- 1Spinal Cord Injury Center, Balgrist University Hospital, University of Zurich, Zurich, Switzerland
- 2Department of Neuroradiology, University Hospital Zurich, Zurich, Switzerland
- 3Department of Neurology, Bern University Hospital (Inselspital), University of Bern, Bern, Switzerland
- 4MR-Center, University Children's Hospital Zurich, Zurich, Switzerland
Background: Spinal cord injury (SCI) and its accompanying changes of brain structure and function have been widely studied and reviewed. Debilitating chronic neuropathic pain (NP) is reported in 53% of SCI patients, and brain changes have been shown to be involved with the presence of this secondary complication. However, there is yet a synthesis of current studies that investigated brain structure, resting connectivity, and metabolite changes that accompanies this condition.
Methods: In this review, a systematic search was performed using Medical Subject Headings heading search terms in PubMed and SCOPUS to gather the appropriate published studies. Neuroimaging studies that investigated supraspinal structural, resting-state connectivity, and metabolite changes in SCI subjects with NP were included. To this end, voxel-based morphometry, diffusion tensor imaging, resting-state functional MRI, magnetic resonance spectroscopy, and PET studies were summarized and reviewed. Further inclusion and exclusion criteria allowed delineation of appropriate studies that included SCI subgroups with and without NP.
Results: A total of 12 studies were eligible for qualitative synthesis. Overall, current studies that investigated NP-associated changes within the SCI cohort show primarily metabolite concentration alterations in sensory-pain processing regions, alongside bidirectional changes of brain structure. Moreover, in comparison to healthy controls, there remains limited evidence of structural and connectivity changes but a range of alterations in metabolite concentrations in SCI subjects with NP.
Conclusions: There is some evidence suggesting that the magnitude and presence of NP following SCI results in both adaptive and maladaptive structural plasticity of sensorimotor regions, alongside altered metabolism of brain areas involved with descending pain modulation, pain perception (i.e., anterior cingulate cortex) and sensory integration (i.e., thalamus). However, based on the fact that only a few studies investigated structural and glucose metabolic changes in chronic SCI subjects with NP, the underlying mechanisms that accompany this condition remains to be further elucidated. Future cross-sectional or longitudinal studies that aim to disentangle NP related to SCI may benefit from stricter constraints in subject cohorts, controlled subgroups, improved pain phenotyping, and implementation of multimodal approaches to discover sensitive biomarkers that profile pain and optimize treatment in SCI subjects with NP.
Introduction
Rationale
Spinal cord injury (SCI) has severe consequences for the individual, commonly causing distinct motor and sensory deficits below the level of lesion, attributed to the damage of the corresponding efferent and afferent neural pathways. Disruption of the somatosensory system after SCI can lead to debilitating chronic neuropathic pain (NP) prevalent in 53% of subjects (1) with a third reporting it to be severe (2). NP is defined by the International Association for the Study of Pain (IASP) as “pain arising as a direct consequence of a lesion or disease affecting the (central) somatosensory system” (3, 4). The pathophysiology of NP involves a complex interaction of neuronal changes, inflammation, glial–neuron interactions, supraspinal and spinal sensitization, and alterations in endogenous pain modulation [see reviews: (5–9)]. Consequences of these mechanistic changes are chronic alterations of nociceptive pathways and pain processing regions within the central nervous system, i.e., spinal cord, brainstem, and the brain. Indeed, current neuroimaging studies investigating chronic pain conditions, i.e., fibromyalgia, complex regional pain syndrome, and chronic low back pain have observed changes in brain structure (10, 11), resting-state connectivity (12–14), and metabolic function (15). Furthermore, gray matter volume in chronic low back pain (16) and connectivity changes in fibromyalgia subjects (17), respectively, were shown to be reversed concurrently following pain treatment (16, 17). Together, these studies suggest that changes of structural and functional plasticity within the brain accompany those who experience chronic pain [see reviews: (18, 19)]. However, in cross-sectional studies alone, determining whether the observed brain differences in chronic pain conditions are pre-existing the pain condition, a marker of chronic pain predisposition, or a direct cause of the pain condition itself remains debatable.
Nevertheless, within the last few years, advanced magnetic resonance imaging (MRI) has provided a valuable tool to investigate the structural and/or functional correlates of NP in the brain (5, 18, 20–22). In this context, four MRI methods have been mainly used:
(1) Structural imaging including voxel-based morphometry (VBM) and voxel-based cortical thickness (VBCT). VBM is an imaging-based analysis method for quantifying changes in gray and white matter volume (GMV and WMV, respectively) (23). VBCT is an applied surface-based metric to study atrophy (24). Both methods can provide complementary information of alterations in density and thickness of brain areas in diseases (25).
(2) Diffusion tensor imaging (DTI) measures the diffusion of water molecules in each image voxel and allows indirect quantification of peripheral and central WM integrity and myelination. In the nervous tissue, physical properties such as neuronal density, axon diameter, fiber bundles, and degree of myelination all affect diffusion metrics (26, 27). Fractional anisotropy (FA) and mean diffusivity (MD) are most commonly used DTI metrics (28–30). FA measures the anisotropy of tissue architecture by the preferential direction of water movement, i.e., water molecules diffuse along the direction of tightly packed axons, whereas MD measures the degree of water diffusion. DTI can also visualize and trace WM tracts indirectly with tractography, which can provide anatomical information on specific fibers of interest (31, 32). Yet, careful interpretation is required as several possible substrates can impact on DTI measures and findings may be heterogenous in different neurological disorders (33).
(3) Functional MRI (fMRI) informs about brain function, measured by alterations in the blood oxygen level-dependent signal (34) or by arterial spin labeling (35) that provides a measure of cerebral blood flow. Under task conditions, the hemodynamic response can primarily reflect the local processing of a brain region (36). fMRI can also measure functional connectivity under resting (rsfMRI) or task conditions. This method utilizes the blood oxygen level-dependent signal to define brain areas that have low-frequency synchronous coactivation or resting-state functional connectivity (rsFC).
(4) Magnetic resonance spectroscopy (MRS) (37) provides in vivo biochemical information including N-acetyl aspartate (NAA), myo-inositol (Ins), creatine (Cr), combined measures of glutamate and glutamine (Glx), glutamate (Glu), choline (Cho), and gamma(γ)-aminobutyric acid (GABA). This method allows the determination of metabolite concentrations of certain brain regions and is valuable to investigate underlying biochemical changes that may complement structural and functional observations in clinical populations (38–40).
Next to MRI-based methods, positron emission tomography (PET) can be used to investigate neurochemical changes, i.e., molecular processes, receptor activity, or density alongside blood flow and glucose metabolism in a variety of clinical applications (41–43).
To date, recent reviews and a meta-analysis have summarized the cortical alterations, alongside metabolite and functional changes after SCI [for reviews and meta-analysis, see (44–47)]. In addition, two reviews summarized the impact of deafferentation and chronic pain upon brain reorganization reported with task-based fMRI in animal and human studies (20, 48). However, possibly due to the heterogeneous nature of SCI, i.e., variable injury level and completeness (American Spinal Cord Injury Association Impairment Scale) (49), injury duration, extent of deafferentation (50, 51), and varied clinical characteristics of NP (52), there is limited understanding of the associated changes of brain structure, rsFC, and metabolism in SCI subjects with and without NP.
Objectives
The overall aim of this systematic review is to determine how current neuroimaging studies have provided understanding of neuroplastic changes that possibly contributes to NP following SCI. A variety of neuroimaging studies with different modalities and parameters have appeared to investigate the changes of brain structure and function that accompany chronic NP following SCI, but a synthesis of the literature is lacking. This review therefore expects to compile and examine the remote brain changes as reported in the current literature in two particular SCI states: (1) SCI without NP and (2) SCI with NP.
Research Questions
Specific research questions include the following:
(1) What are the brain correlates, i.e., structure, functional connectivity, and metabolism that accompany NP after SCI?
(2) What have current neuroimaging results revealed regarding the underlying pathophysiological mechanisms of NP following SCI?
(3) What do future neuroimaging studies need to consider and account for when investigating NP in SCI subjects?
Methods
Study Design and Search Strategy
A search of the online databases PubMeD and SCOPUS was conducted on the 1st July 2019 up to 1st August 2019. No publication year criterion was set. The search was performed using a combination of Medical Subject Headings (MeSH) keywords: “Tetraplegia,” “Quadriplegia,” “Paraplegia,” “Spinal Cord Injury,” “Neuropathic Pain,” “Voxel Based Morphometry,” “Voxel Based Cortical Thickness,” “Diffusion Tensor Imaging,” “Diffusion Magnetic Resonance Imaging,” “DTI,” “Resting-state fMRI,” “rs fMRI,” “Resting-State Functional Connectivity,” “Functional Neuroimaging,” “Functional Magnetic Resonance Imaging,” “fMRI,” “Magnetic Resonance Spectroscopy,” and “Positron-Emission Tomography” to identify neuroimaging studies investigating SCI subjects with and without NP. Specifically, MeSH term combinations involved using logic term “OR” between the SCI terms, i.e., Tetraplegia, Quadriplegia etc. while the term “AND” was added to include the neuroimaging method. To optimize the search for identifying articles that investigated the NP condition, an additional “AND” with “Neuropathic Pain” was placed after the neuroimaging method (see Supplementary Table 1). To miss relevant studies, bibliographies of identified studies were also hand searched. One author (VH) initiated the article search utilizing MeSH term combinations and filtered article abstracts for relevancy after removal of duplicates. This yielded a preselection of 90 articles that were further inspected with the inclusion and exclusion criteria for relevancy. Preselected articles (n = 90) were additionally checked for inclusion and exclusion criteria by two additional authors (MH and LM) (Figure 1).
