- Department of Neuroscience, The Scripps Research Institute, La Jolla, CA, United States
The long-lasting vulnerability to relapse remains the main challenge for the successful treatment of drug addiction. Neural systems that are involved in processing natural rewards and drugs of abuse overlap. However, neuroplasticity that is caused by drug exposure may be responsible for maladaptive, compulsive, and addictive behavior. The orexin (Orx) system participates in regulating numerous physiological processes, including energy metabolism, arousal, and feeding, and is recruited by drugs of abuse. The Orx system is differentially recruited by drugs and natural rewards. Specifically, we found that the Orx system is more engaged by drugs than by non-drugs, such as sweetened condensed milk (SCM) or a glucose saccharin solution (GSS), in an operant model of reward seeking. Although stimuli (S+) that are conditioned to cocaine (COC), ethanol, and SCM/GSS equally elicited reinstatement, Orx receptor blockade reversed conditioned reinstatement for drugs vs. non-drugs. Moreover, the hypothalamic recruitment of Orx cells was greater in rats that were tested with the COC S+ vs. SCM S+, indicating of a preferential role for the Orx system in perseverative, compulsive-like COC seeking and not behavior that is motivated by palatable food. Accumulating evidence indicates that the paraventricular nucleus of the thalamus (PVT), which receives major Orx projections, mediates drug-seeking behavior. All Orx neurons contain dynorphin (Dyn), and Orx and Dyn are co-released. In the VTA, they play opposing roles in reward and motivation. To fully understand the physiological and behavioral roles of Orx transmission in the PVT, one important consideration is that Orx neurons that project to the PVT may co-release Orx with another peptide, such as Dyn. The PVT expresses both Orx receptors and κ opioid receptors, suggesting that Orx and Dyn act in tandem when released in the PVT, in addition to the VTA. The present review discusses recent findings that suggest the maladaptive recruitment of Orx/Dyn-PVT neurotransmission by drugs of abuse vs. a highly palatable food reward.
Introduction
Drug addiction is a chronic relapsing disorder that is characterized by persistent drug seeking and use (1–4). Relapse vulnerability is a challenge for the successful treatment of substance use disorder, and relapse prevention has emerged as a central focus of treatment and medication development efforts (4, 5). Central issues in addiction research involve clarification of the neurobiological mechanisms that underlie the chronic relapsing nature of addiction and the identification of pharmacotherapies for relapse prevention.
Progress has been made in identifying the neurocircuitry that mediates craving, drug seeking, and relapse. Human functional brain imaging studies (6–8) and rodent studies of Fos expression as a marker of neural activation (9–11) have identified interconnected cortical and limbic brain regions that are activated during drug cue-, drug priming-, and stress-induced reinstatement. This circuitry includes the medial prefrontal cortex (mPFC), basolateral amygdala (BLA), central nucleus of the amygdala (CeA), bed nucleus of the stria terminalis (BNST), ventral tegmental area (VTA), nucleus accumbens (NAC), hippocampus (HIP), dorsal striatum (DS), hypothalamus (HYP), and thalamus [THAL; (12–25)].
The paraventricular nucleus of the thalamus (PVT) is a strategic interconnected neuroanatomical region among thalamic nuclei (Figure 1) that influences other structures that have been implicated in drug-seeking behavior (26–28). Of particular interest, the PVT receives abundant innervation by hypothalamic orexin (Orx) neurons (29). Orexin system function has been positively related to states of arousal and maintaining the waking phase (30). Orexin has also been reported to modulate reward function, particularly drug-seeking behavior [for review, see (31)]. Orexin neurons express dynorphin [Dyn; (32)]. In contrast to Orx, Dyn promotes depressive-like behavior and is involved in mediating the aversive effects of stress (33). The PVT expresses Orx receptors (OrxRs) and κ opioid receptors (KORs), suggesting that Orx and Dyn have a functional interaction in the PVT.
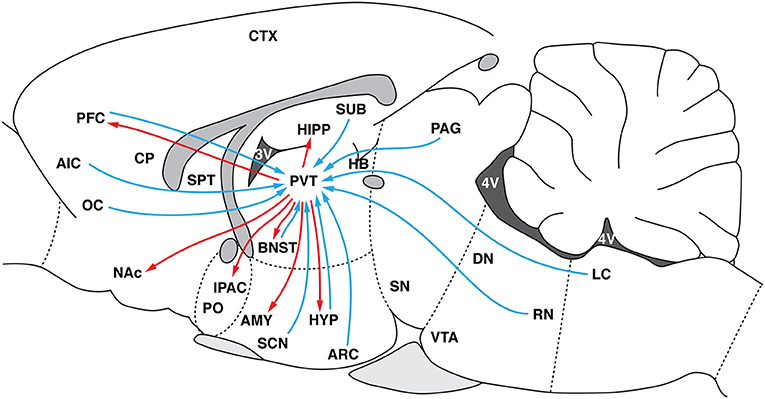
Figure 1.Schematic representation of major PVT efferents (red arrows) and afferents (blue arrows). HYP, hypothalamus; PVT, paraventricular nucleus of the thalamus; ARC, arcuate nucleus of the hypothalamus; LC, locus coeruleus; RN, raphe nucleus; PN, parabrachial nucleus; CTX, cortex; PFC, prefrontal cortex; AIC, agranular insular cortex; OC, orbital cortex; CP, caudate-putamen; SPT, septum; AMY, amygdala; PAG, periaqueductal gray; BNST, bed nucleus of the stria terminalis; NAc, nucleus accumbens; IPAC, interstitial nucleus of the posterior limb of the anterior commissure; PO, preoptic area; HIPP, hippocampus; SUB, subiculum; SN, substantia nigra; VTA, ventral tegmental area, SCN, suprachiasmatic nucleus.
Neural systems that are involved in processing natural rewards and drugs of abuse overlap. Neuroplasticity that is caused by drug exposure may be responsible for maladaptive, compulsive, and addictive behavior (34–37). One important consideration when studying the brain mechanisms that control dug-seeking behavior is to differentiate the mediation of drug-directed behavior from “normal” appetitive behavior. Drugs neuroadaptively influence neural systems that regulate motivation that is normally directed toward natural rewards. Neuroplasticity of this circuitry may be responsible for the maladaptive compulsive behavior that characterizes addiction. This review summarizes recent findings that suggest maladaptive recruitment of the PVT by drugs of abuse. More specifically, this review focuses on Orx transmission in the PVT. There is a functional interaction between Orx and Dyn that impacts drug-seeking behavior. The present review also discusses recent data that demonstrate a differential role for Orx and Dyn in behavior that is directed toward conventional reinforcers. The terms “conventional reinforcer” and “natural reward” are loosely defined in the present review as a non-drug condition (e.g., a sweet, highly palatable solution) that serves as a comparison control for the drug.
Motivational Effects of Drugs of Abuse Implicate the Orexin System
Orexin A (OrxA or hypocretin-1 [Hcrt1]) and orexin B (OrxB or hypocretin-2 [Hcrt2]) are obtained through the proteolytic cleavage of the common precursor prepro-Orx (38–40) and post-translational products of the orexin (Orx) neuropeptide precursor gene. The hypothalamic neuropeptides OrxA and OrxB regulate energy metabolism, arousal, and feeding (41–48). To date, orexin receptor 1 (OrxR1) and OrxR2 have been identified (44, 49, 50). OrxR1 has higher affinity for OrxA (20–30 nM) than OrxB (10- to 1,000-fold lower). OrxR2 has similar affinity for OrxA and OrxB [40 nM range; (44, 49, 50)]. Orexin cell bodies have been identified in the lateral hypothalamus (LH), perifornical hypothalamus (PFA), and dorsomedial hypothalamus (DMH). The LH is associated with reward and motivation (51), and the PFA and DMH are involved in arousal regulation and stress responses (52, 53). Orexin neurons project to the PVT, NAC shell (NACsh), ventral pallidum (VP), VTA, CeA, BNST, and mPFC (52, 54). Over a decade ago, Orx neurons were shown to play a role in modulating reward function and particularly drug-directed behavior (55). Orexin neurons in the LH are activated by stimuli that are predictive of food, morphine, cocaine (COC), and ethanol [EtOH; (19, 55–58)]. Consistent with these observations, intra-VTA microinjections of OrxA produced the renewal of morphine-induced conditioned place preference [CPP; (59, 60)]. Intra-LH administration of the OrxR1 antagonist SB334867 decreased morphine-induced CPP (55). SB334867 also blocked the acquisition of COC-induced behavioral sensitization and the COC-induced potentiation of excitatory currents in dopamine (DA) neurons in the VTA (61). Furthermore, intra-VTA SB334867 administration reduced the motivation to self-administer COC and decreased the COC-induced enhancement of DA signaling in the NAC (62). OrxR1 blockade decreased both EtOH (63) and nicotine (64) self-administration and prevented the reinstatement of COC and EtOH seeking that was induced by cues and stress (57, 63, 65–69). Overall, the Orx system has been implicated in the neurobehavioral and motivational effects of drugs of abuse (61, 70, 71). Orexin projection sites overlap with neural systems that mediate drug craving and seeking (12, 14, 17, 18, 21, 24).
Neuronal Circuitry that Encodes Natural Rewards May Be Usurped by Drugs of Abuse
Neural systems that are involved in processing natural rewards and drugs of abuse overlap. Exposure to drugs of abuse induces neuroadaptations that can cause compulsive-like behavior (34–37). Evidence has shown that the Orx system is recruited by drugs of abuse. A recent study from our laboratory demonstrated differential recruitment of the Orx system by motivational states that were induced by discriminative stimuli that were paired with COC vs. a highly palatable food reward (19). Although weak behavioral effects of OrxR blockade were described for COC (62, 72–74) and EtOH (75, 76) intake, pharmacological manipulation of the Orx system significantly affected the motivational effects of drug-predictive stimuli in CPP and reinstatement studies (55, 57, 63, 66–69). Our laboratory utilized an operant model of reward seeking and found that drugs of abuse engage the Orx system more than non-drugs [e.g., sweetened condensed milk (SCM) and a glucose saccharin solution (GSS); (77)]. For example, although stimuli (S+) that were conditioned to COC, EtOH, and SCM/GSS were equally effective in eliciting reinstatement, systemic SB334867 administration selectively reversed conditioned reinstatement for drugs vs. non-drugs (66, 67). SB334867's preferential effect suggests that drugs neuroadaptively alter neurocircuitry that regulates motivation that is normally directed toward natural rewards, and such alterations can only be revealed by pharmacological manipulations. Notably, baseline levels of responding during self-administration in these studies were considerably higher with SCM than with COC, which could be interpreted as the sweet solution's not really being a “non-drug condition” but rather much more reinforcing than the drug of abuse. However, reinforcer efficacy and the rate of responding on fixed-ratio schedules are not necessarily correlated (78, 79). In ad libitum-fed animals, SCM at the concentration that was used herein produced breakpoints on a progressive-ratio schedule that were similar to breakpoints that were produced by COC (80–82); thus, the acute reinforcing effects of SCM and COC were comparable. Additionally, stimuli that were conditioned to this COC dose and SCM concentration produced identical conditioned reinstatement, suggesting that reliable and comparable conditioning effects under these conditions occurred for COC and SCM despite the difference in baseline levels of responding that were maintained by these reinforcers (66, 83, 84). However, when presented repeatedly, environmental stimuli that were conditioned to drugs of abuse produced perseverative, highly extinction-resistant reward seeking, whereas behavior that was controlled by stimuli that were associated with conventional reward extinguished rapidly in the absence of primary reinforcement (19, 77). This supports the conclusion that the perseveration of reinstatement or reward craving that results from reward-environment associations is a phenomenon that is preferentially linked to drugs of abuse and occurs independently from the initial primary reinforcing strength of the substance that maintains behavior during self-administration. Moreover, for COC, the percentage of Fos+/Orx+ cells was significantly elevated in the LH, DMH, and PFA, and this effect was not observed in SCM rats (19). These findings suggest a role for the Orx system in perseverative, compulsive-like drug seeking but not behavior that is motivated by non-drug palatable food.
