- 1Department of Neurology, Amsterdam Neuroscience Institute, Amsterdam University Medical Center, Amsterdam, Netherlands
- 2Department of Neurology, University Medical Center Groningen, University of Groningen, Groningen, Netherlands
- 3Faculty of Brain Sciences, Institute of Neurology, University College London, London, United Kingdom
- 4Department of Neurology, St. George's University of London, London, United Kingdom
Emerging evidence suggests that Parkinson's disease (PD) results from disrupted oscillatory activity in cortico-basal ganglia-thalamo-cortical (CBGTC) and cerebellar networks which can be partially corrected by applying deep brain stimulation (DBS). The inherent dynamic nature of such oscillatory activity might implicate that is represents temporal aspects of motor control. While the timing of muscle activities in CBGTC networks constitute the temporal dimensions of distinct motor acts, these very networks are also involved in somatosensory processing. In this respect, a temporal aspect of somatosensory processing in motor control concerns matching predicted (feedforward) and actual (feedback) sensory consequences of movement which implies a distinct contribution to demarcating the temporal order of events. Emerging evidence shows that such somatosensory processing is altered in movement disorders. This raises the question how disrupted oscillatory activity is related to impaired temporal processing and how/whether DBS can functionally restore this. In this perspective article, the neural underpinnings of temporal processing will be reviewed and translated to the specific alternated oscillatory neural activity specifically found in Parkinson's disease. These findings will be integrated in a neurophysiological framework linking somatosensory and motor processing. Finally, future implications for neuromodulation will be discussed with potential implications for strategy across a range of movement disorders.
Time and the Brain
Purposeful motor behavior requires adequate spatio-temporal co-ordination of limbs and axial body parts. E.g., in catching a ball, joint movements, are arranged in such a way that the hand reaches the target's location at the correct time. These spatial and temporal characteristics of movement are also distinctively represented in the organization underlying cerebral motor control, embedded in both segregated and integrated neuronal circuitries (1, 2). Regarding motor timing, at least two interrelated levels of organization may be discerned:
(i) planning “to be” at the right time at the right place and
(ii) planning the serial order of required muscle contractions in order “to move” effectively. The first highlights engagement with a dynamic environment and underscores the relation between motor and perceptual timing. Given the relative slow cerebral processing time, such environmental engagement requires anticipation on near-future events. With regard to the temporal orchestration of muscle contractions, proprioception makes an additional sensory contribution to motor control, both in feedforward and feedback modes (3).
The dynamics of an environment, i.e., the succession of events, is perceived as a sequence of spatial changes. Segregated analysis of the various features of (visual) stimuli, such as shape, color, and visual motion, along parallel processing streams with varying synaptic delays, implies that the initial stimulus time is dispersed within the brain, thus losing an absolute (external) measure of time (4, 5). Moreover, a consequence of such necessary cerebral processing time is that the flow of external change is fractionated, demarcating intervals between distinct spatial frames. This is consistent with daily-life experience that at certain speed, a moving dot will appear as a line. Apparently, intervals of minimal change define the threshold at which a moving dot is either perceived as a dot or changes into a line. Based on functional imaging results the cerebellum is particularly implicated in assessing differences between past and future spatial frames enabling anticipation on coming events (6, 7), while the striatum plays a specific role in monitoring minimal intervals of successive spatial change, providing an internal measure of non-contextual time, i.e., an internal clock (5, 8, 9). For the latter, parallel streams of cortical processing steps, with the intrinsic consequence of introducing temporal dispersion, efferent copies might be emitted to the striatum and provide sequential regularity at system level (Figure 1). This is consistent with the model of cortical networks that enable “timing” as a result of time-dependent network changes (10). Closely related to this concept, the basal ganglia have been proposed to play a role in the synchronization of multiple cortical oscillators (11, 12).
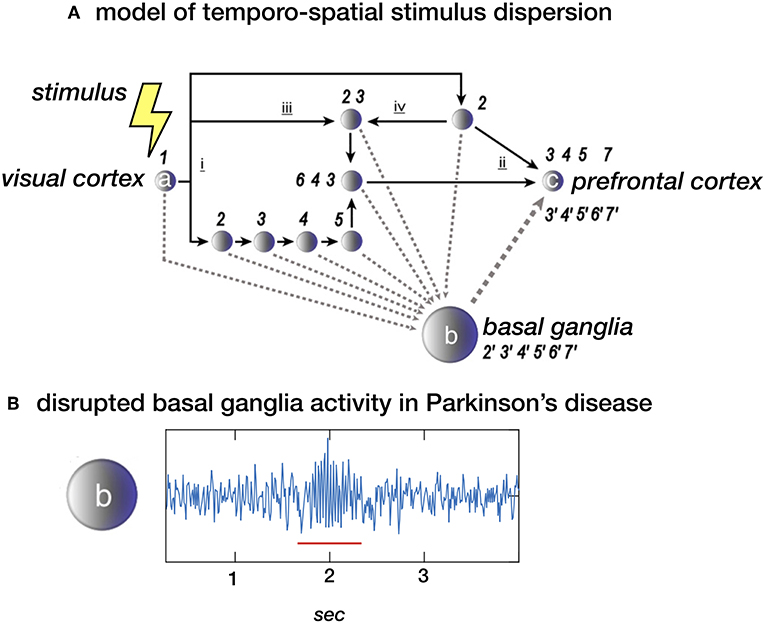
Figure 1. (A) Stimulus dispersion in space and time. Scheme of a simplified neuronal network to illustrate dispersion in space and time following the initial stimulus-induced activation (1) of e.g., the visual cortex (locus a). Successive processing steps (indicated by the numbers 2–7) take place according to principles of functional segregation (i) and integration (ii), as well as bottom-up (iii) and top-down (iv) mechanisms. Differences in synaptic delay along parallel processing streams, due to different number of processing nodes along each pathway, may introduce sequence irregularity. At system-level, however, sequential regularity is maintained by the integration of efferent copies sent to the basal ganglia (locus b). The latter may act as an oscillator, providing a measure of “processing-based” time at network locus c (e.g., the prefrontal cortex). (B) Local field potential (LFP) showing neural activity in the subthalamic nucleus (STN) in a healthy subject of a patient with Parkinsons disease. The “burst” with increased and more synchronized activity in the beta (13–30 Hz) domain (underscored with a red line) is typical for Parkinsons disease. In theory, the proposed model depicted in a. could be disturbed by this pathological activity. (A) Derived from (5), NeuroImage (B) unpublished data.
