- Department of Pharmacology, Penn State College of Medicine, Hershey, PA, United States
Generation of neuronal diversity is a biological strategy widely used in the brain to process complex information. The olfactory bulb is the first relay station of olfactory information in the vertebrate central nervous system. In the olfactory bulb, axons of the olfactory sensory neurons form synapses with dendrites of projection neurons that transmit the olfactory information to the olfactory cortex. Historically, the olfactory bulb projection neurons have been classified into two populations, mitral cells and tufted cells. The somata of these cells are distinctly segregated within the layers of the olfactory bulb; the mitral cells are located in the mitral cell layer while the tufted cells are found in the external plexiform layer. Although mitral and tufted cells share many morphological, biophysical, and molecular characteristics, they differ in soma size, projection patterns of their dendrites and axons, and odor responses. In addition, tufted cells are further subclassified based on the relative depth of their somata location in the external plexiform layer. Evidence suggests that different types of tufted cells have distinct cellular properties and play different roles in olfactory information processing. Therefore, mitral and different types of tufted cells are considered as starting points for parallel pathways of olfactory information processing in the brain. Moreover, recent studies suggest that mitral cells also consist of heterogeneous subpopulations with different cellular properties despite the fact that the mitral cell layer is a single-cell layer. In this review, we first compare the morphology of projection neurons in the olfactory bulb of different vertebrate species. Next, we explore the similarities and differences among subpopulations of projection neurons in the rodent olfactory bulb. We also discuss the timing of neurogenesis as a factor for the generation of projection neuron heterogeneity in the olfactory bulb. Knowledge about the subpopulations of olfactory bulb projection neurons will contribute to a better understanding of the complex olfactory information processing in higher brain regions.
Introduction
Our ability to perceive the world through our senses begins with different sensory organs and results in distinct brain regions processing this information. However, even a single sensory system must process multiple aspects of the sensory modality in order to generate a meaningful sensory experience. For example, we can acquire various types of object information, such as size and shape, color, brightness, location, and motion, through the visual system (Wandell, 1995). A strategy to process multiple visual submodalities is to enhance the functional diversity of the neuronal circuit by expanding the neuronal heterogeneity in the retina (MacNeil and Masland, 1998; Masland, 2012; Baden et al., 2016; Yan et al., 2020). In the primate visual system, strong evidence suggests that the color and motion of an object are differentially processed through parallel pathways (Nassi and Callaway, 2009). These parallel pathways are formed by different types of retinal ganglion cells (RGCs) projecting their axons to different layers of the lateral geniculate nucleus of the thalamus and subsequently different regions of the cortex (Nassi and Callaway, 2009; Schiller, 2010; Seabrook et al., 2017). Thus far, at least 20 different RGC subtypes have been identified based on their different morphological, physiological, and molecular properties in the primate retina (Kolb et al., 1992; Peng et al., 2019). Recent studies expanded the classification of the cells into 40 RGC subtypes in the mouse retina (Sanes and Masland, 2015; Baden et al., 2016; Rheaume et al., 2018).
In terms of evolution, the olfactory system is one of the oldest senses to monitor the outside world. It is said that there are more than 3,000 chemicals that can be detected by our chemosensory system as fragrances (Arctander, 1969), and the mixture of these chemicals produces different odor qualities and behavioral responses (Kay et al., 2005; Saraiva et al., 2016). Unlike the visual system that receives photons with 3–6 types of photoreceptor cells (rod cells and 2–5 types of cone cells) (Baden et al., 2020), the vertebrate olfactory system takes a unique strategy to discriminate these odor qualities by establishing a large repertoire of odorant receptors expressed by olfactory sensory neurons (OSNs) (Buck and Axel, 1991; Zhang and Firestein, 2002; Godfrey et al., 2004; Malnic et al., 2004). However, each OSN expresses only a single type of odorant receptor (Chess et al., 1994; Serizawa et al., 2003; Monahan and Lomvardas, 2015). All OSNs project axons to the olfactory bulb (OB), a structure that is found in all vertebrate animals and the first relay station for the olfactory information in the central nervous system (Mombaerts et al., 1996; Northcutt, 2002). The OSNs expressing the same type of odorant receptor converge their axons usually into 2-3 spherical structures known as glomeruli (Mombaerts et al., 1996). The convergence of OSN axons forms a glomerular map, or odorant receptor map, at the surface of the OB (Mori et al., 1999; Mori and Sakano, 2011). The information from the glomerular map is transmitted to the olfactory cortex through the axons of OB projection neurons (Ghosh et al., 2011; Sosulski et al., 2011; Igarashi et al., 2012; Hirata et al., 2019).
A single odor or an odor mixture activates a distinct combination of OSNs and glomeruli (Malnic et al., 1999; Mori et al., 2006; Johnson and Leon, 2007; Fletcher, 2011). Therefore, processing the glomerular activation pattern on the map is the first step to identify the odors. In addition, the olfactory system processes different aspects of smell sensation; not only the odor quality, but also the odor intensity, pleasantness, and location of the source (Thomas-Danguin et al., 2014). Different concentrations of the same odor activate different numbers of glomeruli and change the temporal profiles of OB projection neuron responses (Johnson and Leon, 2000; Spors and Grinvald, 2002; Gautam et al., 2014; Bolding and Franks, 2018). Specific domains in the glomerular map are responsible for mediating particular odor-induced behaviors in rodents (Bear et al., 2016). For example, signals from the dorsomedial glomeruli are responsible for the innate fear responses caused by predator odors in mice, which is likely controlled by the central amygdala (Kobayakawa et al., 2007; Dewan et al., 2013; Root et al., 2014; Isosaka et al., 2015; Kondoh et al., 2016). In contrast, a subset of ventral glomeruli are targeted by TRPM5 expressing OSNs that are responsible for semiochemical processing, and these glomeruli are innervated by a population of mitral cells projecting to the medial amygdala (Lin et al., 2007; Thompson et al., 2012; Lemons et al., 2017). Mounting evidence demonstrates the importance of OB organization in information processing.
Here, we focus on the subtypes of OB projection neurons. Accumulating evidence shows that OB projection neurons can be subdivided into several subpopulations with different morphological and physiological properties. This suggests that different subpopulations of OB projection neurons may be involved in processing different aspects of smell via parallel pathways. We begin by summarizing the OB projections neurons found in lower vertebrate species to analyze their diversity from an evolutionary perspective, focusing primarily on their morphological properties. Then, we describe the similarities and differences among the subpopulation of projection neurons in the rodent OB. Lastly, we discuss the timing of neurogenesis as a factor for generating heterogeneity of projection neurons in the OB. The authors apologize to those whose work was not included here due to space limitations.
Basic Neural Circuitry of the Mammalian Olfactory System
The mammalian main OB (MOB) has an onion-like layer structure consisting of various cellular populations segregated into individual layers. Figure 1 shows the structure of the rodent OB as an anatomical model for reference. Although we do not go deeper into detail in this review, there are many excellent reviews summarizing the cell types, synapses, and neuronal circuits found in the MOB (Shepherd et al., 2004; Wachowiak and Shipley, 2006; Figueres-Onate et al., 2014; Imai, 2014; Nagayama et al., 2014; Sakano, 2020). Briefly, the OSN axons run tangentially through the olfactory nerve layer (ONL) at the surface of the OB before entering the glomerular layer (GL) (Klenoff and Greer, 1998; Rodriguez-Gil et al., 2015). Here, the OSNs form axodendritic synapses with the OB projection neurons, known as mitral and tufted cells, as well as periglomerular interneurons (Pinching and Powell, 1971b; White, 1972). In addition, OB projection neurons and periglomerular interneurons form reciprocal dendrodendritic synapses within the GL (Pinching and Powell, 1971b; White, 1972). Both mitral and tufted cells are glutamatergic neurons, and they share morphological features such as a single primary dendrite projecting to a single glomerulus as well as the secondary dendrites extending within the external plexiform layer (EPL), a layer beneath the GL (Macrides and Schneider, 1982; Mori et al., 1983). Within the EPL, the secondary dendrites of mitral and tufted cells form dendrodendritic synapses with granule cells, another type of interneuron (Rall et al., 1966; Price and Powell, 1970b). The somata of most tufted cells are found in the EPL while the mitral cell somata are aligned below the EPL to form a thin layer called the mitral cell layer (MCL) (Schneider and Macrides, 1978). The granule cell layer (GCL) is located below the MCL and is the largest layer in the OB formed primarily by the somata of granule cells (Schneider and Macrides, 1978). There is another thin layer between the MCL and GCL known as the internal plexiform layer (IPL) which contains axon collaterals from tufted cells (Liu and Shipley, 1994).