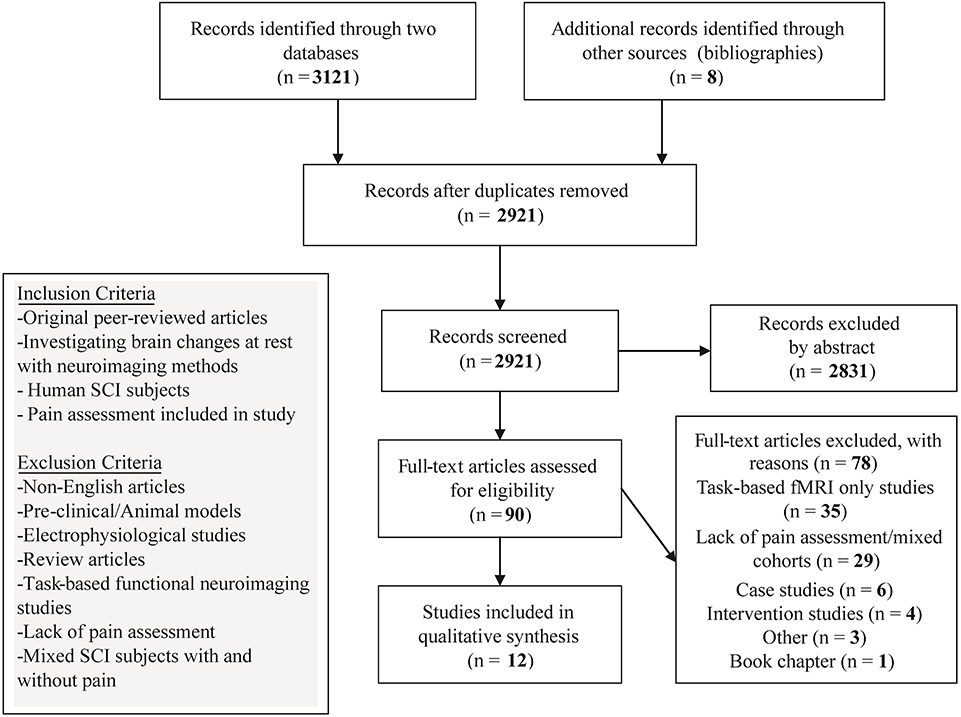
Figure 1. Flow diagrnm of the method followed through the systematic review according to PRISMA standards.
Data Sources, Studies Sections, and Data Extraction
All original studies that investigated supraspinal changes in SCI subgroups with and without NP employing neuroimaging methods were included. Studies that included SCI subjects but lacked pain assessments were excluded. Studies that included pain assessments but did not differentiate SCI subjects into pain subgroups were not included in the qualitative synthesis due to potential confounds in their results. Furthermore, non-English studies, preclinical studies, electrophysiological studies, case studies, intervention studies, review articles, and task-based functional neuroimaging only studies were excluded.
The outcomes extracted and summarized from each study included the following: (1) the modality of the neuroimaging study and subjects' pain assessment implemented in the article; (2) subjects involved, i.e., number, age, chronicity of injury, level of injury, sex, completeness of injury (i.e., Association Impairment Scale A—sensorimotor complete) and presence of NP as assessed by the study's pain characterization (Table 2); (3) whether the study implemented a whole brain and/or a region of interest approach for data analysis; and (4) the main findings of brain alterations from comparisons between healthy controls and/or SCI subgroup (Tables 3–5).
Results
Systematic Search Results
Initial search in the two databases and bibliographies yielded 2,921 articles after the removal of duplicate entries. Screening of abstracts derived 90 articles that were manually screened for relevance. Upon further inspection with the inclusion and exclusion criteria, 35 were excluded due to being task-based only fMRI studies; 29 articles included SCI subjects but were excluded due to lacking pain characterization or including mixed cohorts (SCI with and without NP) for the analysis (see Supplementary Table 2); 4 were intervention studies and 6 single case studies were excluded; 1 was excluded due to being a book chapter; and 3 were excluded due to other reasons (Figure 1, Supplementary Table 2).
As a result, 12 articles were included in the synthesis (Table 1). Four articles included sMRI (one study also included task-based fMRI results that were omitted from the qualitative synthesis); one study also included rsfMRI. One article included sMRI, DTI, and PET. One study included DTI only. One study included rsfMRI only. Five articles included MRS (one study included task-based fMRI results that were omitted from the qualitative synthesis). Article findings are summarized and presented based on the comparison between SCI subgroups to healthy controls (Tables 4, 5) and between the two SCI subgroups (with/without NP) (Table 3). Demographics of SCI subgroups are summarized in Table 2.
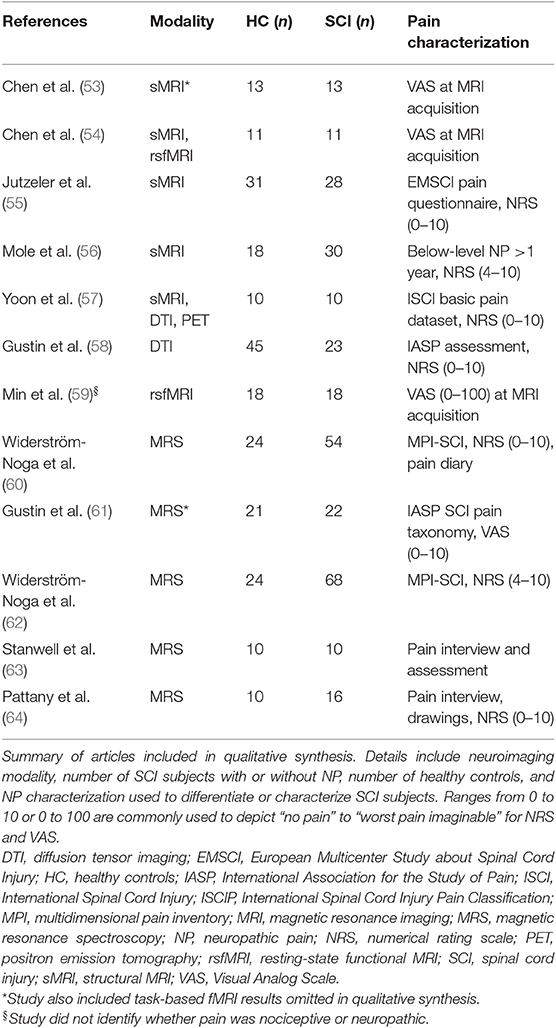
Table 1. Overview of articles investigating brain alterations in SCI subjects without or with NP included in qualitative synthesis.
Pain Assessments Implemented in Included Articles
Within these studies, a range of pain assessments were used to characterize the presence of pain in SCI subjects (Table 1). The presence of NP in SCI subjects were characterized in nine studies with interviews, pain drawings, and questionnaires (55–58, 60–64) (Table 1). Three studies utilized a visual analog scale (VAS) on the day of MRI acquisition to assess pain; NP assessment was not mentioned (53, 54, 59). Seven studies characterized pain intensity with a numerical rating scale (NRS) (55–58, 60–64); two of these studies used NRS on the day of MRS acquisition (60, 64). One study assessed pain intensity but did not report the scale of which they used (63). Three studies characterized pain intensity using a pain diary for a week (with VAS or NRS) before the day of MRI/MRS acquisition (56, 58, 61). Two studies included SCI subjects with NP who had a minimum pain rating of 4 (out of 10) (56, 62).
Neuroimaging Studies Investigating NP Within SCI
Based on the eligible articles, eight studies investigated the impact of NP within SCI subjects (55, 56, 58, 60–64) (Table 2). Two studies did not differentiate their SCI subjects as their whole cohort had some degree of NP (57, 59) (Tables 1, 2).
Volumetric differences between chronic SCI subjects with and without NP were reported in two studies (55, 56). In SCI subjects with NP, one study observed increased GMV of the left anterior cingulate cortex (ACC) and right M1 with decreased GMV of the right S1 and bilateral thalamus (55). This study also showed a positive correlation with pain intensity and GMV of M1 (55). Another study showed decreased GMV of S1 and WMV of S2 in SCI subjects with NP (56) alongside a negative correlation with pain intensity and GMV of S1 (56).
Only one DTI study investigated microstructural differences between chronic SCI subjects with and without NP (58). This study observed decreased MD of the ventral pons to midbrain region, alongside increased MD of prefrontal, premotor and parietal cortices, anterior insula, thalamus, and amygdala in SCI subjects with NP (58). In addition, MD values of the anterior insular, prefrontal, premotor, and parietal cortices were positively correlated with pain intensity, whereas MD values of the amygdala and thalamus were negatively correlated with pain intensity (58).
Four studies investigated metabolite concentration differences between SCI subjects with and without NP (60–64). Two studies observed decreased NAA/Cr and GABA/Cr ratios (61) and decreased absolute Glx and Glx/Ins ratios (62) in the thalamus of SCI subjects with NP. One study reported decreased Glx and Glx/Ins ratios in the ACC of SCI subjects with NP compared to those without NP (62). This study also investigated differences between SCI subjects with differing amounts of NP intensity, observing increased Ins, Cr, and Cho with decreased NAA/Ins and Glx/Ins ratios in the ACC of SCI subjects with greater NP intensity compared to those with a lower NP intensity (62). Another study observed decreased NAA/Ins and Glx/Ins within the thalamus of SCI subjects with greater NP intensity compared to those with a lower NP intensity (60). One study using wavelet-based statistics of MRS data identified mean spectral differences within the prefrontal cortex (PFC) and ACC (but not the thalamus) between SCI subjects with and without NP (63). These differences may be related to a variety of metabolite concentrations, i.e., NAA, GABA, Glu, Ins, and Asp (63) (Table 3).
Neuroimaging Studies Investigating SCI Subjects With NP Compared to Healthy Controls
Eight articles compared SCI subjects with NP to healthy controls (Table 4), showing a variable impact of SCI accompanied by NP upon brain structure, bidirectional microstructural, and connectivity changes and alterations in metabolite concentrations compared to healthy cohorts.
