N. The primate cortico-cerebellar system: anatomy and function. Nat Rev Neurosci. (2006) 7:511–22. doi: 10.1038/nrn1953
35. Strick PL, Dum RP, Fiez JA. Cerebellum and nonmotor function. Annu Rev Neurosci. (2009) 32:413–34. doi: 10.1146/annurev.neuro.31.060407.125606
36. Petersen SE, Fox PT, Posner MI, Mintun M, Raichle ME. Positron emission tomographic studies of the cortical anatomy of single-word processing. Nature. (1988) 331:585–9. doi: 10.1038/331585a0
37. Guell X, Schmahmann JD, Gabrieli J, Ghosh SS. Functional gradients of the cerebellum. Elife. (2018) 7:e36652. doi: 10.7554/eLife.36652.022
38. Schmahmann JD, Guell X, Stoodley CJ, Halko MA. The theory and neuroscience of cerebellar cognition. Annu Rev Neurosci. (2019) 42:337–64. doi: 10.1146/annurev-neuro-070918-050258
39. Habas C, Kamdar N, Nguyen D, Prater K, Beckmann CF, Menon V, et al. Distinct cerebellar contributions to intrinsic connectivity networks. J Neurosci. (2009) 29:8586–94. doi: 10.1523/JNEUROSCI.1868-09.2009
40. Stoodley CJ, Schmahmann JD. Evidence for topographic organization in the cerebellum of motor control versus cognitive and affective processing. Cortex. (2010) 46:831–44. doi: 10.1016/j.cortex.2009.11.008
41. Van Overwalle F, Baetens K, Marien P, Vandekerckhove M. Social cognition and the cerebellum: a meta-analysis of over 350 fMRI studies. Neuroimage. (2014) 86:554–72. doi: 10.1016/j.neuroimage.2013.09.033
42. Singer T, Seymour B, O'Doherty J, Kaube H, Dolan RJ, Frith CD. Empathy for pain involves the affective but not sensory components of pain. Science. (2004) 303:1157–62. doi: 10.1126/science.1093535
43. Mesulam M. Representation, inference, and transcendent encoding in neurocognitive networks of the human brain. Ann Neurol. (2008) 64:367–78. doi: 10.1002/ana.21534
44. King M, Hernandez-Castillo CR, Poldrack RA, Ivry RB, Diedrichsen J. Functional boundaries in the human cerebellum revealed by a multi-domain task battery. Nat Neurosci. (2019) 22:1371–8. doi: 10.1038/s41593-019-0436-x
45. Manto M, Marien P. Schmahmann's syndrome - identification of the third cornerstone of clinical ataxiology. Cerebellum Ataxias. (2015) 2:2. doi: 10.1186/s40673-015-0023-1
46. Schmahmann JD. Dysmetria of thought: clinical consequences of cerebellar dysfunction on cognition and affect. Trends Cogn Sci. (1998) 2:362–371. doi: 10.1016/S1364-6613(98)01218-2
47. Berry DC, Rafferty A, Tiu K, Platt-Mills TF. Cerebellar stroke: a missed diagnosis. Adv Emerg Nurs J. (2017) 39:184–92. doi: 10.1097/TME.0000000000000150
48. Stoodley CJ, MacMore JP, Makris N, Sherman JC, Schmahmann JD. Location of lesion determines motor vs. cognitive consequences in patients with cerebellar stroke. Neuroimage Clin. (2016) 12:765–75. doi: 10.1016/j.nicl.2016.10.013
49. Rekate HL, Grubb RL, Aram DM, Hahn JF, Ratcheson RA. Muteness of cerebellar origin. Arch Neurol. (1985) 42:697–8. doi: 10.1001/archneur.1985.04060070091023
50. Wells EM, Walsh KS, Khademian ZP, Keating RF, Packer RJ. The cerebellar mutism syndrome and its relation to cerebellar cognitive function and the cerebellar cognitive affective disorder. Dev Disabil Res Rev. (2008) 14:221–8. doi: 10.1002/ddrr.25
51. Guell X, Anteraper SA, Ghosh SS, Gabrieli JDE, Schmahmann JD. Neurodevelopmental and psychiatric symptoms in patients with a cyst compressing the cerebellum: an ongoing enigma. Cerebellum. (2019) 19:16–29. doi: 10.1007/s12311-019-01050-4
52. Bird TD. Hereditary ataxia overview. In: Adam MP, Ardinger HH, Pagon RA, Wallace SE, Bean LJH, Stephens K, Amemiya A, editors. GeneReviews®. Seattle, WA: University of Washington (1993).