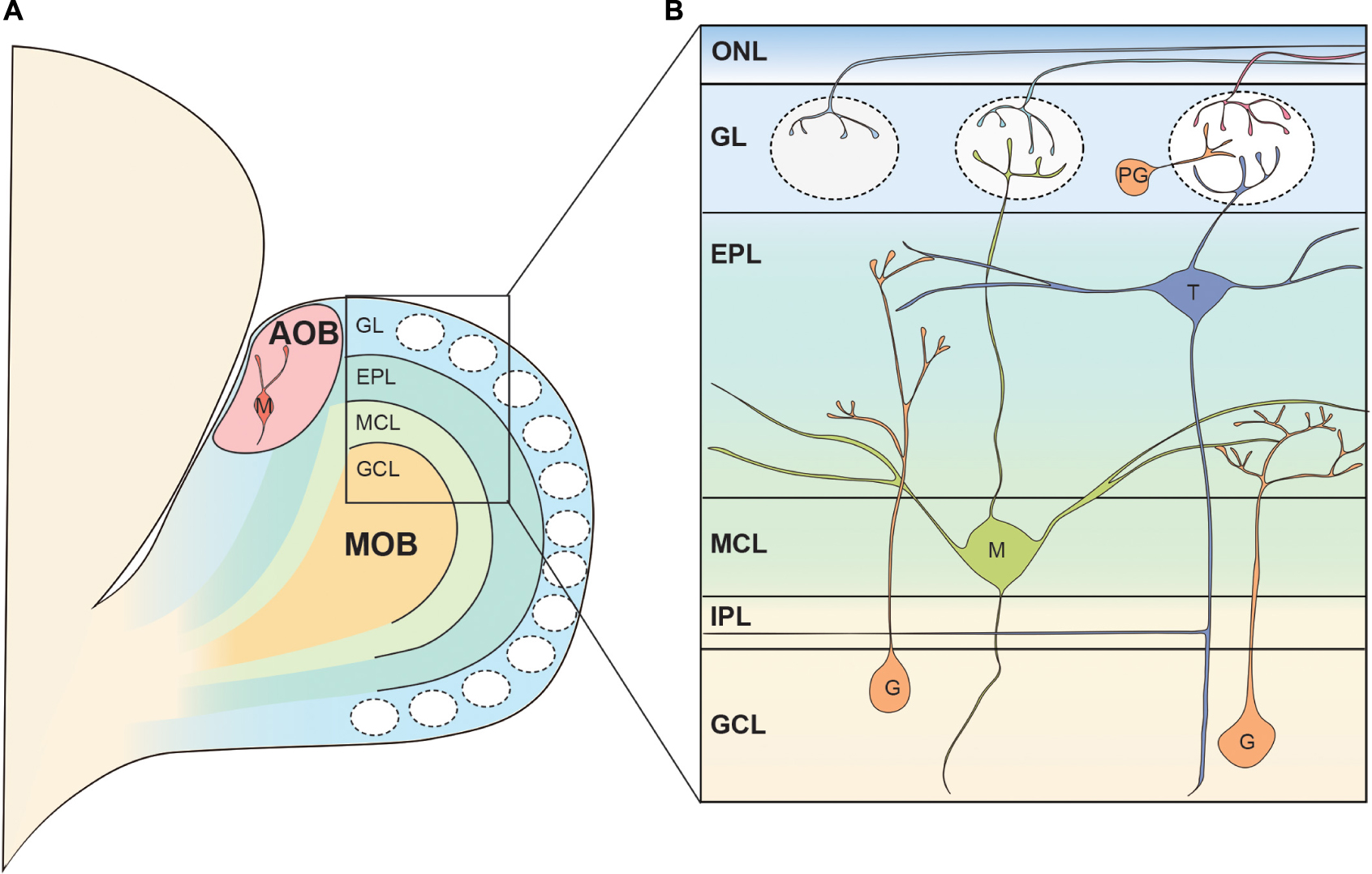
Figure 1. Basic neural circuit in the rodent olfactory bulb. (A) The olfactory bulb (OB) is formed at the most anterior portion of the telencephalon in the rodent brain. The accessory olfactory bulb (AOB) receiving the pheromonal information from the vomeronasal organ is located at the posterodorsal OB. Mitral cells in the AOB have multiple apical dendrites projecting to multiple glomeruli. The rest of the OB is called the main OB (MOB) and is innervated by olfactory sensory neurons (OSNs). The MOB consists of multiple concentric layers. (B) The OSN axons tangentially run at the surface of the MOB within the olfactory nerve layer (ONL), before entering the glomeruli. Mitral (M) and tufted cell (T) somata are located in the mitral cell layer (MCL) and external plexiform layer (EPL), respectively, and project their primary dendrites to a single glomerulus. In the glomerulus, OSN axons form axodendritic synapses with mitral and tufted cells as well as periglomerular cells (PG). The secondary dendrites of mitral and tufted cells form dendrodendritic synapses with granule cells (G) in the EPL. Somata of periglomerular and granule cells are found in the glomerular layer (GL) and the granule cell layer (GCL), respectively. IPL, internal plexiform layer.
In addition to the main olfactory system, rodents have a vomeronasal system, unlike humans, through which they process pheromonal signals with great sensitivity (Silva and Antunes, 2017). For further details on aspects of the organization of the neuronal circuits involved in the pheromonal signals, readers are referred to several excellent and comprehensive studies and reviews (Larriva-Sahd, 2008; Tirindelli et al., 2009; Yokosuka, 2012; Silva and Antunes, 2017; Holy, 2018; Mohrhardt et al., 2018). In the vomeronasal organ, pheromones are received by vomeronasal receptors expressed by vomeronasal sensory neurons, which project axons to the accessory olfactory bulb (AOB) located at the dorsoposterior region of the MOB (Belluscio et al., 1999; Rodriguez et al., 1999; Boschat et al., 2002; Del Punta et al., 2002). Similar to the MOB, there are glomeruli at the surface of the AOB, where the AOB mitral cells receive synaptic inputs from vomeronasal neurons (Barber et al., 1978). However, upon reaching the AOB, individual axons can divide to terminate in multiple glomeruli (Larriva-Sahd, 2008). Therefore, contrary to the MOB, axons of sensory neurons expressing a given receptor form multiple glomeruli in the AOB (Belluscio et al., 1999; Rodriguez et al., 1999). Somata of AOB mitral cells are scattered beneath the glomeruli and a single AOB mitral cell possesses multiple primary dendrites which innervate multiple glomeruli (Takami and Graziadei, 1991; Wagner et al., 2006; Yonekura and Yokoi, 2007; Larriva-Sahd, 2008). The AOB mitral cells extend secondary dendrites in the layer beneath the glomeruli where they form dendrodendritic synapses with AOB granule cells whose somata are localized at the deepest layer of the AOB (Yonekura and Yokoi, 2007; Larriva-Sahd, 2008). Although it is said that AOB mitral cells are the only type of projection neurons in the AOB, at least three types of AOB projection neurons with different somata shape, location, and dendritic morphology have been suggested to exist (Yonekura and Yokoi, 2007; Larriva-Sahd, 2008). In addition, a recent study showed that a subset of AOB projection neurons was intrinsically rhythmogenic (Gorin et al., 2016; Zylbertal et al., 2017). These results raise the possibility that AOB mitral cells also consist of highly heterogeneous subpopulations. The mitral cell axons exit the AOB in bundles and run through a layer between the somata of mitral cells and granule cells before converging on the lateral olfactory tract (LOT), ultimately transmitting the pheromonal information to higher brain regions (von Campenhausen and Mori, 2000; Larriva-Sahd, 2008).