Compared to healthy controls, volumetric changes in SCI subjects with NP were reported in two studies (56, 57); one study did not report this comparison (55). One study with 18 chronic SCI subjects with NP observed no GMV changes but decreased WMV in the bilateral pyramids, medial cuneus, secondary somatosensory cortex (S2), and posterior corona radiata (56).
Another study using multimodal imaging in 10 SCI subjects with NP observed decreased GMV in the medial frontal gyrus, ACC, and anterior insula (57). This study also observed decreased glucose metabolism in the frontal areas (57) and widespread decreases in MD in white matter fibers (i.e., corticospinal tract, thalamocortical tract, and corpus callosum) as well as in frontal regions and sensorimotor areas (57). No changes in FA were observed (57) (Table 4).
Microstructural changes were reported in another study with 12 chronic SCI subjects with NP compared to healthy controls (58). This study observed increased MD in the prefrontal, parietal, and premotor cortices; decreased MD in the thalamus, amygdala, and ventral pons; and no changes in FA (58) (Table 4).
Bidirectional connectivity changes were reported in 18 SCI subjects with a varying amount of pain as identified on the VAS (59). Decreases in rsFC between interhemispheric primary motor cortex (M1), premotor cortex, S1, and S2 were observed alongside increased rsFC of M1 to supplementary motor area and the basal ganglia and S2 (Table 4) (59). Although unclear whether the pain was neuropathic or nociceptive in this study, no correlations between rsFC changes and pain severity were reported (59).
Metabolite changes in the thalamus and ACC between chronic SCI subjects with NP and healthy controls were investigated in four studies (58, 60, 62, 64) (Table 4). Two studies that investigated the thalamus observed decreases in NAA/Ins and NAA/Cr ratios (60, 61), decreased Glx/Ins ratio (60), and GABA/Cr ratio (61). One study investigating SCI subjects with a mild NP showed no significant metabolite alterations in the thalamus compared to healthy controls (60); another study also found no changes in metabolite concentrations in the thalamus (64). One study reports decreased Glx/Ins ratios within the ACC of SCI subjects with more severe NP compared to healthy controls (62).
Neuroimaging Studies Investigating SCI Subjects Without NP Compared to Healthy Controls
Based on the eligible articles, SCI subjects without NP show variable alterations in brain structure and decreased connectivity of frontal and visual areas and metabolite concentrations in the thalamus compared to the healthy condition (53–56, 58, 61, 62, 64) (Table 5).
Three studies reported a range of volumetric changes (54–56). Decreased GMV were observed in the left superior parietal lobule (53), left hippocampus, and superior and middle frontal gyrus (54). Increased GMV of bilateral primary somatosensory cortex (S1), left cuneus (56), and ACC (55) were also observed in SCI subjects without NP compared to healthy controls. Decreased WMV were observed in the right temporal and occipital areas (53) alongside the pyramidal region and posterior corona radiata (56) in SCI subjects without NP. One study did not observe any WMV differences (54).
Microstructural brain changes in SCI subjects without NP was reported in one study (58). Eleven chronic SCI subjects without NP showed decreased FA of fibers connecting the parietal cortex to the midbrain and decreased MD of the premotor and PFC, parietal region, anterior insula, nucleus accumbens, and ventroposterior thalamus (58).
Connectivity changes in acute SCI subjects without pain were reported in one study (54). This study observed decreased amplitude of low-frequency fluctuations in the left orbitofrontal cortex, which correlated negatively with subjects' motor scores alongside decreased intranetwork rsFC of the left middle occipital gyrus in the medial visual network (54) (Table 5).
Metabolite concentrations of SCI subjects without NP were reported in three studies (61, 62, 64). Two studies observed no differences in the ACC or thalamus (61, 62) in SCI subjects without NP compared to controls. In contrast, one study reported increased NAA/Ins ratio and decreased Ins in the thalamus of nine chronic paraplegic SCI subjects without NP compared to controls (64) (Table 5).
Discussion
The primary purpose of this review was to examine the results of current studies that investigated the correlates of chronic NP in brain structure, function, and metabolite changes following SCI. With the adoption of strict exclusion and inclusion criteria in this review (Figure 1, Table 1), current neuroimaging studies report a range of changes in SCI subjects with NP dependent on the comparison to particular cohorts, i.e., to healthy controls or SCI subjects without NP (Tables 3, 4). Some studies report changes in metabolite concentrations within sensory and pain-related regions of SCI subjects with NP compared to those without (Table 3). In addition, a couple of studies report bidirectional volumetric changes, and there remains little evidence of microstructural changes in SCI subjects with NP (Table 3). Moreover, in comparison to healthy controls, there remains limited evidence of volumetric, microstructural, and connectivity changes but a range of alterations in metabolite concentrations in SCI subjects with NP (Table 4). Furthermore, the few studies included here report bidirectional volumetric changes in SCI subjects without NP, and there is limited evidence of microstructure and metabolite alterations in these subjects (Table 4).
NP Following SCI Is Associated With Changes in Brain Metabolites and Structure
Current studies that investigated the influence of NP within SCI show primarily metabolite concentration changes in sensory-pain-related regions (i.e., thalamus and ACC) and bidirectional changes in brain volume and microstructure (Table 3, Figure 2).
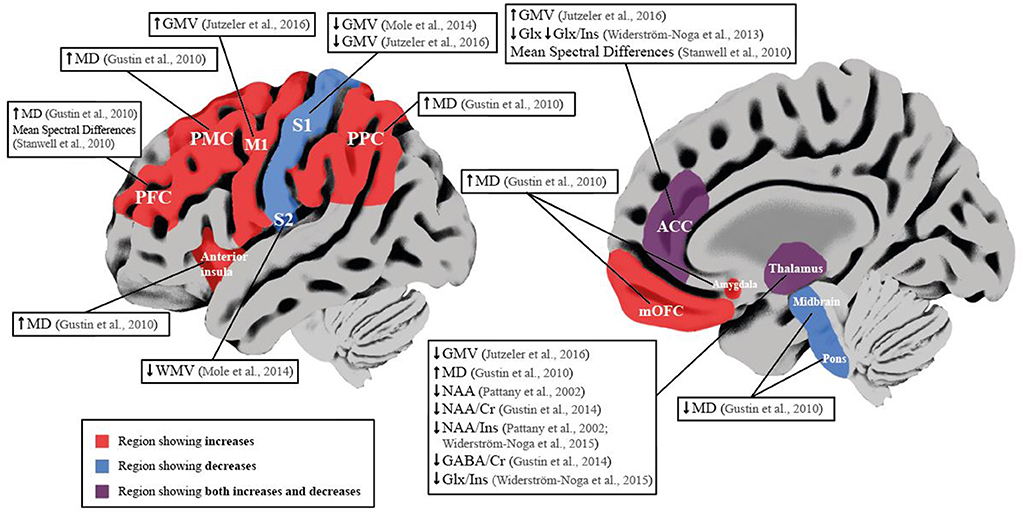
Figure 2. Schematic overview of neuroimaging studies reporting brain differences in SCI subjects with NP compared to SCI subjects without NP. Red brain areas indicate region reported to be affected in articles included in the qualitative synthesis. Labeled boxes describe the type of alteration reported in the brain region. ACC, Anterior Cingulate Cortex; Cr, Creatine; GABA, y-aminobutyric acid; Glx, Glutamate and Glutamine; GMV, Gray Matter Volume; Ins, Myo-inositol; Ml, Prinmy Motor Cortex; MD, Mean Diffusivity; mOFC, Medial Orbitofrontal Cortex; NAA, N-acetyl aspattate; PFC, Prefrontal Cortex; PMC, Premotor Cortex; PPC, Posterior Parietal Cortex; Sl, Primary Somatosensory Cortex; S2, Secondary Somatosensory Cortex; WMV, White Matter Volume. ↓ Significant decrease; ↑ Significant increase.
Within the thalamus of SCI subjects with NP, decreases in NAA ratios to Ins or Cr could be indicative of a loss of neuronal density and dysfunction of inhibitory neurons (61, 64). As decreases in NAA/Cr ratio were positively correlated with the reduction in GABA/Cr ratios, this supports a disruption of normal inhibitory function in SCI subjects with NP compared to those without (61). In addition, Pattany et al., showed negative and positive correlations of NAA and Ins with greater pain intensity, respectively, suggesting neuronal and glial changes and its involvement with pain intensity (64). Moreover, Widerström-Noga et al., reported a decrease in NAA/Ins ratio and Glx/Ins ratio in SCI subjects with intense NP compared to those with mild NP, suggesting a contribution of lower glutamatergic metabolism, increased glial proliferation, or hypertrophy in the thalamus of SCI subjects with more intense NP (60). Similar decreases in NAA/Ins ratio were reported within the ACC in SCI subjects with intense NP compared to those with mild NP (62). This possibly indicates a combination of neuronal dysfunction and gliosis, as absolute concentrations of NAA were not significantly different in SCI subjects with intense NP compared to controls or SCI subjects without NP (62) (Table 3). Furthermore, compared to SCI subjects without NP, SCI subjects with intense NP showed decreased Glx and Glx/Ins ratio, suggesting reduced synaptic activity which may explain the greater amount of emotional distress in this cohort (62), as the ACC is involved in the affective component of pain and descending pain modulation (5, 65–67). To support this view, an earlier study also concluded that mean spectral differences of MRS data within the ACC and PFC delineated SCI subjects with and without NP (63). Structural changes in SCI subjects with NP may be bidirectional, i.e., decreases in GMV in S1 and increases in GMV in M1 both correlate positively with higher pain intensity (55, 56) (Table 3). Decreases in GMV of pain-related regions are often attributed to maladaptive structural plasticity (68–70), whereas increases in GMV reflect preserved structure (also known as “persistent representation”), which correlates to pain intensity (71). In chronic pain conditions, decreases in GMV in sensory-pain-related areas can stem from a variety of factors, i.e., nociceptive input, neurodegeneration, and vascular changes (68, 72, 73). Increases in GMV could be attributed to dendritic branching, neurogenesis, and axonal sprouting (74), and indeed, in subjects with SCI, it has been shown that reorganization of S1 may be attributed to lateral dendritic growth (75). Such bidirectional changes have been previously shown in functional studies in SCI subjects with NP, either reporting increased cortical reorganization (“maladaptive cortical plasticity”) or no change in cortical reorganization (“persistent representation”) correlating positively with higher pain intensity (20, 76). These findings are also reported in functional studies of deafferentated subjects with phantom limb pain (71, 77), and a computational study suggests that these two models are driven by the same underlying mechanism, i.e., abnormal increase in spontaneous activity of deafferented nociceptive pathways (18, 78). It is therefore possible that similar processes occur in SCI subjects with NP reflected in the opposing volumetric changes. One study observed bidirectional microstructural changes in areas involved with spatial cognition, sensory, motor, and affective-motivational functions of SCI subjects with NP (58) (Table 3). Increases in MD were positively correlated with pain intensity in the prefrontal cortex and anterior insula (58), indicating microstructural alterations in regions involved with the cognitive and emotional aspects of pain (58). Decreased MD was positively correlated with pain intensity in the thalamus and amygdala (58), again indicating microstructural changes can be related to pain phenotype. Overall, this may suggest microglial changes that mediate the inflammatory process in SCI subjects with NP as activated microglia are higher in numbers with different phenotypes (larger cell bodies and thicker and shorter processes) (79). It is recognized that activated microglia may contribute to the chronification of NP through hyperexcitability of the sensory neuroaxis and disruption of GABAergic transmission after SCI (80–82).