The Paraventricular Nucleus of the Thalamus Controls Drug-Seeking Behavior
The PVT is part of dorsal midline thalamic nuclei and adjacent to the dorsal aspect of the third ventricle. The PVT plays a major role in regulating arousal, attention, states of awareness, food consumption, and energy balance (28, 85–88). Midline and intralaminar thalamic nuclei were previously hypothesized to participate in processing non-discriminative nociceptive inputs (89), and each nucleus has subsequently been shown to innervate functionally distinct areas of the cortex and striatum (28, 87, 90).
As shown in Figure 1, the PVT receives projections from brainstem regions that are involved in arousal and autonomic nervous system function (91–96). Through its projections to the NAC and PFC (26, 27, 93, 97–104), the PVT affects cortico-striatal mechanisms that are related to reward and motivation (13, 105, 106).
The PVT receives large and distinct inputs from several areas of the HYP, including the suprachiasmatic, arcuate, dorsomedial, and ventromedial nuclei, as well as preoptic and lateral hypothalamic areas (29, 91, 93, 107–111). These structures control the expression of motivated behavior (112). The PVT is the target of hypothalamic Orx neurons, especially from the LH (29). Additionally, the PVT interfaces HYP and cortical-striatal projections that integrate energy balance, arousal, and food reward [e.g., (113)].
The PVT has been consistently shown to be activated during periods of arousal and stressful conditions (114–119). The PVT was also shown to mediate activity of the hypothalamic-pituitary-adrenal axis in response to chronic stress (114, 120, 121). The PVT was not initially thought to be part of the “neurocircuitry of addiction,” but it has been subsequently shown to play a key role in modulating drug-directed behavior. The PVT sends projections to the NAC, CeA, BNST, and PFC, brain regions that are involved in controlling drug-seeking behavior [Figure 1; (26–28)]. Previous studies reported that the PVT is selectively activated during EtOH-seeking behavior (56, 122). Our recent studies found potent and selective PVT activation during COC-seeking behavior but not SCM-seeking behavior (20). The PVT was recruited during the conditioned reinstatement of COC and SCM seeking, but its activation was correlated with COC- but not SCM-seeking behavior (20). These findings were extended by showing that transient inactivation of the PVT selectively prevented the conditioned reinstatement of COC- vs. SCM-seeking behavior (123), further demonstrating an important role for the PVT in drug-seeking behavior.
The PVT as a whole plays a role in the mediation of drug-seeking behavior. Accumulating evidence shows that Orx transmission in the PVT is specifically implicated in the control of drug-seeking behavior. There is a major Orx projection from the LH to the PVT (29, 124) and from the PFC to the PVT (Figure 1). These connections are hypothesized to modulate the expression of emotional and motivated behaviors (125). The PVT has been proposed to be a key relay that gates Orx-coded reward-related communication between the LH and both the NAC and DS (113).
Recruitment of the Orx system by drugs of abuse may induce neuroadaptations that slant its function toward drug-directed behavior. This may explain the greater sensitivity of the Orx system to OrxR antagonism for drug-seeking behavior vs. natural reward-seeking behavior (66) and could explain why transient inactivation of the PVT prevented COC conditioned reinstatement and not behavior that was motivated by stimuli that were paired with a highly palatable food (123, 126). Orexin transmission in the PVT is directly implicated in COC-seeking behavior. A recent study from our laboratory showed that Orx administration in the PVT reinstated (primed) COC-seeking behavior in animals that had a history of COC dependence (127). Surprisingly, however, Orx's priming effect was prevented by co-administration of the OrxR2 antagonist TCSOX229 and not by SB334867. Intra-PVT administration of SB334867 did not exert such effects, thus confirming the results of a previous study (128) that found that intra-PVT SB334867 administration did not impact cue-induced COC-seeking behavior, thus suggesting a key role for OrxR2 signaling in the PVT in COC-seeking behavior.
The PVT receives innervation from the suprachiasmatic nucleus [SCN; the circadian pacemaker in the mammalian brain; (129, 130)]. The studies that are cited above were performed during the rats' active (dark) phase to avoid behavioral confounds that could be caused by circadian oscillations of neuronal activity in the PVT. Importantly when designing such experiments, differences in intrinsic electrical properties of PVT neurons were described in slices that were collected at different time points. For example, when rats are active during their dark phase, neurons have a more depolarized resting membrane potential and lower resting membrane conductance [for details, see (131)]. The consequences of diurnal changes in PVT neurons and the ways in which the role of the PVT in the circadian cycle affects drug-seeking behavior should be investigated in future studies.
Cocaine-Seeking Behavior in the Paraventricular Nucleus of the Thalamus Is Mediated by Orexin Receptor 2
Our laboratory has found that intra-PVT OrxA administration primed COC-seeking behavior in rats with a history of COC dependence. Co-administration of the OrxR1 antagonist SB334867 and OrxA did not affect COC seeking, whereas the OrxR2 antagonist TCSOX229 blocked OrxA-induced COC seeking (127). The data indicate that the priming effect of OrxA administration in the PVT is mediated by OrxR2.
Intra-PVT OrxA administration primed COC-seeking behavior, implicating the Orx projection to the PVT in the control of both drug craving and relapse. Orexin neurons in the LH have been suggested to modulate ventral striatum activation via a relay through the PVT (113, 124, 132). In fact, earlier studies showed that the PVT is substantially innervated by Orx fibers that originate in the LH and PFA, and the densest innervation is found in the posterior PVT (29). Orexin neurons in both the LH and PVT were shown to be activated by EtOH-related stimuli (56). These findings suggest that Orx projections from the LH to the PVT are associated with drug-seeking behavior. Furthermore, anatomical studies showed that Orx fibers were juxtaposed with PVT-activated neurons (56). OrxA may induce its priming effects by intensifying states of arousal in rats. Orexin controls general arousal (133), and the anticipation of food reward activates OrxR-expressing neurons in the PVT in rats (134). Most neurons in the PVT are sensitive to OrxA and OrxB. One important target of Orx-activated PVT neurons is the PFC (135, 136). Cortical activation that is linked to general arousal may be facilitated by Orx inputs to the PVT (137). This may explain the reinstatement of COC-seeking behavior. Intra-PVT OrxA administration was shown to increase DA levels in the NAC (138), suggesting that the PVT may be a key relay for the effects of Orx on the mesolimbic DA system and reward-seeking behavior.
Another possibility could be that OrxA administration in the PVT induces COC-seeking behavior by increasing anxiety- and stress-like behavior. In humans, anxiety, and stress are known to induce intense craving and trigger relapse during abstinence. The PVT sends projections to the BNST and CeA that contain neurons that densely express Dyn and corticotropin-releasing factor [CRF; (100)]. Dynorphin and CRF are implicated in manifestation of the stress response and negative affective states (139–142). Orexin neurons are activated by stress exposure that is reflected by an increase in Fos expression (143, 144), suggesting that Orx transmission in the PVT is required in both behavioral and physiological responses to stressful situations. Supporting this hypothesis, OrxA and OrxB administration in the PVT produces anxiety-like behavior in rats in the open field (145) and elevated plus maze (146). These findings suggest that Orx transmission in the PVT can act as a stressor under certain conditions and thus induce COC-seeking behavior. The fact that OxR1 blockade did not prevent OrxA prime-induced reinstatement is difficult to reconcile when considering numerous studies that found that peripheral SB334867 administration blocked drug-seeking behavior (66, 68, 147, 148) and the significant expression of OrxR1 in the PVT (149, 150). Importantly, however, the lack of an effect of intra-PVT SB334867 administration confirmed earlier studies that reported similar outcomes with regard to the conditioned reinstatement of COC-seeking behavior (128), suggesting a prominent role for PVT OrxR2 signaling and not OrxR1 signaling during COC-seeking behavior.
Notably, studies of drug addiction have primarily focused on the role of OrxR1; fewer studies have examined OrxR2. OrxR2 expression, similar to OrxR1 expression, is high in the PVT (149, 150). OrxR2 antagonism decreases drug intake and drug seeking when OrxR2 antagonists are administered peripherally. Peripheral OrxR2 antagonist administration decreased EtOH self-administration, decreased the acquisition of EtOH-induced CPP, decreased the expression and reinstatement of EtOH-induced CPP (75), decreased the cue-induced reinstatement of nicotine seeking (151), and decreased heroin self-administration in rats that had 12-h access to heroin per day (152). Furthermore, intra-PVT TCSOX229 administration prevented anxiety-like behavior that was induced by footshock (146). Overall, these findings suggest that PVT OrxR2 signaling is involved in mediating both stress- and anxiety-related behavior.
