- 1Chronobiology Laboratory, Department of Physiology, IMIB-Arrixaca, University of Murcia, Murcia, Spain
- 2Ciber Fragilidad y Envejecimiento Saludable, Madrid, Spain
- 3Advanced Technology Institute and Department of Physics, University of Surrey, Guildford, United Kingdom
- 4Chronobiology, Faculty of Health and Medical Sciences, University of Surrey, Guildford, United Kingdom
- 5Surrey Clinical Research Centre, Faculty of Health and Medical Sciences, University of Surrey, Guildford, United Kingdom
The pupillary light reflex (PLR) is a neurological reflex driven by rods, cones, and melanopsin-containing retinal ganglion cells. Our aim was to achieve a more precise picture of the effects of 5-min duration monochromatic light stimuli, alone or in combination, on the human PLR, to determine its spectral sensitivity and to assess the importance of photon flux. Using pupillometry, the PLR was assessed in 13 participants (6 women) aged 27.2 ± 5.41 years (mean ± SD) during 5-min light stimuli of purple (437 nm), blue (479 nm), red (627 nm), and combinations of red+purple or red+blue light. In addition, nine 5-min, photon-matched light stimuli, ranging in 10 nm increments peaking between 420 and 500 nm were tested in 15 participants (8 women) aged 25.7 ± 8.90 years. Maximum pupil constriction, time to achieve this, constriction velocity, area under the curve (AUC) at short (0–60 s), and longer duration (240–300 s) light exposures, and 6-s post-illumination pupillary response (6-s PIPR) were assessed. Photoreceptor activation was estimated by mathematical modeling. The velocity of constriction was significantly faster with blue monochromatic light than with red or purple light. Within the blue light spectrum (between 420 and 500 nm), the velocity of constriction was significantly faster with the 480 nm light stimulus, while the slowest pupil constriction was observed with 430 nm light. Maximum pupil constriction was achieved with 470 nm light, and the greatest AUC0−60 and AUC240−300 was observed with 490 and 460 nm light, respectively. The 6-s PIPR was maximum after 490 nm light stimulus. Both the transient (AUC0−60) and sustained (AUC240−300) response was significantly correlated with melanopic activation. Higher photon fluxes for both purple and blue light produced greater amplitude sustained pupillary constriction. The findings confirm human PLR dependence on wavelength, monochromatic or bichromatic light and photon flux under 5-min duration light stimuli. Since the most rapid and high amplitude PLR occurred within the 460–490 nm light range (alone or combined), our results suggest that color discrimination should be studied under total or partial substitution of this blue light range (460–490 nm) by shorter wavelengths (~440 nm). Thus for nocturnal lighting, replacement of blue light with purple light might be a plausible solution to preserve color discrimination while minimizing melanopic activation.
Introduction
The pupillary light reflex (PLR) is a neurological reflex characterized by a reduction in pupil diameter in response to an increase in retinal illumination, as well as the subsequent redilation of the pupil after light cessation. Its main function is to increase the depth of field and image sharpness in bright light conditions.
Rods and cones were the only known mammal photoreceptors until the discovery of melanopsin (1), a photopigment with a peak of sensitivity (λmax) at 480 nm, which is expressed in the intrinsically photosensitive retinal ganglion cells (ipRGCs) (2). These ipRGCs project to the suprachiasmatic nuclei (SCN), the circadian pacemaker, and other non-image forming brain areas, such as the olivary pretectal nucleus (OPN), a control center for the PLR (3–7). Thus, ipRGCs participate in a common pathway for the PLR and other processes such as circadian entrainment, either by themselves (intrinsically) or through their connections with the outer retinal photoreceptors (extrinsically), the most efficient wavelengths (humans, λmax 446–477 nm) to both entrain the circadian timing system and inhibit melatonin synthesis (8, 9) being those closer to the maximal sensitivity for ipRGCs. In addition, a relationship between circadian status and PLR has recently been reported (10), indicating a complex inverse relationship between both systems. Once this common pathway and its interactions are further studied, it may be plausible to assess the effect of different lights on the human circadian system through their effects on the PLR.
The human PLR follows a general dynamic (11–14), that can be affected by the intensity, spectral composition (15, 16) and duration of the light stimulus. When the stimulus starts, the pupil shows a rapid constriction until it reaches a minimum size (maximal constriction amplitude). After this early transient response, a pupillary re-dilatation occurs (escape), reaching a more sustained state of partial pupil constriction, which lasts until the end of the light stimulus (17) as well as after termination (post-illumination pupil response, PIPR) (12). According to some studies in primates and humans, the early transient pupil constriction is predominantly driven by cones, while control of the sustained and persistent PIPR seems to correspond to a melanopsin-mediated intrinsic response (12, 18–20). Recent studies, however, have suggested that the outer retinal photoreceptors could also participate in this sustained (21–23) and post-illumination pupil response (PIPR) (24, 25). Despite some limitations of pupillometry, it is possible to infer rod and cone function and the intrinsic activation of ipRGCs independently by analyzing the transient, sustained, and persistent (or PIPR) pupillary response to light stimuli of different wavelengths, intensities, and durations (17).
Apart from their relative specificity on the PLR dynamics, each human retinal photoreceptor exhibits different wavelength sensitivities, based on their corresponding photopigments: λmax 498 nm for rods, λmax 420 nm for S-cones, λmax 530 nm for M-cones, λmax 559 nm for L-cones (26). The maximum sensitivity for melanopsin-containing ipRGCs has been established at 480 nm (2, 6, 27), although other PLR studies in humans indicate peak sensitivities around 490 nm (28), based on ocular photoresponses. In primates intensity thresholds for each photoreceptor are also different, being higher for ipRGCs (~10–11 log quanta/cm2/s) (23, 29) than for the classical photoreceptors [cones 2.30 log quanta/cm2/s; rods 1.70 log quanta /cm2/s, at the cornea level (30)]. Furthermore, it has been proposed that melanopsin's spatial conformation and thus its wavelength sensitivity can switch back from the M to R state by absorbing longer wavelength photons, so-called melanopsin bistability (31, 32). This has also been associated with the PLR, exhibiting increased pupil constriction when the light stimulus was preceded by longer wavelength light (32). Tristability (with two silent and one signaling state) has also been suggested as a mechanism for ipRGC to integrate both time and wavelength (33). However, not all studies have been able to demonstrate this long wavelength potentiation of blue light responses (34, 35), while some studies have proposed the existence of retinal pigment epithelium (RPE)-derived regeneration in melanopsin (36, 37), which could be interpreted as a complementary mechanism [reviewed in (38)].
The study of possible interactions between two monochromatic wavelengths when administered simultaneously, as well as assessment of PLR sensitivity over a high resolution short wavelength range will help to provide knowledge on the effect of polychromatic lights on the PLR.
The aim of this study was thus to achieve a more precise picture of the effects of 5-min monochromatic light stimuli, alone or in combination [long (red) combined with short (blue and purple) wavelength lights], on the human PLR (including PIPR), to determine its spectral sensitivity and to confirm the importance of photon flux as a determinant of the human PLR.
Based on previous knowledge, we hypothesized that blue or purple light would produce different responses when combined with red light as a result of melanopsin bistability, probably increasing the sustained response (greater amplitude) due to the conformational change of melanopsin by red light. Regarding monochromatic short wavelength light, we expected greater pupillary responses under the 460–490 nm light range, and under higher light intensities.
Materials and Methods
Participants
This study was approved by the University of Surrey Ethics Committee. Volunteers received appropriate information about the study protocol, signed a written informed consent form (in compliance with the Declaration of Helsinki) before being enrolled and were compensated for their participation.
In both experimental conditions participants were healthy, non-smoking volunteers: 13 (6 women) between 19 and 35 years (27.2 ± 5.41 years, mean ± SD) for Study A, and 15 (8 women) between 19 and 35 years (25.7 ± 8.90 years) for Study B. Data from three participants from Study A were excluded from analysis because of very noisy PLR signals that were not interpretable.
All participants declared no medical or mental health disorders and were not taking any medication that could affect circadian rhythms, according to the general health questionnaires completed during the screening period. None of them were shift workers nor had crossed more than two time zones in the 2 months prior to study admission. They kept regular sleep-wake cycles with no reported sleep disorders (Pittsburgh Sleep Quality Index ≤ 5) (39), and were not extreme morning or evening types (40). A full ophthalmic examination including uncorrected vision, near vision corrected, ophthalmoscopy, pupil reactions, Henson Field Test, refraction, intra-ocular pressure, oculomotor status, stereo acuity, accommodation, and color vision by the Ishihara test, was performed to confirm they all were free from any ocular disorders.
Pre-study Measurements
The protocol used was similar to that previously described (41) with participants maintaining a regular, actigraphically (AWL, Cambridge Neurotechnology, UK) monitored sleep/wake schedule for at least 7 days before and throughout the in-laboratory sessions. For 72 h before and during each laboratory session, participants refrained from caffeinated drinks, alcohol, excessive exercise, bright lights, and non-steroidal anti-inflammatory drug intake.