The concept of minimal intervals of spatial change finds particularly support from a variant of the “flash-lag illusion,” i.e., a “flash-lead” illusion. This phenomenon implies that a moving object is perceived to be behind a spatially concurrent stationary flash before the two disappear (13). Disappearance of the moving dot interrupts building the final “frame,” leaving perception of the preceding frame registering its foregoing location. Assuming that the delay reflects processing time required to construct a “single spatial frame,” it was speculated that this temporal measure is in the magnitude of 100 ms. In the classical “flash-lag” illusion, which implied that the moving object did not disappear but proceeded its trajectory, the moving object is perceived to be ahead of the spatially concurrent stationary flash (14, 15). Here, it is proposed that the percept (“spatial frame”) attributed to the time of the flash is a function of events that happen in the ~100 ms after the flash, i.e., the processing time to construct such frame, with interpolation of the past concerning the moving object (16, 17). In Parkinson's disease (PD), this illusion is disrupted (18). In the present paper, we will discuss the specific alterations of temporal processing in PD and its implications for applying neuromodulation, in particular deep brain stimulation (DBS).
Temporal Processing in Parkinson's Disease
One of the most remarkable features of disturbed temporal processing in PD is noticed when assessing the defining feature of PD; bradykinesia. When asked to make regular finger, hand and arm movements, PD patients show specific difficulty in maintaining sufficient velocity and regularity/rhythm. Furthermore, there is a vast amount of experimental evidence that shows that temporal processing in the perceptual domain is abnormal in PD (19–21). This not only holds for the temporal discrimination threshold (TDT)–the minimum inter-stimulus time between two sensory stimuli which a subject can reliably detect that there are two stimuli rather than one (22) but also for the perception of inter-stimulus intervals (23) and rhythm processing (24). As a consequence, perceptual illusions, such as the rubber hand (25) and flash-lag illusion (18), are perceived differently in PD patients compared to controls. After the application of dopaminergic drugs, several studies have shown a reduction of the TDT more toward healthy controls (22), similar observations are noticed after the application of DBS whereafter the discrimination of auditory inter-stimulus intervals improves (26). As temporal processing improves, recent evidence shows that also perceptual illusions like the rubber hand illusion improve after the application of DBS (27). In several studies the degree of the disturbance in temporal processing is correlated with the severity of Parkinsonian motor symptoms (18, 22, 28). These correlations link the disturbed velocity of movements with temporal processing capacities; the slower a patient moves the slower he / or she can perceive temporal changes.
The pathophysiological mechanism of the disrupted temporal processing in PD are not yet fully elucidated. The fact that there is a dopaminergic depletion in PD and that dopaminergic drugs (and DBS) restore alterations in temporal-processing made initial hypothesis about the its origin go to a “dopaminergic clock” (29). This is further supported by animal-experiments showing that drugs with an opposite effect, neuroleptics, show a decrease of the velocity of the dopaminergic clock and that this is dependent on de D2 affinity of the neuroleptic (30). Further evidence for such dopaminergic clock comes from PET imaging in which TDT values were correlated with the severity of the striatal dopaminergic deficit (31) and fMRI studies in healthy volunteers in which striatal activation occurred during temporal processing (5, 8). Although this correlation provides further evidence for a dopaminergic role. It does not yet elucidate the mechanism at a more explicit neural network level. For this reason, data with a higher temporal resolution; neurophysiological signals, can provide such evidence.
Neurophysiological Alterations in Parkinson's Disease
Stereotactic and functional neurosurgery for movement disorders have provided a unique opportunity to directly record neural activity in the basal ganglia and cortex from either the single neuron, using micro-electrode recordings (MER), or from populations of neurons, using local field potentials (LFP). Furthermore, with advanced signal processing techniques it is currently also possible to extract EEG characteristics, that are specific for movement disorders.