Morphology of OB Projection Neurons in Lower Vertebrates
Fish
The olfactory system of teleost fish sends the unbranched OSN axon to a single glomerulus (Weiss et al., 2020). The layer structure of the teleost fish OB is similar to that of the rodent OB, but a bit more simplistic (Kermen et al., 2013). In the fish OB, there are two types of glutamatergic projection neurons; mitral cells, a major projection neuron, and ruffed cells (Figure 2A1). There is no clear MCL, and both mitral cells and ruffed cells are located in the external cell layer that lies beneath the GL (Satou, 1990). Although they share the same name and have apical dendrites innervating glomeruli allowing them to receive input from OSN axons, the mitral cells of teleost fish are significantly different from those in the mammalian OB. Golgi staining and retrograde tracing of OB neurons in adult teleosts revealed that mitral cells do not extend secondary dendrites (Kosaka and Hama, 1982; Fuller et al., 2006). In most teleost fish, mitral cells possess multiple apical dendrites that project to multiple glomeruli, with the exception of zebrafish (Kosaka and Hama, 1982; Oka, 1983; Fujita et al., 1988; Satou, 1990; Fuller et al., 2006). The zebrafish mitral cells typically have only a single apical dendrite innervating a single glomerulus, and a smaller percentage of mitral cells have multiple apical dendrites that still innervate a single glomerulus (Fuller et al., 2006). In contrast to the mitral cells, the ruffed cells have a membranous field surrounding the initial part of the axon (Kosaka and Hama, 1979; Fuller and Byrd, 2005). A major difference from mitral cells is that ruffed cells do not receive direct input from OSN axons, but receive inhibitory synaptic inputs from interneurons activated by mitral cells (Kosaka, 1980; Satou, 1990). Therefore, the activity patterns of mitral and ruffed cells are contrasting in nature (Zippel, 1999; Zippel et al., 2000).
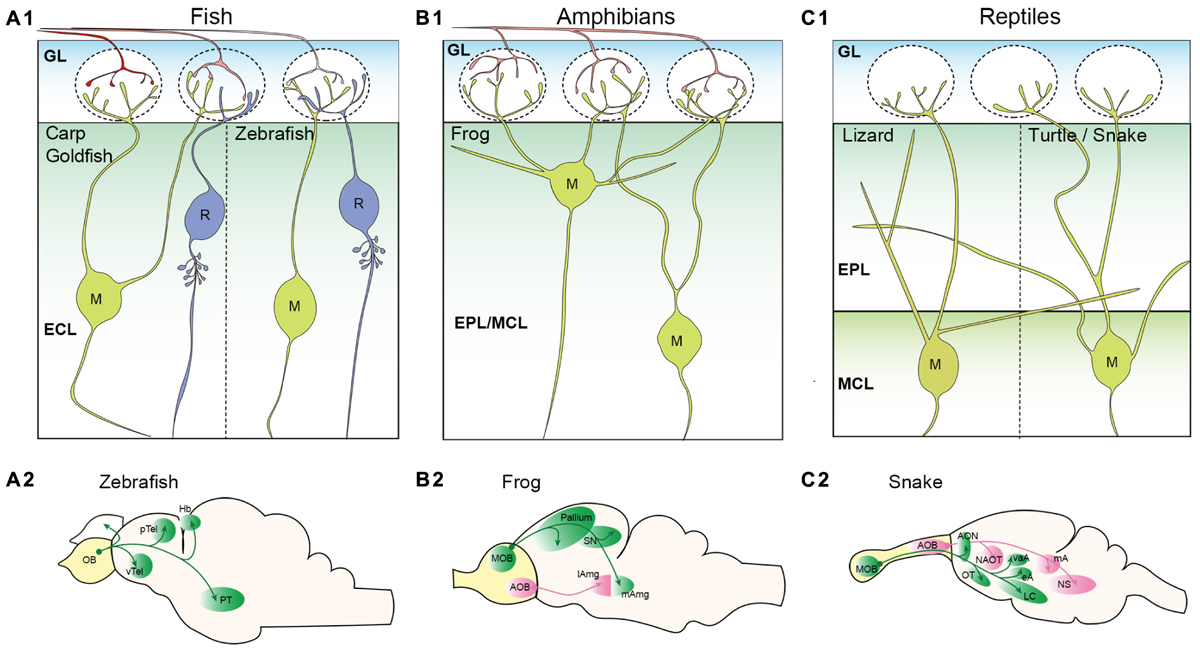
Figure 2. Projection neurons in the non-mammalian vertebrate olfactory bulb. (A) Schematic illustrations of projection neurons in the teleost fish olfactory bulb (OB). Mitral (M), and ruffed cells (R) are located in the external cell layer (ECL) (A1). Most teleost fish including carp and goldfish have mitral cells with multiple apical dendrites projecting to multiple glomeruli. However, zebrafish mitral cells have only a single apical dendrite. No secondary dendrites are observed in the mitral cells. A morphological characteristic of ruffed cells is a membranous field surrounding the initial part of the axon. Axonal projection patterns from the zebrafish OB are shown in (A2) (Miyasaka et al., 2014). (B) Schematic illustrations of projection neurons in the amphibian OB. Somata of frog mitral cells (M) are located in the external plexiform layer (EPL) and mitral cell layer (MCL); these two layers are not clearly segregated (B1). Mitral cells have multiple apical dendrites, and some have secondary dendrites that do not project to the glomeruli. Axonal projection pattern from the frog OB are shown in (B2) (Eisthen and Polese, 2010). (C) Schematic illustrations of projection neurons in the reptile OB. OSN axons are not depicted as it has not been clearly shown whether a single OSN of reptiles project unbranched axon to a single glomerulus. Somata of mitral cells (M) are found in the MCL that is clearly segregated from the EPL. A single primary dendrite is formed in lizard mitral cells, while multiple primary dendrites are found in turtle mitral cells (C1). Both lizard and turtle mitral cells have clear secondary dendrites extending within the EPL. Axonal projection pattern from the snake OB are shown in (C2) (Eisthen and Polese, 2010; Ubeda-Banon et al., 2011). pTel and vTel, posterior and ventral telencephalon; Hb, habenula; PT, posterior tuberculum; SN, septal nucleus; lAmg and mAmg, lateral and medial amygdala; AON, anterior olfactory nucleus; OT, olfactory tubercle; LC, lateral cortex; NAOT, nucleus of accessory olfactory tract; vaA, eA, and mA, ventral anterior, external, and medial amygdala; and NS, nucleus sphericus.
Mitral and ruffed cells project their axons to higher brain regions through either the medial olfactory tract (MOT) or LOT depending on the location of their somata (Fuller et al., 2006; Miyasaka et al., 2014). In the brain, mitral cell axons send their projections to five major regions; posterior and ventral telencephalon, posterior tuberculum, right habenula, and ipsilateral/contralateral OB (Eisthen and Polese, 2010) (Figure 2A2). Genetic labeling of zebrafish mitral cells at single-cell resolution revealed that (1) individual mitral cells can target multiple regions; the MOT and LOT do not determine the target regions, (2) mitral cells innervating the same glomerulus do not show the same axon trajectory and (3) the right habenula is innervated by mitral cells that convey the information from the medial glomeruli (Miyasaka et al., 2009, 2014).
Although there is no distinct AOB in the teleost OB, a report suggested that a specific glomerulus located at the mediodorsal OB, mdG2, may be functionally similar and serve as the accessory olfactory system in teleost fish (Biechl et al., 2017). The mdG2 receives inputs solely from crypt cells, a specific type of OSN activated by kin odor, and the mitral cells targeting the mdG2 send their axons to the intermediate ventral telencephalic nucleus (Ahuja et al., 2013; Biechl et al., 2016, 2017; Gerlach et al., 2019). It is hypothesized that this nucleus in teleost fish may be functionally analogous to the medial amygdala of mammals (Biechl et al., 2017). The evolutionary origin of the accessory olfactory system is an intriguing topic to aid in our understanding of the parallel pathways for olfactory information processing. The axonal projection patterns of the ruffed cells in the fish olfactory system is another research topic that remains to be elucidated.