In-laboratory Protocol
Protocol for Light Stimuli Presentation
A randomized, within-subject design was performed. In both experimental conditions A and B, all the sessions were carried out in the morning. In Study A (Figure 1A) the participants (3 per session) arrived at the laboratory and remained seated in dim light (< 5 lux) for 30 min in order to progressively adapt their vision to the dark conditions. Then, he or she received a drop of tropicamide [Minims Tropicamide (1.0%, Chauvin Pharmaceuticals, Romford, UK)] in the right eye to dilate the pupil. After that, the participant remained in darkness (0 lux + eye mask) for an hour prior to pupil recording in order to avoid any confounding effect due to prior light exposures (42). Once this dark adaptation schedule was completed, pupil recording started. For this, the left non-dilated pupil was recorded [220 frames per second] in darkness for 60 s to obtain a baseline, which was later used as a control to normalize the pupil diameter. Then, the light source was turned on and the right eye (pupil dilated) received a light stimulus for 5 min (Figure 1A) while recording the left pupil, thus assessing the consensual reflex. Only one light condition was tested per laboratory session.
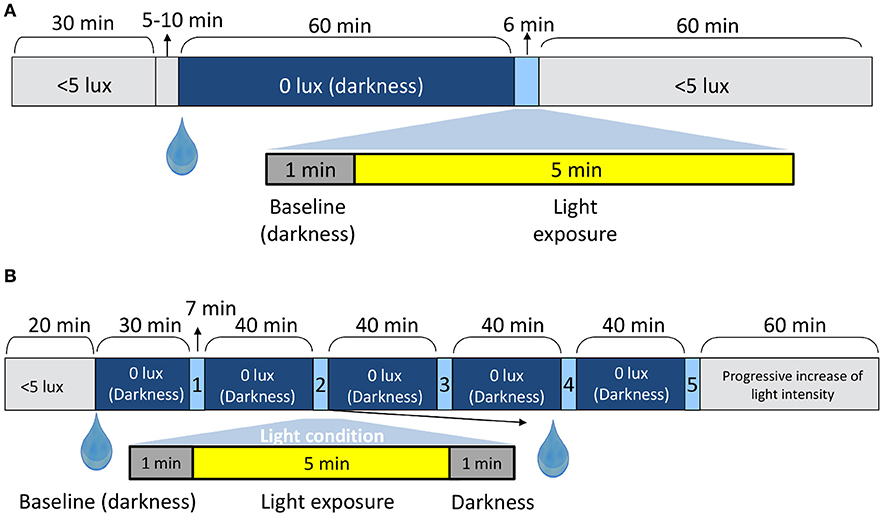
Figure 1. In-laboratory protocol for Study A (A) and Study B (B). The drop symbol shows the time when the pupil dilator was administered. The up arrows point to the duration (mins) of the shorter periods.
The protocol for light stimuli presentation and administration of tropicamide in Study B is shown in Figure 1B and has been detailed in a previous study (10). The pupillary recording followed the same protocol as described for Study A, except that pupil recording continued for 60 s after light offset.
Light Stimuli Characteristics
A 5-min light stimulus was administered to the participant's right eye (dilated) through a specially constructed Ganzfeld sphere (Apollo Lighting, Leeds, UK) coated with white reflectance paint (WRC-680 Labsphere, Pro-Lite Technology, Bedfordshire, UK) to produce patternless illumination. An ultra high-pressure mercury lamp (Focus 100LS3, 100 W, Philips Lighting, Eindhoven, The Netherlands) illuminated the sphere via a fiber optic cable connected to a light box (10, 35, 41)
In Study A, monochromatic light (purple, blue, and red) was produced using narrow bandwidth interference filters (Coherent Ealing Europe Ltd., Watford, UK) peaking at 440, 480, and 630 nm (half maximal bandwidth of 10 nm), respectively. The spectra measured at eye level, exhibited peaks at 437, 479, and 627 nm (Figure 2A), respectively, as measured by a calibrated spectrometer (Ocean Optics BV, Dunedin, Florida, USA). Light irradiances were adjusted using neutral density filters (0.10, 0.60, 0.90) (Kodak, Hemel Hempsted, UK) and were verified at the participant's eye level (cornea) using a calibrated radiometer (R203, Macam Photometrics Ltd., Livingston, Scotland) before and after each light exposure.
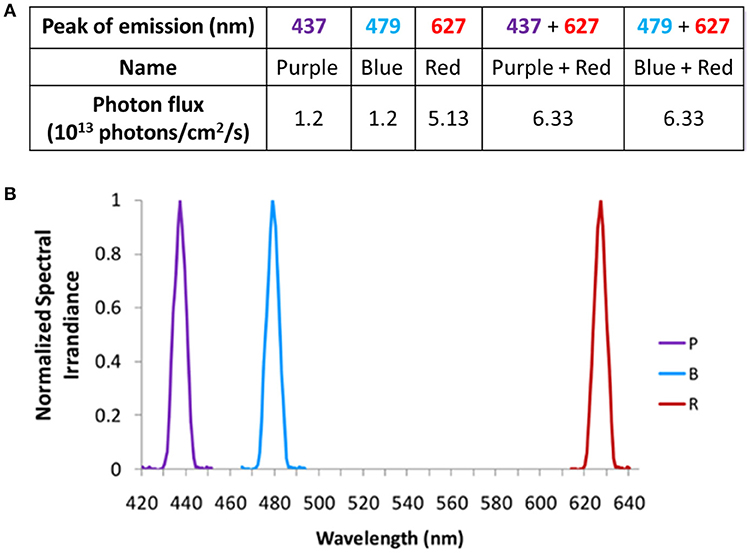
Figure 2. Light wavelengths tested in Study A and their corresponding photon fluxes (A), and normalized spectra (B).
Purple (437 nm), blue (479 nm), and red (627 nm) monochromatic lights were administered alone or in combination [monochromatic purple + monochromatic red (PR) and monochromatic blue + monochromatic red (BR)] (Figures 2A,B). Both purple and blue lights were administered at photopic light intensities of 1.2 × 1013 photons/cm2/s (13.1 log quanta/cm2/s), while 5 x 1013 photons/cm2/s (13.7 log quanta/cm2/s) was selected for red light administration (Figure 2A). The photon densities for each light stimulus were chosen based on the previously determined irradiance response curves to monochromatic light for melatonin suppression (8, 9). In addition, since bistability was demonstrated in human PLR experiments using a higher irradiance of red light (32), a similar decision was made for the current study.
In Study B, 9 × 10 nm increment monochromatic lights (420, 430, 440, 450, 460, 470, 480, 490, and 500 nm), each with a half maximal bandwidth of 7 nm, were obtained using a Bentham M300 monochromator (Figures 3A,B). Technical characteristics of this system have been previously described (10). Due to the narrower spectral range selected and the wavelength dependent grating response of the monochromator, the photon densities tested (Figure 3A) were 10-fold lower than the ones achieved in Study A (Figure 2A). The achieved photon density was approximately 11.9 log quanta/cm2/s at the level of the cornea. Considering a 0.3 correction for optical media, all the photon fluxes tested would have been applied above 11 log quanta/cm2/s, thus being above the photopic threshold (43) and within the limits for melanopsin activation (18). Identical photon fluxes could not be obtained for all the wavelengths tested due to technical limitations of the instrumentation. Some data from Study B have already been published as part of a previous study in which they were correlated with different aspects of the circadian system (10).
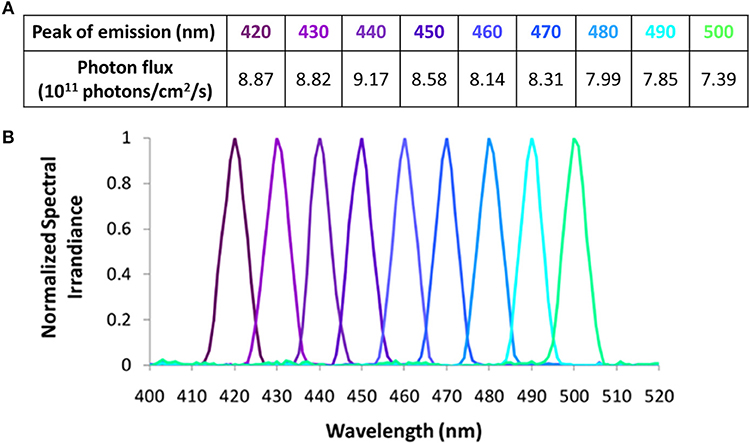
Figure 3. Light wavelengths tested in Study B and their corresponding photon fluxes (A), and normalized spectra (B).
The tested range of wavelengths were selected according to previous studies on short wavelength sensitivity of the human circadian system (8, 9, 44–46). In addition, assessing the effects of wavelengths shorter than the melanopsin λmax peak on the PLR could help to clarify the role of very short wavelength light.
Since two of the lights in Study A and B were almost identical in terms of maximum spectral emission (peak at 437 nm in Study A vs. 440 nm in Study B and peak at 479 nm in Study A vs. 480 nm in Study B), comparison between a higher (1.2 × 1013 photons/cm2/s) and a lower (8–9 × 1011 photons/cm2/s) photon density at those wavelengths was performed.
Pupil Recording
To assess the consensual PLR, a pupillometer system was used. The pupil size was tracked from the infrared illuminated (left) eye through a video pupil tracking system (ViewPoint Eye Tracker®, Arrington Research Inc., Scottsdale, AZ). The researcher helped the participants to be seated in front of the sphere in darkness, resting their forehead and chin on the pupillometer system support while the left eye was focussed by the infrared camera. The system recorded 220 data per second [see (10) for further technical details].