Dynorphin and Orexin Are Co-Localized and Co-Released and Have Opposite Effects
The Dyn/KOR system is widely distributed in the central nervous system (153, 154). Dynorphin has received increasingly more consideration regarding its regulatory action in many functional pathways of the brain. Consistent with its localization in the hippocampus, hypothalamus, amygdala, striatum, and cortex [Figure 2; (153)], these functions are associated with learning and memory, emotional states, reward mechanisms, stress responses, and pain [for review, see (155)]. Indeed, Dyn is involved in several mood- and motivation-related pathophysiological and physiological processes (155–158). The Dyn/KOR system has been hypothesized to be a possible therapeutic target for treating neuropsychiatric disorders, including drug addiction (157–161). Dynorphins are the major post-translational products of the prodynorphin (Pdyn) gene. They are opioid peptides that are derived from the prepro-Dyn precursor, along with enkephalins and endorphins, and contain the leucine (leu)-enkephalin sequence at the N-terminal portion of the molecule (155, 162, 163). There are two forms of Dyn (DynA and DynB). With the exception of DynA(2-13) (an inactive metabolite of DynA (1–17)), Dyns bind to all three opioid receptors (155). Dynorphins, especially DynA, have greater preference for KORs (164). Orexin promotes arousal (165) and is involved in modulating the rewarding effects of food (166, 167), sexual behavior (168), and drugs of abuse (55, 61). In contrast, Dyn facilitates depressive-like behavior and mediates the aversive effects of stress (33, 169). κ Opioid receptor activation has been shown to reduce the rewarding effects of drugs of abuse (156, 158) through actions that are at least partially mediated by midbrain DA systems (170, 171). Cocaine was shown to enhance the Dyn expression, KOR signaling, and KOR levels in both the VTA and striatum (172–176). Cocaine was also shown to upregulate Pdyn gene expression in the NAC (177). A decrease in KOR expression was found in the septum and BLA in animals that were withdrawn from binge COC self-administration (178). Despite the divergent influences on motivation, these two peptides closely interact. Orexin neurons in the LH express Dyn (32), and Orx and Dyn have been shown to act synergistically. Electrical stimulation of the HYP releases both Orx and Dyn (179). Orexin and Dyn are also co-released and play opposing roles in COC self-administration, brain stimulation reward, and impulsivity (180, 181). They also exert opposing effects in the VTA (181, 182), such as opposing actions on VTA neuronal firing rate, in which they counteract each other's effects upon co-release (181). OrxR1 antagonism in the VTA markedly reduced COC intake under a fixed-ratio 5 schedule, and this effect was blocked by KOR antagonism, suggesting that unopposed actions of Dyn within this brain region control COC intake (Figure 3; (181)). The co-application of both Dyn and Orx caused no net changes in VTA DA neuron firing, suggesting that they exert balanced and opposing actions on DA neurons in the VTA (181). Dynorphin has also been shown to attenuate the greater firing rate that is elicited by Orx in hypothalamic neurons (179) and counterbalance the response of basal forebrain neurons to Orx, preventing overexcitation (184).
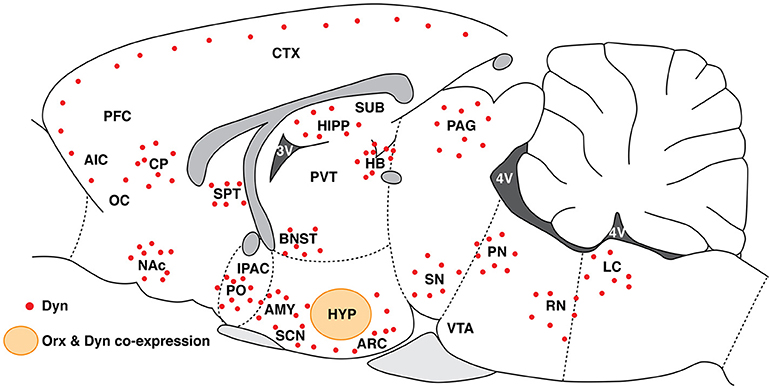
Figure 2.Schematic representation of Dyn distribution (red dots) along the rostro-caudal axis in brain regions that are involved in drug addiction. The orange circle represents the Orx and Dyn overlapping region in the HYP. HYP, hypothalamus; PVT, paraventricular nucleus of the thalamus; ARC, arcuate nucleus of the hypothalamus; LC, locus coeruleus; RN, raphe nucleus; PN, parabrachial nucleus; CTX, cortex; PFC, prefrontal cortex; AIC, agranular insular cortex; OC, orbital cortex; CP, caudate-putamen; SPT, septum; AMY, amygdala; PAG, periaqueductal gray; BNST, bed nucleus of the stria terminalis; NAc, nucleus accumbens; IPAC, interstitial nucleus of the posterior limb of the anterior commissure; PO, preoptic area; HIPP, hippocampus; SUB, subiculum; SN, substantia nigra; VTA, ventral tegmental area; SCN, suprachiasmatic nucleus.
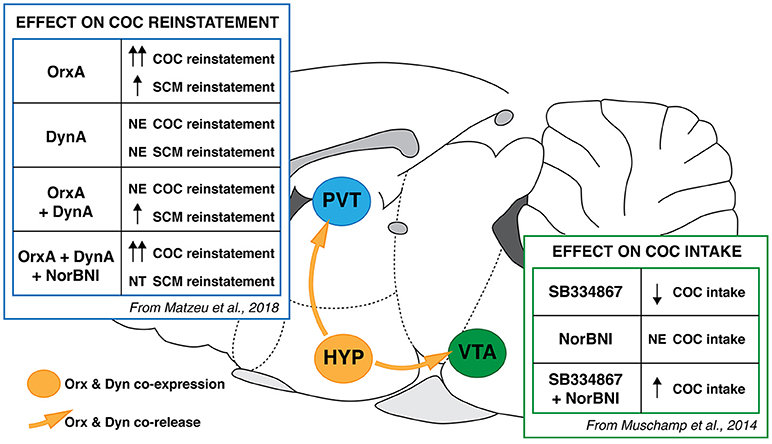
Figure 3.Schematic summary of Orx and Dyn interaction in COC-directed behaviors in the PVT and VTA. (PVT) In rats, OrxA injection in the PVT reinstated COC- and SCM-seeking behavior, with a greater effect in COC animals. DynA blocked OrxA-induced COC seeking but not SCM seeking. nor-Binaltorphimine (nor-BNI) did not induce or potentiate COC-seeking behavior that was induced by OrxA but reversed the effect of DynA (183). (VTA) Intra-VTA administration of SB334867 reduced COC self-administration in rats. nor-BNI administration alone had no effect but reversed the effect of SB334867 (181). PVT, paraventricular nucleus of the thalamus; VTA, ventral tegmental area; NE, no effect; NT, not tested.
Cellular and Behavioral Evidence of Opposing Roles for Dynorphin and Orexin in the Paraventricular Nucleus of the Thalamus
KOR mRNA expression is high in the PVT, where a high degree of correspondence between KOR mRNA and KOR binding was observed (185). Data from our laboratory suggest that Orx and Dyn have opposing effects on excitatory transmission in PVT neurons and OrxA-induced COC-seeking behavior. DynA decreased and OrxA increased PVT glutamatergic (GLUergic) transmission (183). The co-application of DynA and OrxA also counteracted each other's actions on synaptic activity. Behavioral data showed that the priming effect of OrxA was prevented by DynA only in animals that had a history of COC dependence (Figure 3). The effect of DynA was prevented by the KOR antagonist nor-binaltorphimine dihydrochloride, confirming that KORs mediated the DynA-induced blockade of OrxA's priming effect and supporting the hypothesis that KORs may be a target for treating disorders that are associated with greater Orx activity.
Orexin neurons have dense projections to the PVT, and the PVT network is mostly GLUergic. We found an increase in Orx-induced GLU release in PVT neurons, and this effect was comparable to previous findings in the HYP (186, 187), laterodorsal tegmental area (188), nucleus tractus solitarius (189), and neocortex (190). We observed a decrease in GLU transmission that was induced by DynA application, which was similar to effects that were reported in other brain regions (191, 192). Glutamate signaling is modulated by OrxA and DynA in opposite directions. The effects of OrxA were completely reversed by DynA. These two systems are suggested to balance each other in the regulation of PVT neuronal activity (183). Glutamate release in the PVT appears to be regulated by the Orx system, whereas DynA acts locally on presynaptic terminals. DynA and OrxA appear to exert their actions at different loci in the PVT through different neural circuits. Such differential neurocircuitry in the VTA was recently proposed, in which the sensitivity to OrxA was circuit-specific (182). The effects of PVT manipulations with regard to cellular actions were consistent with behavioral data, suggesting that there is a functional interaction between the Orx and Dyn systems.
Our recent study (183) found that intra-PVT injections of OrxA induced COC- and SCM-seeking behavior, and a greater effect was seen in COC animals (Figure 3). The observation that local injections of OrxA in the PVT induced COC- and SCM-seeking behavior strongly supports the hypothesis that Orx projections to the PVT are important in the modulation of reward-seeking behavior in general. An explanation for the general reinstating effects of OrxA for both COC and SCM could involve the known involvement of the Orx system in arousal. Orexin is well known to regulate general arousal (133), and the anticipation of food reward activates Orx-containing neurons in the PVT (134). The medial prefrontal cortex is one target of Orx-activated PVT neurons (135, 136), suggesting that Orx inputs to the PVT facilitate cortical activation that is linked to general arousal (137), which could explain the non-specific effect of intra-PVT OrxA injections on both COC and SCM reinstatement. Furthermore, we found that Dyn did not block SCM seeking that was induced by Orx [Figure 3; (183)], suggesting that although Orx and Dyn might work in tandem, their functional (behavioral) interaction changes following COC dependence. After long access to COC, the Orx and Dyn systems may become “sensitized,” reflected by a greater inhibitory effect of DynA and a greater priming effect of OrxA. Orexin and Dyn system dysregulation that is induced by COC has been previously reported. Using an operant model of reward seeking (i.e., conditioned reinstatement), we showed that the Orx system was engaged to a greater extent by drugs of abuse than by SCM (19, 66, 127). Cocaine appears to produce neuroadaptations in circuits that control the motivation for natural rewards.
Although hypothalamic Orx neurons represent an exclusive source of OrxA to the PVT (54, 125), DynA is expressed in the PFC and CeA [i.e., other brain regions that are connected to the PVT; (125, 154, 193–195)], which may also modulate PVT OrxA transmission. The PFC is implicated in the executive control of drug seeking (18, 196) and has projections to the PVT (125). The PFC is also suggested to supply a considerable Dyn input to the PVT, thereby modulating the effects of Orx. To our knowledge, however, the modulation of COC-seeking behavior by Dyn that is derived from sources other than hypothalamic Orx neurons to the PVT requires further investigation.
The mechanism by which Orx and Dyn in the PVT influence COC seeking is not fully understood. One tentative explanation is that COC induces neuroadaptive changes in HYP Orx/Dyn and consequently alter the PVT OrxR/KOR balance. This could explain the preferential role of Orx and Dyn in COC-seeking behavior vs. SCM-seeking behavior. Hypothetically, synaptic changes at the level of the PVT itself (i.e., modifications of synaptic strength, alterations of peptidergic signaling) or genomic and post-translational modifications may occur, thus affecting Orx and Dyn signaling. This will require further investigation.
