- 1Graduate Program in Neuroscience and Cell Biology, Institute of Biology, Federal University of Pará, Belém, Brazil
- 2Department of Biochemistry, Institute of Chemistry, Federal University of Rio de Janeiro, Rio de Janeiro, Brazil
- 3Department of Electrical and Biomedical Engineering, Institute of Technology, Federal University of Pará, Belém, Brazil
Humans evolved a symbiotic relationship with their gut microbiome, a complex microbial community composed of bacteria, archaea, protists, and viruses, including bacteriophages. The enteric nervous system (ENS) is a gateway for the bidirectional communication between the brain and the gut, mostly through the vagus nerve (VN). Environmental exposure plays a pivotal role in both the composition and functionality of the gut microbiome and may contribute to susceptibility to neurodegenerative disorders, such as Parkinson's disease (PD). The neuropathological hallmark of PD is the widespread appearance of alpha-synuclein aggregates in both the central and peripheral nervous systems, including the ENS. Many studies suggest that gut toxins can induce the formation of α-syn aggregates in the ENS, which may then be transmitted in a prion-like manner to the CNS through the VN. PD is strongly associated with aging and its negative effects on homeostatic mechanisms protecting from inflammation, oxidative stress, and protein malfunction. In this mini-review, we revisit some landmark discoveries in the field of Parkinson's research and focus on the gut-brain axis. In the process, we highlight evidence showing gut-associated dysbiosis and related microbial-derived components as important players and risk factors for PD. Therefore, the gut microbiome emerges as a potential target for protective measures aiming to prevent PD onset.
Introduction
Parkinson's Disease (PD) is a common neurodegenerative disorder typically associated with the progressive loss of dopaminergic neurons located in the midbrain nucleus substantia nigra pars compacta (SNpc) (1). Although the cardinal symptoms of PD are motor impairments attributed to the depletion of the neurotransmitter dopamine in the striatum, a major target of the SNpc (2), it has been long recognized [for review, see (3)] that other non-motor symptoms, including olfactory (4–6) and gastrointestinal (GI) dysfunction (4), appear during the so-called premotor phase of the disease.
The neuropathological hallmark of PD is the presence of cytoplasmic inclusions, called Lewy bodies (LB) or Lewy neurites (7–9), in SNpc neurons (10). LBs are composed mostly of α-synuclein (α-syn) aggregates (11–13), whose aberrant soluble oligomeric conformations are thought to mediate its toxic effects (14). Alpha-syn is an intrinsically disordered protein (IDP), which lacks a stable 3D structure under physiological conditions and is characterized by exacerbated structural plasticity and conformational adaptability (15). As other IDPs possessing amyloidogenic regions (16), α-syn can turn into a promiscuous binder leading to abnormal interactions and the development of PD (17). Tuttle et al. (18) provided a detailed 3D structure of functional α-syn fibrils (see Figure 1), using solid-state NMR spectroscopy. The study may serve as the basis for a better understanding of molecular mechanisms involved in α-syn fibril nucleation and propagation. In addition, such structural information may provide useful insights on possible interactions of α-synuclein with other proteins and small molecules and allow the emergence of new tools with potential to facilitate both the diagnosis and treatment of PD (e.g., imaging agents and therapeutic drugs).
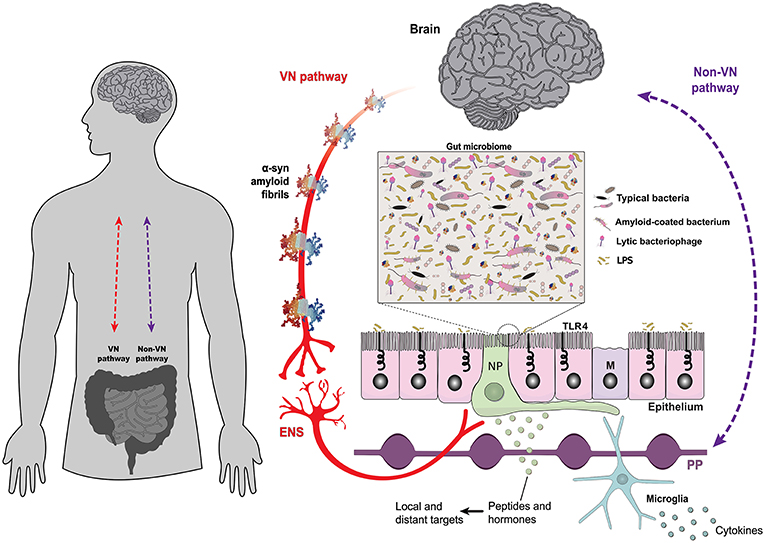
Figure 1. The gut epithelium is a multifunctional interface. The bidirectional interplay between the brain and the gut is mediated by neural, such as the vagus nerve (VN-gateway), and humoral pathways, such as the lymphatic tissue and the bloodstream (Non-VN gateways). A monolayer of epithelial cells separates the intestinal lumen and the complex gut microbiome from the underlying lymphoid and enteric nervous tissues. The structure of alpha-synuclein amyloid fibrils (PDB 2N0A) is based on atomic-resolution molecular data from NGL Viewer (19). Members of the gut microbiome and their extracellular compounds may trigger responses in the VN through enteroendocrine cells, which are contacted by vagus nerve terminals through specialized structures called neuropods (NP) (20). Microbial antigens can cross the gut epithelium through microfold cells, playing a central role in localized inflammatory responses [adapted from Bohórquez et al. (21)]. Toll-like receptors are microbe-sensing proteins, present in intestinal epithelial cells, mediating recognition of commensal bacteria from the harmful/inflammatory ones. ENS, enteric nervous system; M, microfold cells; NP, neuropods; PP, Peyer's patches; TLR4, Toll-like receptor 4; VN, vagus nerve.
Aggregates of α-syn fibrils are also found in neural tissue located outside the central nervous system (CNS) of PD patients, in both the autonomic and enteric nervous system (ENS), an outcome which may be associated with the non-motor symptoms of the disease [for review, see (3)]. These findings led Braak et al. (4) to propose a staging system for the progression of the disease following a specific pattern of α-syn aggregates spreading from peripheral toward more centralized locations in the brain. The triggering event would be the invasion of vulnerable neural structures such as the olfactory epithelium and the ENS, which interface directly with the external environment (5, 22), by a neurotoxicant (“neurotropic virus”) (23). While both structures (24, 25) possess immunological and physical barriers protecting them against environmental insults, these barriers steadily deteriorate with aging [for review, see (26, 27)], which is the biggest risk factor for idiopathic PD (28).