Brain Changes Accompanying Both SCI and NP
Although the effects of SCI-related NP remain difficult to disentangle without subgroups of SCI subjects without NP, some studies report the associated brain changes in SCI and NP by comparing these subjects directly to healthy controls (Table 4).
A couple of studies investigated volumetric changes between SCI subjects with NP to controls. Mole et al., observed only WMV decreases in regions of projection pathways with no changes in GMV (56), whereas Yoon et al., reported GMV decreases within the medial frontal gyrus, ACC, and anterior insula (57). These limited results may suggest an impact of SCI and NP upon affective-motivational, pain modulatory, and cognitive function, as Yoon et al., also reported decreased glucose metabolism of the frontal areas within the same cohort (57). Furthermore, microstructural alterations were reported in a couple of studies and may indicate bidirectional changes in water diffusion that accompany SCI and NP (57, 58). Decreased MD was observed in multiple brain regions including the thalamus, amygdala, sensorimotor cortices, and brainstem regions of SCI subjects with NP (57, 58) (Table 4). These decreases could be due to changes in underlying cell properties (i.e., shape and density) and increased extracellular matrix (83). In contrast, both increased MD (58) and decreased MD (57) was observed in the frontal and parietal cortices (Table 4), which suggests glial/neuronal alterations or inflammation (i.e., edema) (30, 83, 84) that accompanies regions involved with emotional or spatial cognition following SCI and NP. Both studies did not report any changes in FA, suggesting that axonal integrity may not be compromised in SCI and NP at the supraspinal level (57, 58). Connectivity changes within the sensory and motor cortices were observed in one study of 18 incomplete SCI subjects with a varying degree of pain (59). In particular, rsFCs between motor regions were significantly increased while rsFC decreases were seen between sensory regions (59) (Table 4). These results suggest that the impact of incomplete SCI may result in cortical rsFC changes due to damage to primary afferents, loss of sensory feedback, and recruitment of motor regions to compensate for the motor deficit (59). Although the authors suggested that pain was not a contributing factor to rsFC changes as no correlations were observed with pain intensity (59), the presence of pain itself cannot be fully overlooked. A few studies observed changes in metabolite concentrations in sensory-pain-related regions of chronic SCI subjects with NP, i.e., ACC and thalamus (60–62). Compared to controls, SCI subjects with NP showed decreased NAA, Glx, and GABA ratios within the thalamus (60, 61) (Table 4), suggesting lower glutamatergic metabolism, loss of neuronal density, and dysfunction of inhibitory neurons that accompanies SCI and NP (38, 60, 61). Although one study did not observe changes in thalamus metabolite concentrations (64), this may be due to the small number of SCI subjects with NP (n = 7) (Tables 3, 4). Changes in metabolite concentrations within the ACC were observed in one study; decreased Glx ratio was observed in SCI subjects with a high NP intensity, suggesting decreased glutamatergic activity that may contribute to their affective distress (62). In the same study, SCI subjects with a lower NP intensity did not show changes in ACC metabolite concentrations compared to healthy controls, suggesting that NP intensity may be reflected in this affective-motivational pain-related region (62).
Trauma-Induced Brain Changes Following SCI
Based on the articles included here, there is small evidence of volumetric changes in acute and chronic SCI without the presence of NP, but limited evidence on microstructural and metabolite changes in this condition (Table 5).
In acute and incomplete SCI subjects, Chen et al., observed decreased GMV and WMV of regions involved with spatial cognition, memory function, and visual processing (53, 54), which is additionally highlighted by decreases in amplitudes of low-frequency fluctuations within the orbitofrontal cortex and rsFC of the medial visual network (54). These observations could highlight the initial impairment of SCI upon the subjects' movement ability and related brain changes in visuospatial cognitive processing (53, 54). Interestingly, in chronic SCI subjects, increased GMV in areas processing vision (cuneus), sensory information (S1) (56), and affective-motivational/emotional cognition (ACC) was observed (55), whereas decreases in WMV were observed in pathways of projection fibers associated with motor function, i.e., corticospinal tract (56). These volumetric alterations may reflect the damage and cell atrophy to the sensorimotor system, alongside compensatory mechanisms that could accompany chronic SCI subjects without NP, i.e., visual compensation due to lack of sensory feedback (56) and adaptive mechanisms of sensory-pain-related regions in the absence of NP (55, 56). Indeed, S1 and ACC are involved with the sensory-discriminative (85) and affective-motivational/descending control aspects of pain (65, 66), respectively. Microstructural changes in white matter were reported in one study by Gustin et al., showing decreased FA of parietal cortex to midbrain fibers, alongside decreases in MD in cortices involved with executive, motor, sensory, and emotional function (58) (Table 5). Within these regions, decreased FA could reflect impaired axonal integrity or axonal degeneration, whereas decreased MD could reflect changes in neuronal cells, i.e., proliferation or sprouting (30, 83, 86) that may accompany chronic sensorimotor complete SCI without NP (58). Two out of three studies investigating metabolite concentrations in chronic SCI subjects without NP show non-significant changes in the ACC or thalamus (61, 62). However, only one study reported decreases in absolute Ins within the thalamus, which could indicate alterations of glial cells following SCI (64) as Ins is considered a glial marker (38).
Demographics of SCI Subgroups
In general, recruitment of SCI subjects remains difficult; however, controlling the heterogeneity may limit confounds between studies. As summarized in Table 2, current studies include primarily an older cohort of subjects (majority being male) with a chronic SCI. A recent meta-analysis by Burke et al. (1), which included 1,401 SCI subjects, observed a 53% overall prevalence of NP. Further analysis concluded that NP was found to be more prevalent 1 year postinjury, in people aged 50 or older and tetraplegics (1). However, the prevalence of NP with regard to sex and completeness was not presented due to insufficient data and remains unclear (1). Interestingly, in the studies included here, the presence of NP can be observed in SCI subjects with a range of completeness, level of injury, and pain intensity (Table 2). Three studies were able to include a very homogeneous cohort of SCI subjects, i.e., thoracic level of injury and sensorimotor complete, only delineating the subjects based on NP (58, 61, 63). Another included a restricted criterion of injury level (C5 to T5) although liberal with regard to completeness (56). The remaining studies included an array of injury level and completeness alongside NP (55, 57, 60, 62, 64) (Table 2). As the primary aim of these studies were to observe NP changes in SCI subjects, the sum of the SCI cohort may not allow further differentiation.
Overall, it is unclear in the studies included in this review how the heterogeneity of SCI subjects with NP confounds the brain alterations observed (i.e., structure, function, and metabolite changes). This limiting factor could be explored in future studies within a large cohort allowing comparisons for example between different lesion levels of SCI with NP (thoracic vs. cervical SCI subjects with NP).
Methodological Considerations
Alongside the demographics of SCI subjects within each study, it is important to consider the method implemented, i.e., modality, quantity of cohort, and statistical thresholds. All studies that investigated structural changes with VBM at a whole brain level corrected for multiple comparisons using regions of interests or spheres (i.e., p < 0.05 family-wise error or cluster-level correction; Tables 3–5). Statistical thresholds remain controversial based on factors such as sample size, i.e., a lower threshold (i.e., uncorrected levels) may be more suitable due to a small quantity of subjects; however, the number of false-positive results also increase (87). Utilizing regions of interests as a small volume correction also increases the chance of significant results; hence, regions selected should ideally be in accordance with a priori hypothesis shown in a previous work (88). Furthermore, as the VBM studies included here reported volumetric changes by modulating the structural images, the gray matter density changes that accompany NP following SCI remain to be reported. Interestingly, the two DTI studies included here (57, 58) utilized different toolboxes for data analysis, i.e., FMRIB Software Library (89) and Statistical Parametric Mapping (90), which may yield differing results due to differing preprocessing steps. These two studies also differ in subjects recruited as Yoon et al., did not include a SCI cohort without NP (57) (Table 2); hence, more DTI studies are needed. Another discrepancy between each study is the spatial resolutions of the method, i.e., MRS can only investigate particular regions of interest compared to whole brain MRI (i.e., sMRI, DTI, and rsfMRI). To date, only the ACC and thalamus have been reported with MRS; thus, other pain-related regions, i.e., insula, remain to be explored in future studies.