Conclusion
The mesocorticolimbic system, especially the VTA, is the focus of most research that implicates Orx in addiction, thus neglecting other systems (34, 55, 61, 62, 181, 197). The PVT receives Orx projections. The PVT is a key component of COC-seeking circuitry, and Orx transmission in the PVT mediates COC-seeking behavior via OrxR2 (127). Dynorphin is co-released with Orx. Our data show that such an interaction is not anatomically specific (i.e., not only in the VTA)—it also occurs in the PVT. Orexin and Dyn have opposing effects both on excitatory transmission in the PVT and behaviorally (183). Further characterization of the involvement of PVT Orx/Dyn transmission in compulsive drug seeking and the way in which the equilibrium between these co-released peptides is affected by drug experience is critically important for medication development for psychiatric disorders, such as drug addiction.
Author Contributions
All authors listed have made a substantial, direct and intellectual contribution to the work, and approved it for publication.
Funding
This is publication number 29658 from The Scripps Research Institute. Supported by NIH/NIDA and NIH/NIAAA grants DA033344, AA024146, AA006420, AA022249, and AA026999 (RM-F).
Conflict of Interest Statement
The authors declare that the research was conducted in the absence of any commercial or financial relationships that could be construed as a potential conflict of interest.
Acknowledgments
The authors thank Michael Arends for assistance with manuscript preparation.
References
1. Leshner AI. Addiction is a brain disease, and it matters. Science (1997) 278:45–7. doi: 10.1126/science.278.5335.45
2. McLellan AT, Lewis DC, O'Brien CP, Kleber HD. Drug dependence, a chronic medical illness: implications for treatment, insurance, and outcomes evaluation. JAMA (2000) 284:1689–95. doi: 10.1001/jama.284.13.1689
3. O'Brien CP, Childress AR, Ehrman R, Robbins SJ. Conditioning factors in drug abuse: can they explain compulsion? J Psychopharmacol. (1998) 12:15–22. doi: 10.1177/026988119801200103
4. O'Brien CP, McLellan AT. Myths about the treatment of addiction. Lancet (1996) 347:237–40. doi: 10.1016/S0140-6736(96)90409-2
5. DeJong W. Relapse prevention: an emerging technology for promoting long-term drug abstinence. Int J Addict. (1994) 29:681–705. doi: 10.3109/10826089409047904
6. Daglish MR, Nutt DJ. Brain imaging studies in human addicts. Eur Neuropsychopharmacol. (2003) 13:453–8. doi: 10.1016/j.euroneuro.2003.08.006
7. Heinz A, Beck A, Mir J, Grusser SM, Grace AA, Wrase J. (2010). Alcohol craving and relapse prediction: imaging studies. In: Kuhn C. M., Koob G. F. editors. Advances in the Neuroscience of Addiction. 2nd ed. Boca Raton, FL: CRC Press/Taylor and Francis. doi: 10.1201/9781420007350-c4
8. Heinz A, Siessmeier T, Wrase J, Buchholz HG, Grunder G, Kumakura Y, et al. Correlation of alcohol craving with striatal dopamine synthesis capacity and D2/3 receptor availability: a combined [18F]DOPA and [18F]DMFP PET study in detoxified alcoholic patients. Am J Psychiatry (2005) 162:1515–20. doi: 10.1176/appi.ajp.162.8.1515
9. Ciccocioppo R, Sanna PP, Weiss F. Cocaine-predictive stimulus induces drug-seeking behavior and neural activation in limbic brain regions after multiple months of abstinence: reversal by D(1) antagonists. Proc Natl Acad Sci USA. (2001) 98:1976–81. doi: 10.1073/pnas.98.4.1976
10. Dayas CV, Liu X, Simms JA, Weiss F. Distinct patterns of neural activation associated with ethanol seeking: effects of naltrexone. Biol Psychiatry (2007) 61:979–89. doi: 10.1016/j.biopsych.2006.07.034
11. Zhao Y, Dayas CV, Aujla H, Baptista MA, Martin-Fardon R, Weiss F. Activation of group II metabotropic glutamate receptors attenuates both stress and cue-induced ethanol-seeking and modulates c-fos expression in the hippocampus and amygdala. J Neurosci. (2006) 26:9967–74. doi: 10.1523/JNEUROSCI.2384-06.2006
12. Belin D, Everitt BJ. Cocaine seeking habits depend upon dopamine-dependent serial connectivity linking the ventral with the dorsal striatum. Neuron (2008) 57:432–41. doi: 10.1016/j.neuron.2007.12.019
13. Cardinal RN, Parkinson JA, Hall J, Everitt BJ. Emotion and motivation: the role of the amygdala, ventral striatum, and prefrontal cortex. Neurosci Biobehav Rev. (2002) 26:321–52. doi: 10.1016/S0149-7634(02)00007-6
14. Everitt BJ, Dickinson A, Robbins TW. The neuropsychological basis of addictive behaviour. Brain Res Brain Res Rev. (2001) 36:129–38. doi: 10.1016/S0165-0173(01)00088-1
15. George MS, Anton RF, Bloomer C, Teneback C, Drobes DJ, Lorberbaum JP, et al. Activation of prefrontal cortex and anterior thalamus in alcoholic subjects on exposure to alcohol-specific cues. Arch Gen Psychiatry (2001) 58:345–52. doi: 10.1001/archpsyc.58.4.345
16. Goldstein RZ, Volkow ND. Drug addiction and its underlying neurobiological basis: neuroimaging evidence for the involvement of the frontal cortex. Am J Psychiatry (2002) 159:1642–2. doi: 10.1176/appi.ajp.159.10.1642
17. Ito R, Dalley JW, Robbins TW, Everitt BJ. Dopamine release in the dorsal striatum during cocaine-seeking behavior under the control of a drug-associated cue. J Neurosci. (2002) 22:6247–53. doi: 10.1523/JNEUROSCI.22-14-06247.2002
18. Kalivas PW, Volkow ND. The neural basis of addiction: a pathology of motivation and choice. Am J Psychiatry (2005) 162:1403–13. doi: 10.1176/appi.ajp.162.8.1403
19. Martin-Fardon R, Cauvi G, Kerr TM, Weiss F. Differential role of hypothalamic orexin/hypocretin neurons in reward seeking motivated by cocaine versus palatable food. Addict Biol. (2018) 23:6–15. doi: 10.1111/adb.12441
20. Matzeu A, Cauvi G, Kerr TM, Weiss F, Martin-Fardon R. The paraventricular nucleus of the thalamus is differentially recruited by stimuli conditioned to the availability of cocaine versus palatable food. Addict Biol. (2017) 22:70–7. doi: 10.1111/adb.12280
21. McFarland K, Kalivas PW. The circuitry mediating cocaine-induced reinstatement of drug-seeking behavior. J Neurosci. (2001) 21:8655–63. doi: 10.1523/JNEUROSCI.21-21-08655.2001
22. Pierce RC, Vanderschuren LJ. Kicking the habit: the neural basis of ingrained behaviors in cocaine addiction. Neurosci Biobehav Rev. (2010) 35:212–9. doi: 10.1016/j.neubiorev.2010.01.007
23. See RE. Neural substrates of conditioned-cued relapse to drug-seeking behavior. Pharmacol Biochem Behav. (2002) 71:517–29. doi: 10.1016/S0091-3057(01)00682-7
24. Steketee JD, Kalivas PW. Drug wanting: behavioral sensitization and relapse to drug-seeking behavior. Pharmacol Rev. (2011) 63:348–65. doi: 10.1124/pr.109.001933
25. Weiss F. Neurobiology of craving, conditioned reward and relapse. Curr Opin Pharmacol. (2005) 5:9–19. doi: 10.1016/j.coph.2004.11.001
26. Bubser M, Deutch AY. Thalamic paraventricular nucleus neurons collateralize to innervate the prefrontal cortex and nucleus accumbens. Brain Res. (1998) 787:304–10. doi: 10.1016/S0006-8993(97)01373-5
27. Moga MM, Weis RP, Moore RY. Efferent projections of the paraventricular thalamic nucleus in the rat. J Comp Neurol. (1995) 359:221–38. doi: 10.1002/cne.903590204
28. Van der Werf YD, Witter MP, Groenewegen HJ. The intralaminar and midline nuclei of the thalamus. Anatomical and functional evidence for participation in processes of arousal and awareness. Brain Res Rev. (2002) 39:107–140. doi: 10.1016/S0165-0173(02)00181-9
29. Kirouac GJ, Parsons MP, Li S. Orexin (hypocretin) innervation of the paraventricular nucleus of the thalamus. Brain Res. (2005) 1059:179–88. doi: 10.1016/j.brainres.2005.08.035
30. de Lecea L. Hypocretins and the neurobiology of sleep-wake mechanisms. Prog Brain Res. (2012) 198:15–24. doi: 10.1016/B978-0-444-59489-1.00003-3
31. Mahler SV, Smith RJ, Moorman DE, Sartor GC, Aston-Jones G. Multiple roles for orexin/hypocretin in addiction. Prog Brain Res. (2012) 198:79–121. doi: 10.1016/B978-0-444-59489-1.00007-0
32. Chou TC, Lee CE, Lu J, Elmquist JK, Hara J, Willie JT, et al. Orexin (hypocretin) neurons contain dynorphin. J Neurosci. (2001) 21:RC168. doi: 10.1523/JNEUROSCI.21-19-j0003.2001
33. Bruchas MR, Land BB, Chavkin C. The dynorphin/kappa opioid system as a modulator of stress-induced and pro-addictive behaviors. Brain Res. (2010) 1314:44–55. doi: 10.1016/j.brainres.2009.08.062
34. Aston-Jones G, Harris GC. Brain substrates for increased drug seeking during protracted withdrawal. Neuropharmacology (2004) 47(Suppl. 1):167–79. doi: 10.1016/j.neuropharm.2004.06.020
35. Kalivas PW, O'Brien C. Drug addiction as a pathology of staged neuroplasticity. Neuropsychopharmacology (2008) 33:166–80. doi: 10.1038/sj.npp.1301564
36. Kelley AE, Berridge KC. The neuroscience of natural rewards: relevance to addictive drugs. J Neurosci. (2002) 22:3306–11. doi: 10.1523/JNEUROSCI.22-09-03306.2002
37. Wanat MJ, Willuhn I, Clark JJ, Phillips PE. Phasic dopamine release in appetitive behaviors and drug addiction. Curr Drug Abuse Rev. (2009) 2:195–213.