Animal studies have supported the claim that α-syn aggregates propagate in a prion-like manner [(29); for review, see (30)] via microtubule-associated transport along axons (31). In summary, the prion hypothesis of PD proposes that amyloidogenic α-syn would induce a conformational change in the endogenous protein through permissive templating, convert it into a likeness of itself (32, 33) and propagate retrogradely through the vagus nerve or the olfactory tract from the ENS or the olfactory bulb, respectively. Even though definitive proof for this prion hypothesis is still missing (30) and there is the controversial possibility that intestinal α-syn aggregates have a brain origin (34, 35), it has been shown that vagotomy is associated with a decreased risk for PD in humans (36, 37). Also, grafted neurons in PD patients develop α-syn aggregate pathology (38–40) and α-syn from PD patients can cause nigrostriatal degeneration in mice and non-human primates (41). Remarkably, exogenous α-syn fibrils, either PD patient-derived or produced in E. coli, were able to seed the formation of LB-like inclusions which spread from the GI tract to the brain through the vagus nerve in rats (31).
Prior to Braak's hypothesis, however, there was already strong evidence pointing to the role played by exogenous toxins in the etiology of sporadic PD. For instance, postencephalitic parkinsonism (von Economo's disease), which has an autoimmune basis caused by a viral illness (42), is associated with degeneration of the basal ganglia (43). Additionally, the discovery of parkinsonism induced by 1-methyl-4-phenyl-1,2,4,5-tetrahydropyridine (MPTP) through self-administration, in 1982 (44) brought to light a new class of xenobiotic substances that may cause PD-like symptoms by environmental contact. MPTP is a lipophilic compound which readily passes into the brain where it is converted by monoamine oxidase B (MAO-B) to 1-methyl-4-phenylpyridinium (MPP+) (45) which is taken up by dopaminergic cells and impairs mitochondria respiration by poisoning complex 1 (46). There are many heterocyclic molecules that structurally resemble MPTP and are found in the brain from both endogenous and exogenous sources, such as tetrahydroisoquinolines (TIQ) and β-carbolines (β-C). For instance, a TIQ derivative, salsolinol, which is produced by enterobacteria (47) and has been found in the urine of PD patients, may have a double-faced, dose-dependent effect on the nigrostriatal pathway as either a harmful or protective agent (48).
The evidence for the role played by toxins in inducing parkinsonism and the relative scarcity of familial cases (about 10%) (49) underscore the importance of environmental and lifestyle factors over genetic ones in the etiology of the disease (50–52). Some chronic diseases have been associated with a phenomenon called evolutionary mismatch when ancestral traits are no longer adaptive in modern contexts (53, 54). For instance, α-syn is involved with normal synaptic function by regulating, among other things, the size of presynaptic vesicles (55) and the assembly of SNARE proteins involved with the docking of synaptic vesicles to presynaptic membranes (56). However, as old age became common in humans after the early upper Paleolithic (57), the steady increase in longevity seen in modern times may have had a collateral effect on the protein homeostasis (proteostasis) network, which coordinates protein synthesis, folding, trafficking, disaggregation, and degradation (58, 59). The breakdown of proteostasis, which is a common feature of many neurodegenerative diseases (60), means that misfolded proteins may accumulate due to lack of clearance or failure to refold into their native structures (61). In the case of prion-like proteins, this may cause further protein misfolding (template effect) leading to protein aggregation and ultimately cell death (62).
The Gut-Brain Axis and Parkinson's Disease
The gut-brain axis is mediated by intense bidirectional communication between the CNS and the ENS (63). Through the ENS, the gut microbiota influences the development and function of all divisions of the nervous system (64) and this association was established very early during the evolution of multicellular organisms. The first nervous system appeared more than 500 million years ago before the divergence of cnidarians and bilaterians, the two metazoan sister groups (65). That primitive brain had a simple structure, organized as a diffuse nerve net which controlled a restricted set of basic behaviors and was the template for the subsequent evolution of the mammalian ENS (66–68), which retained many of its basic structural characteristics, such as a network of nervous ganglia distributed in the myenteric and submucous plexuses (69). Higher vertebrates went to evolve an additional set of neural structures in the central nervous system (CNS), tasked with the control of more sophisticated behaviors (70). However, the ENS and the CNS maintain intense crosstalk through reciprocal connections mediated by the VN (Figure 1) and pelvic nerve in mammals (71, 72). As the main substrate for this information exchange, the vagus nerve is an attractive target of neurostimulation therapies for the treatment of psychiatric and gastrointestinal disorders (73, 74).
The GI tract harbors a complex microbial ecosystem (Figure 1), consisting of bacteria, archaea, protists, and eukaryotic and prokaryotic viruses, also known as bacteriophages (75–77). The human microbiome has coevolved with its host (78), which keeps a tight leash on the intrinsic competitive nature of the microorganisms that comprise the microbiome, through both the nervous (71, 79, 80) and the immune systems (81, 82). This arrangement maximizes the benefits the host gains from the symbiotic relationship, including protection against pathogens, improved nutrition, and mental health (81). A sub-type of intestinal epithelial cells called enteroendocrine cells, provide a signaling pathway through which the microbiome interacts with the CNS via the vagus nerve (20, 83). Enteroendocrine cells have diverse phenotypes and express a variety of peptides/hormones that can act as signaling molecules on distinct targets, both local and distant, and some are chemoreceptors responding to a variety of luminal stimuli (84, 85). As other intestinal epithelial cells, enteroendocrine cells express toll-like receptors (86), allowing them to detect bacterial products, and activate vagal afferents through basal processes called neuropods (see Figure 1) (20, 87).
The Gut Microbiome and Brain Function
There is increasing evidence of the association between microbiome dysfunction and CNS-related co-morbidities, such as anxiety, depression, autism spectrum disorders, Alzheimer's disease and PD (88–92). This association probably arose as a by-product of natural selection forces acting on microorganisms to adapt to the host and vice-versa (93). The effect of the microbiota on the CNS can lead to behavior modifications (93–95) and even to host manipulation (96) associated with increasing fitness of its bacterial populations. For instance, the microbiome can influence social interactions by acting on the nutritional behavior of individual animals, particularly those from social species where individuals share microbes and interact around foods (97). The proximate neuro-endocrinological and inflammatory mechanisms underlying this type of host manipulation are largely shared by the microbiome and the host (98, 99). For instance, levels of many neurotransmitters that are important for the expression of social behavior, such as serotonin (5-HT), dopamine, norepinephrine (NE), γ-aminobutyric acid (GABA), and glutamate are either expressed or regulated by bacteria (100–102). Particularly, most of the body's serotonin (5-HT) (5-hydroxytryptamine) is produced in the gut by enterochromaffin cells (EC) under the influence of the microbiome (103). The activation of 5-HT4 receptors induces the maturation of the ENS and regulates its adult function (104). In the gut, there are three major metabolic pathways leading from the essential amino acid tryptophan (Trp) to 5-HT, kynurenine (Kyn), and indole derivatives, which are under the direct or indirect control of the microbiota (105). During inflammatory states, most tryptophan is diverted to the production of Kyn and its metabolites kynurenine acid (KYNA) and quinolinic acid (QUIN) (106). While KYNA is considered neuroprotective, QUIN can cause excitotoxicity as an agonist of N-methyl-d-aspartate (NMDA) receptor and contribute to the neuropathogenesis of PD [for review, see (107)].