Data Analysis
Data Pre-processing
Pupil diameter was analyzed using software specifically designed by the Chronobiology Laboratory and the Artificial Intelligence Group at the University of Murcia (Pupilabware®), as already described (10). This processing included the determination of baseline (mean pupil diameter during the 60 s in darkness prior to the light stimulus) and normalized pupil size (NPS), i.e., ratio of the measured pupil diameter divided by the baseline pupil size.
Primary Pupil Outcome Parameters
Minimum diameter (expressed as relative maximum rapid pupil constriction), time to minimum (time required to achieve the relative maximum rapid pupil constriction), velocity of pupil constriction as and area under the curve (), where t0 is the initial time point of pupil response and t1 is the end time, 100 is the baseline pupil size, and NPS is normalized pupil size. Two AUCs were calculated: AUC0−60, corresponding to the first minute of light exposure and AUC240−300 that corresponds to the last minute of light exposure within a 5 min light stimulus. AUC was expressed as “arbitrary units” (A.U./AU) (Figure 4). Pupil diameter 6-s after light offset (6-s PIPR) was calculated in Study B.
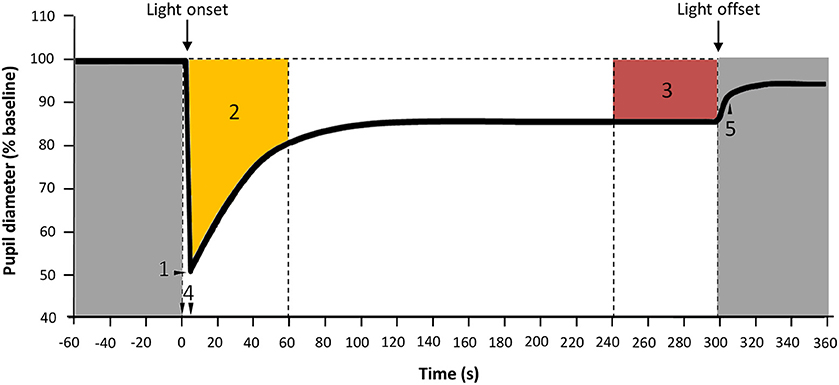
Figure 4. Parameters assessed for PLR. 1: Maximum constriction; 2: Area under the curve from 0 to 60 s of light exposure (AUC0−60, yellow); 3: Area under the curve from 240 to 300 s of light exposure (AUC240−300, red), expressed in A.U.; 4: Time from light onset to the minimum pupil diameter reached during constriction; 5: 6-s PIPR. Gray areas indicate lights off.
Statistical Analysis
All statistical analyses were carried out using SPSS 25 (SPSS Inc., Chicago, IL, USA). When not all participants received all light conditions, missing parameters were replaced by the average parameter under that light condition. For parameters that did not have a normal distribution Friedman's non-parametric test for related samples (post-hoc Wilcoxon) was performed instead of repeated measures ANOVA (Bonferroni post-hoc). The significance level was set at p < 0.05. Bonferroni correction was applied after post-hoc pairwise comparisons. When only two conditions were compared, a paired or unpaired Student's t-test (or Mann-Whitney U) was performed. All the results were expressed as mean ± standard error of the mean (SEM).
Photoreceptor Activation
The photoreceptor activation was assessed for each light condition using the Irradiance Toolbox (v1.), developed by Lucas et al. (47), that calculates the α-opic lux parameter, which in turn represents the excitation of each of the 5 photoreceptors under different light spectra. This calculation is based on the estimated sensitivity curves for each photoreceptor (47). Both absolute values (obtained directly from the toolbox) and the relative and absolute contribution for each photoreceptor were assessed.
Results
Study A
Monochromatic purple (437 nm; n = 3), blue (479 nm; n = 9), and red (627 nm; n = 8) light stimuli and the combinations “purple + red” (PR; n = 7) and “blue + red” (BR; n = 9), were tested (photon densities indicated in Figure 2A). Figures 5A,B show the average pupil recordings for each light condition. As expected, under all tested light conditions, pupil constriction reached its minimum relative diameter within the first 10-s after light onset (transient response) (Figure 5C), re-dilating in a rapid manner during the following 50-s (escape), followed by the sustained part (steady state photoequilibrium) of the PLR.
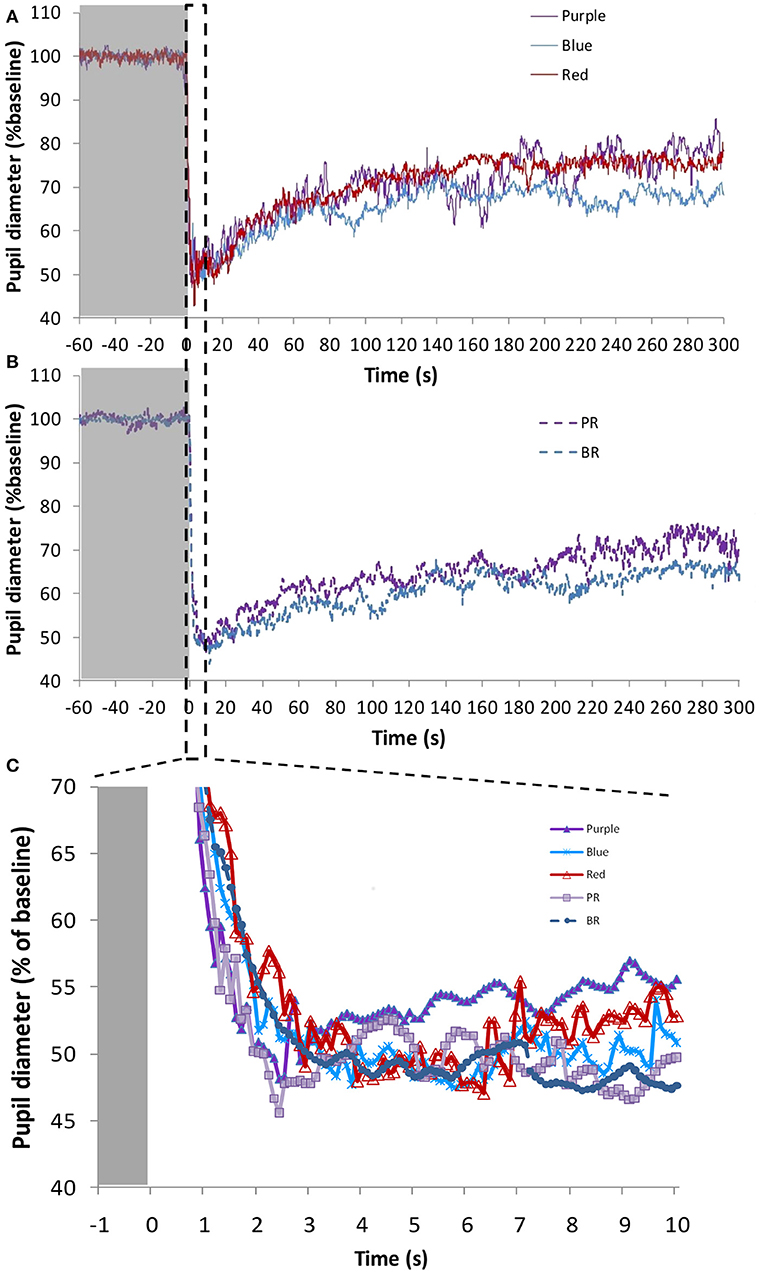
Figure 5. Average pupil recordings (A) for purple (437 nm; n = 3), blue (479 nm; n = 9), and red (627 nm; n = 8) light at 1.2 × 1013 photons/cm2/s (purple and blue), and 5.13 × 1013 photons/cm2/s (red), and (B) for purple + red (PR; n = 7) and blue + red (BR; n = 9) at 6.33 × 1013 photons/cm2/s. SEM bars have been omitted for clarity. Averaged first 10 s of pupil constriction (C) with error bars omitted for clarity.
There were no significant differences in the maximum pupil constriction following the light stimuli. Constriction under blue 479 nm light (57.5 ± 3.7%) > red 627 nm light (54.4 ± 2.3%) ≈ purple 437 nm light (54.2 ± 3.7%). Although the time needed to reach the minimum diameter tended to be shorter under the blue light condition, there were no significant differences between the different light stimuli. The velocity of pupil constriction, however, was significantly faster (one-way repeated measures ANOVA, F = 51.168, df = 2.065, p < 0.001) with blue light (27.9 ± 10.3%/s) than with the red (14.6 ± 1.7%/s) and purple (15.7 ± 1.1%/s) light (Bonferroni post-hoc test, p < 0.001).
The AUC of two PLR periods (first, AUC0−60, and last, AUC240−300, minute of light exposure) was calculated (Figure 6A), to evaluate the transient and sustained response, respectively. As expected AUC0−60 was always higher (thus, smaller diameter during the transient response) than the AUC240−300 for all light conditions (Wilcoxon post-hoc, p < 0.017). There were no significant differences in the AUC0−60 (transient response) between the light conditions, with the highest value found under the blue + red light condition (2,720 ± 223 A.U.). Similarly, in the sustained response (AUC240−300) there were no significant differences between the different light conditions (although significant overall effect, Friedman's test, χ2 = 10.8, df = 4, p = 0.029), although blue light alone or in combination tended to produce a more sustained higher amplitude response (blue, 1,908 ± 241 A.U.; blue + red, 2,076 ± 260) than purple (purple 1,350 ± 191 A.U.; purple + red 1,740 ± 222 A.U.) or red (1,456 ± 311 A.U.) light wavelengths.