53. Soong BW, Morrison PJ. Spinocerebellar ataxias. Handb Clin Neurol. (2018) 155:143–74. doi: 10.1016/B978-0-444-64189-2.00010-X
54. Schols L, Bauer P, Schmidt T, Schulte T, Riess O. Autosomal dominant cerebellar ataxias: clinical features, genetics, and pathogenesis. Lancet Neurol. (2004) 3:291–304. doi: 10.1016/S1474-4422(04)00737-9
55. Soong BW, Paulson HL. Spinocerebellar ataxias: an update. Curr Opin Neurol. (2007) 20:438–46. doi: 10.1097/WCO.0b013e3281fbd3dd
56. Ruano L, Melo C, Silva MC, Coutinho P. The global epidemiology of hereditary ataxia and spastic paraplegia: a systematic review of prevalence studies. Neuroepidemiology. (2014) 42:174–83. doi: 10.1159/000358801
57. Soong BW, Lu YC, Choo KB, Lee HY. Frequency analysis of autosomal dominant cerebellar ataxias in Taiwanese patients and clinical and molecular characterization of spinocerebellar ataxia type 6. Arch Neurol. (2001) 58:1105–9. doi: 10.1001/archneur.58.7.1105
58. Maruyama H, Izumi Y, Morino H, Oda M, Toji H, Nakamura S, et al. Difference in disease-free survival curve and regional distribution according to subtype of spinocerebellar ataxia: a study of 1,286 Japanese patients. Am J Med Genet. (2002) 114:578–83. doi: 10.1002/ajmg.10514
59. Schmitz-Hubsch T, Coudert M, Bauer P, Giunti P, Globas C, Baliko L, et al. Spinocerebellar ataxia types 1, 2, 3, and 6: disease severity and nonataxia symptoms. Neurology. (2008) 71:982–9. doi: 10.1212/01.wnl.0000325057.33666.72
60. Klinke I, Minnerop M, Schmitz-Hubsch T, Hendriks M, Klockgether T, Wullner U, et al. Neuropsychological features of patients with spinocerebellar ataxia (SCA) types 1, 2, 3, and 6. Cerebellum. (2010) 9:433–42. doi: 10.1007/s12311-010-0183-8
61. Moriarty A, Cook A, Hunt H, Adams ME, Cipolotti L, Giunti P. A longitudinal investigation into cognition and disease progression in spinocerebellar ataxia types 1, 2, 3, 6, and 7. Orphanet J Rare Dis. (2016) 11:82. doi: 10.1186/s13023-016-0447-6
62. Giocondo F, Curcio G. Spinocerebellar ataxia: a critical review of cognitive and socio-cognitive deficits. Int J Neurosci. (2018) 128:182–91. doi: 10.1080/00207454.2017.1377198
63. Burk K, Abele M, Fetter M, Dichgans J, Skalej M, Laccone F, et al. Autosomal dominant cerebellar ataxia type I clinical features and MRI in families with SCA1, SCA2 and SCA3. Brain. (1996) 119(Pt 5):1497–505. doi: 10.1093/brain/119.5.1497
64. Schulz JB, Borkert J, Wolf S, Schmitz-Hubsch T, Rakowicz M, Mariotti C, et al. Visualization, quantification and correlation of brain atrophy with clinical symptoms in spinocerebellar ataxia types 1, 3 and 6. Neuroimage. (2010) 49:158–68. doi: 10.1016/j.neuroimage.2009.07.027
65. Wu T, Wang C, Wang J, Hallett M, Zang Y, Chan P. Preclinical and clinical neural network changes in SCA2 parkinsonism. Parkinsonism Relat Disord. (2013) 19:158–64. doi: 10.1016/j.parkreldis.2012.08.011
66. Duarte JV, Faustino R, Lobo M, Cunha G, Nunes C, Ferreira C, et al. Parametric fMRI of paced motor responses uncovers novel whole-brain imaging biomarkers in spinocerebellar ataxia type 3. Hum Brain Mapp. (2016) 37:3656–68. doi: 10.1002/hbm.23266
67. Stefanescu MR, Dohnalek M, Maderwald S, Thurling M, Minnerop M, Beck A, et al. Structural and functional MRI abnormalities of cerebellar cortex and nuclei in SCA3, SCA6 and Friedreich's ataxia. Brain. (2015) 138(Pt 5):1182–97. doi: 10.1093/brain/awv064