Although α-syn aggregates are also seen in the ENS of normally aging subjects (108), especially in the appendix (109), it is more prevalent in PD patients (110). Recent in vivo models showed that accumulation of α-syn aggregates in the ENS can be induced by alterations in the gut microbiome (111). Interestingly, Sampson et al. (112) demonstrated in mice, genetically modified to overexpress α-syn, that the presence of gut microbiota is necessary to promote pathological alterations and motor deficits similar to PD. They also demonstrated that fecal transplants from PD patients impair motor function in the same mouse strain, strongly suggesting that gut microbes may play a pivotal role in the onset of synucleinopathies such as PD (112). Underlying these findings is the fact that microbial amyloids produced by some members of the gut microbiota can be released in the extracellular space, where they can be internalized by neighboring cells, including neurons, and seed the formation of pathological aggregates of endogenous α-syn through permissive templating (113, 114). The failure of normal clearance mechanisms such as the ubiquitin-proteasome system, characteristic of both familial and idiopathic PD (115), to degrade the misfolded protein, may facilitate the seeding process.
The concept of microbial dysbiosis also comprises the bacteriophage components of the microbiome (116). Bacteriophages (phages) are viral parasites of bacteria and are important regulators of host-microbiome interactions through horizontal gene transfer and antagonistic coevolution (117, 118). Besides targeting bacteria, phages can impact human health by playing a direct role on intestinal inflammatory processes (119) and possibly causing α-syn misfolding (120). A recent study showed significant differences in the gut phagobiota of PD patients and healthy individuals and a depletion of Lactococcus bacteria (121) in the former, which is associated with the regulation of gut permeability (122) and dopamine production (102), two factors linked with the early signs of PD in the gut (123). Phage therapy has recently returned to the spotlight as an alternative antimicrobial strategy (124, 125). Eventually, it may also contribute to fighting PD through targeted approaches to manipulate the microbiome (121).
Probiotic bacteria have been linked to improved GI symptoms associated with PD (126). Probiotics affect the functionality of the CNS through beneficial interactions with the commensal gut microbiota and modulation of gut-derived inflammation (127). The microbiota of PD patients exhibits a pro-inflammatory profile (128, 129) due to increased intestinal permeability to endotoxins (lipopolysaccharide) (130). Bacterial amyloids may also favor a pro-inflammatory environment in the gut (131). A common bacterial component, the Curli fimbriae, share structural and biophysical properties with amyloids and are produced by E. coli through coordinated biosynthetic processes (132). Other components of the gut microbiome are also known to produce functional extracellular amyloids [e.g., Salmonella, Klebsiella, Citrobacter, and Bacillus species; (133)]. Since probiotic treatment induces an anti-inflammatory peripheral immune response in multiple sclerosis patients (134) there is a possibility they may also be beneficial for PD patients, although there are no reports corroborating this hypothesis. One option is to take advantage of Lactobacilli's ability to inhibit the formation of biofilms by pathogenic bacteria (135, 136). One caveat, however, is that the effects of probiotics are highly variable, being person-specific, as shown in a recent study (137). This limitation may be counteracted with the use of genetically-modified probiotics able to deliver novel therapeutics efficiently and with site specificity (138). Despite the increasing number of probiotic products available to consumers and the aggressive marketing proclaiming their efficacy, there have been few studies addressing concerns about efficacy and, more importantly, the safety of these products (139). There is an urgent need for more studies about the therapeutic potential of specific bacterial strains to help maintain oxidative and protein homeostasis in the ENS.
Concluding Remarks
Aging is the main risk factor for the development of PD (140) and delaying the aging process is neuroprotective to PD in animal models (141). Aging is also associated with the accumulation of neuroinflammatory sequelae and the breakdown of homeostatic mechanisms that protect against protein misfolding, oxidative stress, decreased mitochondrial function, etc. The gut, as one of the main gateways to environmental exposure to the brain, may contribute to increasing the susceptibility to these factors. The microbiome has a protective effect mediating this exposure, and dysbiosis seems to be a pivotal risk factor for PD and other neurological disorders. Thus, the adoption of preventive measures to ensure a healthy microbiome throughout the lifetime can potentially decrease the risk of developing PD and other neurodegenerative diseases. The widespread use of antibiotics, for instance, which can kill gut bacteria indiscriminately, can cause a shift of the microbiome to an alternative stable state with unknown consequences in the long term (142).
Author Contributions
All authors listed have made a substantial, direct and intellectual contribution to the work, and approved it for publication.
Conflict of Interest Statement
The authors declare that the research was conducted in the absence of any commercial or financial relationships that could be construed as a potential conflict of interest.
References
1. Dauer W, Przedborski S. Parkinson's disease: mechanisms and models. Neuron. (2003) 39:889–909. doi: 10.1016/S0896-6273(03)00568-3
2. Hughes AJ, Daniel SE, Kilford L, Lees AJ. Accuracy of clinical diagnosis of idiopathic Parkinson's disease: a clinico-pathological study of 100 cases. J Neurol Neurosurg Psychiatry. (1992) 55:181–4.
3. Garcia-Ruiz PJ, Chaudhuri KR, Martinez-Martin P. Non-motor symptoms of Parkinson's disease A review…from the past. J Neurol Sci. (2014) 338:30–3. doi: 10.1016/j.jns.2014.01.002
4. Braak H, Rüb U, Gai WP, Del Tredici K. Idiopathic Parkinson's disease: possible routes by which vulnerable neuronal types may be subject to neuroinvasion by an unknown pathogen. J Neural Transm. (2003) 110:517–36. doi: 10.1007/s00702-002-0808-2
5. Doty RL, Deems DA, Stellar S. Olfactory dysfunction in parkinsonism A general deficit unrelated to neurologic signs, disease stage, or disease duration. Neurology. (1988) 38:1237–1237.
6. Pearce RK, Hawkes CH, Daniel SE. The anterior olfactory nucleus in Parkinson's disease. Mov Disord. (1995) 10:283–7. doi: 10.1002/mds.870100309
7. Lewy F. Paralysis agitans pathologische anatomie. In: Lewandowski M, editor. Handbuch der Neurologie. Berlin: Springer (1912). p. 920–33.
8. Okazaki H, Lipkin LE, Aronson SM. Diffuse intracytoplasmic ganglionic inclusions (Lewy type) associated with progressive dementia and quadriparesis in flexion. J Neuropathol Exp Neurol. (1961) 20:237–44.