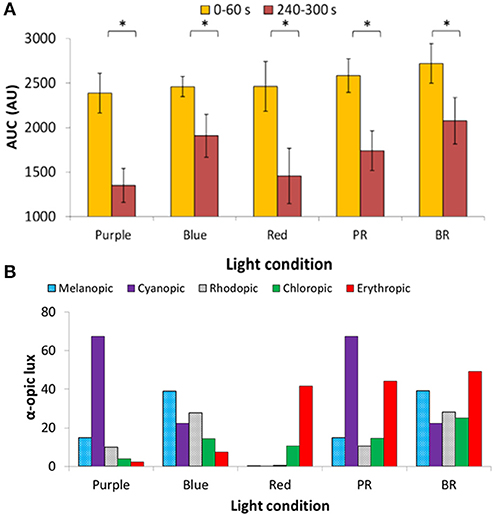
Figure 6. Area under the curve (AUC, expressed in A.U.) during two time windows: from 0 to 60 s of light exposure (0–60 s; first minute of light exposure, in yellow) and from 240 to 300 s of light exposure (240–300 s; last minute of 5-min light exposure, in red). Two-way repeated measures ANOVA showed an overall significant difference between 0–60 and 240–300 s (p < 0.05), but not between wavelengths nor interaction between both factors. Paired-sample t-tests revealed significant differences (indicated by *) between AUC0−60 and AUC240−300 under all the light conditions (p < 0.05) (A). Activation of the different photoreceptors under each light condition used, assessed by the α-opic lux parameter calculated by the Irradiance toolbox (47) (B). P indicates purple (437 nm; n = 3); B indicates blue (479 nm; n = 9); R indicates red (627 nm; n = 8); PR indicates purple + red (n = 7); and BR indicates blue + red (n = 9).
The retinal photoreceptor excitations were obtained for each light condition (Figure 6B) by calculating the α-opic lux parameter, a parameter which represents each photoreceptor excitation [Irradiance Toolbox (47)]. In the case of purple light, the highest activation was for S-cones (67.3 cyanopic lux, absolute value; 68.4% of the total), while for blue light, melanopic excitation was highest (39.0 melanopic lux, absolute value; 35.2% of the total). For red light, as expected, the predominant excitation corresponded to L-cones (41.8 erythropic lux, absolute value; 78.7% of the total) with less excitation of M-cones (10.7 chloropic lux, absolute value; 20.1% of the total). Rod activation was also highest under blue light, both alone (B) (27.7 rhodopic lux, absolute value; 25% of the total) and in combination with red light (BR) (28.2 rhodopic lux, absolute value; 17.2% of the total).
Study B
Nine monochromatic light stimuli in 10 nm increments peaking between 420 and 500 nm were tested (peaks of emission at 420 (n = 15), 430 (n = 14), 440 (n = 15), 450 (n = 15), 460 (n = 15), 470 (n = 15), 480 (n = 13), 490 (n = 14), and 500 (n = 13) nm) at the photon densities indicated in the Table (Figure 3A). The average PLR for each light condition is shown in Figure 7 (for clarity, the nine wavelengths tested have been represented in two separate graphs, Figures 7A,B). As expected, typical PLR dynamics were obtained in all cases, reaching the maximum pupil constriction within the first 10-s of light exposure (Figure 7C).
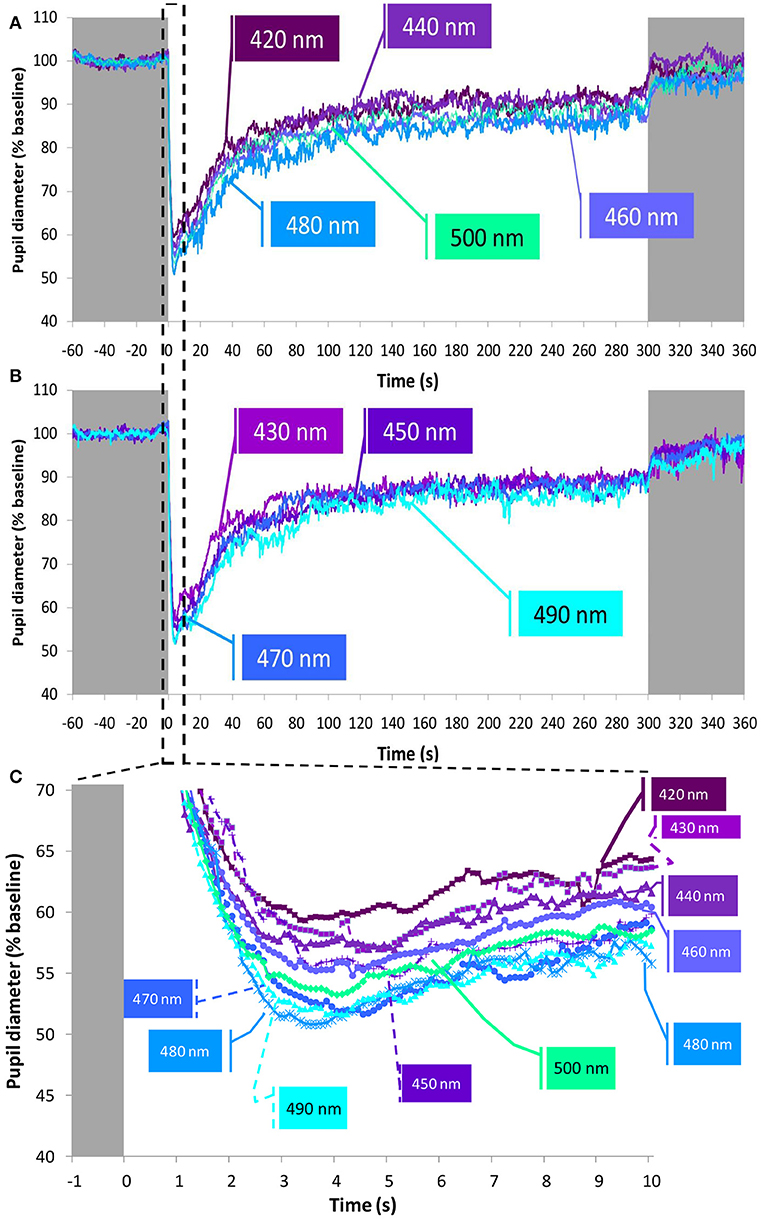
Figure 7. Average pupil recordings at 420 nm (n = 15), 440 nm (n = 15), 460 nm (n = 15), 480 nm (n = 13), and 500 nm (n = 13) light (A) and at 430 nm (n = 14), 450 nm (n = 15), 470 nm (n = 15), and 490 nm (n = 14) light (B). SEM bars have been omitted for clarity. See Methods and Figure 3A for photon flux details of the light stimuli. Averaged first 10 s of pupil constriction (C) with error bars omitted for clarity.
The maximum relative rapid pupil constriction under the light stimuli was achieved with the longer light wavelengths (one-way repeated measures ANOVA, F = 5.204, df = 5.841, p < 0.001), reaching the greatest pupil constriction at 470 nm (50.2 ± 1.6%), and the smallest constriction at 430 nm (42.7 ± 1.9%). According to pairwise comparisons (Bonferroni post-hoc, p < 0.05), only the constriction at 430 nm (not at 420 nm nor 440 nm) was significantly smaller than that found at 470 nm (50.2 ± 1.6%), 480 nm (49.9 ± 1.8%), 490 nm (49.4 ± 1.8%), and 500 nm (49.7 ± 1.2 %).
It took less time to reach the minimum pupil diameter with 480 nm (3.7 ± 0.2 s) and 490 nm (3.8 ± 0.4 s) light than with 420 nm (4.6 ± 0.5 s) light, although these differences were not statistically significant. However, the velocity of constriction was different (Friedman test, χ2 = 29.417, df = 8, p < 0.001) with the 480 nm light stimulus eliciting the most rapid pupil constriction (14.3 ± 0.9%/s), while the slowest pupil constriction was observed with 430 nm light (9.9 ± 1.1%/s) (Wilcoxon post-hoc, p = 0.002).
Regarding the AUC (A.U.) during the transient (first minute of light exposure, AUC0−60), and sustained response (last minute of light exposure, AUC240−300) (Figure 8A), again as expected, AUC0−60 was always greater than AUC240−300 for all light conditions and wavelengths (Wilcoxon post-hoc, p = 0.001), although an interaction between both factors was evident (p = 0.032). Accordingly, transient and sustained responses were analyzed separately. The transient response (AUC0−60) tended to increase from 420 nm (1,517 ± 141 A.U.) to 490 nm (2,007 ± 147 A.U.), decreasing again at 500 nm (1,778 ± 108 A.U.) (one-way repeated measures ANOVA, F = 4.861, df = 8, p < 0.001). By contrast the greatest AUC240−300 occurred with 460 nm (880 ± 131 A.U.) and 480 nm (838 ± 100 A.U.) light (Friedman test, χ2 = 17.102, df = 8, p = 0.029), the latter being significantly different when compared to the 420 nm light stimulus (lowest, with 567 ± 100 A.U.) (Wilcoxon post-hoc, p = 0.005).