68. Falcon MI, Gomez CM, Chen EE, Shereen A, Solodkin A. Early cerebellar network shifting in spinocerebellar ataxia type 6. Cereb Cortex. (2016) 26:3205–18. doi: 10.1093/cercor/bhv154
69. Mormina E, Petracca M, Bommarito G, Piaggio N, Cocozza S, Inglese M. Cerebellum and neurodegenerative diseases: beyond conventional magnetic resonance imaging. World J Radiol. (2017) 9:371–88. doi: 10.4329/wjr.v9.i10.371
70. Lin YC, Lee YC, Hsu TY, Liao YC, Soong BW. Comparable progression of spinocerebellar ataxias between caucasians and Chinese. Parkinsonism Relat Disord. (2019) 62:156–62. doi: 10.1016/j.parkreldis.2018.12.023
71. Hashimoto Y, Honda T, Matsumura K, Nakao M, Soga K, Katano K, et al. Quantitative evaluation of human cerebellum-dependent motor learning through prism adaptation of hand-reaching movement. PLoS ONE. (2015) 10:e0119376. doi: 10.1371/journal.pone.0119376
72. Honda T, Nagao S, Hashimoto Y, Ishikawa K, Yokota T, Mizusawa H, et al. Tandem internal models execute motor learning in the cerebellum. Proc Natl Acad Sci USA. (2018) 115:7428–33. doi: 10.1073/pnas.1716489115
73. Pro JD, Smith CH, Sumi SM. Presenile Alzheimer disease: amyloid plaques in the cerebellum. Neurology. (1980) 30:820–5. doi: 10.1212/WNL.30.8.820
74. Larner AJ. The cerebellum in Alzheimer's disease. Dement Geriatr Cogn Disord. (1997) 8:203–9. doi: 10.1159/000106632
75. Canu E, Frisoni GB, Agosta F, Pievani M, Bonetti M, Filippi M. Early and late onset Alzheimer's disease patients have distinct patterns of white matter damage. Neurobiol Aging. (2012) 33:1023–33. doi: 10.1016/j.neurobiolaging.2010.09.021
76. Wang Z, Yan C, Zhao C, Qi Z, Zhou W, Lu J, et al. Spatial patterns of intrinsic brain activity in mild cognitive impairment and Alzheimer's disease: a resting-state functional MRI study. Hum Brain Mapp. (2011) 32:1720–40. doi: 10.1002/hbm.21140
77. Mascali D, DiNuzzo M, Gili T, Moraschi M, Fratini M, Maraviglia B, et al. Intrinsic patterns of coupling between correlation and amplitude of low-frequency fMRI fluctuations are disrupted in degenerative dementia mainly due to functional disconnection. PLoS ONE. (2015) 10:e0120988. doi: 10.1371/journal.pone.0120988
78. Castellazzi G, Palesi F, Casali S, Vitali P, Sinforiani E, Wheeler-Kingshott CA, et al. A comprehensive assessment of resting state networks: bidirectional modification of functional integrity in cerebro-cerebellar networks in dementia. Front Neurosci. (2014) 8:223. doi: 10.3389/fnins.2014.00223
79. Fried LP, Tangen CM, Walston J, Newman AB, Hirsch C, Gottdiener J, et al. Frailty in older adults: evidence for a phenotype. J Gerontol A Biol Sci Med Sci. (2001) 56:M146–56. doi: 10.1093/gerona/56.3.M146
80. Liu LK, Chen CH, Lee WJ, Wu YH, Hwang AC, Lin MH, et al. Cognitive frailty and its association with all-cause mortality among community-dwelling older adults in Taiwan: results from I-Lan longitudinal aging study. Rejuvenation Res. (2018) 21:510–7. doi: 10.1089/rej.2017.2038
81. Chen WT, Chou KH, Liu LK, Lee PL, Lee WJ, Chen LK, et al. Reduced cerebellar gray matter is a neural signature of physical frailty. Hum Brain Mapp. (2015) 36:3666–76. doi: 10.1002/hbm.22870
82. Bostan AC, Dum RP, Strick PL. Cerebellar networks with the cerebral cortex and basal ganglia. Trends Cogn Sci. (2013) 17:241–54. doi: 10.1016/j.tics.2013.03.003