9. Polymeropoulos MH, Lavedan C, Leroy E, Ide SE, Dehejia A, Dutra A, et al. Mutation in the α-synuclein gene identified in families with Parkinson's disease. Science. (1997) 276:2045–7. doi: 10.1126/science.276.5321.2045
10. Gibb WR, Lees AJ. The relevance of the Lewy body to the pathogenesis of idiopathic Parkinson's disease. J Neurol Neurosurg Psychiatry. (1988) 51:745–52. doi: 10.1136/jnnp.51.6.745
11. Goedert M. Alpha-synuclein and neurodegenerative diseases. Nat Rev Neurosci. (2001) 2:492–501. doi: 10.1038/35081564
12. Gründemann J, Schlaudraff F, Haeckel O, Liss B. Elevated α-synuclein mRNA levels in individual UV-laser-microdissected dopaminergic substantia nigra neurons in idiopathic Parkinson's disease. Nucleic Acids Res. (2008) 36:e38. doi: 10.1093/nar/gkn084
13. Spillantini MG, Crowther RA, Jakes R, Hasegawa M, Goedert M. α-Synuclein in filamentous inclusions of Lewy bodies from Parkinson's disease and dementia with Lewy bodies. Proc Natl Acad Sci USA. (1998) 95:6469–73. doi: 10.1073/pnas.95.11.6469
14. Stefanis L. α-Synuclein in Parkinson's disease. Cold Spring Harb Perspect Med. (2012) 2:a009399. doi: 10.1101/cshperspect.a009399
15. Uversky VN, Dunker AK. Understanding protein non-folding. Biochim Biophys Acta BBA Proteins Proteomics. (2010) 1804:1231–64. doi: 10.1016/j.bbapap.2010.01.017
16. Esteras-Chopo A, Serrano L, López de la Paz M. The amyloid stretch hypothesis: recruiting proteins toward the dark side. Proc Natl Acad Sci USA. (2005) 102:16672–7. doi: 10.1073/pnas.0505905102
17. Uversky VN. Wrecked regulation of intrinsically disordered proteins in diseases: pathogenicity of deregulated regulators. Front Mol Biosci. (2014) 1:6. doi: 10.3389/fmolb.2014.00006
18. Tuttle MD, Comellas G, Nieuwkoop AJ, Covell DJ, Berthold DA, Kloepper KD, et al. Solid-state NMR structure of a pathogenic fibril of full-length human α-synuclein. Nat Struct Mol Biol. (2016) 23:409–15. doi: 10.1038/nsmb.3194
19. Rose AS, Bradley AR, Valasatava Y, Duarte JM, Prlic A, Rose PW. NGL viewer: web-based molecular graphics for large complexes. Bioinforma. (2018) 34:3755–8. doi: 10.1093/bioinformatics/bty419
20. Kaelberer MM, Buchanan KL, Klein ME, Barth BB, Montoya MM, Shen X, et al. A gut-brain neural circuit for nutrient sensory transduction. Science. (2018) 361:eaat5236. doi: 10.1126/science.aat5236
21. Bohórquez DV, Shahid RA, Erdmann A, Kreger AM, Wang Y, Calakos N, et al. Neuroepithelial circuit formed by innervation of sensory enteroendocrine cells. J Clin Invest. (2015) 125:782–6. doi: 10.1172/JCI78361
22. Anselmi L, Bove C, Coleman FH, Le K, Subramanian MP, Venkiteswaran K, et al. Ingestion of subthreshold doses of environmental toxins induces ascending Parkinsonism in the rat. NPJ Parkinson Dis. (2018) 4:30. doi: 10.1038/s41531-018-0066-0
23. Hawkes CH, Del Tredici K, Braak H. Parkinson's disease: a dual-hit hypothesis. Neuropathol Appl Neurobiol. (2007) 33:599–614. doi: 10.1111/j.1365-2990.2007.00874.x
24. Gershon MD, Bursztajn S. Properties of the enteric nervous system: limitation of access of intravascular macromolecules to the myenteric plexus and muscularis externa. J Comp Neurol. (1978) 180:467–88. doi: 10.1002/cne.901800305
25. Mellert TK, Getchell ML, Sparks L, Getchell TV. Characterization of the immune barrier in human olfactory mucosa. Otolaryngol Head Neck Surg. (1992) 106:181–8.
26. Attems J, Walker L, Jellinger KA. Olfaction and aging: a mini-review. Gerontology. (2015) 61:485–90. doi: 10.1159/000381619
27. Bowman GL, Dayon L, Kirkland R, Wojcik J, Peyratout G, Severin IC, et al. Blood-brain barrier breakdown, neuroinflammation, and cognitive decline in older adults. Alzheimer Dement. (2018) 14:1640–50. doi: 10.1016/j.jalz.2018.06.2857
28. Reeve A, Simcox E, Turnbull D. Ageing and Parkinson's disease: why is advancing age the biggest risk factor? Ageing Res Rev. (2014) 14:19–30. doi: 10.1016/j.arr.2014.01.004
29. Okuzumi A, Kurosawa M, Hatano T, Takanashi M, Nojiri S, Fukuhara T, et al. Rapid dissemination of alpha-synuclein seeds through neural circuits in an in-vivo prion-like seeding experiment. Acta Neuropathol Commun. (2018) 6:96. doi: 10.1186/s40478-018-0587-0
30. Steiner JA, Quansah E, Brundin P. The concept of alpha-synuclein as a prion-like protein: ten years after. Cell Tissue Res. (2018) 373:161–73. doi: 10.1007/s00441-018-2814-1
31. Holmqvist S, Chutna O, Bousset L, Aldrin-Kirk P, Li W, Björklund T, et al. Direct evidence of Parkinson pathology spread from the gastrointestinal tract to the brain in rats. Acta Neuropathol. (2014) 128:805–20. doi: 10.1007/s00401-014-1343-6
32. Eisenberg D, Jucker M. The amyloid state of proteins in human diseases. Cell. (2012) 148:1188–203. doi: 10.1016/j.cell.2012.02.022
33. Stopschinski BE, Diamond MI. The prion model for progression and diversity of neurodegenerative diseases. Lancet Neurol. (2017) 16:323–32. doi: 10.1016/S1474-4422(17)30037-6
34. Lawson VA, Furness JB, Klemm HM, Pontell L, Chan E, Hill AF, et al. The brain to gut pathway: a possible route of prion transmission. Gut. (2010) 59:1643–51. doi: 10.1136/gut.2010.222620