Amphibians
In contrast to the mammalian olfactory system, a single OSN of amphibians typically innervates multiple glomeruli (Gilbert et al., 2013; Hassenklover and Manzini, 2013). The OB of the frog has a layer structure similar to, but not as fully concentric as, that of the mammalian OB (Byrd and Burd, 1991; Scalia et al., 1991a; Kratskin et al., 2000). The projection neurons called mitral cells are scattered among the EPL and MCL (Kratskin et al., 2000). The basic morphology of the frog mitral cells resembles that of rodent mitral and tufted cells, except for their multiple (1–6) primary dendrites that innervate multiple glomeruli (Jiang and Holley, 1992) (Figure 2B1). Jiang and Holley (1992) further demonstrated that the mitral cells located superficially, close to the GL, extend their primary dendrites with a larger angle and targeted glomeruli across a wider field of range. The primary and secondary dendrites of the frog mitral cells are not clearly distinct in that some dendrites send several branches into the GL while the others remain in the EPL (Jiang and Holley, 1992). Mitral cells with similar morphology were also observed in the salamander OB (Herrick, 1924). A unique feature of amphibians is the process of metamorphosis in which species transform from an immature to mature state. This is an intriguing process with respect to olfactory system development and evolution. The studies of the OB morphology in the clawed frog showed that the basic structure of the mature OB was apparent in tadpoles (around larval stage 48/49) and remained constant throughout the late larval stage and into adulthood, with only the size increasing (Byrd and Burd, 1991; Nezlin and Schild, 2000). The majority of MOB projection neurons in both frog and salamander tadpoles (stages 51–56) had more than one glomerular tuft (up to 4) innervating different glomeruli (Herrick, 1924; Nezlin et al., 2003).
As shown in Figure 2B2, OB projection neurons project to the dorsal, lateral, and medial pallium as well as the lateral and medial septal nuclei in the frog brain (Eisthen and Polese, 2010). They also innervate the lateral amygdala (Scalia et al., 1991b). Contrary to the lack of an apparent AOB in the teleost fish, the AOB in the frog is quite evident and is located at the ventrolateral region of the OB. Anterograde HRP tracing experiments revealed that the AOB projection neurons project to the medial amygdala (Scalia et al., 1991b). While the projection from the MOB and AOB may converge in the amygdala, the medial amygdaloid nucleus may be connected exclusively to the AOB (Scalia et al., 1991b) (Figure 2B2).
Reptiles
Whether a single OSN of reptiles projects unbranched axon to a single glomerulus has not been clearly described. The MOB of reptiles also exhibits a distinct layer structure in which the GL, EPL, MCL, and GCL are clearly visible, however, they may have a thicker IPL than mammalian OB (Kirillova and Lin, 1998; Pinato and Midtgaard, 2003; Kondoh et al., 2013). Mitral cells are distributed throughout the MCL in the turtle OB (Kirillova and Lin, 1998). The morphology of turtle mitral cells was determined by reconstruction after electrophysiological recordings and showed the existence of long secondary dendrites that reach almost half of the bulbar circumference (Mori et al., 1981). In the turtle and snake OB, a single mitral cell possesses more than one primary dendrite and therefore is capable of targeting multiple glomeruli (Mori et al., 1981; Iwahori et al., 1989) (Figure 2C1). However, mitral cells in the lizard OB have only one dendritic tuft in a glomerulus, which was shown with Golgi staining (Llahi et al., 1985) (Figure 2C1). While not yet experimentally concluded, the existence of tufted cells in the reptile OB is suggested due to the discernible segregation of the EPL and MCL. In fact, the mitral cells observed in the EPL of the lizard OB are described as displaced mitral cells (Llahi et al., 1985). Cells varying in soma size were identified in the EPL and MCL of the snake MOB (Kondoh et al., 2013), and the Japanese striped snake has two mitral cell types that are morphologically distinct based on the somata locations within the MCL (Iwahori et al., 1989). These support a concept suggestive of a transition between mitral cells and tufted cells.
In the snake brain, the axonal projections from the MOB terminate at the AON, olfactory tubercle (OT), the lateral cortex, and some amygdaloid nuclei (Halpern, 1976; Martinez-Garcia et al., 1991; Lohman and Smeets, 1993; Lanuza and Halpern, 1998; Eisthen and Polese, 2010; Ubeda-Banon et al., 2011) (Figure 2C2). Overall, the projection patterns of most reptilian MOB projection neurons are somewhat comparable to that of the rodent (see Figures 2C2, 4). However, in contrast to the mammalian OB projection neurons, some axons from the snake MOB enter the ipsilateral stria medullaris thalami, cross the midline in the habenular commissure, enter the contralateral stria medullaris thalami and terminate in the contralateral lateral pallium (Halpern, 1976; Lanuza and Halpern, 1998). The accessory olfactory system becomes more noticeable in reptiles compared to amphibians considering that the AOB is caudally located and clearly separated from the MOB (Martínez-Marcos and Halpern, 2009; Ubeda-Banon et al., 2011; Kondoh et al., 2013). The AOB mitral cells in the reptile give rise to more than one primary dendrite with multiple tufts in the GL (Llahi et al., 1985). The axons of AOB mitral cells follow along the accessory olfactory tract and project to three portions of the amygdala: the nucleus sphericus, medial amygdala, and nucleus of the accessory olfactory tract (Halpern, 1976; Martinez-Garcia et al., 1991; Lohman and Smeets, 1993; Lanuza and Halpern, 1998; Eisthen and Polese, 2010; Ubeda-Banon et al., 2011) (Figure 2C2). In some reptilian species including a type of lizard, the AOB projects to the bed nucleus of stria terminalis (BNST) (Martinez-Garcia et al., 1991).
Evolutionary Morphological Changes of OB Projection Neurons
Different species have morphologically distinct MOB projection neurons. As described above, the mitral cells of teleost fish, amphibians, and reptiles, excluding zebrafish and lizards, typically possess multiple primary dendrites projecting to multiple glomeruli while only a single primary dendrite is formed in the mammalian mitral cells (Kosaka and Hama, 1982; Oka, 1983; Fujita et al., 1988; Satou, 1990; Dryer and Graziadei, 1994; Fuller et al., 2006). These results suggest that OB projection neurons have reduced the number of their primary dendrites from multiple to a single primary dendrite over the course of evolution. Interestingly, rodent MOB mitral cells form multiple primary dendrites with tufts in multiple glomeruli at the initial stage of development (Malun and Brunjes, 1996; Lin et al., 2000). Although it is reasonable to assume that having a single primary dendrite projecting to a single glomerulus assists in odor discrimination, it is still largely unknown how each mitral cell is able to “select” one dendrite during maturation. A recent study showed that spontaneous network activity among immature projection neurons in the neonatal OB is essential for the pruning of excess primary dendrites, but the OSN activity does not appear to be necessary in this process (Lin et al., 2000; Fujimoto et al., 2019). Comparing the development of OB projection neurons in different species would provide us with interesting insights into the molecular and cellular mechanisms underlying the selection of a single primary dendrite.
It is also noteworthy that clear secondary dendrites seem to be first apparent in the reptilian mitral cells, whereas the distinction between the primary and secondary dendrites in amphibian mitral cells is difficult to observe. From an evolutionary perspective, this information suggests that information processing in the OB became more intricate and complex as life began to shift from living in water to living on land. As discussed in the latter section, difference in the length of secondary dendrites is important for OB projection neurons to differentially respond to the odor inputs in the rodent OB. Formation of the two characteristic dendrites might be a stepping stone to generate the parallel processing pathways in the olfactory system.
Similarities and Differences Between Mitral and Tufted Cells in the Rodent OB
Henceforth, we will focus on the subpopulation of MOB projection neurons reported from the rodent olfactory system. The projection neurons in the rodent OB are essentially classified into two types; mitral cells, named after their shape resembling that of a bishop’s miter, and tufted cells, named by Ramon y Cajal (1911) and Figueres-Onate et al. (2014). An increasing number of studies report that the two cell types exhibit different properties in response to odor stimuli, and dendrite/axonal projection patterns, suggesting that mitral and tufted cells process different aspects of olfactory information as described below.