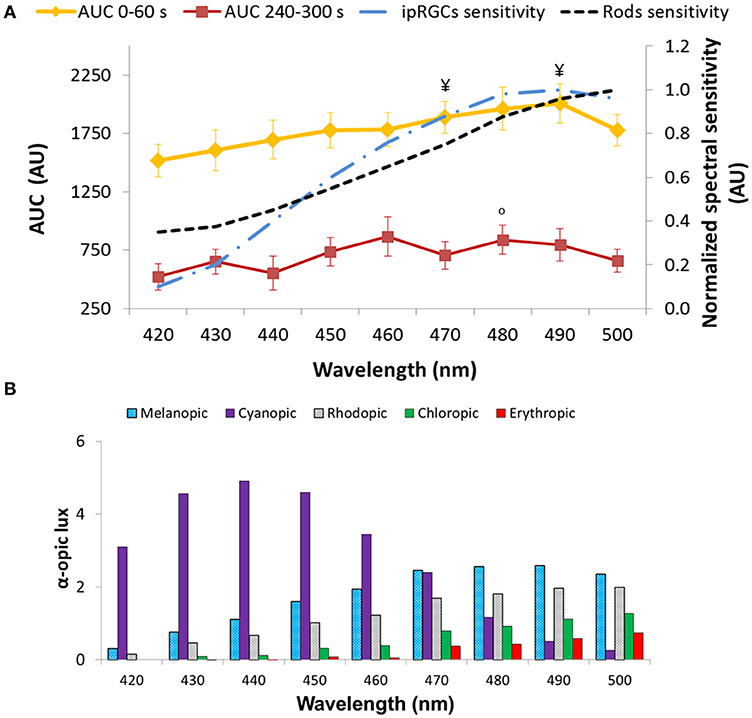
Figure 8. Area under the curve (AUC, expressed in A.U.) over two time windows: from 0 to 60 s of light exposure (0–60 s; first minute of light exposure, in yellow) and from 240 to 300 s of light exposure (240–300 s; last minute of light exposure, in red). Paired-sample t-tests revealed significant differences between AUC0−60 and AUC240−300 for all the wavelengths tested (p < 0.05). Two-way repeated measures ANOVA showed an overall significant difference between 0–60 and 240–300 s (p < 0.001), wavelength (p < 0.001), and a significant interaction between both factors for all light conditions. ¥ indicates statistically significant differences (Wilcoxon post-hoc, p ≤ 0.001) in AUC0−60 vs. 430 nm. °indicates a statistically significant differences (Wilcoxon post-hoc, p < 0.005) in AUC240−300 vs. 420 nm. Plots of the ipRGC and rod normalized sensitivities are superimposed (A). Activation of the different photoreceptors according to each light tested, determined by the α-opic lux parameter calculated by the Irradiance toolbox (47) (B).
The 6-s PIPR could only be measured in Study B, since no recording of the post-illumination response was performed in Study A. The pupil constriction 6 s after the end of light stimulus was greater at the longer wavelengths within the 420–500 nm range (Friedman test, χ2 = 17.227, df = 8, p = 0.028), being maximum at 490 nm (6.82 ± 1.88%) vs. the minima at 420 (1.61 ± 1.93%) (Wilcoxon post-hoc, p = 0.001) and 440 nm (1.37 ± 1.82%).
Retinal photoreceptor activation was assessed [Irradiance Toolbox, (47)] as shown in Figure 8B. Melanopic activation was lowest when exposed to 420 nm (0.3 melanopic lux, absolute value; 8.7%), increasing at every exposure, reaching its peak at 490 nm light (2.6 melanopic lux, absolute value; 38.2%), after which it decreased again at 500 nm (2.4 melanopic lux, absolute value; 35.5%), thus showing the same pattern as the transient response (AUC0−60) (R = 0.924, p < 0.01). There was also a significant correlation between the sustained response (AUC240−300) and melanopic activation (R = 0.699, p = 0.036).
Photon Flux Comparison
The effect of light intensity on the PLR was compared for purple (~440 nm) and blue (~480 nm) light considering the highest (Study A, 1.2 × 1013 photons/cm2/s or ~13 log quanta/cm2/s) and lowest (Study B, 8 × 1011 and 9.2 × 1011 photons/cm2/s, respectively or ~12 log quanta/cm2/s) photon fluxes.
Figure 9 represents the average PLR recording for each wavelength (Figure 9A, purple light; Figure 9B, blue light) at ~13 log quanta/cm2/s and ~12 log quanta/cm2/s photon fluxes. As expected, higher photon fluxes produced a greater transient and sustained pupillary constriction for both wavelengths than lower photon fluxes. The rapid pupil constriction was greater at higher compared to lower photon fluxes for both purple (54.2 ± 3.7 vs. 46.8 ± 2.3%, differences not statistically significant) and blue (57.5 ± 3.7 vs. 49.9 ± 1.8%, Mann-Whitney U test, Z = −2.147, p = 0.032) lights (Figure 9, central panel). Also as expected, it tended to take less time to reach the minimum pupil diameter under higher compared to lower photon flux for both purple (3.75 ± 0.62 vs. 4.10 ± 0.40 s) and blue (3.58 ± 1.36 vs. 3.74 ± 0.24 s) light conditions, although the differences did not reach statistical significance.
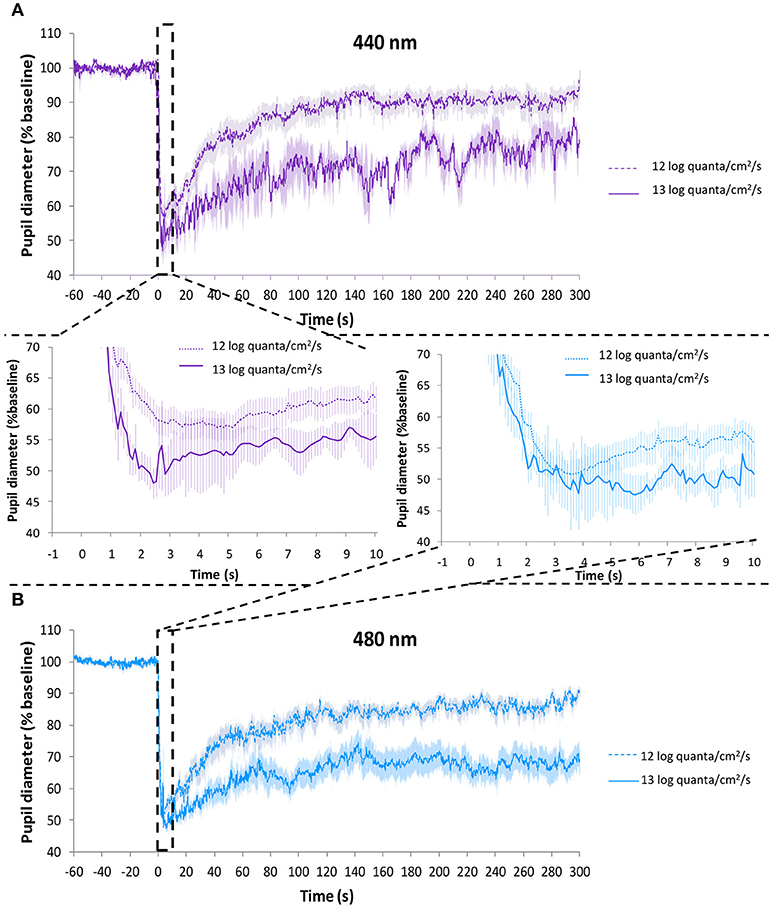
Figure 9. Average PLR recordings under purple light (~440 nm) at 9.2 × 1011 photons/cm2/s (~12 log quanta/cm2/s, discontinuous line) and 1.2 × 1013 photons/cm2/s (~13 log quanta/cm2/s, continuous line) (A). Average PLR recordings under blue light (~480 nm) at 8.0 × 1011 photons/cm2/s (~12 log quanta/cm2/s, discontinuous line) and 1.2 × 1013 photons/cm2/s (~13 log quanta/cm2/s, continuous line) (B). Error bars indicate SEM. Averaged first 10 s of pupil constriction are shown in the central panel.
The integrative parameter “velocity of constriction” was significantly faster under higher photon fluxes for blue light (27.9 ± 10.3 higher vs. 14.3 ± 0.9%/s lower photon flux, p = 0.035). Purple light also tended to be faster, although statistical significance was not reached (15.7 ± 1.1 higher vs. 12.2 ± 1.4 %/s lower photon flux, p = 0.207). The velocity of pupil constriction was significantly affected by both wavelength (one-way repeated measures ANOVA, F = 5.007, df = 1, p = 0.038) and photon flux (F = 5.466, df = 1, p = 0.031), interaction between these factors was not significant (one-way mixed design ANOVA).
Both the transient (AUC0−60; Figure 10A) and sustained (AUC240−300; Figure 10B) responses were greater under higher photon fluxes for both the purple and blue light conditions (Mann-Whitney U-test, Z = −2.371, p < 0.016).