Taken together, methodological differences in future studies may be observed based on the cohort recruited for the hypotheses, neuroimaging modality, or parameters alongside the toolbox and statistical threshold used for data analysis. Therefore, this field may benefit from open data sources and data sharing between SCI centers, in particular the detailed demographics of SCI subjects, the technical parameters of the particular scanner utilized, and the available dataset (i.e., structural MRI, rsfMRI data).
Limitations
This review is constrained by some important limitations. First, for the purpose of this review, we included a strict criterion (clear information regarding pain) and excluded multiple studies (Supplementary Table 2). Therefore, the overall effects of SCI on the brain may be overlooked. Indeed, previous studies have shown longitudinal structural changes within sensorimotor regions and white matter pathways (91, 92) and microstructural and rsFC changes [see reviews and meta-analysis: (44–47)]. Second, we excluded task-based functional results that may reflect the cortical reorganization due to the presence of NP following SCI, and indeed, the direction of functional reorganization and its correlation to pain can be debated after deafferentation, i.e., in phantom-limb and SCI (20, 21, 71, 76, 77). Third, the papers on supraspinal changes included here were broadly discussed within the neuroimaging method; hence, the underlying interpretations require clarification using a multimodal approach (i.e., neuroimaging, neurophysiology, and clinical assessment) or available animal models. In addition, this review did not discuss studies that investigated pain processing exclusively in the spinal cord, and certainly, this structure is involved in descending pain modulation and pain processing (93–96). Finally, this review did not include a meta-analyses due to the small number of studies alongside the differing methodologies used (Tables 1–5). Instead, an overview of the reported alterations in neuroanatomical landmarks (based on the imaging method) and its correlation with possible biological changes in the SCI condition was reported. This review may also overlook studies that utilized surface-based methods compared to voxel-based approaches.
Conclusion
The aim of this review was to compile the neuroimaging findings that provide evidence of supraspinal alterations after SCI with NP under no task conditions. Overall, there is some evidence suggesting that the magnitude and presence of NP following SCI results in both adaptive and maladaptive structural plasticity of sensorimotor regions, alongside altered metabolism of brain areas involved with descending pain modulation, pain perception (i.e., ACC and PFC), and sensory integration (i.e., thalamus and S1). In SCI subjects with NP, there remains two reproducible findings: decreases in NAA within the thalamus (60, 61, 64) and decreased GMV of the primary somatosensory cortex (55, 56) (Figure 2). However, based on the fact that only a few studies investigated volumetric, microstructural, and glucose metabolic changes in chronic SCI subjects with NP (55–58), the underlying mechanisms that accompany such changes in these cohorts remain to be further elucidated.
Future cross-sectional studies that aim to disentangle NP within the SCI condition may require stricter constraints in subject cohorts with broader characterization of SCI, i.e., integrity of afferent pathways (complete, incomplete) (51), and pain (including NP) as this may confound the results. In addition to standardizing protocols and scanning sequences, an approach to deal with the problem of cohorts heterogeneity might be the advancement of statistical methods to understand the multiple complex interactions of SCI subjects' characteristics and their effects on neuroplasticity. Cumbersome, longitudinal studies will be vital to understand the alterations of supraspinal areas that accompany NP in SCI subjects. Although there are three studies which have investigated SCI subjects longitudinally (91, 97, 98), with one being a training study (97), changes associated with NP still remain unclear. These studies did not subgroup the SCI cohort into a NP/non-NP group (possibly due to low numbers) (91, 98) (Supplementary Table 2). However, one study reported a positive correlation over 6 months with greater NP intensity and increased iron levels (as measured by quantitative MRI) in the right S2, left ACC, and bilateral cerebellum (98), which may further indicates altered pain processing. In contrast, another study was unable to observe any structural changes in the brain (or spinal cord), which were related to NP longitudinally (91). These results remain to be replicated in future longitudinal studies, which may additionally benefit from the utilization of improved pain phenotyping, i.e., pain drawings, questionnaires, etc. (99) alongside tracking the start of pain development. Taken together, such considerations may allow classifications of the clinical, neurophysiological, and neuroimaging subtypes of NP in SCI subjects, provide additional insight to the complex mechanism of NP, and discover sensitive biomarkers that profile pain and optimize treatment.
Author Contributions
MH, LM, and VH designed the study and read and assessed full-texts for eligibility. VH performed the literature search, removed duplicates and with screened the records with LM and MH. VH wrote the first draft of the review. All authors read and revised drafts of manuscripts and approved the final version.
Conflict of Interest
The authors declare that the research was conducted in the absence of any commercial or financial relationships that could be construed as a potential conflict of interest.
Acknowledgments
This study was funded by the Swiss National Science Foundation (320030_169250) and the Swiss Spinal Cord Injury Cohort Study Nested Project Grant (2016-N-005).
Supplementary Material
The Supplementary Material for this article can be found online at: https://www.frontiersin.org/articles/10.3389/fneur.2019.01413/full#supplementary-material
References
1. Burke D, Fullen BM, Stokes D, Lennon O. Neuropathic pain prevalence following spinal cord injury: a systematic review and meta-analysis. Eur J Pain. (2017) 21:29–44. doi: 10.1002/ejp.905
2. Baastrup C, Finnerup NB. Pain in spinal cord injury. Pain Manag. (2012) 2:87–94. doi: 10.2217/pmt.11.70
3. Treede RD, Jensen TS, Campbell JN, Cruccu G, Dostrovsky JO, Griffin JW, et al. Neuropathic pain: redefinition and a grading system for clinical and research purposes. Neurology. (2008) 70:1630–5. doi: 10.1212/01.wnl.0000282763.29778.59
4. IASP. IASP Taxonomy 2012 Update. IASP Taxonomy (2012). Available online at: http://www.iasp-pain.org/Taxonomy?navItemNumber=576
5. Bingel U, Tracey I. Imaging CNS modulation of pain in humans. Physiology. (2008) 23:371–80. doi: 10.1152/physiol.00024.2008
6. Basbaum AI, Bautista DM, Scherrer G, Julius D. Cellular and molecular mechanisms of pain. Cell. (2009) 139:267–84. doi: 10.1016/j.cell.2009.09.028
7. Hulsebosch CE, Hains BC, Crown ED, Carlton SM. Mechanisms of chronic central neuropathic pain after spinal cord injury. Brain Res Rev. (2009) 60:202–13. doi: 10.1016/j.brainresrev.2008.12.010
8. Costigan M, Scholz J, Woolf CJ. Neuropathic pain. Annu Rev Neurosci. (2009) 32:1–32. doi: 10.1146/annurev.neuro.051508.135531
9. Ratté S, Prescott SA. Afferent hyperexcitability in neuropathic pain and the inconvenient truth about its degeneracy. Curr Opin Neurobiol. (2016) 36:31–7. doi: 10.1016/j.conb.2015.08.007
10. Apkarian AV, Sosa Y, Sonty S, Levy RM, Harden RN, Parrish TB, et al. Chronic back pain is associated with decreased prefrontal and thalamic gray matter density. J Neurosci. (2004) 24:10410–5. doi: 10.1523/JNEUROSCI.2541-04.2004
11. Schmidt-Wilcke T, Luerdinga R, Weigand T, Jürgens T, Schuierer G, Leinisch E, et al. Striatal grey matter increase in patients suffering from fibromyalgia–a voxel-based morphometry study. Pain. (2007) 132(Suppl.): S109–16. doi: 10.1016/j.pain.2007.05.010
12. Ichesco E, Schmidt-Wilcke T, Bhavsar R, Clauw DJ, Peltier SJ, Kim J, et al. Altered resting state connectivity of the insular cortex in individuals with fibromyalgia. J Pain. (2014) 15:815–826.e1. doi: 10.1016/j.jpain.2014.04.007
13. Jiang Y, Oathes D, Hush J, Darnall B, Charvat M, Mackey S, et al. Perturbed connectivity of the amygdala and its subregions with the central executive and default mode networks in chronic pain. Pain. (2016) 157:1970–8. doi: 10.1097/j.pain.0000000000000606
14. Kim JH, Choi SH, Jang JH, Lee DH, Lee KJ, Lee WJ, et al. Impaired insula functional connectivity associated with persistent pain perception in patients with complex regional pain syndrome. PLoS ONE. (2017) 12:e0180479. doi: 10.1371/journal.pone.0180479
15. Shiraishi S, Kobayashi H, Nihashi T, Kato K, Iwano S, Nishino M, et al. Cerebral glucose metabolism change in patients with complex regional pain syndrome: a PET study. Radiat Med. (2006) 24:335–44. doi: 10.1007/s11604-006-0035-0
16. Seminowicz DA, Wideman TH, Naso L, Hatami-Khoroushahi Z, Fallatah S, Ware MA, et al. Effective treatment of chronic low back pain in humans reverses abnormal brain anatomy and function. J Neurosci. (2011) 31:7540–50. doi: 10.1523/JNEUROSCI.5280-10.2011
17. Napadow V, Kim J, Clauw DJ, Harris RE. Decreased intrinsic brain connectivity is associated with reduced clinical pain in fibromyalgia. Arthrit Rheumat. (2012) 64:2398–403. doi: 10.1002/art.34412