Morphological Properties
In the rodent OB, the projection neurons located in the MCL are defined as mitral cells, and others found in the EPL and GL are deemed tufted cells (Ramon y Cajal, 1911; Shepherd et al., 2004; Greer et al., 2008; Ennis et al., 2015). However, based on the relative depth of somata location in the EPL and GL, tufted cells are further subclassified into the external, middle, and internal tufted cells, also known as superficial, intermediate, and deep tufted cells, respectively (Ramon y Cajal, 1911; Macrides and Schneider, 1982; Orona et al., 1984; Shepherd et al., 2004). The internal tufted cells are sometimes identified as displaced mitral cells because of their proximity to the MCL (Mori et al., 1983; Kishi et al., 1984; Shepherd et al., 2004). The size of mitral cell somata (20–25 μm) is typically larger than that of tufted cells (10–20 μm) (Pinching and Powell, 1971a). Both mature mitral and tufted cells have a single primary dendrite with a tuft at the tip residing in a single glomerulus where they receive excitatory input from OSN axons (Ramon y Cajal, 1911; Mori et al., 1983). Mitral and tufted cells also form dendrodendritic synapses with inhibitory periglomerular interneurons in the GL (Pinching and Powell, 1971b).
In the rodent OB, different types of projection neurons possess different lengths of secondary dendrites. Mori et al. studied projection neurons in the rabbit OB, and showed that a single mitral cell possesses ∼15,000 μm of secondary dendrites, which is almost four times longer than that of middle tufted cells (∼4,000 μm) (Mori et al., 1983). Internal tufted cells have an intermediate length of secondary dendrites (∼12,000 μm) (Mori et al., 1983). Also in the mouse OB, it was shown that the total dendritic length of a mitral cell (∼15,000 μm) is much longer than that of a middle tufted cell (∼7,500 μm) (Igarashi et al., 2012). The tufted cells located in the most superficial EPL or GL are classified as the external tufted cells. Like the other mitral and tufted cells, the primary dendrites of external tufted cells are generally uni-glomerular, with a small subpopulation being di-glomerular (Ennis and Hayar, 2008). There are two distinct subpopulations of external tufted cells, and the most prominent dissimilarity between the populations is the presence or absence of secondary dendrites (Macrides and Schneider, 1982; Hayar et al., 2004a, b; Antal et al., 2006; Liu and Shipley, 2008; Hirata et al., 2019). The somata of external tufted cells with secondary dendrites are generally found in the deeper one-third of the GL, or in the EPL near the boundary with the GL. Morphometric analysis using 300 μm thick rat OB slices showed that the total length of secondary dendrites of external tufted cells in the slice was ∼1,200 μm (Antal et al., 2006). Although we cannot directly compare the results due to the differences in species and method, it is reasonable to assume that mitral cells possess the longest secondary dendrites while those of the external tufted cells are the shortest. Interestingly, the other group of external tufted cells whose somata are only found in the GL lack secondary dendrites (Macrides and Schneider, 1982; Hayar et al., 2004a, b; Antal et al., 2006; Liu and Shipley, 2008; Hirata et al., 2019). Based on the data acquired thus far, it can be asserted that the deeper the location of projection neurons cell somata in the OB, the longer the secondary dendrites are as shown in Figure 3A.
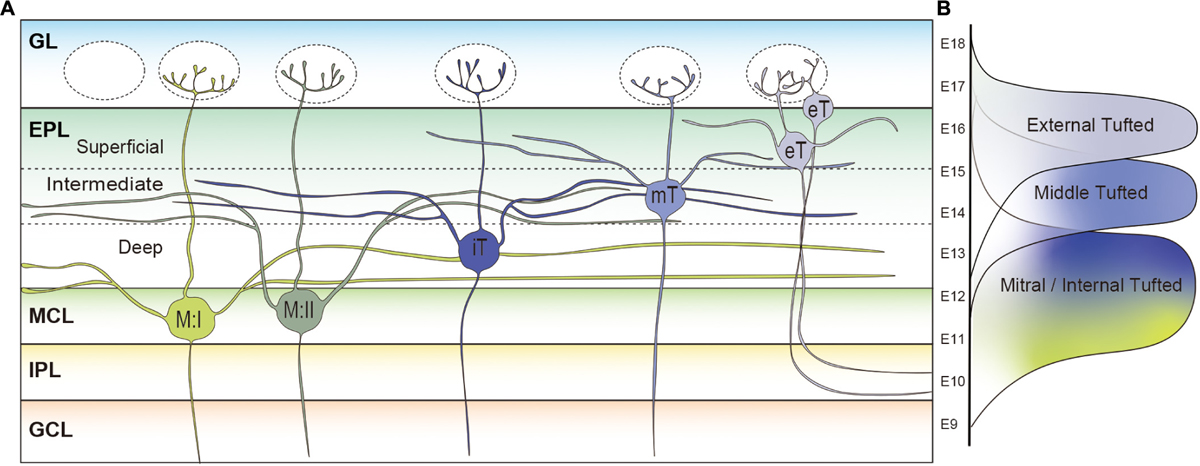
Figure 3. Subpopulations of projection neurons in rodent main olfactory bulb. (A) Morphological differences of projection neurons in the rodent olfactory bulb (OB). Type I mitral cells (M:I) extend their secondary dendrites in the deepest sublayer of the external plexiform layer (dEPL) while those of type II mitral cells (M:II) project to the intermediate EPL (iEPL). Somata of internal tufted cells (iT) are found in the dEPL, and their secondary dendrites extend in the iEPL. Middle tufted cells are mostly located in the iEPL. Tufted cells located in the superficial EPL (sEPL) or glomerular layer (GL) are classified as external tufted cells (eT). The external tufted cells are further subclassified into cells with secondary dendrites projecting to the sEPL or cells absent of secondary dendrites. The deeper the location of projection neurons cell somata in the OB, the longer the secondary dendrites are. (B) Timings of MOB projection neuron generation in the developing mouse brain. Mitral cells are generated between E9 and E13 followed by the middle (E12–E16) and external (E13–E18) tufted cells.
In addition to the differences in length, Figure 3A shows that mitral and tufted cells extend their secondary dendrites in different sublayers of the EPL. The secondary dendrites of mitral cells appear to remain restricted to the deepest portion of the EPL (dEPL), proximal to the MCL, while those of tufted cells extend in the most superficial portion of the EPL (sEPL), proximal to the GL (Macrides and Schneider, 1982; Mori et al., 1983; Orona et al., 1984; Imamura and Greer, 2015). Even among tufted cells, it has been shown that the external tufted cells extend secondary dendrites to the most outer region of the sEPL and the middle tufted cells to the inner region of the sEPL (Macrides and Schneider, 1982; Mori et al., 1983). Several studies defined an intermediate EPL (iEPL) between sEPL and dEPL, where the secondary dendrites of internal tufted cells are projected (Orona et al., 1984; Mouradian and Scott, 1988). As discussed in a later section, there are mitral cells that extend secondary dendrites to the iEPL or the inner sEPL (Orona et al., 1984). Therefore, the regions in the EPL occupied by secondary dendrites of mitral cells and tufted cells gradually shift from deep to superficial. Interestingly, granule cells are also subgrouped into at least three populations based on their dendritic extension patterns in the EPL: the type-I granule cell ramifies spiny dendrites at any depth of the EPL; dendrites of the type-II granule cell extend only in the deep EPL; and the type-III granule cell ramifies spiny dendrites predominantly in the superficial EPL (Schneider and Macrides, 1978; Mori et al., 1983; Orona et al., 1983; Merkle et al., 2007). A computational analysis indicated that lateral inhibition mediated by dendrodendritic synapses between secondary dendrites of projection neurons and granule cell dendrites in the EPL could spread through granule cells only in a mitral-mitral or tufted-tufted way, but not mitral-tufted (Cavarretta et al., 2018). However, it was also shown that individual granule cells could influence a large group of both mitral and tufted cells belonging to at least 15 glomerular modules (Arnson and Strowbridge, 2017).