In PD, an excessive domination of beta (13–30 Hz) oscillations is found throughout the motor system. Up to now, the origin of these oscillation in patients is not yet established but recent animal studies have shown that dopaminergic depletion leads to increased firing of striatal spinal projection neurons of the indirect pathway which in turn become prone to being recruited for exaggerated beta oscillations (32) These beta-oscillations decrease after the application of dopaminergic medication (33) and DBS (34). One of the limitations of these findings is that no reference values are present from healthy controls, which makes disease-specificity difficult to prove. However, the amount of beta oscillations correlates with contralateral akinesia-rigidity scores (35) and are more pronounced in PD as in dystonia in a recent meta-analysis (36). Furthermore, not only, the power of local beta oscillations but also the coherence in the beta range between cortex and STN is altered (37) and can be restored by applying DBS (38). One recent, finding is that the presence of increased beta activity changes over time and transient increases of beta activation occurs in so called “bursts” (39, 40). The longer the average burst duration is, the more severe PD symptoms are. Further evidence for a relation between symptom severity with the stability of beta oscillations over time comes from a study in which the variability of beta-power inversely correlated with symptom severity in PD (41). This is consistent with the implication that longer beta bursts reflect reduced beta power variability. In other words, excessive enhanced synchronization of activity in the beta band is present in PD and is correlated with the severity of motor symptoms.
Linking Neurophysiological Alterations with Temporal Processing
So far, no experimental evidence is available that directly links disturbed (beta) oscillatory activity to disturbed temporal processing in PD. However, beta oscillations have been involved in many other processes beyond movement. Transient beta oscillations play an important role in the successful stopping of actions (42, 43). Furthermore, beta oscillations play a role in “status quo maintenance” (44) and “top-down” based attention (45). Next to this, the volatility of beta oscillations (beta modulation) is involved in the sequencing of complex sensorimotor processes including repetitive movements (46) as well as passive listening to isochronous sounds (47). Based on these findings and the arguments presented in the previous sections one may infer that the correlation of PD symptom severity with (i) the amount of beta oscillations as well as (ii) altered perceptual time processing, provides an indirect arguments for the context that exaggerated oscillatory activity in the motor system [e.g., (38)] represents a neuronal mechanisms causing altered temporal processing in general. In other words, one may ask whether it is possible that exaggerated synchronized activity discards temporally sensitive information (efferent copies) as a noise filter (Figure 2) And consequently, is the magnitude of established change in perceptual illusions determined based on exaggerated beta oscillations? These hypotheses can be tested by combining psychophysical paradigms with time-locked neurophysiological recordings [e.g., (48)].
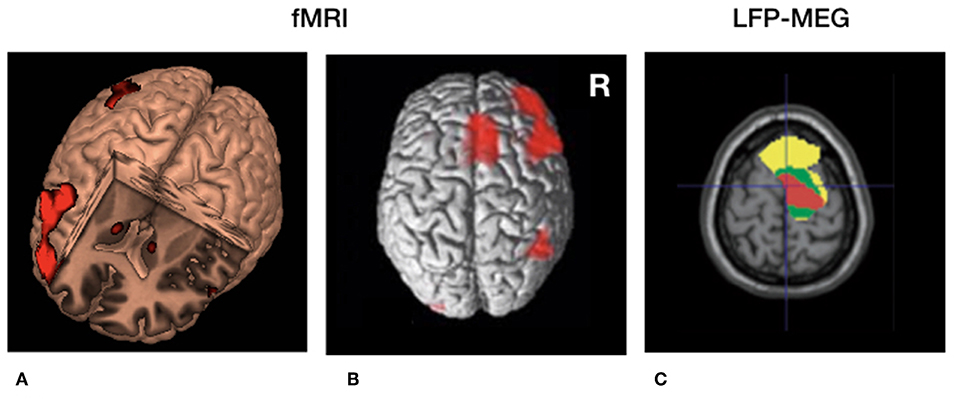
Figure 2. Cerebral circuitry involved in temporal processing and cerebral circuitry selectively modulated by deep brain stimulation (DBS) in Parkinson's disease. (A) 3D fMRI activation pattern in healthy volunteers during a temporal estimation task showing increased bilateral basal ganglia activity and right frontal activation. (B) Similar rendered contrast showing additional activation of the supplementary motor area (SMA). (C) Beta (13–30 Hz) coherence between local field potentials (LFP's) of the subthalamic nucleus (STN) and frontal regions recorded with magnetoencephalography (MEG). Yellow, low beta (13–21 Hz); Green, high beta (21–30 Hz) coherence. Red = decreased cortical beta coherence after the application of DBS. (A,B) Derived with permission from (5), NeuroImage (C) derived with permission from (38), Brain.
Implications for Neuromodulation
Although DBS is an established treatment for refractory movement disorders, it has its limitations in terms of efficacy and side-effects. One of the reasons for this is that not only pathological but also physiological neural activity is suppressed. Given the natural fluctuation of the severity of Parkinsonian symptoms, e.g., due to dopaminergic medication and fatigue, DBS might, in PD, only be effective when pathological neural activity is present, and symptoms are present [e.g., (49)]. This implies that DBS should indeed not be provided continuously but in an intermittent and adaptive way, adaptive DBS (aDBS) (50). Up to now, all the experimental evidence for aDBS comes from paradigms in which high frequency stimulation (±130 Hz) is either switched ON or OFF or modulated in amplitude based on the amount of pathological beta oscillations (Figure 3). On the other hand, beta oscillations also fulfill a physiological, anti-kinetic role in terminating actions (43). When applying DBS in such a way that it is only switched on when disruptive beta oscillations are present, DBS might provide a more profound effect while stimulation induced side effects would be reduced (51).