35. Ulusoy A, Phillips RJ, Helwig M, Klinkenberg M, Powley TL, Di Monte DA. Brain-to-stomach transfer of α-synuclein via vagal preganglionic projections. Acta Neuropathol. (2017) 133:381–93. doi: 10.1007/s00401-016-1661-y
36. Liu B, Fang F, Pedersen NL, Tillander A, Ludvigsson JF, Ekbom A, et al. Vagotomy and Parkinson disease: a Swedish register-based matched-cohort study. Neurology. (2017a) 88:1996–2002. doi: 10.1212/WNL.0000000000003961
37. Svensson E, Horváth-Puhó E, Thomsen RW, Djurhuus JC, Pedersen L, Borghammer P, et al. Vagotomy and subsequent risk of Parkinson's disease. Ann Neurol. (2015) 78:522–9. doi: 10.1002/ana.24448
38. Kordower JH, Chu Y, Hauser RA, Freeman TB, Olanow CW. Lewy body-like pathology in long-term embryonic nigral transplants in Parkinson's disease. Nat Med. (2008a) 14:504–6. doi: 10.1038/nm1747
39. Kordower JH, Chu Y, Hauser RA, Olanow CW, Freeman TB. Transplanted dopaminergic neurons develop PD pathologic changes: a second case report. Mov Disord. (2008b) 23:2303–6. doi: 10.1002/mds.22369
40. Li J-Y, Englund E, Holton JL, Soulet D, Hagell P, Lees AJ, et al. Lewy bodies in grafted neurons in subjects with Parkinson's disease suggest host-to-graft disease propagation. Nat Med. (2008) 14:501–3. doi: 10.1038/nm1746
41. Recasens A, Dehay B, Bové J, Carballo-Carbajal I, Dovero S, Pérez-Villalba A, et al. Lewy body extracts from Parkinson disease brains trigger α-synuclein pathology and neurodegeneration in mice and monkeys. Ann Neurol. (2014) 75:351–62. doi: 10.1002/ana.24066
42. Jang H, Boltz DA, Webster RG, Smeyne RJ. Viral parkinsonism. Biochim Biophys Acta. (2009) 1792:714–21. doi: 10.1016/j.bbadis.2008.08.001
43. von Economo C. Encephalitis lethargica: its sequelae and treatment. JAMA. (1931) 98:255. doi: 10.1001/jama.1932.02730290071039
45. Chiba K, Trevor A, Castagnoli N. Metabolism of the neurotoxic tertiary amine, MPTP, by brain monoamine oxidase. Biochem Biophys Res Commun. (1984) 120:574–8.
46. Javitch JA, D'Amato RJ, Strittmatter SM, Snyder SH. Parkinsonism-inducing neurotoxin, N-methyl-4-phenyl-1,2,3,6 -tetrahydropyridine: uptake of the metabolite N-methyl-4-phenylpyridine by dopamine neurons explains selective toxicity. Proc Natl Acad Sci USA. (1985) 82:2173–7.
47. Villageliú DN, Borts DJ, Lyte M. Production of the neurotoxin salsolinol by a gut-associated bacterium and its modulation by alcohol. Front Microbiol. (2018) 9:3092. doi: 10.3389/fmicb.2018.03092
48. Kurnik-Łucka M, Panula P, Bugajski A, Gil K. Salsolinol: an unintelligible and double-faced molecule—lessons learned from in vivo and in vitro experiments. Neurotox Res. (2018) 33:485–514. doi: 10.1007/s12640-017-9818-6
49. Thomas B, Beal MF. Parkinson's disease. Hum Mol Genet. (2007) 16:R183–94. doi: 10.1093/hmg/ddm159
50. Garcia-Ruiz PJ, Espay AJ. Parkinson disease: an evolutionary perspective. Front Neurol. (2017) 8:157. doi: 10.3389/fneur.2017.00157
51. Jomova K, Vondrakova D, Lawson M, Valko M. Metals, oxidative stress and neurodegenerative disorders. Mol Cell Biochem. (2010) 345:91–104. doi: 10.1007/s11010-010-0563-x
52. Kalinderi K, Bostantjopoulou S, Fidani L. The genetic background of Parkinson's disease: current progress and future prospects. Acta Neurol Scand. (2016) 134:314–26. doi: 10.1111/ane.12563
53. Gluckman PD, Hanson MA. Living with the past: evolution, development, and patterns of disease. Science. (2004) 305:1733–6. doi: 10.1126/science.1095292
54. Tooby J, Cosmides L. The past explains the present: emotional adaptations and the structure of ancestral environments. Ethol Sociobiol. (1990) 11:375–424.
55. Murphy DD, Rueter SM, Trojanowski JQ, Lee VM. Synucleins are developmentally expressed, and alpha-synuclein regulates the size of the presynaptic vesicular pool in primary hippocampal neurons. J Neurosci. (2000) 20:3214–20. doi: 10.1523/jneurosci.20-09-03214.2000
56. Burré J, Sharma M, Tsetsenis T, Buchman V, Etherton MR, Südhof TC. Alpha-synuclein promotes SNARE-complex assembly in vivo and in vitro. Science. (2010) 329:1663–7. doi: 10.1126/science.1195227
57. Caspari R, Lee S-H. Older age becomes common late in human evolution. Proc Natl Acad Sci USA. (2004) 101:10895–900. doi: 10.1073/pnas.0402857101
59. Powers ET, Morimoto RI, Dillin A, Kelly JW, Balch WE. Biological and chemical approaches to diseases of proteostasis deficiency. Annu Rev Biochem. (2009) 78:959–91. doi: 10.1146/annurev.biochem.052308.114844
60. Balch WE, Morimoto RI, Dillin A, Kelly JW. Adapting proteostasis for disease intervention. Science. (2008) 319:916–9. doi: 10.1126/science.1141448
61. Kikis EA, Gidalevitz T, Morimoto RI. Protein homeostasis in models of aging and age-related conformational disease. In: N. Tavernarakis, editor. Protein Metabolism and Homeostasis in Aging Advances in Experimental Medicine and Biology. Boston, MA: Springer (2010). p. 138–59.
62. Bobela W, Aebischer P, Schneider BL. Alpha-synuclein as a mediator in the interplay between aging and Parkinson's disease. Biomolecules. (2015) 5:2675–700. doi: 10.3390/biom5042675
63. Carabotti M, Scirocco A, Maselli MA, Severi C. The gut-brain axis: interactions between enteric microbiota, central and enteric nervous systems. Ann Gastroenterol Q Publ Hell Soc Gastroenterol. (2015) 28:203–9.