The activity of OB projection neurons is also regulated by other interneurons, such as short-axon cells (Pressler and Strowbridge, 2006; Parrish-Aungst et al., 2007; Eyre et al., 2008; Eyre et al., 2009; Arenkiel et al., 2011; Burton, 2017; Burton et al., 2017). In the EPL, mitral and tufted cells form reciprocal and non-reciprocal connections with EPL-located interneurons (EPL-IN) expressing parvalbumin (PV), somatostatin (SST), vasoactive intestinal peptide (VIP), and/or Corticotropin-Releasing Hormone (CRH) (Toida et al., 1994; Lepousez et al., 2010; Huang et al., 2013; Matsuno et al., 2017). EPL-INs form broader patterns of connectivity with mitral and tufted cells than granule cells (Huang et al., 2016), which is consistent with the broader odor tuning of EPL-INs (Kato et al., 2013; Miyamichi et al., 2013). Interestingly, CRH-positive EPL-INs provide stronger inhibition onto tufted cells than mitral cells, and tufted cells exhibit more linearly additive responses to odor mixtures without EPL-IN inhibitions (Liu G. et al., 2019). On the other hand, SST-positive EPL-INs are located in the dEPL and extend dendrites specifically into the dEPL (Lepousez et al., 2010). Together with the results showing that lateral inhibition differs between mitral cells and tufted cells (Geramita et al., 2016; Geramita and Urban, 2017; Matsuno et al., 2017), these results suggest that mitral and tufted cell activities are regulated mostly by different inhibitory circuits.
Axons of OB projection neurons ramify both within the OB and in the olfactory cortex (Kishi et al., 1984; Ojima et al., 1984; Orona et al., 1984; Igarashi et al., 2012). Within the OB, the axon collaterals terminate predominantly in the GCL to form asymmetric synapses on somata and dendrites of granule cells and short axon cells (Price and Powell, 1970a, b; Eyre et al., 2008). In the rabbit OB, the collaterals of mitral cells were distributed widely from the deep portion to the most superficial portion of the GCL (Kishi et al., 1984). The collaterals of internal tufted cells tended to be distributed more superficially in the GCL than mitral cells while those of middle tufted cells were distributed in the most superficial GCL (Kishi et al., 1984). It was also reported that the collaterals of external tufted cells run through the IPL to connect lateral and medial sides of the odor map (Liu and Shipley, 1994; Belluscio et al., 2002; Lodovichi et al., 2003). Since granule cells that project their dendrites to the superficial and deep EPL tend to localize in the superficial and deep GCL, respectively (Orona et al., 1983; Imamura et al., 2006), different types of OB projection neurons seem to construct distinct neuronal microcircuits within the OB.
Projection neuron axons extend from the ventrolateral side of the OB and form the LOT before innervating the olfactory cortex (Kishi et al., 1984; Yamatani et al., 2004; Walz et al., 2006; Igarashi et al., 2012). AOB mitral cell axons pass through the deepest layer of the LOT, and the axons of the MOB mitral and tufted cells are found in the intermediate and superficial layers, respectively (Inaki et al., 2004; Yamatani et al., 2004). The location of the axons assists in preserving the topographical organization of the olfactory information as it extends from the OB to the olfactory cortex. Target regions of the AOB mitral cells and MOB mitral and tufted cells rarely overlap (Figure 4). The AOB mitral cells transmit the information from the vomeronasal organ to the bed nucleus of the accessory olfactory tract (BAOT), the BNST, the medial amygdaloid nucleus (MEA), and the posteromedial cortical amygdaloid nucleus (PMCo) (Scalia and Winans, 1975; Davis et al., 1978; Yoshihara et al., 1999; von Campenhausen and Mori, 2000). The MOB mitral and tufted cells innervate the olfactory cortex consisting of the AON, the anterior and posterior piriform cortex (aPC and pPC), the OT, the lateral entorhinal cortex (LEC), the MEA, and the anterior and posterolateral cortical amygdaloid nucleus (ACo and PLCo) (Scalia and Winans, 1975; Haberly and Price, 1977; Yoshihara et al., 1999; Miyamichi et al., 2010; Ghosh et al., 2011; Sosulski et al., 2011; Hintiryan et al., 2012; Igarashi et al., 2012; Hirata et al., 2019). Within the MEA, AOB mitral cell axons terminate in the deep region, and the MOB mitral cell axons are found in the superficial layer without overlap (Kang et al., 2009). Several studies showed that a single mitral cell innervates the entire olfactory cortex while a tufted cell projects only to part of the AON and OT (Nagayama et al., 2010; Igarashi et al., 2012; Hirata et al., 2019). Within the OT, middle tufted cells project to the lateral portion, whereas the medial portion is preferentially innervated by mitral cells (Igarashi et al., 2012). Although it was previously undetermined if external tufted cells project their axons outside the OB (Schoenfeld et al., 1985; Tobin et al., 2010; Lukas et al., 2019), Hirata et al. (2019) recently showed that at least a subpopulation of external tufted cells do target the anterolateral edge of the OT as well as the pars externa of the AON.
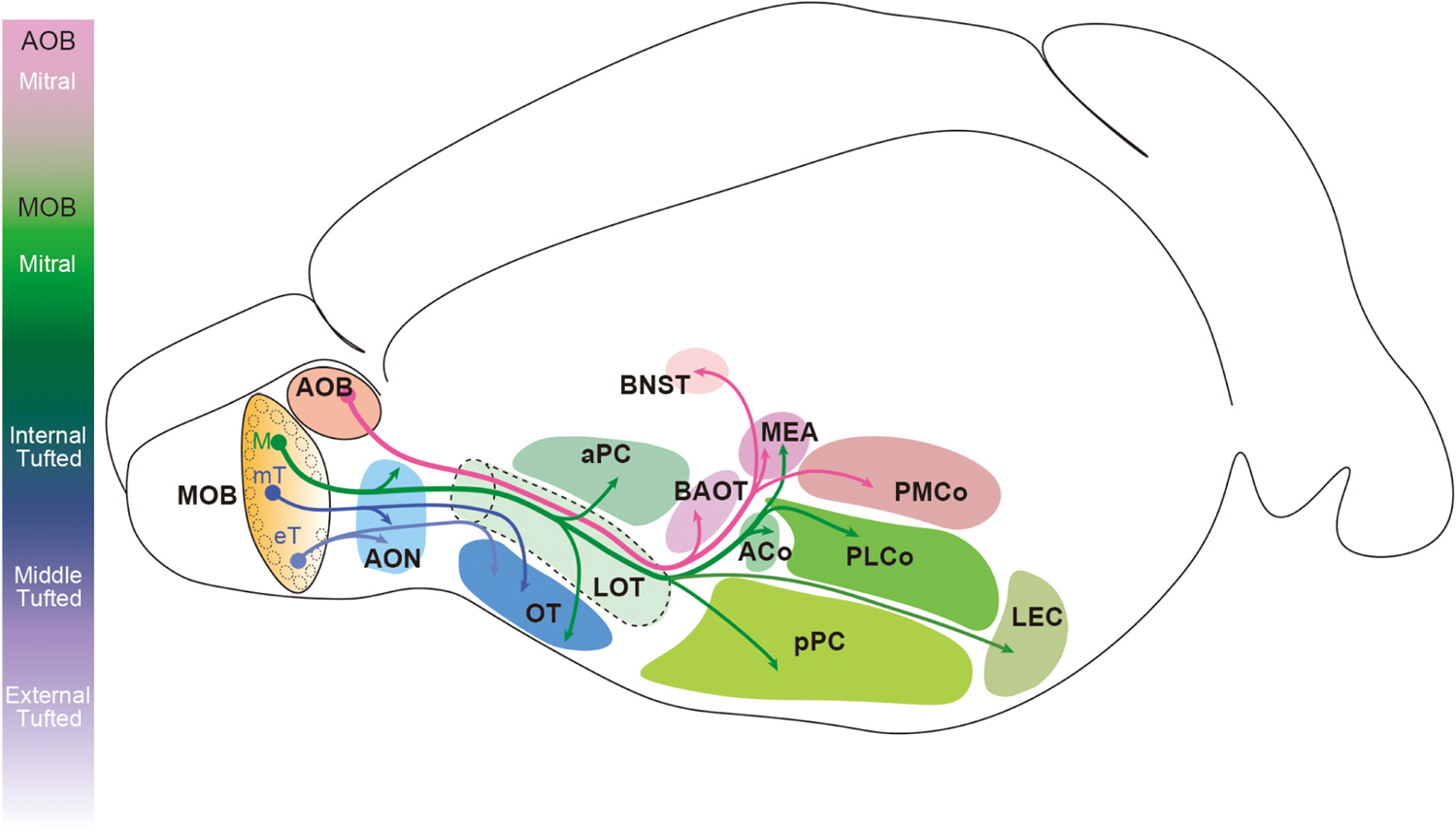
Figure 4. Innervation patterns of olfactory bulb projection neurons in the rodent brain. Mitral cells in the accessory olfactory bulb (AOB) project their axons to the bed nucleus of the accessory olfactory tract (BAOT), the bed nucleus of stria terminalis (BNST), the medial amygdaloid nucleus (MEA), and the posteromedial cortical amygdaloid nucleus (PMCo). Mitral cells (M) in the main olfactory bulb (MOB) innervate the anterior olfactory nucleus (AON), the anterior and posterior piriform cortex (aPC and pPC), the olfactory tubercle (OT), the lateral entorhinal cortex (LEC), the MEA, and the anterior and posterolateral cortical amygdaloid nucleus (ACo and PLCo). However, the axons of tufted cells (mT and eT) project only to the anterior portion of the olfactory cortex including the pars externa of the AON and the anterolateral OT.