83. Pedroso JL, Braga-Neto P, de Souza PV, Barsottini OG. The cerebellum in Parkinson's disease and Parkinsonism in cerebellar disorders. Brain. (2013) 136(Pt 9):e248. doi: 10.1093/brain/awt089
84. Wu T, Hallett M. The cerebellum in Parkinson's disease. Brain. (2013) 136(Pt 3):696–709. doi: 10.1093/brain/aws360
85. Gellersen HM, Guo CC, O'Callaghan C, Tan RH, Sami S, Hornberger M. Cerebellar atrophy in neurodegeneration-a meta-analysis. J Neurol Neurosurg Psychiatry. (2017) 88:780–8. doi: 10.1136/jnnp-2017-315607
86. Rusholt EHL, Salvesen L, Brudek T, Tesfay B, Pakkenberg B, Olesen MV. Pathological changes in the cerebellum of patients with multiple system atrophy and Parkinson's disease - a stereological study. Brain Pathol. (2019). doi: 10.1111/bpa.12806
87. Mormina E, Arrigo A, Calamuneri A, Granata F, Quartarone A, Ghilardi MF, et al. Diffusion tensor imaging parameters' changes of cerebellar hemispheres in Parkinson's disease. Neuroradiology. (2015) 57:327–34. doi: 10.1007/s00234-014-1473-5
88. Wu T, Long X, Zang Y, Wang L, Hallett M, Li K, et al. Regional homogeneity changes in patients with Parkinson's disease. Hum Brain Mapp. (2009) 30:1502–10. doi: 10.1002/hbm.20622
89. Liu H, Edmiston EK, Fan G, Xu K, Zhao B, Shang X, et al. Altered resting-state functional connectivity of the dentate nucleus in Parkinson's disease. Psychiatry Res. (2013) 211:64–71. doi: 10.1016/j.pscychresns.2012.10.007
90. Mirdamadi JL. Cerebellar role in Parkinson's disease. J Neurophysiol. (2016) 116:917–9. doi: 10.1152/jn.01132.2015
91. Dobson R, Giovannoni G. Multiple sclerosis - a review. Eur J Neurol. (2019) 26:27–40. doi: 10.1111/ene.13819
92. Ormerod IE, Miller DH, McDonald WI, du Boulay EP, Rudge P, Kendall BE, et al. The role of NMR imaging in the assessment of multiple sclerosis and isolated neurological lesions. A quantitative study. Brain. (1987) 110(Pt 6):1579–616. doi: 10.1093/brain/110.6.1579
93. Edwards SG, Gong QY, Liu C, Zvartau ME, Jaspan T, Roberts N, et al. Infratentorial atrophy on magnetic resonance imaging and disability in multiple sclerosis. Brain. (1999) 122(Pt 2):291–301. doi: 10.1093/brain/122.2.291
94. D'Ambrosio A, Pagani E, Riccitelli GC, Colombo B, Rodegher M, Falini A, et al. Cerebellar contribution to motor and cognitive performance in multiple sclerosis: an MRI sub-regional volumetric analysis. Mult Scler. (2017) 23:1194–203. doi: 10.1177/1352458516674567
95. Cocozza S, Pontillo G, Russo C, Russo CV, Costabile T, Pepe A, et al. Cerebellum and cognition in progressive MS patients: functional changes beyond atrophy? J Neurol. (2018) 265:2260–6. doi: 10.1007/s00415-018-8985-6
96. Sbardella E, Upadhyay N, Tona F, Prosperini L, De Giglio L, Petsas N, et al. Dentate nucleus connectivity in adult patients with multiple sclerosis: functional changes at rest and correlation with clinical features. Mult Scler. (2017) 23:546–55. doi: 10.1177/1352458516657438
97. Parmar K, Stadelmann C, Rocca MA, Langdon D, D'Angelo E, D'Souza M, et al. The role of the cerebellum in multiple sclerosis-150 years after Charcot. Neurosci Biobehav Rev. (2018) 89:85–98. doi: 10.1016/j.neubiorev.2018.02.012
98. Hoche F, Guell X, Vangel MG, Sherman JC, Schmahmann JD. The cerebellar cognitive affective/Schmahmann syndrome scale. Brain. (2018) 141:248–70. doi: 10.1093/brain/awx317