18. Kuner R, Flor H. Structural plasticity and reorganisation in chronic pain. Nat Rev Neurosci. (2016) 18:20–30. doi: 10.1038/nrn.2016.162
19. Martucci KT, MacKey SC. Neuroimaging of pain: human evidence and clinical relevance of central nervous system processes and modulation. Anesthesiology. (2018) 128:1241–54. doi: 10.1097/ALN.0000000000002137
20. Jutzeler CR, Freund P, Huber E, Curt A, Kramer JLK. Neuropathic pain and functional reorganization in the primary sensorimotor cortex after spinal cord injury. J Pain. (2015) 16:1256–67. doi: 10.1016/j.jpain.2015.08.008
21. Jutzeler CR, Curt A, Kramer JL. Relationship between chronic pain and brain reorganization after deafferentation: a systematic review of functional MRI findings. NeuroImage Clin. (2015) 9:599–606. doi: 10.1016/j.nicl.2015.09.018
22. Makin TR, Scholz J, Henderson Slater D, Johansen-Berg H, Tracey I. Reassessing cortical reorganization in the primary sensorimotor cortex following arm amputation. Brain. (2015) 138:2140–6. doi: 10.1093/brain/awv161
23. Ashburner J, Friston KJ. Voxel-based morphometry—the methods. Neuroimage. (2000) 11:805–21. doi: 10.1006/nimg.2000.0582
24. Dale AM, Fischi B, Sereno MI. Cortical surface-based analysis: I. Segmentation and surface reconstruction. Neuroimage. (1999) 9:179–94. doi: 10.1006/nimg.1998.0395
25. Baron JC, Chételat G, Desgranges B, Perchey G, Landeau B, de la Sayette V, et al. In vivo mapping of gray matter loss with voxel-based morphometry in mild Alzheimer's disease. Neuroimage. (2001) 14:298–309. doi: 10.1006/nimg.2001.0848
26. Beaulieu C. The basis of anisotropic water diffusion in the nervous system - A technical review. NMR Biomed. (2002) 15:435–55. doi: 10.1002/nbm.782
27. Sen PN, Basser PJ. A model for diffusion in white matter in the brain. Biophys J. (2005) 89:2927–38. doi: 10.1529/biophysj.105.063016
28. Alexander AL, Lee JE, Lazar M, Field AS. Diffusion tensor imaging of the brain. Neurotherapeutics. (2007) 4:316–29. doi: 10.1016/j.nurt.2007.05.011
29. Guleria S, Gupta RK, Saksena S, Chandra A, Srivastava RN, Husain M, et al. Retrograde Wallerian degeneration of cranial corticospinal tracts in cervical spinal cord injury patients using diffusion tensor imaging. J Neurosci Res. (2008) 86:2271–80. doi: 10.1002/jnr.21664
30. Pierpaoli C, Jezzard P, Basser PJ, Barnett A, Di Chiro G. Diffusion tensor MR imaging of the human brain. Radiology. (1996) 201:637–48. doi: 10.1148/radiology.201.3.8939209
31. Basser PJ, Pajevic S, Pierpaoli C, Duda J, Aldroubi A. In vivo fiber tractography using DT-MRI data. Magn. Reson. Med. (2000) 44:625–32. doi: 10.1002/1522-2594(200010)44:4<625::aid-mrm17>3.0.co;2-o
32. Catani M, Thiebaut de Schotten M. Diffusion tensor imaging tractography atlas for virtual in vivo dissections. Cortex. (2008) 44:1105–32. doi: 10.1016/j.cortex.2008.05.004
33. Soares JM, Marques P, Alves V, Sousa N. A hitchhiker's guide to diffusion tensor imaging. Front. Neurosci. (2013) 7:31. doi: 10.3389/fnins.2013.00031
34. Ogawa S, Lee TM, Nayak AS, Glynn P. Oxygenation-sensitive contrast in magnetic resonance image of rodent brain at high magnetic fields. Magn Reson Med. (1990) 14:68–78. doi: 10.1002/mrm.1910140108
35. Williams DS, Detre JA, Leigh JS, Koretsky AP. Magnetic resonance imaging of perfusion using spin inversion of arterial water.[erratum appears in Proc Natl Acad Sci U S A 1992 May 1;89(9):4220]. Proc Natl Acad Sci USA. (1992) 89:212–6. doi: 10.1073/pnas.89.1.212
36. Logothetis NK. The underpinnings of the BOLD functional magnetic resonance imaging signal. J Neurosci. (2003) 23:3963–71. doi: 10.1523/JNEUROSCI.23-10-03963.2003
37. Purcell EM, Torrey HC, Pound RV. Resonance absorption by nuclear magnetic moments in a solid. Phys Rev. (1946) 69:37–8. doi: 10.1103/PhysRev.69.37
38. Soares DP, Law M. Magnetic resonance spectroscopy of the brain: review of metabolites and clinical applications. Clin Radiol. (2009) 64:12–21. doi: 10.1016/j.crad.2008.07.002
39. Jackman LM, Sternhell S. Applications of Nuclear Magnetic Resonance Spectroscopy in Organic Chemistry. 2nd Edn. Oxford: Pergamon Press (1969).
40. Gujar S, Maheshwari S, Björkman-Burtscher I, Sundgren P. Magnetic resonance spectroscopy. J Neuroophthalmol. (2005) 25:217–26. doi: 10.1097/01.wno.0000177307.21081.81
41. Shukla AK, Kumar U. Positron emission tomography: an overview. J Med Phys. (2006) 31:13. doi: 10.4103/0971-6203.25665
42. Anand SS, Singh H, Dash AK. Clinical applications of PET and PET-CT. Med J Armed Forces India. (2009) 65:353–8. doi: 10.1016/S0377-1237(09)80099-3
43. Mariani G, Bruselli L, Kuwert T, Kim EE, Flotats A, Israel O, et al. A review on the clinical uses of SPECT/CT. Eur J Nuclear Med Mol Imaging. (2010) 37:1959–85. doi: 10.1007/s00259-010-1390-8
44. Nardone R, Höller Y, Sebastianelli L, Versace V, Saltuari L, Brigo F, et al. Cortical morphometric changes after spinal cord injury. Brain Res Bull. (2018) 137:107–19. doi: 10.1016/j.brainresbull.2017.11.013
45. Dahlberg LS, Becerra L, Borsook D, Linnman C. Brain changes after spinal cord injury, a quantitative meta-analysis and review. Neurosci Biobehav Rev. (2018) 90:272–93. doi: 10.1016/j.neubiorev.2018.04.018
46. Athanasiou A, Klados MA, Pandria N, Foroglou N, Kavazidi KR, Polyzoidis K, et al. A systematic review of investigations into functional brain connectivity following spinal cord injury. Front Hum Neurosci. (2017) 11:517. doi: 10.3389/fnhum.2017.00517
47. Wang W, Xie W, Zhang Q, Liu L, Liu J, Zhou S, et al. Reorganization of the brain in spinal cord injury: a meta-analysis of functional MRI studies. Neuroradiology. (2019) 61:1309–18. doi: 10.1007/s00234-019-02272-3
48. Nardone R, Höller Y, Brigo F, Seidl M, Christova M, Bergmann J, et al. Functional brain reorganization after spinal cord injury: systematic review of animal and human studies. Brain Res. (2013) 1504:58–73. doi: 10.1016/j.brainres.2012.12.034
49. Kirshblum SC, Biering-Sørensen F, Betz R, Burns S, Donovan W, Graves DE, et al. International standards for neurological classification of spinal cord injury: cases with classification challenges. Top Spinal Cord Inj Rehabil. (2014) 20:81–9. doi: 10.1310/sci2002-81
50. Ahuja CS, Wilson JR, Nori S, Kotter MRN, Druschel C, Curt A, et al. Traumatic spinal cord injury. Nat Rev Dis Prim. (2017) 3:17018. doi: 10.1038/nrdp.2017.18
51. Wrigley PJ, Siddall PJ, Gustin SM. New evidence for preserved somatosensory pathways in complete spinal cord injury: a fMRI study. Hum Brain Mapp. (2018) 39:588–98. doi: 10.1002/hbm.23868
52. Widerström-Noga E. Neuropathic pain and spinal cord injury: phenotypes and pharmacological management. Drugs. (2017) 77:967–84. doi: 10.1007/s40265-017-0747-8
53. Chen Q, Zheng W, Chen X, Li X, Wang L, Qin W, et al. Reorganization of the somatosensory pathway after subacute incomplete cervical cord injury. NeuroImage Clin. (2019) 21:101674. doi: 10.1016/j.nicl.2019.101674
54. Chen Q, Zheng W, Chen X, Li X, Wang L, Qin W, et al. Whether visual-related structural and functional changes occur in brain of patients with acute incomplete cervical cord injury: a multimodal based MRI study. Neuroscience. (2018) 393:284–94. doi: 10.1016/j.neuroscience.2018.10.014
55. Jutzeler CR, Huber E, Callaghan MF, Luechinger R, Curt A, Kramer JL, et al. Association of pain and CNS structural changes after spinal cord injury. Sci Rep. (2016) 6:18534. doi: 10.1038/srep18534
56. Mole TB, MacIver K, Sluming V, Ridgway GR, Nurmikko TJ. Specific brain morphometric changes in spinal cord injury with and without neuropathic pain. NeuroImage Clin. (2014) 5:28–35. doi: 10.1016/j.nicl.2014.05.014
57. Yoon EJ, Kim YK, Shin HI, Lee Y, Kim SE. Cortical and white matter alterations in patients with neuropathic pain after spinal cord injury. Brain Res. (2013) 1540:64–73. doi: 10.1016/j.brainres.2013.10.007
58. Gustin SM, Wrigley PJ, Siddall PJ, Henderson LA. Brain anatomy changes associated with persistent neuropathic pain following spinal cord injury. Cereb Cortex. (2010) 20:1409–19. doi: 10.1093/cercor/bhp205
59. Min YS, Chang Y, Park JW, Lee JM, Cha J, Yang JJ, et al. Change of brain functional connectivity in patients with spinal cord injury: graph theory based approach. Ann Rehabil Med. (2015) 39:374–83. doi: 10.5535/arm.2015.39.3.374
60. Widerström-Noga E, Cruz-Almeida Y, Felix ER, Pattany PM. Somatosensory phenotype is associated with thalamic metabolites and pain intensity after spinal cord injury. Pain. (2015) 156:166–74. doi: 10.1016/j.pain.0000000000000019
61. Gustin SM, Wrigley PJ, Youssef AM, McIndoe L, Wilcox SL, Rae CD, et al. Thalamic activity and biochemical changes in individuals with neuropathic pain following spinal cord injury HHS Public Access. Pain. (2014) 155:1027–36. doi: 10.1016/j.pain.2014.02.008
62. Widerström-Noga E, Pattany PM, Cruz-Almeida Y, Felix ER, Perez S, Cardenas DD, et al. Metabolite concentrations in the anterior cingulate cortex predict high neuropathic pain impact after spinal cord injury. Pain. (2013) 154:204–12. doi: 10.1016/j.pain.2012.07.022
63. Stanwell P, Siddall P, Keshava N, Cocuzzo D, Ramadan S, Lin A, et al. Neuro magnetic resonance spectroscopy using wavelet decomposition and statistical testing identifies biochemical changes in people with spinal cord injury and pain. Neuroimage. (2010) 53:544–52. doi: 10.1016/j.neuroimage.2010.06.051
64. Pattany PM, Yezierski RP, Widerström-Noga EG, Bowen BC, Martinez-Arizala A, Garcia BR, et al. Proton magnetic resonance spectroscopy of the thalamus in patients with chronic neuropathic pain after spinal cord injury. Am J Neuroradiol. (2002) 23:901–5.