38. de Lecea L, Kilduff TS, Peyron C, Gao X, Foye PE, Danielson PE, et al. The hypocretins: hypothalamus-specific peptides with neuroexcitatory activity. Proc Natl Acad Sci USA. (1998) 95:322–7. doi: 10.1073/pnas.95.1.322
39. Gatfield J, Brisbare-Roch C, Jenck F, Boss C. Orexin receptor antagonists: a new concept in CNS disorders? ChemMedChem (2010) 5:1197–214. doi: 10.1002/cmdc.201000132
40. Tsujino N, Sakurai T. Orexin/hypocretin: a neuropeptide at the interface of sleep, energy homeostasis, and reward system. Pharmacol Rev. (2009) 61:162–76. doi: 10.1124/pr.109.001321
41. Edwards CM, Abusnana S, Sunter D, Murphy KG, Ghatei MA, Bloom SR. The effect of the orexins on food intake: comparison with neuropeptide Y, melanin-concentrating hormone and galanin. J Endocrinol. (1999) 160:R7–12. doi: 10.1677/joe.0.160R007
42. Haynes AC, Chapman H, Taylor C, Moore GB, Cawthorne MA, Tadayyon M, et al. Anorectic, thermogenic and anti-obesity activity of a selective orexin-1 receptor antagonist in ob/ob mice. Regul Pept. (2002) 104:153–9. doi: 10.1016/S0167-0115(01)00358-5
43. Haynes AC, Jackson B, Chapman H, Tadayyon M, Johns A, Porter RA, et al. A selective orexin-1 receptor antagonist reduces food consumption in male and female rats. Regul Pept. (2000) 96:45–51. doi: 10.1016/S0167-0115(00)00199-3
44. Sakurai T, Amemiya A, Ishii M, Matsuzaki I, Chemelli RM, Tanaka H, et al. Orexins and orexin receptors: a family of hypothalamic neuropeptides and G protein-coupled receptors that regulate feeding behavior. Cell (1998) 92:573–85. doi: 10.1016/S0092-8674(00)80949-6
45. Sutcliffe JG, de Lecea L. The hypocretins: setting the arousal threshold. Nat Rev Neurosci. (2002) 3:339–49. doi: 10.1038/nrn808
46. Taheri S, Zeitzer JM, Mignot E. The role of hypocretins (orexins) in sleep regulation and narcolepsy. Annu Rev Neurosci. (2002) 25:283–313. doi: 10.1146/annurev.neuro.25.112701.142826
47. Teske JA, Billington CJ, Kotz CM. Hypocretin/orexin and energy expenditure. Acta Physiol. (2010) 198:303–12. doi: 10.1111/j.1748-1716.2010.02075.x
48. Willie JT, Chemelli RM, Sinton CM, Yanagisawa M. To eat or to sleep? Orexin in the regulation of feeding and wakefulness. Annu Rev Neurosci. (2001) 24:429–58. doi: 10.1146/annurev.neuro.24.1.429
49. Ammoun S, Holmqvist T, Shariatmadari R, Oonk HB, Detheux M, Parmentier M, et al. Distinct recognition of OX1 and OX2 receptors by orexin peptides. J Pharmacol Exp Ther. (2003) 305:507–14. doi: 10.1124/jpet.102.048025
50. Scammell TE, Winrow CJ. Orexin receptors: pharmacology and therapeutic opportunities. Annu Rev Pharmacol Toxicol. (2011) 51:243–66. doi: 10.1146/annurev-pharmtox-010510-100528
51. DiLeone RJ, Georgescu D, Nestler EJ. Lateral hypothalamic neuropeptides in reward and drug addiction. Life Sci. (2003) 73:759–68. doi: 10.1016/S0024-3205(03)00408-9
52. Baldo BA, Daniel RA, Berridge CW, Kelley AE. Overlapping distributions of orexin/hypocretin- and dopamine-beta-hydroxylase immunoreactive fibers in rat brain regions mediating arousal, motivation, and stress. J Comp Neurol. (2003) 464:220–37. doi: 10.1002/cne.10783
53. Winsky-Sommerer R, Yamanaka A, Diano S, Borok E, Roberts AJ, Sakurai T, et al. Interaction between the corticotropin-releasing factor system and hypocretins (orexins): a novel circuit mediating stress response. J Neurosci. (2004) 24:11439–48. doi: 10.1523/JNEUROSCI.3459-04.2004
54. Peyron C, Tighe DK, van den Pol AN, de Lecea L, Heller HC, Sutcliffe JG, et al. Neurons containing hypocretin (orexin) project to multiple neuronal systems. J Neurosci. (1998) 18:9996–10015. doi: 10.1523/JNEUROSCI.18-23-09996.1998
55. Harris GC, Wimmer M, Aston-Jones G. A role for lateral hypothalamic orexin neurons in reward seeking. Nature (2005) 437:556–9. doi: 10.1038/nature04071
56. Dayas CV, McGranahan TM, Martin-Fardon R, Weiss F. Stimuli linked to ethanol availability activate hypothalamic CART and orexin neurons in a reinstatement model of relapse. Biol Psychiatry (2008) 63:152–7. doi: 10.1016/j.biopsych.2007.02.002
57. Jupp B, Krstew E, Dezsi G, Lawrence AJ. Discrete cue-conditioned alcohol-seeking after protracted abstinence: pattern of neural activation and involvement of orexin receptors. Br J Pharmacol. (2011) 162:880–9. doi: 10.1111/j.1476-5381.2010.01088.x
58. Martin-Fardon R, Zorrilla EP, Ciccocioppo R, Weiss F. Role of innate and drug-induced dysregulation of brain stress and arousal systems in addiction: Focus on corticotropin-releasing factor, nociceptin/orphanin FQ, and orexin/hypocretin. Brain Res. (2010) 1314:145–61. doi: 10.1016/j.brainres.2009.12.027
59. Porter RA, Chan WN, Coulton S, Johns A, Hadley MS, Widdowson K, et al. 1,3-Biarylureas as selective non-peptide antagonists of the orexin-1 receptor. Bioorg Med Chem Lett. (2001) 11:1907–10. doi: 10.1016/S0960-894X(01)00343-2
60. Smart D, Sabido-David C, Brough SJ, Jewitt F, Johns A, Porter RA, et al. SB-334867-A: the first selective orexin-1 receptor antagonist. Br J Pharmacol. (2001) 132:1179–82. doi: 10.1038/sj.bjp.0703953
61. Borgland SL, Taha SA, Sarti F, Fields HL, Bonci A. Orexin A in the VTA is critical for the induction of synaptic plasticity and behavioral sensitization to cocaine. Neuron (2006) 49:589–601. doi: 10.1016/j.neuron.2006.01.016
62. Espana RA, Oleson EB, Locke JL, Brookshire BR, Roberts DC, Jones SR. The hypocretin-orexin system regulates cocaine self-administration via actions on the mesolimbic dopamine system. Eur J Neurosci. (2010) 31:336–48. doi: 10.1111/j.1460-9568.2009.07065.x
63. Lawrence AJ, Cowen MS, Yang HJ, Chen F, Oldfield B. The orexin system regulates alcohol-seeking in rats. Br J Pharmacol. (2006) 148:752–9. doi: 10.1038/sj.bjp.0706789
64. Hollander JA, Lu Q, Cameron MD, Kamenecka TM, Kenny PJ. Insular hypocretin transmission regulates nicotine reward. Proc Natl Acad Sci USA. (2008) 105:19480–19485. doi: 10.1073/pnas.0808023105
65. Boutrel B, Kenny PJ, Specio SE, Martin-Fardon R, Markou A, Koob GF, et al. Role for hypocretin in mediating stress-induced reinstatement of cocaine-seeking behavior. Proc Natl Acad Sci USA. (2005) 102:19168–73. doi: 10.1073/pnas.0507480102
66. Martin-Fardon R, Weiss F. Blockade of hypocretin receptor-1 preferentially prevents cocaine seeking: comparison with natural reward seeking. Neuroreport (2014) 25:485–8. doi: 10.1097/WNR.0000000000000120
67. Martin-Fardon R, Weiss F. N-(2-methyl-6-benzoxazolyl)-N'-1,5-naphthyridin-4-yl urea (SB334867), a hypocretin receptor-1 antagonist, preferentially prevents ethanol seeking: comparison with natural reward seeking. Addict Biol. (2014) 19:233–6. doi: 10.1111/j.1369-1600.2012.00480.x
68. Richards JK, Simms JA, Steensland P, Taha SA, Borgland SL, Bonci A, et al. Inhibition of orexin-1/hypocretin-1 receptors inhibits yohimbine-induced reinstatement of ethanol and sucrose seeking in Long-Evans rats. Psychopharmacology (2008) 199:109–17. doi: 10.1007/s00213-008-1136-5
69. Smith RJ, Tahsili-Fahadan P, Aston-Jones G. Orexin/hypocretin is necessary for context-driven cocaine-seeking. Neuropharmacology (2009) 58:179–84. doi: 10.1016/j.neuropharm.2009.06.042
70. Bonci A, Borgland S. Role of orexin/hypocretin and CRF in the formation of drug-dependent synaptic plasticity in the mesolimbic system. Neuropharmacology (2009) 56(Suppl. 1):107–11. doi: 10.1016/j.neuropharm.2008.07.024
71. Thompson JL, Borgland SL. A role for hypocretin/orexin in motivation. Behav Brain Res. (2011) 217:446–53. doi: 10.1016/j.bbr.2010.09.028
72. Riday TT, Fish EW, Robinson JE, Jarrett TM, McGuigan MM, Malanga CJ. Orexin-1 receptor antagonism does not reduce the rewarding potency of cocaine in Swiss-Webster mice. Brain Res. (2012) 1431:53–61. doi: 10.1016/j.brainres.2011.11.003
73. Sharf R, Guarnieri DJ, Taylor JR, DiLeone RJ. Orexin mediates morphine place preference, but not morphine-induced hyperactivity or sensitization. Brain Res. (2010) 1317:24–32. doi: 10.1016/j.brainres.2009.12.035
74. Smith RJ, See RE, Aston-Jones G. Orexin/hypocretin signaling at the orexin 1 receptor regulates cue-elicited cocaine-seeking. Eur J Neurosci. (2009) 30:493–503. doi: 10.1111/j.1460-9568.2009.06844.x
75. Shoblock JR, Welty N, Aluisio L, Fraser I, Motley ST, Morton K, et al. Selective blockade of the orexin-2 receptor attenuates ethanol self-administration, place preference, and reinstatement. Psychopharmacology (2011) 215:191–203. doi: 10.1007/s00213-010-2127-x
76. Voorhees CM, Cunningham CL. Involvement of the orexin/hypocretin system in ethanol conditioned place preference. Psychopharmacology (2011) 214:805–18. doi: 10.1007/s00213-010-2082-6
77. Martin-Fardon R, Weiss F. Perseveration of craving: effects of stimuli conditioned to drugs of abuse versus conventional reinforcers differing in demand. Addict Biol. (2016) 22:923–32. doi: 10.1111/adb.12374
78. Richardson NR, Roberts DC. Progressive ratio schedules in drug self-administration studies in rats: a method to evaluate reinforcing efficacy. J Neurosci Methods (1996) 66:1–11. doi: 10.1016/0165-0270(95)00153-0
79. Stafford D, LeSage MG, Glowa JR. Progressive-ratio schedules of drug delivery in the analysis of drug self-administration: a review. Psychopharmacology (1998) 139:169–84.