Physiological Properties
Odor responses of mitral and tufted cells are regulated by their intrinsic physiological properties and the intraglomerular and interglomerular circuitry (Aungst et al., 2003; Shao et al., 2012, 2013; Adam et al., 2014; Fukunaga et al., 2014). Many studies have reported the dissimilarity in odor responses between mitral and tufted cells. First, a distinct combination of odorants excites or suppresses the output of mitral and tufted cells. Interestingly, a specific odor can inhibit mitral cell excitation, while the external tufted cells show a distinct lack of the odor-specific suppression which is believed to be shaped by interglomerular inhibition (Adam et al., 2014; Kollo et al., 2014; Banerjee et al., 2015; Economo et al., 2016). Molecular receptive range (MRR) is defined as the odor spectrum that activated by a given odorant receptor, glomerulus, or a mitral or tufted cell, and is important for odor discrimination. It was reported that middle tufted cells have a broader MRR than mitral cells (Nagayama et al., 2004; Adam et al., 2014). Similarly, even among the projection neurons targeting the same glomerulus, the deeper the somata location, the narrower the MRRs (Kikuta et al., 2013). Since the MRRs of OB projection neurons are largely regulated by lateral inhibition mediated by the dendrodendritic synapses formed between mitral/tufted cells and granule cells (Yokoi et al., 1995; Tan et al., 2010; Geramita et al., 2016), the length of the secondary dendrites may be a critical determinant of the MRR.
It has been suggested that not only the MRRs but also the temporal components of the projection neuron activity contributes to odor identification (Schaefer and Margrie, 2007; Uchida et al., 2014; Wilson et al., 2017; Blazing and Franks, 2020). There are notable differences between mitral and tufted cells in temporal activation patterns after odor stimulation. Tufted cells can respond to lower concentrations (∼10 times lower than mitral cells) of odor stimuli with a higher frequency (>100 Hz), whereas the typical firing rate of mitral cells is less than 100 Hz (Nagayama et al., 2004; Igarashi et al., 2012; Kikuta et al., 2013; Adam et al., 2014). In vitro studies suggested that the greater excitability of tufted cells is caused by stronger afferent excitation, greater intrinsic excitability, and less inhibitory tone (Schneider and Scott, 1983; Burton and Urban, 2014; Arnson and Strowbridge, 2017; Geramita and Urban, 2017). On the other hand, mitral cells respond to strong OSN stimulation with sustained firing, or persistent discharge, that continues after odor stimulation (Adachi et al., 2005; Matsumoto et al., 2009; Geramita and Urban, 2017; Vaaga and Westbrook, 2017). The timing of firing onset in reference to the respiratory cycle is also different between mitral and tufted cells. Tufted cell spiking is phase-locked to OSN stimulation without sustained firing and starts during the middle of the inhalation phase (early-onset), while mitral cells respond with later-onset during the transition phase from inhalation to exhalation in anesthetized freely breathing rodents (Fukunaga et al., 2012; Igarashi et al., 2012). However, in an artificial inhalation paradigm, superficial, middle, and deep projection neurons were not reliably distinguished based on the timing of their inhalation-evoked activity (Diaz-Quesada et al., 2018; Short and Wachowiak, 2019).
External tufted cells receive direct OSN input and provide feedforward excitation to other neurons in the GL including periglomerular and short-axon cells and therefore are involved in interglomerular suppression of other OB projection neurons (Aungst et al., 2003; Hayar et al., 2004a; Whitesell et al., 2013; Liu and Liu, 2018). In addition, as described in the previous section, at least a subset of external tufted cells target their axons to the anterolateral edge of the OT and the pars externa of the AON (Hirata et al., 2019), suggesting that they contribute to parallel pathways of the olfactory system. Focusing on intrinsic physiological properties, the external tufted cells inherently generate rhythmic theta bursts (1–10 Hz) of action potentials and respond optimally to rhythmic, sniffing-related input (Hayar et al., 2004b; Liu and Shipley, 2008). On the other hand, mitral cells have biphasic membrane potentials that control the responsivity to OSN stimuli (Heyward et al., 2001; Kollo et al., 2014). As suggested from the differences in intrinsic properties, responses to odor stimuli of external tufted cells are distinct from mitral cells (Vaaga and Westbrook, 2016, 2017). Moreover, cholecystokinin (CCK) is a neuropeptide that is known to express strongly in a subset of the external tufted cells (Seroogy et al., 1985; Liu and Shipley, 1994; Gutierrez-Mecinas et al., 2005; Baltanas et al., 2011), although in situ hybridization analysis and recent immunohistochemical studies indicate a weak CCK expression also in mitral cells (Ingram et al., 1989; Hirata et al., 2019). Optical imaging of different mouse OB projection neurons showed that CCK-positive external tufted cells exhibited a shorter range of odor response latencies and durations than mitral cells and other external tufted cell populations (Short and Wachowiak, 2019). Thus, external tufted cells likely transmit the olfactory information to specific regions in the olfactory cortex with unique temporal patterns. On the other hand, vasopressin, a neuropeptide, is predominantly expressed by external tufted cells with secondary dendrites and some middle tufted cells, but not by mitral cells, in the rodent OB (Tobin et al., 2010; Lukas et al., 2019). It is proposed that the vasopressin-positive external tufted cells are involved in mechanisms of social recognition via the odor signatures (Dluzen et al., 1998a, b; Tobin et al., 2010; Wacker et al., 2011). However, recent studies showed that OSN stimulation primarily caused strong inhibition of the vasopressin-positive external tufted cells, suggesting that OSN axons do not directly activate them (Lukas et al., 2019). Whether the vasopressin-positive external tufted cells form a distinct neural circuit/pathway from the other external tufted cells is needed to be further elucidated.
The various types of oscillations of local field potential (LFP) are produced in the OB after odor stimulation, and they are associated with odor perception, discrimination, and learning (Martin et al., 2004; Beshel et al., 2007; Kay et al., 2009; Lepousez and Lledo, 2013; Martin and Ravel, 2014; Li et al., 2015; Liu P. et al., 2020; Losacco et al., 2020). A previous report showed that the local circuits produce fast-frequency (65–100 Hz) and slow-frequency (35–65 Hz) gamma oscillations of LFP in the OB (Kay, 2003). Several reports suggested that the early-onset fast gamma-oscillations and later-onset slow gamma oscillations are generated mainly by tufted cell and mitral cell subsystems, respectively (Manabe and Mori, 2013; Frederick et al., 2016). On the other hand, it was shown that glomerular networks coordinate theta oscillations (2–12 Hz) (Hayar et al., 2004b; Fukunaga et al., 2014). These findings imply that synchronized oscillatory activity at different frequency may be a key mechanism for OB projection neurons to process the different aspects of odor information in parallel.