65. Ossipov MH, Dussor GO, Porreca F. Central modulation of pain. J Clin Invest. (2010) 120:3779–87. doi: 10.1172/JCI43766
66. Ossipov MH, Morimura K, Porreca F. Descending pain modulation and chronification of pain. Curr Opin Supp Palliat Care. (2014) 8:143–51. doi: 10.1097/SPC.0000000000000055
67. Morton DL, Sandhu JS, Jones AK. Brain imaging of pain: state of the art. J Pain Res. (2016) 9:613–24. doi: 10.2147/JPR.S60433
68. May A. Chronic pain may change the structure of the brain. Pain. (2008) 137:7–15. doi: 10.1016/j.pain.2008.02.034
69. Apkarian AV, Bushnell MC, Treede RD, Zubieta JK. Human brain mechanisms of pain perception and regulation in health and disease. Eur J Pain. (2005) 9:463–84. doi: 10.1016/j.ejpain.2004.11.001
70. Pan PL, Zhong JG, Shang HF, Zhu YL, Xiao PR, Dai ZY, et al. Quantitative meta-analysis of grey matter anomalies in neuropathic pain. Eur J Pain. (2015) 19:1224–31. doi: 10.1002/ejp.670
71. Makin TR, Scholz J, Filippini N, Henderson Slater D, Tracey I, Johansen-Berg H. Phantom pain is associated with preserved structure and function in the former hand area. Nat Commun. (2013) 4:1570. doi: 10.1038/ncomms2571
72. Obermann M, Nebel K, Schumann C, Holle D, Gizewski ER, Maschke M, et al. Gray matter changes related to chronic posttraumatic headache. Neurology. (2009) 73:978–83. doi: 10.1212/WNL.0b013e3181b8791a
73. Rodriguez-Raecke R, Niemeier A, Ihle K, Ruether W, May A. Brain gray matter decrease in chronic pain is the consequence and not the cause of pain. J Neurosci. (2009) 29:13746–50. doi: 10.1523/JNEUROSCI.3687-09.2009
74. Zatorre RJ, Fields RD, Johansen-Berg H. Plasticity in gray and white: neuroimaging changes in brain structure during learning. Nat Neurosci. (2012) 15:528–36. doi: 10.1038/nn.3045
75. Henderson LA, Gustin SM, Macey PM, Wrigley PJ, Siddall PJ. Functional reorganization of the brain in humans following spinal cord injury: evidence for underlying changes in cortical anatomy. J Neurosci. (2011) 31:2630–7. doi: 10.1523/JNEUROSCI.2717-10.2011
76. Wrigley PJ, Press SR, Gustin SM, Macefield VG, Gandevia SC, Cousins MJ, et al. Neuropathic pain and primary somatosensory cortex reorganization following spinal cord injury. Pain. (2009) 141:52–9. doi: 10.1016/j.pain.2008.10.007
77. Flor H, Elbert T, Knecht S, Wienbruch C, Pantev C, Birbaumer N, et al. Phantom-limb pain as a perceptual correlate of cortical reorganization following arm amputation. Nature. (1995) 375:482–4. doi: 10.1038/375482a0
78. Boström KJ, de Lussanet MH, Weiss T, Puta C, Wagner H. A computational model unifies apparently contradictory findings concerning phantom pain. Sci Rep. (2014) 4:5298. doi: 10.1038/srep05298
79. Edgar JM, Griffiths IR. White matter structure. A microscopist's view. In: Johansen-Berg H, Behrens TEJ, editors. Diffusion MRI: From Quantitative Measurement to in vivo Neuroanatomy, 2nd Edn. London; Oxford, UK; San Diego, CA: Elsevier (2013). p. 127–153. doi: 10.1016/B978-0-12-396460-1.00007-X
80. Hains BC, Waxman SG. Activated microglia contribute to the maintenance of chronic pain after spinal cord injury. J Neurosci. (2006) 26:4308–17. doi: 10.1523/JNEUROSCI.0003-06.2006
81. Gwak YS, Hulsebosch CE. GABA and central neuropathic pain following spinal cord injury. Neuropharmacology. (2011) 60:799–808. doi: 10.1016/j.neuropharm.2010.12.030
82. Gwak YS, Hulsebosch CE, Leem JW. Neuronal-glial interactions maintain chronic neuropathic pain after spinal cord injury. Neural Plasticity. (2017) 2017:2480689. doi: 10.1155/2017/2480689
83. Pierpaoli C, Righini A, Linfante I, Tao-Cheng JH, Alger JR, Di Chiro G. Histopathologic correlates of abnormal water diffusion in cerebral ischemia: diffusion-weighted MR imaging and light and electron microscopic study. Radiology. (1993) 189:439–48. doi: 10.1148/radiology.189.2.8210373
84. Basser PJ, Pierpaoli C. Microstructural and physiological features of tissues elucidated by quantitative-diffusion-tensor MRI. J Magn Reson. (2011) 213:560–70. doi: 10.1016/j.jmr.2011.09.022
85. Groh A, Krieger P, Mease RA, Henderson L. Acute and chronic pain processing in the thalamocortical system of humans and animal models. Neuroscience. (2018) 387:58–71. doi: 10.1016/j.neuroscience.2017.09.042
86. Pierpaoli C, Barnett A, Pajevic S, Chen R, Penix LR, Virta A, et al. Water diffusion changes in wallerian degeneration and their dependence on white matter architecture. Neuroimage. (2001) 13:1174–85. doi: 10.1006/nimg.2001.0765
87. Henley SM, Ridgway GR, Scahill RI, Klöppel S, Tabrizi SJ, Fox NC, et al. Pitfalls in the use of voxel-based morphometry as a biomarker: examples from Huntington disease. Am J Neuroradiol. (2010) 31:711–9. doi: 10.3174/ajnr.A1939
88. Whitwell JL. Voxel-based morphometry: An automated technique for assessing structural changes in the brain. J Neurosci. (2009) 29:9661–4. doi: 10.1523/JNEUROSCI.2160-09.2009
89. Jenkinson M, Beckmann CF, Behrens TE, Woolrich MW, Smith SM. FSL 1. Neuroimage. (2012) 62:782–90. doi: 10.1016/j.neuroimage.2011.09.015
90. Penny W, Friston K, Ashburner J, Kiebel S, Nichols T. Statistical Parametric Mapping: The Analysis of Functional Brain Images, Statistical Parametric Mapping: The Analysis of Functional Brain Images (2007). doi: 10.1016/B978-0-12-372560-8.X5000-1
91. Grabher P, Callaghan MF, Ashburner J, Weiskopf N, Thompson AJ, Curt A, et al. Tracking sensory system atrophy and outcome prediction in spinal cord injury. Ann Neurol. (2015) 78:751–61. doi: 10.1002/ana.24508
92. Freund P, Weiskopf N, Ashburner J, Wolf K, Sutter R, Altmann DR, et al. MRI investigation of the sensorimotor cortex and the corticospinal tract after acute spinal cord injury: a prospective longitudinal study. Lancet Neurol. (2013) 12:873–81. doi: 10.1016/S1474-4422(13)70146-7
93. Eippert F, Bingel U, Schoell ED, Yacubian J, Klinger R, Lorenz J, et al. Activation of the opioidergic descending pain control system underlies placebo analgesia. Neuron. (2009) 63:533–43. doi: 10.1016/j.neuron.2009.07.014
94. Eippert F, Büchel C. Spinal and supraspinal mechanisms of placebo analgesia. In: Colloca L, Arve Flaten M, Meissner K, editors. Placebo and Pain: From Bench to Bedside. London; Oxford, UK; San Diego, CA: Elsevier (2013). p. 53–71. doi: 10.1016/B978-0-12-397928-5.00007-6
95. Sprenger C, Eippert F, Finsterbusch J, Bingel U, Rose M, Büchel C. Attention modulates spinal cord responses to pain. Curr Biol. (2012) 22:1019–22. doi: 10.1016/j.cub.2012.04.006
96. Youssef AM, Macefield VG, Henderson LA. Pain inhibits pain; human brainstem mechanisms. Neuroimage. (2016) 124:54–62. doi: 10.1016/j.neuroimage.2015.08.060
97. Villiger M, Grabher P, Hepp-Reymond MC, Kiper D, Curt A, Bolliger M, et al. Relationship between structural brainstem and brain plasticity and lower-limb training in spinal cord injury: a longitudinal pilot study. Front Hum Neurosci. (2015) 9:254. doi: 10.3389/fnhum.2015.00254
98. Ziegler G, Grabher P, Thompson A, Altmann D, Hupp M, Ashburner J, et al. Progressive neurodegeneration following spinal cord injury. Neurology. (2018) 90:e1257–66. doi: 10.1212/WNL.0000000000005258
99. Kutch JJ, Ichesco E, Hampson JP, Labus JS, Farmer MA, Martucci KT, et al. Brain signature and functional impact of centralized pain: a Multidisciplinary Approach to the Study of Chronic Pelvic Pain (MAPP) Network Study. Pain. (2017) 158:1979–91. doi: 10.1097/j.pain.0000000000001001
100. Ilvesmäki T, Koskinen E, Brander A, Luoto T, Öhman J, Eskola H. Spinal cord injury induces widespread chronic changes in cerebral white matter. Hum Brain Mapp. (2017) 38:3636–3-47. doi: 10.1002/hbm.23619
101. Koskinen EA, Hakulinen U, Brander AE, Luoto TM, Ylinen A, Ohman JE. Clinical correlates of cerebral diffusion tensor imaging findings in chronic traumatic spinal cord injury. Spinal Cord. (2014) 52:202–8. doi: 10.1038/sc.2013.163