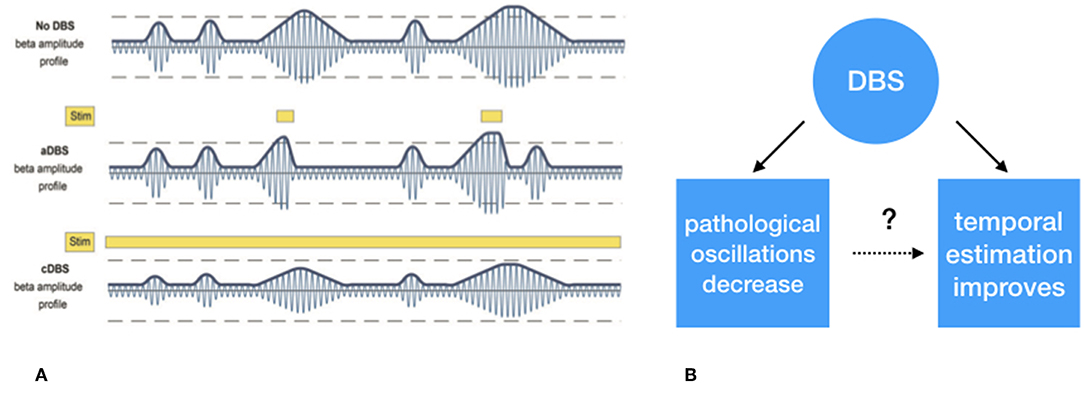
Figure 3. (A) Schematic representation of exaggerated beta (13–30 Hz) “burst” activity derived from the subthalamic nucleus. The first row depicts the similar periodically enhanced beta activity as depicted in Figure 1. The second row depicts the effect of selective suppression of beta burst activity of a certain magnitude and duration. This leads to selective, i.e., adaptive, stimulation. In the third row, the non-selective effect of continuous stimulation, conventional DBS, is depicted which leads to an overall suppression of beta activity, irrespective its magnitude, and duration. (B) Whether the suppression of exaggerated leads to improved temporal estimation is to be tested. (A) Derived with permission from (39), Brain.
Although the approach described before adapts stimulation on the amount of pathological oscillations, it is still independent of sensorimotor processing. Another approach to time neuromodulation is to apply stimulation based on the presence of events when pathological activation occurs. Such approach has been clinically applied for almost a decade in epilepsy patients [e.g., (52)] and recently also a patient with Tourette syndrome (53). In these two disorders DBS was triggered by the presence of epileptic activity and presence of neural activity associated with tics, respectively. In theory, DBS could also be triggered by other potentials that are, for example, related to movement initiation. This approach has recently been trialed a in a stroke recovery model (54). In this study, electrical stimulation time-locked to transient LFO's, which occur during skilled upper limb tasks, significantly improved upper limb function. Such form of precision medicine highlights the importance of the accurate timing of neuromodulation. Further support for such a phase-specific role of neuromodulation comes from tremor studies in which DBS (55) or transcranial direct current stimulation [TDCS, (49)] aligned to tremor phase, resulted in significant tremor suppression with minimally delivered energy. Although, such an “event-based” form of stimulation has not been trialed in PD, a recent paper (56) showed the temporal course of STN activation during inhibitory, motor, and cognitive tasks, which might form the basis for such stimulation algorithms. By applying these event based analyses of LFP's the effects of different forms of stimulation can be tested and a the role between physiological oscillatory activity [e.g., (48)] and disturbed oscillatory activity could be better elucidated (Figure 3). In line with this, by filtering disturbed oscillatory activity, but leaving physiological neuronal activity unaffected, sensorimotor processing, including temporal processing might be return to physiological conditions. Evidence for this comes from recent studies showing that conventional (continuous) DBS shows both a more constant suppression of subcortical (39) and cortical beta activity (57), which might lack the essential volatility to show peri-stimulus beta modulation to process essential temporal information. Of course this needs to be proven by empirical findings and we hope to test these hypotheses in the nearby future.
Parallels within other Movement Disorders
Dystonia is another movement disorder in which temporal processing has been extensively tested. Temporal discrimination thresholds have been found to be elevated across both the visual and somatosensory domains (58). Interestingly in cervical dystonia, abnormalities in temporal discrimination in relatives has led to the hypothesis that abnormalities in TDT are a marker of non-manifesting gene carriage [acting as an endophenotype, (59)]. In contrast to PD, TDT abnormalities persist despite the efficacy of GPi-DBS, suggesting that DBS does not appear to improve dystonic motor activity by correcting abnormalities in sensory processing, at least as measured by the TDT (60). A distributed network is likely to be involved in the processing of TDT, however, notably much of the variability of TDT values across subjects has been linked to neural markers of inhibition in the primary somatosensory cortex [S1, (61)]. Furthermore, high frequency repetitive stimulation over the S1 increases neurophysiological makers of inhibition and sensory function and such changes correlate with improvements in TDT (62). Thus, similar to PD, such findings hint that neural correlates of TDT abnormalities could be used to provide a richer input environment to inform adaptive DBS strategies. This is particularly relevant in dystonia as clinical response often lags many months behind changes or the initiation of DBS offering the operator little real time feedback by which to optimize stimulation parameters.