80. Hodos W. Progressive ratio as a measure of reward strength. Science (1961) 134:943–4. doi: 10.1126/science.134.3483.943
81. Martin-Fardon R, Weiss F. BTCP is a potent reinforcer in rats: comparison of behavior maintained on fixed- and progressive-ratio schedules. Pharmacol Biochem Behav. (2002) 72:343–53. doi: 10.1016/S0091-3057(01)00764-X
82. Roberts DC. Self-administration of GBR 12909 on a fixed ratio and progressive ratio schedule in rats. Psychopharmacology (1993) 111:202–6. doi: 10.1007/BF02245524
83. Baptista MA, Martin-Fardon R, Weiss F. Preferential effects of the metabotropic glutamate 2/3 receptor agonist LY379268 on conditioned reinstatement versus primary reinforcement: comparison between cocaine and a potent conventional reinforcer. J Neurosci. (2004) 24:4723–7. doi: 10.1523/JNEUROSCI.0176-04.2004
84. Martin-Fardon R, Baptista MA, Dayas CV, Weiss F. Dissociation of the effects of MTEP [3-[(2-methyl-1,3-thiazol-4-yl)ethynyl]piperidine] on conditioned reinstatement and reinforcement: comparison between cocaine and a conventional reinforcer. J Pharmacol Exp Ther. (2009) 329:1084–90. doi: 10.1124/jpet.109.151357
85. Bentivoglio M, Balercia G, Kruger L. The specificity of the nonspecific thalamus: the midline nuclei. Prog Brain Res. (1991) 87:53–80. doi: 10.1016/S0079-6123(08)63047-2
86. Colavito V, Tesoriero C, Wirtu AT, Grassi-Zucconi G, Bentivoglio M. Limbic thalamus and state-dependent behavior: The paraventricular nucleus of the thalamic midline as a node in circadian timing and sleep/wake-regulatory networks. Neurosci Biobehav Rev. (2015) 54:3–17. doi: 10.1016/j.neubiorev.2014.11.021
87. Groenewegen HJ, Berendse HW. The specificity of the 'nonspecific' midline and intralaminar thalamic nuclei. Trends Neurosci. (1994) 17:52–7. doi: 10.1016/0166-2236(94)90074-4
88. Kirouac GJ. Placing the paraventricular nucleus of the thalamus within the brain circuits that control behavior. Neurosci Biobehav Rev. (2015) 56:315–29. doi: 10.1016/j.neubiorev.2015.08.005
89. Berendse HW, Groenewegen HJ. Restricted cortical termination fields of the midline and intralaminar thalamic nuclei in the rat. Neuroscience (1991) 42:73–102. doi: 10.1016/0306-4522(91)90151-D
90. Smith Y, Raju DV, Pare JF, Sidibe M. The thalamostriatal system: a highly specific network of the basal ganglia circuitry. Trends Neurosci. (2004) 27:520–7. doi: 10.1016/j.tins.2004.07.004
91. Chen S, Su HS. Afferent connections of the thalamic paraventricular and parataenial nuclei in the rat–a retrograde tracing study with iontophoretic application of Fluoro-Gold. Brain Res. (1990) 522:1–6. doi: 10.1016/0006-8993(90)91570-7
92. Cornwall J, Phillipson OT. Afferent projections to the dorsal thalamus of the rat as shown by retrograde lectin transport. II. The midline nuclei. Brain Res Bull (1988) 21:147–61. doi: 10.1016/0361-9230(88)90227-4
93. Hsu DT, Price JL. Paraventricular thalamic nucleus: subcortical connections and innervation by serotonin, orexin, and corticotropin-releasing hormone in macaque monkeys. J Comp Neurol, (2009) 512:825–48. doi: 10.1002/cne.21934
94. Krout KE, Belzer RE, Loewy AD. Brainstem projections to midline and intralaminar thalamic nuclei of the rat. J Comp Neurol. (2002) 448:53–101. doi: 10.1002/cne.10236
95. Krout KE, Loewy AD. Parabrachial nucleus projections to midline and intralaminar thalamic nuclei of the rat. J Comp Neurol. (2000) 428:475–94. doi: 10.1002/1096-9861(20001218)428:3<475::AID-CNE6>3.0.CO;2-9
96. Ruggiero DA, Anwar S, Kim J, Glickstein SB. Visceral afferent pathways to the thalamus and olfactory tubercle: behavioral implications. Brain Res. (1998) 799:159–71. doi: 10.1016/S0006-8993(98)00442-9
97. Berendse HW, Groenewegen HJ. Organization of the thalamostriatal projections in the rat, with special emphasis on the ventral striatum. J Comp Neurol. (1990) 299:187–228. doi: 10.1002/cne.902990206
98. Brog JS, Salyapongse A, Deutch AY, Zahm DS. The patterns of afferent innervation of the core and shell in the “accumbens” part of the rat ventral striatum: immunohistochemical detection of retrogradely transported fluoro-gold. J Comp Neurol. (1993) 338:255–278. doi: 10.1002/cne.903380209
99. Freedman LJ, Cassell MD. Relationship of thalamic basal forebrain projection neurons to the peptidergic innervation of the midline thalamus. J Comp Neurol. (1994) 348:321–42. doi: 10.1002/cne.903480302
100. Li S, Kirouac GJ. Projections from the paraventricular nucleus of the thalamus to the forebrain, with special emphasis on the extended amygdala. J Comp Neurol. (2008) 506:263–87. doi: 10.1002/cne.21502
101. Otake K, Nakamura Y. Single midline thalamic neurons projecting to both the ventral striatum and the prefrontal cortex in the rat. Neuroscience (1998) 86:635–49. doi: 10.1016/S0306-4522(98)00062-1
102. Parsons MP, Li S, Kirouac GJ. Functional and anatomical connection between the paraventricular nucleus of the thalamus and dopamine fibers of the nucleus accumbens. J Comp Neurol. (2007) 500:1050–63. doi: 10.1002/cne.21224
103. Su HS, Bentivoglio M. Thalamic midline cell populations projecting to the nucleus accumbens, amygdala, and hippocampus in the rat. J Comp Neurol. (1990) 297:582–93.
104. Vertes RP, Hoover WB. Projections of the paraventricular and paratenial nuclei of the dorsal midline thalamus in the rat. J Comp Neurol. (2008) 508:212–37. doi: 10.1002/cne.21679
105. Pennartz CM, Groenewegen HJ, Lopes da Silva FH. The nucleus accumbens as a complex of functionally distinct neuronal ensembles: an integration of behavioural, electrophysiological and anatomical data. Prog Neurobiol. (1994) 42:719–61. doi: 10.1016/0301-0082(94)90025-6
106. Walker DL, Toufexis DJ, Davis M. Role of the bed nucleus of the stria terminalis versus the amygdala in fear, stress, and anxiety. Eur J Pharmacol. (2003) 463:199–216.
107. Cornwall J, Phillipson OT. Afferent projections to the dorsal thalamus of the rat as shown by retrograde lectin transport–I. The mediodorsal nucleus. Neuroscience (1988) 24:1035–49. doi: 10.1016/0306-4522(88)90085-1
108. Kirouac GJ, Parsons MP, Li S. Innervation of the paraventricular nucleus of the thalamus from cocaine- and amphetamine-regulated transcript (CART) containing neurons of the hypothalamus. J Comp Neurol. (2006) 497:155–65. doi: 10.1002/cne.20971
109. Novak CM, Harris JA, Smale L, Nunez AA. Suprachiasmatic nucleus projections to the paraventricular thalamic nucleus in nocturnal rats (Rattus norvegicus) and diurnal nile grass rats (Arviacanthis niloticus). Brain Res. (2000) 874:147–57. doi: 10.1016/S0006-8993(00)02572-5
110. Otake K. Cholecystokinin and substance P immunoreactive projections to the paraventricular thalamic nucleus in the rat. Neurosci Res. (2005) 51:383–94. doi: 10.1016/j.neures.2004.12.009
111. Peng ZC, Bentivoglio M. The thalamic paraventricular nucleus relays information from the suprachiasmatic nucleus to the amygdala: a combined anterograde and retrograde tracing study in the rat at the light and electron microscopic levels. J Neurocytol. (2004) 33:101–16. doi: 10.1023/B:NEUR.0000029651.51195.f9