64. Diaz Heijtz R, Wang S, Anuar F, Qian Y, Bjorkholm B, Samuelsson A, et al. Normal gut microbiota modulates brain development and behavior. Proc Natl Acad Sci USA. (2011) 108:3047–52. doi: 10.1073/pnas.1010529108
65. Kelava I, Rentzsch F, Technau U. Evolution of eumetazoan nervous systems: insights from cnidarians. Philos Trans R Soc B Biol Sci. (2015) 370:20150065. doi: 10.1098/rstb.2015.0065
66. Furness JB, Stebbing MJ. The first brain: species comparisons and evolutionary implications for the enteric and central nervous systems. Neurogastroenterol Motil. (2018) 30:13234. doi: 10.1111/nmo.13234
67. Koizumi O. Origin and evolution of the nervous system considered from the diffuse nervous system of cnidarians. In: Goffredo S, Dubinsky, Z, editors. The Cnidaria, Past, Present and Future: The World of Medusa and Her Sisters. Cham: Springer International Publishing (2016). p. 73–91.
68. Shimizu H, Koizumi O, Fujisawa T. Three digestive movements in Hydra regulated by the diffuse nerve net in the body column. J Comp Physiol A Neuroethol Sens Neural Behav Physiol. (2004) 190:623–30. doi: 10.1007/s00359-004-0518-3
69. Costa M, Brookes SJH, Hennig GW. Anatomy and physiology of the enteric nervous system. Gut. (2000) 47:iv15–9. doi: 10.1136/gut.47.suppl_4.iv15
70. Kaas J. Evolution of Nervous Systems. 2nd ed. Academic Press (2017). Available online at: https://www.sciencedirect.com/referencework/9780128040966/evolution-of-nervous-systems (accessed April 6, 2019).
71. Powell N, Walker MM, Talley NJ. The mucosal immune system: master regulator of bidirectional gut-brain communications. Nat Rev Gastroenterol Hepatol. (2017) 14:143–59. doi: 10.1038/nrgastro.2016.191
72. Rao M, Gershon MD. The bowel and beyond: the enteric nervous system in neurological disorders. Nat Rev Gastroenterol Hepatol. (2016) 13:517–28. doi: 10.1038/nrgastro.2016.107
73. Breit S, Kupferberg A, Rogler G, Hasler G. Vagus nerve as modulator of the brain–gut axis in psychiatric and inflammatory disorders. Front Psychiatry. (2018) 9:44. doi: 10.3389/fpsyt.2018.00044
74. Johnson RL, Wilson CG. A review of vagus nerve stimulation as a therapeutic intervention. J Inflamm Res. (2018) 11:203–13. doi: 10.2147/JIR.S163248
75. Gerritsen J, Smidt H, Rijkers GT, de Vos WM. Intestinal microbiota in human health and disease: the impact of probiotics. Genes Nutr. (2011) 6:209–40. doi: 10.1007/s12263-011-0229-7
76. Ley RE, Lozupone CA, Hamady M, Knight R, Gordon JI. Worlds within worlds: evolution of the vertebrate gut microbiota. Nat Rev Microbiol. (2008) 6:776–88. doi: 10.1038/nrmicro1978
77. Selber-Hnatiw S, Rukundo B, Ahmadi M, Akoubi H, Al-Bizri H, Aliu AF, et al. Human gut microbiota: toward an ecology of disease. Front Microbiol. (2017) 8:1265. doi: 10.3389/fmicb.2017.01265
78. Moeller AH, Caro-Quintero A, Mjungu D, Georgiev AV, Lonsdorf EV, Muller MN, et al. Cospeciation of gut microbiota with hominids. Science. (2016) 353:380–2. doi: 10.1126/science.aaf3951
79. Bailey MT, Dowd SE, Galley JD, Hufnagle AR, Allen RG, Lyte M. Exposure to a social stressor alters the structure of the intestinal microbiota: implications for stressor-induced immunomodulation. Brain Behav Immun. (2011) 25:397–407. doi: 10.1016/j.bbi.2010.10.023
80. Galley JD, Nelson MC, Yu Z, Dowd SE, Walter J, Kumar PS, et al. Exposure to a social stressor disrupts the community structure of the colonic mucosa-associated microbiota. BMC Microbiol. (2014) 14:189. doi: 10.1186/1471-2180-14-189
81. Foster KR, Schluter J, Coyte KZ, Rakoff-Nahoum S. The evolution of the host microbiome as an ecosystem on a leash. Nature. (2017) 548:43–51. doi: 10.1038/nature23292
82. Zilber-Rosenberg I, Rosenberg E. Role of microorganisms in the evolution of animals and plants: the hologenome theory of evolution. FEMS Microbiol Rev. (2008) 32:723–35. doi: 10.1111/j.1574-6976.2008.00123.x
84. Feher J. 8.3—Intestinal and colonic chemoreception and motility. In: Feher J, editor. Quantitative Human Physiology, 2nd Edn. Boston: Academic Press (2017). p. 796–809.
85. Latorre R, Sternini C, De Giorgio R, Greenwood-Van Meerveld B. Enteroendocrine cells: a review of their role in brain-gut communication. Neurogastroenterol Motil. (2016) 28:620–30. doi: 10.1111/nmo.12754
86. Bogunovic M, Davé SH, Tilstra JS, Chang DTW, Harpaz N, Xiong H, et al. Enteroendocrine cells express functional Toll-like receptors. Am J Physiol Gastrointest Liver Physiol. (2007) 292:G1770–1783. doi: 10.1152/ajpgi.00249.2006