Compared to cerebellar ataxia, as seen in cerebellar degeneration, PD patients show a selective disturbance in rhythmic temporal prediction, and not in single interval prediction (19, 21). These findings are in line with the role of the basal ganglia in monitoring minimal intervals of successive spatial change, providing an internal measure of non-contextual time (See section Time and the Brain).
Future work
In order to further elucidate the true nature of disturbed temporal processing and its potential therapeutic consequences in PD, several new avenues are currently being explored. Testing temporal processing draws on psychophysics, and there are some drawbacks of the different psychophysical paradigms which have been previously applied. Since most of these paradigms rely on self-report, they can be subject to bias. Standard staircase methodology in which the separation between two stimuli is slowly increased or decreased in a predictable manner allows the obtained thresholds to be readily biased by a decision strategy unrelated to temporal discrimination ability (63). For example, if stimuli are gradually changed in the direction of threshold over several trials experimentally it has been shown that some observers develop a habit of repeating the same response and thus continuing to make the response after the threshold point has been reached. This “error of habituation” affects the data by falsely increasing thresholds (64). Within some paradigms catch trials (single stimuli trials) are also introduced which attempts to mitigate these errors and encourages participants to evaluate the sensory information arriving on each trial. However, such biases are best mitigated by randomizing the order of presentation (64).
The analysis of psychophysical paradigms has also progressed hugely since the methods of limits were established and their limitations acknowledged in the 1960s. By using reaction time data as well as well as accuracy of response inferences can also be made about the decision making components integral to the TDT. Such factors have begun to be explored in PD. For example, computational modeling has revealed that timing deficits in PD cannot be solely attributed to perceptual temporal distortions, but are also associated with impulsive decision strategies that bias patients toward premature responses (65). Similarly, drift diffusion decision modeling in a large group of subjects with cervical dystonia points to a more conservative decision strategy in cervical dystonia over and above a temporal discrimination deficit (63). Such finding are highly feasible as the subtle neuropsychiatric profile of many movement disorders are increasingly appreciated.
Although such studies increase the complexity of interpretation of simple TDT threshold embracing such techniques and analysis may prove its utility in the future. We are still far from having disease specific psychophysical markers for temporal processing. By better estimation of the true psychophysical deficit psychophysical deficits unique to particular disease states may be found. This yields greater power to researchers to discover the corresponding neural correlate which could be used for aDBS. This especially involves the experiments in which state of art neuromodulation, neurophysiology and psychophysics are simultaneously applied.
Conclusion
Temporal processing is disturbed in PD while the severity of the movement disorder is sometimes correlated with the magnitude of changes in perceptual temporal processing. It is not yet established whether there is a causative link but pathological neural oscillatory activity might play a role. Furthermore, DBS improves motor performance and perceptual temporal processing and reduces pathological neural oscillatory activity. These observations provide indirect evidence that temporal processing is similarly affected by the same pathological neural oscillatory activity. As we move toward an era of more effective adaptive DBS finding neural correlates of temporal processing abnormalities may allow DBS to be dynamically titrated in response to a wider range of pathophysiological parameters. By bringing together neuromodulation, advanced neurophysiology and psychophysics, these hypotheses can be tested.
Author Contributions
BdJ and AS respectively wrote the 1st and 6th + 7th sections of the manuscript. MB wrote the first draft of the manuscript. MB. BdJ, AS, and ME revised the manuscript.
Conflict of Interest Statement
The authors declare that the research was conducted in the absence of any commercial or financial relationships that could be construed as a potential conflict of interest.
References
1. Medendorp WP. Spatial constancy mechanisms in motor control. Philosoph Trans R Soc B Biol Sci. (2011) 366:476–91. doi: 10.1098/rstb.2010.0089