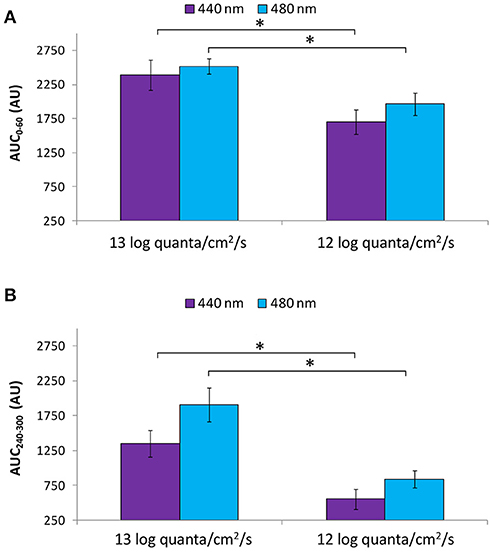
Figure 10. Area under the curve (AUC, expressed in A.U.) over two time windows: from 0 to 60 s of light exposure (0–60 s; first minute of light exposure) (A) and from 240 to 300 s of light exposure (240–300 s; last minute of light exposure) (B) at higher (~13 log quanta/cm2/s) and lower (~12 log quanta/cm2/s) intensity purple (440 nm) and blue (480 nm) light. Error bars indicate SEM. *statistically significant differences (Mann Whitney U-test, p < 0.016).
Discussion
We aimed to better characterize the human PLR under high resolution, 5-min monochromatic light stimuli (10 nm increments) alone and in combination. Our results show higher responsiveness of the pupil to blue light stimuli, alone or in combination with red light, considering rapid pupil constriction, transient, sustained and post-illumination pupil responses, and velocity of pupil constriction. Higher light intensities also produced, as expected, higher responsiveness.
Traditionally when evaluating the PLR, single monochromatic lights have been tested. Some studies, however, have used combined monochromatic light stimuli (LEDs of various bandwidths) or spectrally tunable light sources, that have provided good evidence to understand the contributions and interactions of the retinal photoreceptors in the PLR (13, 15, 16, 20, 48–54), as well as regarding light-induced melatonin suppression (35). In the present study blue and purple monochromatic lights were combined with red at the same final intensity. In agreement with previous reports (32, 55) the smallest pupil diameter tended to occur in the presence of blue light (~480 nm alone or combined with red). According to the hypothesis of bistability, melanopsin may switch from a M state (not responsive to 480 nm light) to a R state (responsive to 480 nm light) by absorbing longer wavelength photons (32, 56, 57), the retinal epithelium being required for melanopsin regeneration (58). Thus, it may be plausible that red light (627 nm), when present, could elicit this conformational change, increasing the sustained response, not only due to the increased light intensity, but also to its effect on melanopsin. Although the differences did not reach statistical significance, red light tended to produce a greater effect in combination with purple light (compared to purple light alone) than with blue light (compared to blue light alone), probably because blue light can elicit the maximal intrinsic photoresponse on its own, while with purple 440 nm light, although the melanopsin is stimulated, its activation is not maximal (45, 59). This tendency, however, could also be explained by the previously described spectral opponency between S-cones and both L- cones and ipRGCs (49, 50, 54). Thus, red (activating L-cones) plus blue (activating melanopsin) light would produce a summation in the pupil constriction (13), while the combination red plus purple light would activate L- and S-cones, respectively, thus producing opponency instead of summation, resulting in smaller constriction amplitude (49, 50, 54). Other studies, however, suggest linear/non-linear summation of the 5 types of photoreceptors stimulation (51), which contradicts the opponency hypothesis. Although both light combinations were delivered at the same photon flux, the differences in pupil response due to different colors (driven by chromatic pathways) have been previously described as being 3-fold larger than those driven by luminance pathways (16).
In our study, the velocity of pupil constriction was significantly faster under blue light than under red and purple light stimuli. However, based on previous studies, the melanopsin-ipRGCs intrinsic photoresponse would produce slow and sustained pupil constrictions, while the extrinsic pathway (mainly cone-driven) would produce fast and relatively transient responses (22, 25). The findings with blue light are thus not in agreement with the expected slow response produced by the melanopsin-ipRGCs (22, 25). The delayed response previously attributed to melanopsin-ipRGCs has also been questioned in a study using a square-wave pulse, suggesting a mechanism possibly associated with cone-mediated signals (20). In addition, we could speculate that an overlapping action of S-cones may produce this more rapid response, although considering the previously described S-cone opponency, this may not be a plausible explanation (49, 50, 54).
The post-illumination pupil response (PIPR) after short light stimuli (e.g., 1-s) has been suggested as a good marker for estimating the melanopsin function (12). However, in this study we used longer duration light stimuli (5-min) in order to assess the overall PLR dynamics. Thus in order to assess the contribution of each photoreceptor we used mathematical modeling [Irradiance Toolbox (v1) application, developed by Lucas et al. (47)], which provided the theoretical photoreceptor activation under each light condition. From these results we observed that under blue light stimuli there appears to be a summation of intrinsic (melanopsin-ipRGCs) and extrinsic (rods and cones) activation, which may explain the faster pupil constriction under blue light than under red or purple light stimuli, since the latter mainly involves cone activation with little intrinsic melanopsin activation.
When evaluating the PLR under a high resolution wavelength range (namely every 10 nm) from 420 to 500 nm, the highest pupil responsiveness was observed between 470–490 nm (depending on the PLR parameter analyzed). When looking at the transient response (AUC0−60) a progressive increase (i.e., greater pupillary constriction) was observed from 420 to 490 nm. These PLR results are in agreement with Gooley et al. (28), who found in a blind subject with degeneration of the outer retina while the inner retina remained intact, that pupillary constriction was short-wavelength sensitive with a fitted peak sensitivity of 490 nm. The shorter (purple) wavelengths (420–440 nm) would activate S-cones so the lower amplitude pupil response found with these wavelengths may also be due to spectral opponency (49, 50, 54), these lights thus producing less constriction than the longer wavelengths with melanopsin activation. The 6-s PIPR parameter, calculated after 5-min light stimuli, also showed greater constriction (smaller diameters) with longer wavelengths, again the maximum pupil constriction being at 490 nm. 6-s PIPR has previously been found to be a good marker for melanopsin activation after 1-s light stimuli (12), so for the first time, as far as we know, this parameter has been calculated after longer light exposures. Our results also support the hypothesis that ipRGCs contribute significantly, not only to the pupillary sustained response (AUC240−300) (23, 28) (which tended to be greater at 460 nm), but also to the transient part of the reflex (AUC0−60). The transient responses showing a similar pattern to the theoretical melanopic activation further supports this idea.
The role of rods, however, cannot be excluded since rods (i) have been found to contribute to the sustained response (23) [as well as to the PIPR (24)], (ii) have a peak of sensitivity at 498 nm (60), and (iii) are partially activated under 480 nm light (as presented in Figure 8B). Cones, however, according to previous studies, contribute little to the sustained response (23, 28), since they quickly adapt to long duration light stimuli.
The PLR has been widely shown to increase with higher light intensities (18, 61, 62). In our studies (A and B) the light stimuli were similar, allowing us to compare very close wavelengths at different photon fluxes (13.08 vs. 11.93 log quanta/cm2/s from Study A and B, respectively). Thus, at ~440 and ~480 nm, as expected, higher photon fluxes produced a greater sustained pupillary response at both wavelengths and faster velocity of pupil constriction. Overall these results are in accordance with a higher contribution of ipRGCs at higher photon fluxes (since their activation threshold is higher) and at longer duration light stimuli (23), while cone photoreceptors would contribute to non-visual light responses at the beginning of light exposure (63).
Although pupillometry has become a useful tool to evaluate non-visual light responses, it is not problem free. The PLR has been shown to depend on wake and circadian phase (64). Thus, experiments need to be controlled for time of day, wake up time and circadian phase of the participants. In the present study performing experiments during the morning at the same clock time for each participant, as well as controlling the sleep/wake cycle of the participants 7 days prior to the PLR assessments, was designed to minimize the differences between different days, time of day and wake status. PLR could also be influenced by other processes such as changes in accommodation states, in the state of arousal or even cognitive activity (23), thus even when participants are instructed to refrain from alcohol, caffeinated drinks, bright lights, excessive exercise, and non-steroidal anti-inflammatory drug intake as in the present study, there may be additional confounding factors. Despite these limitations, a close association between the observed pupillary responses and the melatonin suppression response with monochromatic lights has been reported (9, 35, 41, 46). In addition, a relationship between circadian status and PLR has been recently proposed (10). Overall this suggests that pupillometry may, in future with more evidence from different approaches, become a practical tool to evaluate the efficiency of light sources on circadian system activation in a quick, non-invasive, and relatively inexpensive way [reviewed in (65)].
Thus, if we consider pupillometry as a proxy to evaluate circadian effects of light and considering that monochromatic blue light is most effective at suppressing melatonin (8, 9, 44), we propose that substitution of blue light by purple light in polychromatic light sources may be a solution for nocturnal illumination to minimize non-visual light responses. In order to test this it will be necessary to determine, not only whether the color discrimination is acceptable under these spectra, but also the specific effects of purple light on melatonin synthesis, sleep and alertness in humans, not only isolated, (9, 46) or in combination with blue (66) or red (35) light, but also by replacing blue light within more complex light spectra (67). In this sense, further studies about the potential risks of using purple light at the intensities required should also be conducted.