87. Liddle RA. Neuropods. Cell Mol Gastroenterol Hepatol. (2019) 7:739–47. doi: 10.1016/j.jcmgh.2019.01.006
88. Dinan TG, Cryan JF. Gut feelings on ParkinsonŠs and depression. Cerebrum. (2017) 2017:cer-04-17.
89. Hsiao EY, McBride SW, Hsien S, Sharon G, Hyde ER, McCue T, et al. Microbiota modulate behavioral and physiological abnormalities associated with neurodevelopmental disorders. Cell. (2013) 155:1451–63. doi: 10.1016/j.cell.2013.11.024
90. Kelly JR, Minuto C, Cryan JF, Clarke G, Dinan TG. Cross talk: the microbiota and neurodevelopmental disorders. Front Neurosci. (2017) 11:490. doi: 10.3389/fnins.2017.00490
91. Pellegrini C, Antonioli L, Colucci R, Blandizzi C, Fornai M. Interplay among gut microbiota, intestinal mucosal barrier and enteric neuro-immune system: a common path to neurodegenerative diseases? Acta Neuropathol. (2018) 136:345–61. doi: 10.1007/s00401-018-1856-5
92. Unger MM, Spiegel J, Dillmann K-U, Grundmann D, Philippeit H, Bürmann J, et al. Short chain fatty acids and gut microbiota differ between patients with Parkinson's disease and age-matched controls. Parkinson Relat Disord. (2016) 32:66–72. doi: 10.1016/j.parkreldis.2016.08.019
93. Johnson KV-A, Foster KR. Why does the microbiome affect behaviour? Nat Rev Microbiol. (2018) 16:647–55. doi: 10.1038/s41579-018-0014-3
94. Akami M, Andongma AA, Zhengzhong C, Nan J, Khaeso K, Jurkevitch E, et al. Intestinal bacteria modulate the foraging behavior of the oriental fruit fly Bactrocera dorsalis (Diptera: Tephritidae). PLoS ONE. (2019) 14:e0210109. doi: 10.1371/journal.pone.0210109
95. Schretter CE, Vielmetter J, Bartos I, Marka Z, Marka S, Argade S, et al. A gut microbial factor modulates locomotor behaviour in Drosophila. Nature. (2018) 563:402. doi: 10.1038/s41586-018-0634-9
96. Lewin-Epstein O, Aharonov R, Hadany L. Microbes can help explain the evolution of host altruism. Nat Commun. (2017) 8:14040. doi: 10.1038/ncomms14040
97. Pasquaretta C, Gómez-Moracho T, Heeb P, Lihoreau M. Exploring interactions between the gut microbiota and social behavior through nutrition. Genes. (2018) 9:E534. doi: 10.3390/genes9110534
98. Freestone P. Communication between bacteria and their hosts. Scientifica. (2013) 2013:361073. doi: 10.1155/2013/361073
99. Mazzoli R, Pessione E. The Neuro-endocrinological Role of Microbial Glutamate and GABA Signaling. Front Microbiol. (2016) 7:1934. doi: 10.3389/fmicb.2016.01934
100. Liu R, Hong J, Xu X, Feng Q, Zhang D, Gu Y, et al. Gut microbiome and serum metabolome alterations in obesity and after weight-loss intervention. Nat Med. (2017b) 23:859–68. doi: 10.1038/nm.4358
101. Strandwitz P, Kim KH, Terekhova D, Liu JK, Sharma A, Levering J, et al. GABA-modulating bacteria of the human gut microbiota. Nat Microbiol. (2019) 4:396. doi: 10.1038/s41564-018-0307-3
102. Vodolazov IR, Dbar SD, Oleskin AV, Stoyanova LG. Exogenous and endogenous neuroactive biogenic amines: studies with Lactococcus lactis subsp. lactis Appl Biochem Microbiol. (2018) 54:603–10. doi: 10.1134/S0003683818060157
103. Yano JM, Yu K, Donaldson GP, Shastri GG, Ann P, Ma L, et al. Indigenous bacteria from the gut microbiota regulate host serotonin biosynthesis. Cell. (2015) 161:264–76. doi: 10.1016/j.cell.2015.02.047
104. Vadder FD, Grasset E, Holm LM, Karsenty G, Macpherson AJ, Olofsson LE, et al. Gut microbiota regulates maturation of the adult enteric nervous system via enteric serotonin networks. Proc Natl Acad Sci USA. (2018) 115:6458–63. doi: 10.1073/pnas.1720017115
105. Agus A, Planchais J, Sokol H. Gut microbiota regulation of tryptophan metabolism in health and disease. Cell Host Microbe. (2018) 23:716–24. doi: 10.1016/j.chom.2018.05.003
106. Keszthelyi D, Troost FJ, Jonkers DM, van Donkelaar EL, Dekker J, Buurman WA, et al. Does acute tryptophan depletion affect peripheral serotonin metabolism in the intestine? Am J Clin Nutr. (2012) 95:603–8. doi: 10.3945/ajcn.111.028589
107. Lim CK, Fernández-Gomez FJ, Braidy N, Estrada C, Costa C, Costa S, et al. Involvement of the kynurenine pathway in the pathogenesis of Parkinson's disease. Prog Neurobiol. (2017) 155:76–95. doi: 10.1016/j.pneurobio.2015.12.009
108. Böttner M, Zorenkov D, Hellwig I, Barrenschee M, Harde J, Fricke T, et al. Expression pattern and localization of alpha-synuclein in the human enteric nervous system. Neurobiol Dis. (2012) 48:474–80. doi: 10.1016/j.nbd.2012.07.018
109. Gray MT, Munoz DG, Gray DA, Schlossmacher MG, Woulfe JM. Alpha-synuclein in the appendiceal mucosa of neurologically intact subjects. Mov Disord. (2014) 29:991–8. doi: 10.1002/mds.25779
110. Barrenschee M, Zorenkov D, Böttner M, Lange C, Cossais F, Scharf AB, et al. Distinct pattern of enteric phospho-alpha-synuclein aggregates and gene expression profiles in patients with Parkinson's disease. Acta Neuropathol Commun. (2017) 5:1. doi: 10.1186/s40478-016-0408-2
111. Chen SG, Stribinskis V, Rane MJ, Demuth DR, Gozal E, Roberts AM, et al. Exposure to the functional bacterial amyloid protein curli enhances alpha-synuclein aggregation in aged fischer 344 rats and Caenorhabditis elegans. Sci Rep. (2016) 6:34477. doi: 10.1038/srep34477
112. Sampson TR, Debelius JW, Thron T, Janssen S, Shastri GG, Ilhan ZE, et al. Gut microbiota regulate motor deficits and neuroinflammation in a model of Parkinson's disease. Cell. (2016) 167:1469–80.e12. doi: 10.1016/j.cell.2016.11.018
113. Friedland RP, Chapman MR. The role of microbial amyloid in neurodegeneration. PLoS Pathog. (2017) 13:e1006654. doi: 10.1371/journal.ppat.1006654
114. Soto C, Pritzkow S. Protein misfolding, aggregation, and conformational strains in neurodegenerative diseases. Nat Neurosci. (2018) 21:1332–40. doi: 10.1038/s41593-018-0235-9
115. McNaught KSP, Olanow CW, Halliwell B, Isacson O, Jenner P. Failure of the ubiquitin–proteasome system in Parkinson's disease. Nat Rev Neurosci. (2001) 2:589–94. doi: 10.1038/35086067
116. Manrique P, Dills M, Young MJ. The human gut phage community and its implications for health and disease. Viruses. (2017) 9:E141. doi: 10.3390/v9060141