2. Ciullo V, Vecchio D, Gili T, Spalletta G, Piras F. Segregation of brain structural networks supports spatio-temporal predictive processing. Front Hum Neurosci. (2018) 12:212. doi: 10.3389/fnhum.2018.00212
3. Gritsenko V, Yakovenko S, Kalaska JF. Integration of predictive feedforward and sensory feedback signals for online control of visually guided movement. J Neurophysiol. (2009) 102:914–30. doi: 10.1152/jn.91324.2008
4. Bartels A, Zeki S. The chronoarchitecture of the human brain–natural viewing conditions reveal a time-based anatomy of the brain. Neuroimage. (2004) 22:419–33. doi: 10.1016/j.neuroimage.2004.01.007
5. Beudel M, Renken R, Leenders KL, de Jong BM. Cerebral representations of space and time. Neuroimage. (2009) 44:1032–40. doi: 10.1016/j.neuroimage.2008.09.028
6. Spencer RMC, Zelaznik HN, Diedrichsen J, Ivry RB. Disrupted timing of discontinuous but not continuous movements by cerebellar lesions. Science. (2003) 300:1437–9. doi: 10.1126/science.1083661
7. Schlerf JE, Spencer RMC, Zelaznik HN, Ivry RB. Timing of rhythmic movements in patients with cerebellar degeneration. Cerebellum. (2007) 6:221–31. doi: 10.1080/14734220701370643
8. Meck WH, Malapani C. Neuroimaging of interval timing. Brain Res Cogn Brain Res. (2004) 21:133–7. doi: 10.1016/j.cogbrainres.2004.07.010
9. Shen B, Wang Z-R, Wang X-P. The fast spiking subpopulation of striatal neurons coding for temporal cognition of movements. Front Cell Neurosci. (2017) 11:406. doi: 10.3389/fncel.2017.00406
10. Karmarkar UR, Buonomano DV. Timing in the absence of clocks: encoding time in neural network states. Neuron. (2007) 53:427–38. doi: 10.1016/j.neuron.2007.01.006
11. Matell MS, Meck WH, Nicolelis MAL. Interval timing and the encoding of signal duration by ensembles of cortical and striatal neurons. Behav Neurosci. (2003) 117:760–73. doi: 10.1037/0735-7044.117.4.760
12. Buhusi C, Meck W. What makes us tick? Functional and neural mechanisms of interval timing. Nat Rev Neurosci. (2005) 6:755–65. doi: 10.1038/nrn1764
13. Roulston BW, Self MW, Zeki S. Perceptual compression of space through position integration. Proc Biol Sci. (2006) 273:2507–12. doi: 10.1098/rspb.2006.3616
15. Eagleman DM, Sejnowski TJ. Motion integration and postdiction in visual awareness. Science. (2000) 287:2036–8. doi: 10.1126/science.287.5460.2036
16. Schneider KA. The flash-lag, fröhlich and related motion illusions are natural consequences of discrete sampling in the visual system. Front Psychol. (2018) 9:1227. doi: 10.3389/fpsyg.2018.01227
17. Beudel M, de Geus CM, Leenders KL, de Jong BM. Acceleration bias in visually perceived velocity change and effects of Parkinson's bradykinesia. Neuro Rep. (2013) 24:773–8. doi: 10.1097/WNR.0b013e328363f739
18. Beudel M, Roosma E, Martinez Manzanera OE, van Laar T, Maurits NM, de Jong BM. Parkinson bradykinesia correlates with EEG background frequency and perceptual forward projection. Parkinsonism Relat Disord. (2015) 21:783–8. doi: 10.1016/j.parkreldis.2015.05.004
19. Beudel M, Galama S, Leenders KL, de Jong BM. Time estimation in Parkinson's disease and degenerative cerebellar disease. Neuroreport. (2008) 19:1055–8. doi: 10.1097/WNR.0b013e328303b7b9
20. Conte A, Khan N, Defazio G, Rothwell JC, Berardelli A. Pathophysiology of somatosensory abnormalities in Parkinson disease. Nat Publ Group. (2013) 9:687–97. doi: 10.1038/nrneurol.2013.224
21. Breska A, Ivry RB. Double dissociation of single-interval and rhythmic temporal prediction in cerebellar degeneration and Parkinson's disease. Proc Natl Acad Sci USA. (2018) 115:12283–8. doi: 10.1073/pnas.1810596115
22. Artieda J, Pastor MA, Lacruz F, Obeso JA. Temporal discrimination is abnormal in Parkinson's disease. Brain. (1992) 115:199–210. doi: 10.1093/brain/115.1.199
23. O'Boyle DJ, Freeman JS, Cody FW. The accuracy and precision of timing of self-paced, repetitive movements in subjects with Parkinson's disease. Brain. (1996) 119(Pt 1):51–70. doi: 10.1093/brain/119.1.51
24. Grahn JA, Brett M. Impairment of beat-based rhythm discrimination in Parkinson's disease. Cortex. (2009) 45:54–61. doi: 10.1016/j.cortex.2008.01.005
25. Ding C, Palmer CJ, Hohwy J, Youssef GJ, Paton B, Tsuchiya N, et al. Parkinson's disease alters multisensory perception: Insights from the Rubber Hand Illusion. Neuropsychologia. (2017) 97:38–45. doi: 10.1016/j.neuropsychologia.2017.01.031
26. Koch G, Brusa L, Caltagirone C, Oliveri M, Peppe A, Tiraboschi P, et al. Subthalamic deep brain stimulation improves time perception in Parkinson's disease. Neuro Rep. (2004) 15:1071–3. doi: 10.1097/00001756-200404290-00028