99. Slemmer JE, Haasdijk ED, Engel DC, Plesnila N, Weber JT. Aldolase C-positive cerebellar Purkinje cells are resistant to delayed death after cerebral trauma and AMPA-mediated excitotoxicity. Eur J Neurosci. (2007) 26:649–56. doi: 10.1111/j.1460-9568.2007.05708.x
100. Diedrichsen J, King M, Hernandez-Castillo C, Sereno M, Ivry RB. Universal transform or multiple functionality? Understanding the contribution of the human cerebellum across task domains. Neuron. (2019) 102:918–28. doi: 10.1016/j.neuron.2019.04.021
101. Guell X, Gabrieli JDE, Schmahmann JD. Triple representation of language, working memory, social and emotion processing in the cerebellum: convergent evidence from task and seed-based resting-state fMRI analyses in a single large cohort. Neuroimage. (2018) 172:437–49. doi: 10.1016/j.neuroimage.2018.01.082
102. Isope P, Barbour B. Properties of unitary granule cell–>Purkinje cell synapses in adult rat cerebellar slices. J Neurosci. (2002) 22:9668–78. doi: 10.1523/JNEUROSCI.22-22-09668.2002
103. Valera AM, Binda F, Pawlowski SA, Dupont JL, Casella JF, Rothstein JD, et al. Stereotyped spatial patterns of functional synaptic connectivity in the cerebellar cortex. Elife. (2016) 5:e09862. doi: 10.7554/eLife.09862
104. Boillat Y, Bazin PL, O'Brien K, Fartaria MJ, Bonnier G, Krueger G, et al. Surface-based characteristics of the cerebellar cortex visualized with ultra-high field MRI. Neuroimage. (2018) 172:1–8. doi: 10.1016/j.neuroimage.2018.01.016
105. Solodkin A, Peri E, Chen EE, Ben-Jacob E, Gomez CM. Loss of intrinsic organization of cerebellar networks in spinocerebellar ataxia type 1: correlates with disease severity and duration. Cerebellum. (2011) 10:218–32. doi: 10.1007/s12311-010-0214-5
106. Schmahmann JD. An emerging concept. The cerebellar contribution to higher function. Arch Neurol. (1991) 48:1178–87. doi: 10.1001/archneur.1991.00530230086029
107. Guell X, Gabrieli JDE, Schmahmann JD. Embodied cognition and the cerebellum: perspectives from the dysmetria of thought and the universal cerebellar transform theories. Cortex. (2018) 100:140–8. doi: 10.1016/j.cortex.2017.07.005
Keywords: zebrin, cerebrocerebellar circuits, neuroimaging, cerebellar disorders, functional topography
Citation: Lin Y-C, Hsu C-CH, Wang P-N, Lin C-P and Chang L-H (2020) The Relationship Between Zebrin Expression and Cerebellar Functions: Insights From Neuroimaging Studies. Front. Neurol. 11:315. doi: 10.3389/fneur.2020.00315
Received: 13 December 2019; Accepted: 31 March 2020;
Published: 22 April 2020.
Edited by:
Sirio Cocozza, University of Naples Federico II, ItalyReviewed by:
Marcondes C. França Jr., Campinas State University, BrazilSerena Ruggieri, Sapienza University of Rome, Italy
Copyright © 2020 Lin, Hsu, Wang, Lin and Chang. This is an open-access article distributed under the terms of the Creative Commons Attribution License (CC BY). The use, distribution or reproduction in other forums is permitted, provided the original author(s) and the copyright owner(s) are credited and that the original publication in this journal is cited, in accordance with accepted academic practice. No use, distribution or reproduction is permitted which does not comply with these terms.
*Correspondence: Li-Hung Chang, bGlodW5nJiN4MDAwNDA7eW0uZWR1LnR3