112. Swanson LW. Cerebral hemisphere regulation of motivated behavior. Brain Res. (2000) 886:113–164.
113. Kelley AE, Baldo BA, Pratt WE. A proposed hypothalamic-thalamic-striatal axis for the integration of energy balance, arousal, and food reward. J Comp Neurol. (2005) 493:72–85. doi: 10.1002/cne.20769
114. Bhatnagar S, Dallman M. Neuroanatomical basis for facilitation of hypothalamic-pituitary-adrenal responses to a novel stressor after chronic stress. Neuroscience (1998) 84:1025–39. doi: 10.1016/S0306-4522(97)00577-0
115. Bubser M, Deutch AY. Stress induces Fos expression in neurons of the thalamic paraventricular nucleus that innervate limbic forebrain sites. Synapse (1999) 32:13–22. doi: 10.1002/(SICI)1098-2396(199904)32:1<13::AID-SYN2>3.0.CO;2-R
116. Novak CM, Nunez AA. Daily rhythms in Fos activity in the rat ventrolateral preoptic area and midline thalamic nuclei. Am J Physiol. (1998) 275(5 Pt 2):R1620–6. doi: 10.1152/ajpregu.1998.275.5.R1620
117. Novak CM, Smale L, Nunez AA. Rhythms in Fos expression in brain areas related to the sleep-wake cycle in the diurnal Arvicanthis niloticus. Am J Physiol Regul Integr Comp Physiol. (2000) 278:R1267–74. doi: 10.1152/ajpregu.2000.278.5.R1267
118. Otake K, Kin K, Nakamura Y. Fos expression in afferents to the rat midline thalamus following immobilization stress. Neurosci Res. (2002) 43:269–82. doi: 10.1016/S0168-0102(02)00042-1
119. Peng ZC, Grassi-Zucconi G, Bentivoglio M. Fos-related protein expression in the midline paraventricular nucleus of the rat thalamus: basal oscillation and relationship with limbic efferents. Exp Brain Res. (1995) 104:21–9. doi: 10.1007/BF00229852
120. Bhatnagar S, Dallman MF. The paraventricular nucleus of the thalamus alters rhythms in core temperature and energy balance in a state-dependent manner. Brain Res. (1999) 851:66–75. doi: 10.1016/S0006-8993(99)02108-3
121. Jaferi A, Nowak N, Bhatnagar S. Negative feedback functions in chronically stressed rats: role of the posterior paraventricular thalamus. Physiol Behav. (2003) 78:365–73. doi: 10.1016/S0031-9384(03)00014-3
122. Hamlin AS, Clemens KJ, Choi EA, McNally GP. Paraventricular thalamus mediates context-induced reinstatement (renewal) of extinguished reward seeking. Eur J Neurosci. (2009) 29:802–12. doi: 10.1111/j.1460-9568.2009.06623.x
123. Matzeu A, Weiss F, Martin-Fardon R. Transient inactivation of the posterior paraventricular nucleus of the thalamus blocks cocaine-seeking behavior. Neurosci Lett. (2015) 608:34–9. doi: 10.1016/j.neulet.2015.10.016
124. Parsons MP, Li S, Kirouac GJ. The paraventricular nucleus of the thalamus as an interface between the orexin and CART peptides and the shell of the nucleus accumbens. Synapse (2006) 59:480–90. doi: 10.1002/syn.20264
125. Li S, Kirouac GJ. Sources of inputs to the anterior and posterior aspects of the paraventricular nucleus of the thalamus. Brain Struct Funct. (2012) 217:257–73. doi: 10.1007/s00429-011-0360-7
126. James MH, Charnley JL, Jones E, Levi EM, Yeoh JW, Flynn JR, et al. Cocaine- and amphetamine-regulated transcript (CART) signaling within the paraventricular thalamus modulates cocaine-seeking behaviour. PLoS ONE (2010) 5:e12980. doi: 10.1371/journal.pone.0012980
127. Matzeu A, Kerr TM, Weiss F, Martin-Fardon R. Orexin-A/Hypocretin-1 mediates cocaine-seeking behavior in the posterior paraventricular nucleus of the Thalamus via Orexin/Hypocretin receptor-2. J Pharmacol Exp Ther. (2016) 359:273–9. doi: 10.1124/jpet.116.235945
128. James MH, Charnley JL, Levi EM, Jones E, Yeoh JW, Smith DW, et al. Orexin-1 receptor signalling within the ventral tegmental area, but not the paraventricular thalamus, is critical to regulating cue-induced reinstatement of cocaine-seeking. Int J Neuropsychopharmacol. (2011) 14:684–90. doi: 10.1017/S1461145711000423
129. Dibner C, Schibler U, Albrecht U. The mammalian circadian timing system: organization and coordination of central and peripheral clocks. Annu Rev Physiol. (2010) 72:517–49. doi: 10.1146/annurev-physiol-021909-135821
130. Morin LP, Allen CN. The circadian visual system, 2005. Brain Res Rev. (2006) 51:1–60. doi: 10.1016/j.brainresrev.2005.08.003
131. Kolaj M, Zhang L, Ronnekleiv OK, Renaud LP. Midline thalamic paraventricular nucleus neurons display diurnal variation in resting membrane potentials, conductances, and firing patterns in vitro. J Neurophysiol. (2012) 107:1835–44. doi: 10.1152/jn.00974.2011
132. Kelley AE, Baldo BA, Pratt WE, Will MJ. Corticostriatal-hypothalamic circuitry and food motivation: integration of energy, action and reward. Physiol Behav. (2005) 86:773–95. doi: 10.1016/j.physbeh.2005.08.066
133. Sakurai T, Mieda M, Tsujino N. The orexin system: roles in sleep/wake regulation. Ann N Y Acad Sci. (2010) 1200:149–61. doi: 10.1111/j.1749-6632.2010.05513.x
134. Choi DL, Davis JF, Fitzgerald ME, Benoit SC. The role of orexin-A in food motivation, reward-based feeding behavior and food-induced neuronal activation in rats. Neuroscience (2010) 167:11–20. doi: 10.1016/j.neuroscience.2010.02.002
135. Huang H, Ghosh P, van den Pol AN. Prefrontal cortex-projecting glutamatergic thalamic paraventricular nucleus-excited by hypocretin: a feedforward circuit that may enhance cognitive arousal. J Neurophysiol. (2006) 95:1656–68. doi: 10.1152/jn.00927.2005
136. Ishibashi M, Takano S, Yanagida H, Takatsuna M, Nakajima K, Oomura Y, et al. Effects of orexins/hypocretins on neuronal activity in the paraventricular nucleus of the thalamus in rats in vitro. Peptides (2005) 26:471–81. doi: 10.1016/j.peptides.2004.10.014
137. Sato-Suzuki I, Kita I, Seki Y, Oguri M, Arita H. Cortical arousal induced by microinjection of orexins into the paraventricular nucleus of the rat. Behav Brain Res. (2002) 128:169–77. doi: 10.1016/S0166-4328(01)00307-2
138. Choi DL, Davis JF, Magrisso IJ, Fitzgerald ME, Lipton JW, Benoit SC. Orexin signaling in the paraventricular thalamic nucleus modulates mesolimbic dopamine and hedonic feeding in the rat. Neuroscience (2012) 210:243–8. doi: 10.1016/j.neuroscience.2012.02.036
139. Davis M. Are different parts of the extended amygdala involved in fear versus anxiety? Biol Psychiatry (1998) 44:1239–47. doi: 10.1016/S0006-3223(98)00288-1
140. Davis M, Walker DL, Miles L, Grillon C. Phasic vs sustained fear in rats and humans: role of the extended amygdala in fear vs anxiety. Neuropsychopharmacology (2010) 35:105–35. doi: 10.1038/npp.2009.109
141. Heinrichs SC, Koob GF. Corticotropin-releasing factor in brain: a role in activation, arousal, and affect regulation. J Pharmacol Exp Ther. (2004) 311:427–40. doi: 10.1124/jpet.103.052092
142. Shirayama Y, Chaki S. Neurochemistry of the nucleus accumbens and its relevance to depression and antidepressant action in rodents. Curr Neuropharmacol. (2006) 4:277–91.
143. Espana RA, Valentino RJ, Berridge CW. Fos immunoreactivity in hypocretin-synthesizing and hypocretin-1 receptor-expressing neurons: effects of diurnal and nocturnal spontaneous waking, stress and hypocretin-1 administration. Neuroscience (2003) 121:201–17. doi: 10.1016/S0306-4522(03)00334-8
144. Furlong TM, Vianna DM, Liu L, Carrive P. Hypocretin/orexin contributes to the expression of some but not all forms of stress and arousal. Eur J Neurosci. (2009) 30:1603–14. doi: 10.1111/j.1460-9568.2009.06952.x
145. Li Y, Li S, Wei C, Wang H, Sui N, Kirouac GJ. Changes in emotional behavior produced by orexin microinjections in the paraventricular nucleus of the thalamus. Pharmacol Biochem Behav. (2010) 95:121–8. doi: 10.1016/j.pbb.2009.12.016
146. Li Y, Li S, Wei C, Wang H, Sui N, Kirouac GJ. Orexins in the paraventricular nucleus of the thalamus mediate anxiety-like responses in rats. Psychopharmacology (2010) 212:251–65. doi: 10.1007/s00213-010-1948-y
147. Bentzley BS, Aston-Jones G. Orexin-1 receptor signaling increases motivation for cocaine-associated cues. Eur J Neurosci. (2015) 41:1149–56. doi: 10.1111/ejn.12866
148. Zhou L, Smith RJ, Do PH, Aston-Jones G, See RE. Repeated orexin 1 receptor antagonism effects on cocaine seeking in rats. Neuropharmacology (2012) 63:1201–7. doi: 10.1016/j.neuropharm.2012.07.044
149. Marcus JN, Aschkenasi CJ, Lee CE, Chemelli RM, Saper CB, Yanagisawa M, et al. Differential expression of orexin receptors 1 and 2 in the rat brain. J Comp Neurol. (2001) 435:6–25. doi: 10.1002/cne.1190
150. Trivedi P, Yu H, MacNeil DJ, Van der Ploeg LH, Guan XM. Distribution of orexin receptor mRNA in the rat brain. FEBS Lett. (1998) 438:71–5.
151. Uslaner JM, Winrow CJ, Gotter AL, Roecker AJ, Coleman PJ, Hutson PH, et al. Selective orexin 2 receptor antagonism blocks cue-induced reinstatement, but not nicotine self-administration or nicotine-induced reinstatement. Behav Brain Res. (2014) 269:61–5. doi: 10.1016/j.bbr.2014.04.012
152. Schmeichel BE, Barbier E, Misra KK, Contet C, Schlosburg JE, Grigoriadis D, et al. Hypocretin receptor 2 antagonism dose-dependently reduces escalated heroin self-administration in rats. Neuropsychopharmacology (2015) 40:1123–9. doi: 10.1038/npp.2014.293
153. Merchenthaler I, Maderdrut JL, Cianchetta P, Shughrue P, Bronstein D. In situ hybridization histochemical localization of prodynorphin messenger RNA in the central nervous system of the rat. J Comp Neurol. (1997) 384:211–32.
154. Watson SJ, Khachaturian H, Akil H, Coy DH, Goldstein A. Comparison of the distribution of dynorphin systems and enkephalin systems in brain. Science (1982) 218:1134–6.