27. Ding C, Palmer CJ, Hohwy J, Youssef GJ, Paton B, Tsuchiya N, et al. Deep Brain Stimulation for Parkinson's disease changes perception in the Rubber Hand Illusion. Sci Rep. (2018) 8:13842. doi: 10.1038/s41598-018-31867-8
28. Conte A, Leodori G, Ferrazzano G, De Bartolo MI, Manzo N, Fabbrini G, et al. Somatosensory temporal discrimination threshold in Parkinson's disease parallels disease severity and duration. Clin Neurophysiol. (2016) 127:2985–9. doi: 10.1016/j.clinph.2016.06.026
29. Meck WH, Benson AM. Dissecting the brain's internal clock: how frontal-striatal circuitry keeps time and shifts attention. Brain Cogn. (2002) 48:195–211. doi: 10.1006/brcg.2001.1313
30. Meck WH. Affinity for the dopamine D2 receptor predicts neuroleptic potency in decreasing the speed of an internal clock. Pharmacol Biochem Behav. (1986) 25:1185–9. doi: 10.1016/0091-3057(86)90109-7
31. Lyoo CH, Ryu YH, Lee MJ, Lee MS. Striatal dopamine loss and discriminative sensory dysfunction in Parkinson's disease. Acta Neurol Scand. (2012) 126:344–9. doi: 10.1111/j.1600-0404.2012.01657.x
32. Sharott A, Vinciati F, Nakamura KC, Magill PJ. A population of indirect pathway striatal projection neurons is selectively entrained to parkinsonian beta oscillations. J Neurosci. (2017) 37:9977–98. doi: 10.1523/JNEUROSCI.0658-17.2017
33. Kühn AA, Kupsch A, Schneider G-H, Brown P. Reduction in subthalamic 8-35 Hz oscillatory activity correlates with clinical improvement in Parkinson's disease. Eur J Neurosci. (2006) 23:1956–60. doi: 10.1111/j.1460-9568.2006.04717.x
34. Eusebio A, Thevathasan W, Doyle Gaynor L, Pogosyan A, Bye E, Foltynie T, et al. Deep brain stimulation can suppress pathological synchronisation in parkinsonian patients. J Neurol Neurosurg Psychiatr. (2011) 82:569–73. doi: 10.1136/jnnp.2010.217489
35. Beudel M, Oswal A, Jha A, Foltynie T, Zrinzo L, Hariz M, et al. Oscillatory beta power correlates with akinesia-rigidity in the Parkinsonian subthalamic nucleus. Mov Disord. (2017) 32:174–5. doi: 10.1002/mds.26860
36. Pi-a-Fuentes D, van Dijk JMC, Drost G, van Zijl JC, van Laar T, Tijssen MAJ, et al. Direct Comparison of oscillatory activity in the motor system of Parkinson's disease and dystonia: a review of the literature and meta-analysis. Clin Neurophysiol. (2019) 130:917–24. doi: 10.1016/j.clinph.2019.02.015
37. Litvak V, Jha A, Eusebio A, Oostenveld R, Foltynie T, Limousin P, et al. Resting oscillatory cortico-subthalamic connectivity in patients with Parkinson's disease. Brain. (2011) 134:359–74. doi: 10.1093/brain/awq332
38. Oswal A, Beudel M, Zrinzo L, Limousin P, Hariz M, Foltynie T, et al. Deep brain stimulation modulates synchrony within spatially and spectrally distinct resting state networks in Parkinson's disease. Brain. (2016) 139:1482–96. doi: 10.1093/brain/aww048
39. Tinkhauser G, Pogosyan A, Little S, Beudel M, Herz DM, Tan H, et al. The modulatory effect of adaptive deep brain stimulation on beta bursts in Parkinson's disease. Brain. (2017) 140:1053–67. doi: 10.1093/brain/awx010
40. Anidi C, O'Day JJ, Anderson RW, Afzal MF, Syrkin-Nikolau J, Velisar A, et al. Neuromodulation targets pathological not physiological beta bursts during gait in Parkinson's disease. Neurobiol Dis. (2018) 120:107–17. doi: 10.1016/j.nbd.2018.09.004
41. Little S, Pogosyan A, Kühn AA, Brown P. β band stability over time correlates with Parkinsonian rigidity and bradykinesia. Exp Neurol. (2012) 236:383–8. doi: 10.1016/j.expneurol.2012.04.024
42. Jha A, Nachev P, Barnes G, Husain M, Brown P, Litvak V. The frontal control of stopping. Cereb Cortex. (2015) 25:4392–406. doi: 10.1093/cercor/bhv027
43. Wessel JR, Ghahremani A, Udupa K, Saha U, Kalia SK, Hodaie M, et al. Stop-related subthalamic beta activity indexes global motor suppression in Parkinson's disease. Mov Disord. (2016) 31:1846–53. doi: 10.1002/mds.26732
44. Engel AK, Fries P. Beta-band oscillations–signalling the status quo? Curr Opin Neurobiol. (2010) 20:156–65. doi: 10.1016/j.conb.2010.02.015
45. Buschman TJ, Miller EK. Top-down versus bottom-up control of attention in the prefrontal and posterior parietal cortices. Science. (2007) 315:1860–2. doi: 10.1126/science.1138071
46. Joundi RA, Brittain J-S, Green AL, Aziz TZ, Brown P, Jenkinson N. Persistent suppression of subthalamic beta-band activity during rhythmic finger tapping in Parkinson's disease. Clin Neurophysiol. (2013) 124:565–73. doi: 10.1016/j.clinph.2012.07.029
47. Fujioka T, Trainor LJ, Large EW, Ross B. Internalized timing of isochronous sounds is represented in neuromagnetic beta oscillations. J Neurosci. (2012) 32:1791–802. doi: 10.1523/JNEUROSCI.4107-11.2012
48. Hall TM, Nazarpour K, Jackson A. Real-time estimation and biofeedback of single-neuron firing rates using local field potentials. Nat Commun. (2014) 5:5462. doi: 10.1038/ncomms6462