Author Contributions
MB-C, KH, SS, VR, JM, MR, and DS conception and design of the study. MB-C, KH, CI, and VR data acquisition. MB-C, VR, JM, and MR data analysis. MB-C, JM, MR, and DS wrote first draft of manuscript. All authors contributed to manuscript revision, read and approved the submitted version.
Funding
This work was supported by the Instituto de Salud Carlos III, the Ministry of Economy and competitiveness through a CIBERFES grant (CB16/10/00239), and grant 19899/GERM/15 awarded to JM (co financed by FEDER). Research fellowship granted to MB-C [AP2009 1051] awarded by Spanish Ministry of Education and Science and [20401/SF/17] by Fundación Séneca. The work was partly funded by a UK EPSRC MILES grant [EP/1000992/1]. SS gratefully acknowledges EPSRC Leadership Fellowship funding under project [EP/H005587/1]. DS is a Royal Society Wolfson Research Merit Award holder.
Conflict of Interest Statement
VR is a scientific advisor to Lumie.
The remaining authors declare that the research was conducted in the absence of any commercial or financial relationships that could be construed as a potential conflict of interest.
Acknowledgments
We thank Dr. Benita Middleton for her assistance with administration of the pupil dilator.
References
1. Provencio I, Jiang G, De Grip WJ, Hayes WP, Rollag MD. Melanopsin: an opsin in melanophores, brain, and eye. Proc Natl Acad Sci USA. (1998) 95:340–5.
2. Berson DM, Dunn FA, Takao M. Phototransduction by retinal ganglion cells that set the circadian clock. Science (2002) 295:1070–3. doi: 10.1126/science.1067262
3. Berson DM. Strange vision: ganglion cells as circadian photoreceptors. Trends Neurosci. (2003) 26:314–20. doi: 10.1016/S0166-2236(03)00130-9
4. Gooley JJ, Lu J, Fischer D, Saper CB. A broad role for melanopsin in nonvisual photoreception. J Neurosci. (2003) 23:7093–106. doi: 10.1523/JNEUROSCI.23-18-07093.2003
5. Hannibal J, Fahrenkrug J. Target areas innervated by PACAP-immunoreactive retinal ganglion cells. Cell Tissue Res. (2004) 316:99–113. doi: 10.1007/s00441-004-0858-x
6. Hattar S, Liao HW, Takao M, Berson DM, Yau KW. Melanopsin-containing retinal ganglion cells: architecture, projections, and intrinsic photosensitivity. Science (2002) 295:1065–70. doi: 10.1126/science.1069609
7. Hattar S, Kumar M, Park A, Tong P, Tung J, Yau K-W, et al. Central projections of melanopsin-expressing retinal ganglion cells in the mouse. J Comp Neurol. (2006) 497:326–49. doi: 10.1002/cne.20970
8. Brainard GC, Hanifin JP, Greeson JM, Byrne B, Glickman G, Gerner E, et al. Action spectrum for melatonin regulation in humans: evidence for a novel circadian photoreceptor. J Neurosci. (2001) 21:6405–12. doi: 10.1523/JNEUROSCI.21-16-06405.2001
9. Thapan K, Arendt J, Skene DJ. An action spectrum for melatonin suppression: evidence for a novel non-rod, non-cone photoreceptor system in humans. J Physiol. (2001) 535:261–7. doi: 10.1111/j.1469-7793.2001.t01-1-00261.x
10. Bonmati-Carrion MA, Hild K, Isherwood C, Sweeney SJ, Revell VL, Skene DJ, et al. Relationship between human pupillary light reflex and circadian system status. PLoS ONE (2016) 11:e0162476. doi: 10.1371/journal.pone.0162476
11. Löwenstein O. Pupillographic studies. Arch Ophthalmol. (1942) 27:969. doi: 10.1001/archopht.1942.00880050139010
12. Adhikari P, Zele AJ, Feigl B. The Post-Illumination Pupil Response (PIPR). Invest Ophthalmol Vis Sci. (2015) 56:3838–49. doi: 10.1167/iovs.14-16233
13. Barrionuevo PA, Nicandro N, McAnany JJ, Zele AJ, Gamlin P, Cao D. Assessing rod, cone, and melanopsin contributions to human pupil flicker responses. Invest Ophthalmol Vis Sci. (2014) 55:719–27. doi: 10.1167/iovs.13-13252
14. Joyce DS, Feigl B, Zele AJ. Melanopsin-mediated post-illumination pupil response in the peripheral retina. J Vis. (2016) 16:5. doi: 10.1167/16.8.5
15. Tsujimura S, Wolffsohn JS, Gilmartin B. A linear chromatic mechanism drives the pupillary response. Proc Biol Sci. (2001) 268:2203–9. doi: 10.1098/rspb.2001.1775
16. Tsujimura S, Wolffsohn JS, Gilmartin B. Pupil response to color signals in cone-contrast space. Curr Eye Res. (2006) 31:401–8. doi: 10.1080/02713680600681327
17. Kawasaki A, Kardon RH. Intrinsically photosensitive retinal ganglion cells. J Neuroophthalmol. (2007) 27:195–204. doi: 10.1097/WNO.0b013e31814b1df9
18. Gamlin PDR, McDougal DH, Pokorny J, Smith VC, Yau K-W, Dacey DM. Human and macaque pupil responses driven by melanopsin-containing retinal ganglion cells. Vision Res. (2007) 47:946–54. doi: 10.1016/j.visres.2006.12.015
19. Feigl B, Zele AJ. Melanopsin-expressing intrinsically photosensitive retinal ganglion cells in retinal disease. Optom Vis Sci. (2014) 91:894–903. doi: 10.1097/OPX.0000000000000284
20. Tsujimura S, Tokuda Y. Delayed response of human melanopsin retinal ganglion cells on the pupillary light reflex. Ophthalmic Physiol Opt. (2011) 31:469–79. doi: 10.1111/j.1475-1313.2011.00846.x
21. Drouyer E, Rieux C, Hut RA, Cooper HM. Responses of suprachiasmatic nucleus neurons to light and dark adaptation: relative contributions of melanopsin and rod-cone inputs. J Neurosci. (2007) 27:9623–31. doi: 10.1523/JNEUROSCI.1391-07.2007
22. Wong KY, Dunn FA, Graham DM, Berson DM. Synaptic influences on rat ganglion-cell photoreceptors. J Physiol. (2007) 582:279–96. doi: 10.1113/jphysiol.2007.133751
23. McDougal DH, Gamlin PD. The influence of intrinsically-photosensitive retinal ganglion cells on the spectral sensitivity and response dynamics of the human pupillary light reflex. Vision Res. (2010) 50:72–87. doi: 10.1016/j.visres.2009.10.012
24. Adhikari P, Feigl B, Zele AJ. Rhodopsin and melanopsin contributions to the early redilation phase of the post-illumination pupil response (PIPR). PLoS ONE (2016) 11:e0161175. doi: 10.1371/journal.pone.0161175
25. Joyce DS, Feigl B, Cao D, Zele AJ. Temporal characteristics of melanopsin inputs to the human pupil light reflex. Vision Res. (2015) 107:58–66. doi: 10.1016/j.visres.2014.12.001
26. Neitz J, Neitz M. The genetics of normal and defective color vision. Vision Res. (2011) 51:633–51. doi: 10.1016/j.visres.2010.12.002
27. Provencio I, Rodriguez IR, Jiang G, Hayes WP, Moreira EF, Rollag MD. A novel human opsin in the inner retina. J Neurosci. (2000) 20:600–5. doi: 10.1523/JNEUROSCI.20-02-00600.2000
28. Gooley JJ, Mien IH, Hilaire MAS, Yeo S, Chua EC, van Reen E, et al. Melanopsin and rod – cone photoreceptors play different roles in mediating pupillary light responses during exposure to continuous light in humans. J Neurosci. (2012) 32:14242–53. doi: 10.1523/JNEUROSCI.1321-12.2012
29. Vartanian GV, Li BY, Chervenak AP, Walch OJ, Pack W, Ala-Laurila P, et al. Melatonin suppression by light in humans is more sensitive than previously reported. J Biol Rhythms (2015) 30:351–4. doi: 10.1177/0748730415585413
30. Koenig D, Hofer H. The absolute threshold of cone vision. J Vis. (2011) 11:21. doi: 10.1167/11.1.21
31. Mure LS, Rieux C, Hattar S, Cooper HM. Melanopsin-dependent nonvisual responses: evidence for photopigment bistability in vivo. J Biol Rhythm (2007) 22:411–24. doi: 10.1177/0748730407306043
32. Mure LS, Cornut P-L, Rieux C, Drouyer E, Denis P, Gronfier C, et al. Melanopsin bistability: a fly's eye technology in the human retina. PLoS ONE (2009) 4:e5991. doi: 10.1371/journal.pone.0005991
33. Emanuel AJ, Do MTH. Melanopsin tristability for sustained and broadband phototransduction. Neuron (2015) 85:1043–55. doi: 10.1016/j.neuron.2015.02.011
34. Mawad K, van Gelder RN. Absence of long-wavelength photic potentiation of murine intrinsically photosensitive retinal ganglion cell firing in vitro. J Biol Rhythms (2008) 23:387–91. doi: 10.1177/0748730408323063
35. Papamichael C, Skene DJ, Revell VL. Human nonvisual responses to simultaneous presentation of blue and red monochromatic light. J Biol Rhythms (2012) 27:70–8. doi: 10.1177/0748730411431447
36. Wong KY, Dunn FA, Berson DM. Photoreceptor adaptation in intrinsically photosensitive retinal ganglion cells. Neuron (2005) 48:1001–10. doi: 10.1016/J.NEURON.2005.11.016
37. Walker MT, Brown RL, Cronin TW, Robinson PR. Photochemistry of retinal chromophore in mouse melanopsin. Proc Natl Acad Sci USA. (2008) 105:8861–5. doi: 10.1073/pnas.0711397105
38. Sonoda T, Lee SK. A novel role for the visual retinoid cycle in melanopsin chromophore regeneration. J Neurosci. (2016) 36:9016–8. doi: 10.1523/JNEUROSCI.1883-16.2016
39. Buysse DJ, Reynolds CF III Monk TH, Berman SR, Kupfer DJ. The Pittsburgh sleep quality index: a new instrument for psychiatric practice and research. Psychiatry Res. (1989) 28:193–213.