102. Freund P, Schneider T, Nagy Z, Hutton C, Weiskopf N, Friston K, et al. Degeneration of the injured cervical cord is associated with remote changes in corticospinal tract integrity and upper limb impairment. PLoS ONE. (2012) 7:e51729. doi: 10.1371/journal.pone.0051729
103. Chen Q, Zheng W, Chen X, Wan L, Qin W, Qi Z, et al. Brain gray matter atrophy after spinal cord injury: a voxel-based morphometry study. Front Hum Neurosci. (2017) 11:211. doi: 10.3389/fnhum.2017.00211
104. Hawasli AH, Rutlin J, Roland JL, Murphy RKJ, Song SK, Leuthardt EC, et al. Spinal cord injury disrupts resting-state networks in the human brain. J Neurotrauma. (2018) 35:864–73. doi: 10.1089/neu.2017.5212
105. Puri BK, Smith HC, Cox IJ, Sargentoni J, Savic G, Maskill DW, et al. The human motor cortex after incomplete spinal cord injury: an investigation using proton magnetic resonance spectroscopy. J Neurol Neurosurg Psychiatry. (1998) 65:748–54. doi: 10.1136/jnnp.65.5.748
106. Hou JM, Yan RB, Xiang ZM, Zhang H, Liu J, Wu YT, et al. Brain sensorimotor system atrophy during the early stage of spinal cord injury in humans. Neuroscience. (2014) 266:208–15. doi: 10.1016/j.neuroscience.2014.02.013
107. Freund P, Weiskopf N, Ward NS, Hutton C, Gall A, Ciccarelli O, et al. Disability, atrophy and cortical reorganization following spinal cord injury. Brain. (2011) 134:1610–22. doi: 10.1093/brain/awr093
108. Seif M, Curt A, Thompson AJ, Grabher P, Weiskopf N, Freund P. Quantitative MRI of rostral spinal cord and brain regions is predictive of functional recovery in acute spinal cord injury. NeuroImage Clin. (2018) 20:556–63. doi: 10.1016/j.nicl.2018.08.026
109. Hou JM, Sun TS, Xiang ZM, Zhang JZ, Zhang ZC, Zhao M, et al. Alterations of resting-state regional and network-level neural function after acute spinal cord injury. Neuroscience. (2014) 277:446–54. doi: 10.1016/j.neuroscience.2014.07.045
110. Pan Y, Dou WB, Wang YH, Luo HW, Ge YX, Yan SY, et al. Non-concomitant cortical structural and functional alterations in sensorimotor areas following incomplete spinal cord injury. Neural Regen Res. (2017) 12:2059–66. doi: 10.4103/1673-5374.221165
111. Zhu L, Wu G, Zhou X, Li J, Wen Z, Lin F. Altered spontaneous brain activity in patients with acute spinal cord injury revealed by resting-state functional MRI. PLoS ONE. (2015) 10:e0118816. doi: 10.1371/journal.pone.0118816
112. Hou J, Xiang Z, Yan R, Zhao M, Wu Y, Zhong J, et al. Motor recovery at 6 months after admission is related to structural and functional reorganization of the spine and brain in patients with spinal cord injury. Hum Brain Mapp. (2016) 37:2195–209. doi: 10.1002/hbm.23163
113. Freund P, Wheeler-Kingshott CA, Nagy Z, Gorgoraptis N, Weiskopf N, Friston K, et al. Axonal integrity predicts cortical reorganisation following cervical injury. J Neurol Neurosurg Psychiatry. (2012) 83:629–37. doi: 10.1136/jnnp-2011-301875
114. Lundell H, Christensen MS, Barthélemy D, Willerslev-Olsen M, Biering-Sørensen F, Nielsen JB. Cerebral activation is correlated to regional atrophy of the spinal cord and functional motor disability in spinal cord injured individuals. Neuroimage. (2011) 54:1254–61. doi: 10.1016/j.neuroimage.2010.09.009
115. Cunningham EE, Noble JW, Krassioukov A, Boyd LA, Eng JJ. Decreased white matter fractional anisotropy is associated with poorer functional motor skills following spinal cord injury: a pilot study. Spinal Cord. (2018) 57:206–13. doi: 10.1038/s41393-018-0191-y
116. Roelcke U, Curt A, Otte A, Missimer J, Maguire RP, Dietz V, et al. Influence of spinal cord injury on cerebral sensorimotor systems: a PET study. J Neurol Neurosurg Psychiatry. (1997) 62:61–5. doi: 10.1136/jnnp.62.1.61
117. Sun P, Murphy RK, Gamble P, George A, Song SK, Ray WZ. Diffusion assessment of cortical changes, induced by traumatic spinal cord injury. Brain Sci. (2017) 7:21. doi: 10.3390/brainsci7020021
118. Wrigley PJ, Gustin SM, Macey PM, Nash PG, Gandevia SC, Macefield VG, et al. Anatomical changes in human motor cortex and motor pathways following complete thoracic spinal cord injury. Cereb Cortex. (2009) 19:224–32. doi: 10.1093/cercor/bhn072
119. Kaushal M, Oni-Orisan A, Chen G, Li W, Leschke J, Ward BD, et al. Evaluation of whole-brain resting-state functional connectivity in spinal cord injury: a large-scale network analysis using network-based statistic. J Neurotrauma. (2017) 34:1278–82. doi: 10.1089/neu.2016.4649
120. Oni-Orisan A, Kaushal M, Li W, Leschke J, Ward BD, Vedantam A, et al. Alterations in cortical sensorimotor connectivity following complete cervical spinal cord injury: a prospective resting-state fMRI study. PLoS ONE. (2016) 11:e0150351. doi: 10.1371/journal.pone.0150351
121. Jurkiewicz MT, Crawley AP, Verrier MC, Fehlings MG, Mikulis DJ. Somatosensory cortical atrophy after spinal cord injury: a voxel-based morphometry study. Neurology. (2006). 66:762–4. doi: 10.1212/01.wnl.0000201276.28141.40
122. Kaushal M, Oni-Orisan A, Chen G, Li W, Leschke J, Ward D, et al. Large-scale network analysis of whole-brain resting-state functional connectivity in spinal cord injury: a comparative study. Brain Connect. (2017) 7:413–23. doi: 10.1089/brain.2016.0468
123. Guo Y, Gao F, Liu Y, Guo H, Yu W, Chen Z, et al. White matter microstructure alterations in patients with spinal cord injury assessed by diffusion tensor imaging. Front Hum Neurosci. (2019) 13:11. doi: 10.3389/fnhum.2019.00011
124. Crawley AP, Jurkiewicz MT, Yim A, Heyn S, Verrier MC, Fehlings MG, et al. Absence of localized grey matter volume changes in the motor cortex following spinal cord injury. Brain Res. (2004) 1028:19–25. doi: 10.1016/j.brainres.2004.08.060
125. Wei CW, Tharmakulasingam J, Crawley A, Kideckel DM, Mikulis DJ, Bradbury CL, et al. Use of diffusion-tensor imaging in traumatic spinal cord injury to identify concomitant traumatic brain injury. Arch Phys Med Rehabil. (2008) 89(12 Suppl):S85–91. doi: 10.1016/j.apmr.2008.07.005
126. Min YS, Park JW, Jin SU, Jang KE, Nam HU, Lee YS, et al. Alteration of resting-state brain sensorimotor connectivity following spinal cord injury: a resting-state functional magnetic resonance imaging study. J Neurotrauma. (2015) 32:1422–7. doi: 10.1089/neu.2014.3661
127. Karunakaran KD, He J, Zhao J, Cui JL, Zang YF, Zhang Z, et al. Differences in cortical gray matter atrophy of paraplegia and tetraplegia after complete spinal cord injury. J Neurotrauma. (2019) 36:2045–51. doi: 10.1089/neu.2018.6040
Keywords: neuroimaging, spinal cord injury, neuropathic pain, brain plasticity, systematic review
Citation: Huynh V, Rosner J, Curt A, Kollias S, Hubli M and Michels L (2020) Disentangling the Effects of Spinal Cord Injury and Related Neuropathic Pain on Supraspinal Neuroplasticity: A Systematic Review on Neuroimaging. Front. Neurol. 10:1413. doi: 10.3389/fneur.2019.01413
Received: 07 October 2019; Accepted: 27 December 2019;
Published: 05 February 2020.
Edited by:
Francesca Caramia, Sapienza University of Rome, ItalyReviewed by:
Yvonne Höller, Paracelsus Medical University, AustriaTobias Schmidt-Wilcke, Ruhr University Bochum, Germany
Copyright © 2020 Huynh, Rosner, Curt, Kollias, Hubli and Michels. This is an open-access article distributed under the terms of the Creative Commons Attribution License (CC BY). The use, distribution or reproduction in other forums is permitted, provided the original author(s) and the copyright owner(s) are credited and that the original publication in this journal is cited, in accordance with accepted academic practice. No use, distribution or reproduction is permitted which does not comply with these terms.
*Correspondence: Vincent Huynh, dmluY2VudC5odXluaEBiYWxncmlzdC5jaA==
†These authors share last authorship