49. Brittain J-S, Sharott A, Brown P. The highs and lows of beta activity in cortico-basal ganglia loops. Eur J Neurosci. (2014) 39:1951–9. doi: 10.1111/ejn.12574
50. Little S, Brown P. What brain signals are suitable for feedback control of deep brain stimulation in Parkinson's disease? Ann N Y Acad Sci. (2012) 1265:9–24. doi: 10.1111/j.1749-6632.2012.06650.x
51. Little S, Tripoliti E, Beudel M, Pogosyan A, Cagnan H, Herz D, et al. Adaptive deep brain stimulation for Parkinson's disease demonstrates reduced speech side effects compared to conventional stimulation in the acute setting. J Neurol Neurosurg Psychiatr. (2016) 87:1388–9. doi: 10.1136/jnnp-2016-313518
52. Geller EB, Skarpaas TL, Gross RE, Goodman RR, Barkley GL, Bazil CW, et al. Brain-responsive neurostimulation in patients with medically intractable mesial temporal lobe epilepsy. Epilepsia. (2017) 58:994–1004. doi: 10.1111/epi.13740
53. Molina R, Okun MS, Shute JB, Opri E, Rossi PJ, Martinez-Ramirez D, et al. Report of a patient undergoing chronic responsive deep brain stimulation for Tourette syndrome: proof of concept. J Neurosurg. (2017) 129:277–565. doi: 10.3171/2017.6.JNS17626
54. Ramanathan DS, Guo L, Gulati T, Davidson G, Hishinuma AK, Won S-J, et al. Low-frequency cortical activity is a neuromodulatory target that tracks recovery after stroke. Nat Med. (2018) 24:1257–67. doi: 10.1038/s41591-018-0058-y
55. Cagnan H, Pedrosa D, Little S, Pogosyan A, Cheeran B, Aziz T, et al. Stimulating at the right time: phase-specific deep brain stimulation. Brain. (2017) 140:132–45. doi: 10.1093/brain/aww286
56. Neumann W-J, Schroll H, de Almeida Marcelino AL, Horn A, Ewert S, Irmen F, et al. Functional segregation of basal ganglia pathways in Parkinson's disease. Brain. (2018) 12:366–316. doi: 10.1093/brain/awy206
57. Gulberti A, Moll CKE, Hamel W, Buhmann C, Koeppen JA, Boelmans K, et al. Predictive timing functions of cortical beta oscillations are impaired in Parkinson's disease and influenced by L-DOPA and deep brain stimulation of the subthalamic nucleus. NeuroImage Clin. (2015) 9:436–49. doi: 10.1016/j.nicl.2015.09.013
58. Tinazzi M, Frasson E, Bertolasi L, Fiaschi A, Aglioti S. Temporal discrimination of somesthetic stimuli is impaired in dystonic patients. Neuro Rep. (1999) 10:1547–50. doi: 10.1097/00001756-199905140-00028
59. Conte A, McGovern EM, Narasimham S, Beck R, Killian O, O'Riordan S, et al. Temporal discrimination: mechanisms and relevance to adult onset dystonia. Front Neurol. (2017) 8:625. doi: 10.3389/fneur.2017.00625
60. Sadnicka A, Kimmich O, Pisarek C, Ruge D, Galea J, Kassavetis P, et al. Pallidal stimulation for cervical dystonia does not correct abnormal temporal discrimination. Mov Disord. (2013) 28:1874–7. doi: 10.1002/mds.25581
61. Rocchi L, Casula E, Tocco P, Berardelli A, Rothwell J. Somatosensory temporal discrimination threshold involves inhibitory mechanisms in the primary somatosensory area. J Neurosci. (2016) 36:325–35. doi: 10.1523/JNEUROSCI.2008-15.2016
62. Rocchi L, Erro R, Antelmi E, Berardelli A, Tinazzi M, Liguori R, et al. High frequency somatosensory stimulation increases sensori-motor inhibition and leads to perceptual improvement in healthy subjects. Clin Neurophysiol. (2017) 128:1015–25. doi: 10.1016/j.clinph.2017.03.046
63. Sadnicka A, Daum C, Cordivari C, Bhatia KP, Rothwell JC, Manohar S, et al. Mind the gap: temporal discrimination and dystonia. Eur J Neurol. (2017) 24:796–806. doi: 10.1111/ene.13293
64. Gescheider GA. Psychophysics: The Fundamentals. Abingdon, UK: Psychology Press (2013). doi: 10.4324/9780203774458
Keywords: Parkinson's disease, deep brain stimulation, oscillations, timing, movement disorders
Citation: Beudel M, Sadnicka A, Edwards M and de Jong BM (2019) Linking Pathological Oscillations With Altered Temporal Processing in Parkinsons Disease: Neurophysiological Mechanisms and Implications for Neuromodulation. Front. Neurol. 10:462. doi: 10.3389/fneur.2019.00462
Received: 29 January 2019; Accepted: 16 April 2019;
Published: 10 May 2019.
Edited by:
Tim Anderson, University of Otago, Christchurch, New ZealandReviewed by:
Antonella Conte, Sapienza University of Rome, ItalyRick Helmich, Radboud University Nijmegen Medical Centre, Netherlands
Copyright © 2019 Beudel, Sadnicka, Edwards and de Jong. This is an open-access article distributed under the terms of the Creative Commons Attribution License (CC BY). The use, distribution or reproduction in other forums is permitted, provided the original author(s) and the copyright owner(s) are credited and that the original publication in this journal is cited, in accordance with accepted academic practice. No use, distribution or reproduction is permitted which does not comply with these terms.
*Correspondence: Martijn Beudel, bS5iZXVkZWxAYW1jLnV2YS5ubA==