40. Horne JA, Östberg O. A self-assessment questionnaire to determine morningness-eveningness in human circadian rhythms. Int J Chronobiol. (1976) 4:97–110.
41. Revell VL, Skene DJ. Light-induced melatonin suppression in humans with polychromatic and monochromatic light. Chronobiol Int. (2007) 24:1125–37. doi: 10.1080/07420520701800652
42. Joyce DS, Feigl B, Zele AJ. The effects of short-term light adaptation on the human post-illumination pupil response. Investig Opthalmology Vis Sci. (2016) 57:5672. doi: 10.1167/iovs.16-19934
43. Dacey DM, Liao H-W, Peterson BB, Robinson FR, Smith VC, Pokorny J, et al. Melanopsin-expressing ganglion cells in primate retina signal colour and irradiance and project to the LGN. Nature (2005) 433:749–54. doi: 10.1038/nature03387
44. Lockley SW, Brainard GC, Czeisler CA. High sensitivity of the human circadian melatonin rhythm to resetting by short wavelength light. J Clin Endocrinol Metab. (2003) 88:4502–5. doi: 10.1210/jc.2003-030570
45. Zaidi FH, Hull JT, Peirson SN, Wulff K, Aeschbach D, Gooley JJ, et al. Short-wavelength light sensitivity of circadian, pupillary, and visual awareness in humans lacking an outer retina. Curr Biol. (2007) 17:2122–8. doi: 10.1016/j.cub.2007.11.034
46. Brainard GC, Sliney D, Hanifin JP, Glickman G, Byrne B, Greeson JM, et al. Sensitivity of the human circadian system to short-wavelength (420-nm) light. J Biol Rhythms (2008) 23:379–86. doi: 10.1177/0748730408323089
47. Lucas RJ, Peirson SN, Berson DM, Brown TM, Cooper HM, Czeisler CA, et al. Measuring and using light in the melanopsin age. Trends Neurosci. (2014) 37:1–9. doi: 10.1016/j.tins.2013.10.004
48. Viénot F, Bailacq S, Rohellec JL. The effect of controlled photopigment excitations on pupil aperture. Ophthalmic Physiol Opt. (2010) 30:484–91. doi: 10.1111/j.1475-1313.2010.00754.x
49. Spitschan M, Jain S, Brainard DH, Aguirre GK. Opponent melanopsin and S-cone signals in the human pupillary light response. Proc Natl Acad Sci USA. (2014) 111:15568–72. doi: 10.1073/pnas.1400942111
50. Cao D, Nicandro N, Barrionuevo PA. A five-primary photostimulator suitable for studying intrinsically photosensitive retinal ganglion cell functions in humans. J Vis. (2015) 15:15.1.27. doi: 10.1167/15.1.27
51. Barrionuevo PA, Cao D. Luminance and chromatic signals interact differently with melanopsin activation to control the pupil light response. J Vis. (2016) 16:29. doi: 10.1167/16.11.29
52. Spitschan M, Bock AS, Ryan J, Frazzetta G, Brainard DH, Aguirre GK. The human visual cortex response to melanopsin-directed stimulation is accompanied by a distinct perceptual experience. Proc Natl Acad Sci USA. (2017) 114:12291–6. doi: 10.1073/pnas.1711522114
53. Murray IJ, Kremers J, McKeefry D, Parry NRA. Paradoxical pupil responses to isolated M-cone increments. J Opt Soc Am A (2018) 35:B66. doi: 10.1364/JOSAA.35.000B66
54. Woelders T, Leenheers T, Gordijn MCM, Hut RA, Beersma DGM, Wams EJ. Melanopsin- and L-cone-induced pupil constriction is inhibited by S- and M-cones in humans. Proc Natl Acad Sci USA. (2018) 115:792–7. doi: 10.1073/pnas.1716281115
55. Park JC, Moura AL, Raza AS, Rhee DW, Kardon RH, Hood DC. Toward a clinical protocol for assessing rod, cone, and melanopsin contributions to the human pupil response. Invest Ophthalmol Vis Sci. (2011) 52:6624–35. doi: 10.1167/iovs.11-7586
56. Melyan Z, Tarttelin EE, Bellingham J, Lucas RJ, Hankins MW. Addition of human melanopsin renders mammalian cells photoresponsive. Nature (2005) 433:741–5. doi: 10.1038/nature03344
57. Koyanagi M, Kubokawa K, Tsukamoto H, Shichida Y, Terakita A. Cephalochordate melanopsin: evolutionary linkage between invertebrate visual cells and vertebrate photosensitive retinal ganglion cells. Curr Biol. (2005) 15:1065–9. doi: 10.1016/j.cub.2005.04.063
58. Zhao X, Pack W, Khan NW, Wong KY. Prolonged inner retinal photoreception depends on the visual retinoid cycle. J Neurosci. (2016) 36:4209–17. doi: 10.1523/JNEUROSCI.2629-14.2016
59. Bailes HJ, Lucas RJ. Human melanopsin forms a pigment maximally sensitive to blue light (λmax ≈ 479 nm) supporting activation of G(q/11) and G(i/o) signalling cascades. Proc Biol Sci. (2013) 280:20122987. doi: 10.1098/rspb.2012.2987
60. Bowmaker JK, Dartnall HJ. Visual pigments of rods and cones in a human retina. J Physiol. (1980) 298:501–11.
61. Kardon R, Anderson SC, Damarjian TG, Grace EM, Stone E, Kawasaki A. Chromatic pupil responses: preferential activation of the melanopsin-mediated versus outer photoreceptor-mediated pupil light reflex. Ophthalmology (2009) 116:1564–73. doi: 10.1016/j.ophtha.2009.02.007
62. Herbst K, Sander B, Lund-Andersen H, Broendsted AE, Kessel L, Hansen MS, et al. Intrinsically photosensitive retinal ganglion cell function in relation to age: a pupillometric study in humans with special reference to the age-related optic properties of the lens. BMC Ophthalmol. (2012) 12:4. doi: 10.1186/1471-2415-12-4
63. Alpern M, Campbell FW. The spectral sensitivity of the consensual light reflex. J Physiol. (1962) 164:478–507. doi: 10.1113/jphysiol.1962.sp007033
64. Münch M, Léon L, Crippa SV, Kawasaki A. Circadian and wake-dependent effects on the pupil light reflex in response to narrow-bandwidth light pulses. Invest Ophthalmol Vis Sci. (2012) 53:4546–55. doi: 10.1167/iovs.12-9494
65. Bonmati-Carrion MA, Arguelles-Prieto R, Martinez-Madrid MJ, Reiter R, Hardeland R, Rol MA, et al. Protecting the melatonin rhythm through circadian healthy light exposure. Int J Mol Sci. (2014) 15:23448–500. doi: 10.3390/ijms151223448
66. Revell VL, Barrett DCG, Schlangen LJM, Skene DJ. Predicting human nocturnal nonvisual responses to monochromatic and polychromatic light with a melanopsin photosensitivity function. Chronobiol Int. (2010) 27:1762–77. doi: 10.3109/07420528.2010.516048
Keywords: pupillometry, light, pupillary light reflex, ipRGC, melanopsin, human melanopic lux
Citation: Bonmati-Carrion MA, Hild K, Isherwood CM, Sweeney SJ, Revell VL, Madrid JA, Rol MA and Skene DJ (2018) Effect of Single and Combined Monochromatic Light on the Human Pupillary Light Response. Front. Neurol. 9:1019. doi: 10.3389/fneur.2018.01019
Received: 08 August 2018; Accepted: 12 November 2018;
Published: 29 November 2018.
Edited by:
Paul Gamlin, University of Alabama at Birmingham, United StatesReviewed by:
Manuel Spitschan, University of Oxford, United KingdomDaniel S. Joyce, Stanford University, United States
Copyright © 2018 Bonmati-Carrion, Hild, Isherwood, Sweeney, Revell, Madrid, Rol and Skene. This is an open-access article distributed under the terms of the Creative Commons Attribution License (CC BY). The use, distribution or reproduction in other forums is permitted, provided the original author(s) and the copyright owner(s) are credited and that the original publication in this journal is cited, in accordance with accepted academic practice. No use, distribution or reproduction is permitted which does not comply with these terms.
*Correspondence: Debra J. Skene, ZC5za2VuZUBzdXJyZXkuYWMudWs=