117. Duerkop BA. Bacteriophages shift the focus of the mammalian microbiota. PLoS Pathog. (2018) 14:e1007310. doi: 10.1371/journal.ppat.1007310
118. Scanlan PD. Bacteria–bacteriophage coevolution in the human gut: implications for microbial diversity and functionality. Trends Microbiol. (2017) 25:614–23. doi: 10.1016/j.tim.2017.02.012
119. Gogokhia L, Buhrke K, Bell R, Hoffman B, Brown DG, Hanke-Gogokhia C, et al. Expansion of bacteriophages is linked to aggravated intestinal inflammation and colitis. Cell Host Microbe. (2019) 25:285–99.e8. doi: 10.1016/j.chom.2019.01.008
120. Tetz G, Tetz V, Tetz G, Tetz V. Bacteriophages as new human viral pathogens. Microorganisms. (2018b) 6:54. doi: 10.3390/microorganisms6020054
121. Tetz G, Brown SM, Hao Y, Tetz V. Parkinson's disease and bacteriophages as its overlooked contributors. Sci Rep. (2018a) 8:10812. doi: 10.1038/s41598-018-29173-4
122. Darby TM, Owens JA, Saeedi BJ, Luo L, Matthews JD, Robinson BS, et al. Lactococcus lactis subsp. cremoris is an efficacious beneficial bacterium that limits tissue injury in the intestine iScience. (2019) 12:356–67. doi: 10.1016/j.isci.2019.01.030
123. Houser MC, Tansey MG. The gut-brain axis: is intestinal inflammation a silent driver of Parkinson's disease pathogenesis? NPJ Parkinson Dis. (2017) 3:3. doi: 10.1038/s41531-016-0002-0
124. Chan BK, Abedon ST, Loc-Carrillo C. Phage cocktails and the future of phage therapy. Future Microbiol. (2013) 8:769–83. doi: 10.2217/fmb.13.47
125. Górski A, Miedzybrodzki R, Weber-Dabrowska B, Fortuna W, Letkiewicz S, Rogóz P, et al. Phage therapy: combating infections with potential for evolving from merely a treatment for complications to targeting diseases. Front Microbiol. (2016) 7:1515. doi: 10.3389/fmicb.2016.01515
126. Barichella M, Pacchetti C, Bolliri C, Cassani E, Iorio L, Pusani C, et al. Probiotics and prebiotic fiber for constipation associated with Parkinson disease: an RCT. Neurology. (2016) 87:1274–80. doi: 10.1212/WNL.0000000000003127
127. Wang H, Lee I-S, Braun C, Enck P. Effect of probiotics on central nervous system functions in animals and humans: a systematic review. J Neurogastroenterol Motil. (2016) 22:589–605. doi: 10.5056/jnm16018
128. Bedarf JR, Hildebrand F, Coelho LP, Sunagawa S, Bahram M, Goeser F, et al. Functional implications of microbial and viral gut metagenome changes in early stage L-DOPA-naïve Parkinson's disease patients. Genome Med. (2017) 9:39. doi: 10.1186/s13073-017-0428-y
129. Petrov VA, Saltykova IV, Zhukova IA, Alifirova VM, Zhukova NG, Dorofeeva YB, et al. Analysis of gut microbiota in patients with Parkinson's disease. Bull Exp Biol Med. (2017) 162:734–7. doi: 10.1007/s10517-017-3700-7
130. Forsyth CB, Shannon KM, Kordower JH, Voigt RM, Shaikh M, Jaglin JA, et al. Increased intestinal permeability correlates with sigmoid mucosa alpha-synuclein staining and endotoxin exposure markers in early Parkinson's disease. PLoS ONE. (2011) 6:e28032. doi: 10.1371/journal.pone.0028032
131. Miraglia F, Colla E. Microbiome, Parkinson's disease and molecular mimicry. Cells. (2019) 8:222. doi: 10.3390/cells8030222
132. Taglialegna A, Lasa I, Valle J. Amyloid Structures as Biofilm Matrix Scaffolds. J Bacteriol. (2016) 198:2579–88. doi: 10.1128/JB.00122-16
133. Van Gerven N, Van der Verren SE, Reiter DM, Remaut H. The role of functional amyloids in bacterial virulence. J Mol Biol. (2018) 430:3657–84. doi: 10.1016/j.jmb.2018.07.010
134. Tankou SK, Regev K, Healy BC, Tjon E, Laghi L, Cox LM, et al. A probiotic modulates the microbiome and immunity in multiple sclerosis. Ann Neurol. (2018) 83:1147–61. doi: 10.1002/ana.25244
135. Söderling EM, Marttinen AM, Haukioja AL. Probiotic lactobacilli interfere with Streptococcus mutans biofilm formation in vitro. Curr Microbiol. (2011) 62:618–22. doi: 10.1007/s00284-010-9752-9
136. Vuotto C, Longo F, Donelli G. Probiotics to counteract biofilm-associated infections: promising and conflicting data. Int J Oral Sci. (2014) 6:189–94. doi: 10.1038/ijos.2014.52
137. Zmora N, Zilberman-Schapira G, Suez J, Mor U, Dori-Bachash M, Bashiardes S, et al. Personalized gut mucosal colonization resistance to empiric probiotics is associated with unique host and microbiome features. Cell. (2018) 174:1388–405. doi: 10.1016/j.cell.2018.08.041
138. Singh B, Mal G, Marotta F. Designer probiotics: paving the way to living therapeutics. Trends Biotechnol. (2017) 35:679–82. doi: 10.1016/j.tibtech.2017.04.001
139. Cohen PA. Probiotic safety—no guarantees. JAMA Intern Med. (2018) 178:1577–8. doi: 10.1001/jamainternmed.2018.5403
140. Driver JA, Logroscino G, Gaziano JM, Kurth T. Incidence and remaining lifetime risk of Parkinson disease in advanced age. Neurology. (2009) 72:432–8. doi: 10.1212/01.wnl.0000341769.50075.bb
141. Cooper JF, Dues DJ, Spielbauer KK, Machiela E, Senchuk MM, Raamsdonk JMV. Delaying aging is neuroprotective in Parkinson's disease: a genetic analysis in C. elegans models NPJ Parkinson Dis. (2015) 1:15022. doi: 10.1038/npjparkd.2015.22
Keywords: Parkinson's disease, enteric nervous system, microbiome, neurotoxicants, probiotics
Citation: Santos SF, de Oliveira HL, Yamada ES, Neves BC and Pereira A Jr (2019) The Gut and Parkinson's Disease—A Bidirectional Pathway. Front. Neurol. 10:574. doi: 10.3389/fneur.2019.00574
Received: 25 January 2019; Accepted: 15 May 2019;
Published: 04 June 2019.
Edited by:
Foteini Christidi, National and Kapodistrian University of Athens, GreeceReviewed by:
Kun Lu, University of North Carolina at Chapel Hill, United StatesDenis Soulet, Laval University, Canada
Copyright © 2019 Santos, de Oliveira, Yamada, Neves and Pereira. This is an open-access article distributed under the terms of the Creative Commons Attribution License (CC BY). The use, distribution or reproduction in other forums is permitted, provided the original author(s) and the copyright owner(s) are credited and that the original publication in this journal is cited, in accordance with accepted academic practice. No use, distribution or reproduction is permitted which does not comply with these terms.
*Correspondence: Antonio Pereira Jr., YXBlcmVpcmEmI3gwMDA0MDt1ZnBhLmJy