155. Schwarzer C. 30 years of dynorphins–new insights on their functions in neuropsychiatric diseases. Pharmacol Ther. (2009) 123:353–70. doi: 10.1016/j.pharmthera.2009.05.006
156. Bruijnzeel AW. kappa-Opioid receptor signaling and brain reward function. Brain Res Rev. (2009) 62:127–46. doi: 10.1016/j.brainresrev.2009.09.008
157. Tejeda HA, Shippenberg TS, Henriksson R. The dynorphin/kappa-opioid receptor system and its role in psychiatric disorders. Cell Mol Life Sci. (2012) 69:857–96. doi: 10.1007/s00018-011-0844-x
158. Wee S, Koob GF. The role of the dynorphin-kappa opioid system in the reinforcing effects of drugs of abuse. Psychopharmacology (2010) 210:121–35. doi: 10.1007/s00213-010-1825-8
159. Butelman ER, Yuferov V, Kreek MJ. kappa-opioid receptor/dynorphin system: genetic and pharmacotherapeutic implications for addiction. Trends Neurosci. (2012) 35:587–96. doi: 10.1016/j.tins.2012.05.005
160. Knoll AT, Carlezon W. A. Jr. Dynorphin, stress, and depression. Brain Res. (2010) 1314:56–73. doi: 10.1016/j.brainres.2009.09.074
161. Walker BM, Koob GF. Pharmacological evidence for a motivational role of kappa-opioid systems in ethanol dependence. Neuropsychopharmacology (2008) 33:643–52. doi: 10.1038/sj.npp.1301438
162. Rossier J. Opioid peptides have found their roots. Nature (1982) 298:221–2. doi: 10.1038/298221a0
163. Rossier J, Chapouthier G. Brain opiates. Endeavour (1982) 6:168–76. doi: 10.1016/0160-9327(82)90072-2
164. Chavkin C, James IF, Goldstein A. Dynorphin is a specific endogenous ligand of the kappa opioid receptor. Science (1982) 215:413–5. doi: 10.1126/science.6120570
165. Adamantidis AR, Zhang F, Aravanis AM, Deisseroth K, de Lecea L. Neural substrates of awakening probed with optogenetic control of hypocretin neurons. Nature (2007) 450:420–4. doi: 10.1038/nature06310
166. Borgland SL, Chang SJ, Bowers MS, Thompson JL, Vittoz N, Floresco SB, et al. Orexin A/hypocretin-1 selectively promotes motivation for positive reinforcers. J Neurosci (2009) 29:11215–25. doi: 10.1523/JNEUROSCI.6096-08.2009
167. Sharf R, Sarhan M, Brayton CE, Guarnieri DJ, Taylor JR, DiLeone RJ. Orexin signaling via the orexin 1 receptor mediates operant responding for food reinforcement. Biol Psychiatry (2010) 67:753–60. doi: 10.1016/j.biopsych.2009.12.035
168. Muschamp JW, Dominguez JM, Sato SM, Shen RY, Hull EM. A role for hypocretin (orexin) in male sexual behavior. J Neurosci. (2007) 27:2837–45. doi: 10.1523/JNEUROSCI.4121-06.2007
169. Carroll FI, Carlezon W. A. Jr. Development of kappa opioid receptor antagonists. J Med Chem. (2013) 56:2178–95. doi: 10.1021/jm301783x
170. Shippenberg TS, Zapata A, Chefer VI. Dynorphin and the pathophysiology of drug addiction. Pharmacol Ther. (2007) 116:306–21. doi: 10.1016/j.pharmthera.2007.06.011
171. Zhang Y, Butelman ER, Schlussman SD, Ho A, Kreek MJ. Effect of the endogenous kappa opioid agonist dynorphin A(1-17) on cocaine-evoked increases in striatal dopamine levels and cocaine-induced place preference in C57BL/6J mice. Psychopharmacology (2004) 172:422–9. doi: 10.1007/s00213-003-1688-3
172. Daunais JB, Roberts DC, McGinty JF. Cocaine self-administration increases preprodynorphin, but not c-fos, mRNA in rat striatum. Neuroreport (1993) 4:543–6. doi: 10.1097/00001756-199305000-00020
173. Piras AP, Zhou Y, Schlussman SD, Ho A, Kreek MJ. Acute withdrawal from chronic escalating-dose binge cocaine administration alters kappa opioid receptor stimulation of [35S] guanosine 5'-O-[gamma-thio]triphosphate acid binding in the rat ventral tegmental area. Neuroscience (2010) 169:751–7. doi: 10.1016/j.neuroscience.2010.04.060
174. Spangler R, Ho A, Zhou Y, Maggos CE, Yuferov V, Kreek MJ. Regulation of kappa opioid receptor mRNA in the rat brain by “binge' pattern cocaine administration and correlation with preprodynorphin mRNA. Brain Res Mol Brain Res. (1996) 38:71–6.
175. Spangler R, Unterwald EM, Kreek MJ. 'Binge' cocaine administration induces a sustained increase of prodynorphin mRNA in rat caudate-putamen. Brain Res Mol Brain Res. (1993) 19:323–7.
176. Unterwald EM, Rubenfeld JM, Kreek MJ. Repeated cocaine administration upregulates kappa and mu, but not delta, opioid receptors. Neuroreport (1994) 5:1613–6.
177. Caputi FF, Di Benedetto M, Carretta D, Bastias del Carmen Candia S, D'Addario C, Cavina C, et al. Dynorphin/KOP and nociceptin/NOP gene expression and epigenetic changes by cocaine in rat striatum and nucleus accumbens. Prog Neuropsychopharmacol Biol Psychiatry (2014) 49:36–46. doi: 10.1016/j.pnpbp.2013.10.016
178. Bailey A, Gianotti R, Ho A, Kreek MJ. Downregulation of kappa-opioid receptors in basolateral amygdala and septum of rats withdrawn for 14 days from an escalating dose “binge” cocaine administration paradigm. Synapse (2007) 61:820–6. doi: 10.1002/syn.20436
179. Li Y, van den Pol AN. Differential target-dependent actions of coexpressed inhibitory dynorphin and excitatory hypocretin/orexin neuropeptides. J Neurosci. (2006) 26:13037–47. doi: 10.1523/JNEUROSCI.3380-06.2006
180. Li X, Marchant NJ, Shaham Y. Opposing roles of cotransmission of dynorphin and hypocretin on reward and motivation. Proc Natl Acad Sci USA. (2014) 111:5765–6. doi: 10.1073/pnas.1403603111
181. Muschamp JW, Hollander JA, Thompson JL, Voren G, Hassinger LC, Onvani S, et al. Hypocretin (orexin) facilitates reward by attenuating the antireward effects of its cotransmitter dynorphin in ventral tegmental area. Proc Natl Acad Sci USA. (2014) 111:E1648–55. doi: 10.1073/pnas.1315542111
182. Baimel C, Lau BK, Qiao M, Borgland SL. Projection-target-defined effects of orexin and dynorphin on VTA dopamine neurons. Cell Rep. (2017) 18:1346–55. doi: 10.1016/j.celrep.2017.01.030
183. Matzeu A, Kallupi M, George O, Schweitzer P, Martin-Fardon R. Dynorphin counteracts orexin in the paraventricular nucleus of the thalamus: cellular and behavioral evidence. Neuropsychopharmacology (2018) 43:1010–20. doi: 10.1038/npp.2017.250
184. Ferrari LL, Agostinelli LJ, Krashes MJ, Lowell BB, Scammell TE, Arrigoni E. Dynorphin inhibits basal forebrain cholinergic neurons by pre- and postsynaptic mechanisms. J Physiol. (2016) 594:1069–85. doi: 10.1113/JP271657
185. Mansour A, Fox CA, Meng F, Akil H, Watson SJ. Kappa 1 receptor mRNA distribution in the rat CNS: comparison to kappa receptor binding and prodynorphin mRNA. Mol Cell Neurosci. (1994) 5:124–44. doi: 10.1006/mcne.1994.1015
186. Li Y, Gao XB, Sakurai T, van den Pol AN. Hypocretin/Orexin excites hypocretin neurons via a local glutamate neuron-A potential mechanism for orchestrating the hypothalamic arousal system. Neuron (2002) 36:1169–81. doi: 10.1016/S0896-6273(02)01132-7
187. van den Pol AN, Gao XB, Obrietan K, Kilduff TS, Belousov AB. Presynaptic and postsynaptic actions and modulation of neuroendocrine neurons by a new hypothalamic peptide, hypocretin/orexin. J Neurosci. (1998) 18:7962–71.
188. Burlet S, Tyler CJ, Leonard CS. Direct and indirect excitation of laterodorsal tegmental neurons by Hypocretin/Orexin peptides: implications for wakefulness and narcolepsy. J Neurosci. (2002) 22:2862–72. doi: 10.1523/JNEUROSCI.22-07-02862.2002
189. Smith BN, Davis SF, Van Den Pol AN, Xu W. Selective enhancement of excitatory synaptic activity in the rat nucleus tractus solitarius by hypocretin 2. Neuroscience (2002) 115:707–14. doi: 10.1016/S0306-4522(02)00488-8
190. Aracri P, Banfi D, Pasini ME, Amadeo A, Becchetti A. Hypocretin (orexin) regulates glutamate input to fast-spiking interneurons in layer V of the Fr2 region of the murine prefrontal cortex. Cereb Cortex (2015) 25:1330–47. doi: 10.1093/cercor/bht326
191. Crowley NA, Bloodgood DW, Hardaway JA, Kendra AM, McCall JG, Al-Hasani R, et al. Dynorphin controls the gain of an amygdalar anxiety circuit. Cell Rep. (2016) 14:2774–83. doi: 10.1016/j.celrep.2016.02.069
192. Wagner JJ, Terman GW, Chavkin C. Endogenous dynorphins inhibit excitatory neurotransmission and block LTP induction in the hippocampus. Nature (1993) 363:451–4.
193. Healy DJ, Meador-Woodruff JH. Prodynorphin-derived peptide expression in primate cortex and striatum. Neuropeptides (1994) 27:277–84. doi: 10.1016/0143-4179(94)90108-2
194. Ramsdell CD, Meador-Woodruff JH. Expression of prodynorphin-derived peptides and mRNA in guinea-pig cortex. Neuropeptides (1993) 25:131–8. doi: 10.1016/0143-4179(93)90093-P
195. Weber E, Evans CJ, Barchas JD. Predominance of the amino-terminal octapeptide fragment of dynorphin in rat brain regions. Nature (1982) 299:77–9.
196. Kalivas PW, Volkow N, Seamans J. Unmanageable motivation in addiction: a pathology in prefrontal-accumbens glutamate transmission. Neuron (2005) 45:647–50. doi: 10.1016/j.neuron.2005.02.005
Keywords: paraventricular nucleus of the thalamus, orexin, dynorphin, drug addiction, drug-seeking behavior, natural reward
Citation: Matzeu A and Martin-Fardon R (2018) Drug Seeking and Relapse: New Evidence of a Role for Orexin and Dynorphin Co-transmission in the Paraventricular Nucleus of the Thalamus. Front. Neurol. 9:720. doi: 10.3389/fneur.2018.00720
Received: 24 March 2018; Accepted: 08 August 2018;
Published: 28 August 2018.
Edited by:
M. Foster Olive, Arizona State University, United StatesReviewed by:
Nicholas W. Gilpin, LSU Health Sciences Center New Orleans, United StatesSelena Bartlett, Translational Research Institute, Australia
Copyright © 2018 Matzeu and Martin-Fardon. This is an open-access article distributed under the terms of the Creative Commons Attribution License (CC BY). The use, distribution or reproduction in other forums is permitted, provided the original author(s) and the copyright owner(s) are credited and that the original publication in this journal is cited, in accordance with accepted academic practice. No use, distribution or reproduction is permitted which does not comply with these terms.
*Correspondence: Alessandra Matzeu, YW1hdHpldUBzY3JpcHBzLmVkdQ==