Heterogeneity of Mitral Cells
Since the MCL is only about the size of one cell body in thickness, heterogeneity of mitral cells has not been deeply investigated. However, several lines of evidence indicate that mitral cells do consist of heterogeneous subpopulations. Although, as noted above, mitral cells usually extend their secondary dendrites in the dEPL (Mori et al., 1983; Orona et al., 1984), some mitral cells extend their secondary dendrites in the iEPL in the rat OB, even though their somata lay in the MCL (Orona et al., 1984; Mouradian and Scott, 1988). Orona et al. (1984) classified the former mitral cells as Type I and the latter as Type II mitral cells (Figure 3A). Interestingly, the total secondary dendrite length of Type II mitral cells was longer than that of the middle tufted cell but shorter than Type I mitral cells (Orona et al., 1984). It remains to be seen whether there is a difference in axonal projection patterns between Type I and Type II mitral cells.
Mitral cells may also be subclassified based on their location along different planes of the OB. For example, mitral cells located at different regions along the dorsomedial-ventrolateral axis in the MCL tend to exhibit different projection patterns toward the OT, cortical amygdala, and MEA (Haberly and Price, 1977; Scott et al., 1980; Miyamichi et al., 2010; Imamura et al., 2011; Inokuchi et al., 2017). Retrograde labeling of OB projection neurons from the olfactory cortex revealed that the cortical amygdala (ACo and PLCo) and OT receive afferent projections preferentially from mitral cells in the dorsomedial and ventrolateral MCL, respectively (Haberly and Price, 1977; Scott et al., 1980; Miyamichi et al., 2010; Imamura et al., 2011). MOB mitral cells that project to the MEA are locally found at the ventral region of the OB and mediate odor-induced attractive social responses (Lin et al., 2007; Thompson et al., 2012; Inokuchi et al., 2017; Lemons et al., 2017).
In contrast to the studies reporting differences between mitral and tufted cells, only a few studies have suggested heterogeneous physiological and molecular properties among mitral cells. It has been shown that the α3 subunit of the GABAA receptor, as well as a subunit of voltage-gated potassium channel (Kv1.2), are expressed by subsets of mitral cells (Panzanelli et al., 2005; Padmanabhan and Urban, 2010). In addition, a subunit of hyperpolarization-activated cyclic nucleotide-gated channel, HCN2, was expressed in glomeruli in a mosaic pattern (Angelo and Margrie, 2011; Angelo et al., 2012). These studies also reported on the diversity of intrinsic biophysical properties among mitral cells, such as firing frequency and the Ih sag current, which are supposedly reflective of varying Kv1.2 and HCN2 expression levels (Padmanabhan and Urban, 2010; Angelo et al., 2012). Moreover, these differences in molecular and biophysical properties may endow mitral cells with different odor response properties (Dhawale et al., 2010; Kikuta et al., 2013).
Implications of Parallel Pathways for Olfactory Processing
Increasing evidence has suggested that mitral and tufted cells differentially transmit olfactory information to the olfactory cortex even when they receive OSN inputs within the same glomerulus. It is noteworthy that the piriform cortex is innervated only by mitral cell axons, and the piriform cortex is one of the major brain regions that serves a critical role in odor encoding, odor identification across different odor concentrations, odor learning, and discrimination and perception of complex odor mixtures (Wilson, 2000; Barnes et al., 2008; Stettler and Axel, 2009; Chapuis and Wilson, 2011; Choi et al., 2011; Haddad et al., 2013; Bolding and Franks, 2017, 2018; Iurilli and Datta, 2017; Roland et al., 2017; Meissner-Bernard et al., 2019); see also reviews in Wilson and Sullivan (2011), Bekkers and Suzuki (2013), Blazing and Franks (2020). The narrower MRR of mitral cells is likely advantageous in the process of accurate odor encoding. However, it is also suggested that mitral cells in the rat MOB do not receive lateral inhibition broadly from surrounding glomeruli via interneurons, but rather receive lateral inhibition from only a small number of spatially distributed glomeruli (Fantana et al., 2008; Shmuel et al., 2019). Moreover, individual odors activate ensembles of spatially distributed neurons in the piriform cortex that lack apparent topographical organization with respect to the odor map (Stettler and Axel, 2009; Miyamichi et al., 2010; Ghosh et al., 2011; Sosulski et al., 2011; Igarashi et al., 2012). Instead, it is proposed that neurons in the piriform cortex stochastically sample glomeruli to generate a systematic population-level representation to identify the odors (Schaffer et al., 2018; Pashkovski et al., 2020). Thus, the patterns of connectivity from mitral cells to neurons in the piriform cortex should be elucidated at the level of synapses, which may identify novel mitral cell subpopulations and pathways.
The tufted cell pathway has a more rapid activation with lower odor concentration compared to mitral cells. This implies that tufted cells may transmit information from the glomeruli to the olfactory cortex with less spatial and temporal modification, and, therefore, may be involved in the olfactory functions in which speed is required for efficient processing. Morphological analyses showed that tufted cells, including the external tufted cells, project to part of the AON and the lateral OT (Nagayama et al., 2010; Igarashi et al., 2012; Hirata et al., 2019). It has been widely argued that the OT is involved in reward and motivational aspects of odor information processing, primarily due to the fact that the OT is a component of the ventral striatum that connects with the reward system, including the ventral tegmental area (Ikemoto, 2007; Wesson and Wilson, 2011; Gadziola et al., 2015; Yamaguchi, 2017; Zhang et al., 2017). It is particularly noteworthy that an odor associated with punishment activates the lateral domain of the OT and induces aversive behavior (Murata et al., 2015; Yamaguchi, 2017). The neural pathway originating from tufted cells may be necessary to escape quickly from these aversive odor sources. In contrast, an odor associated with reward activates the anteromedial domain of the OT and induces attractive behavior (Murata et al., 2015; Yamaguchi, 2017; Zhang et al., 2017), which may be mediated via the mitral cell pathway. Neurons in the AON that receive inputs from most of the MOB projection neurons decussate to the contralateral OB (Schoenfeld et al., 1985; Scott et al., 1985; Illig and Eudy, 2008; Yan et al., 2008). A previous report showed that AON neurons exhibit respiration phase-locked firing pattern to ipsi-nostril stimulation, and this activity is attenuated by contra-nostril stimulation, indicating that the AON is involved in the function of odor source localization (Kikuta et al., 2010; Liu A. et al., 2020). Since a subset of external tufted cells targets the anterolateral edge of the OT as well as the pars externa of the AON (Hirata et al., 2019), this external tufted cell subset may play a critical role in the behaviors induced by reward-related odors and odor source localization.
Another well-known feature of the external tufted cells is to link the isofunctional glomeruli within the OB (Schoenfeld et al., 1985; Liu and Shipley, 1994). It has been known that each rodent OB has two mirror-image OR maps, one in the lateral side and the other in the medial side, within which the two glomeruli representing a particular OR are mapped symmetrically (Nagao et al., 2000). The axon collaterals of an external tufted cell run through the IPL and terminate beneath the mitral cells at the corresponding region of the other side of the two maps (Belluscio et al., 2002; Lodovichi et al., 2003). The axons synapse onto the dendrites of granule cells within the IPL and therefore inhibit the surrounding mitral and tufted cells, which results in mutual inhibition between the lateral and medial maps (Belluscio et al., 2002). Although the functional roles of each map in odor information processing are unknown, a few differences in odor response patterns between glomeruli in these maps have been reported (Zhou and Belluscio, 2008, 2012; Baker et al., 2019; Sato et al., 2020). The functional difference of two maps may be elucidated through further study on the external tufted cells.
Generation of Different Types of OB Projection Neurons
The strategy to assign neurons having different birthdates with different properties is widely used in the brain to generate a variety of neuronal subtypes useful for processing complex information. In the developing retina, all types of cells, including Müller glia, are generated from a single pool of progenitors (Turner and Cepko, 1987; Bassett and Wallace, 2012). The fate of the precursors is largely determined by the timing of neurogenesis, namely that the first neurons born are the RGCs followed by cone photoreceptors, horizontal cells, amacrine cells, rod photoreceptors, and bipolar cells (Bassett and Wallace, 2012). Müller glia are the last of the cells to emerge in the retina. Even among the amacrine cells, it is suggested that the birthdates specify their destinations and subtype identities (Voinescu et al., 2009). The cerebral cortex is made up of six layers, and each layer contains pyramidal projection neurons that possess distinct dendritic morphologies and axonal target regions as well as different molecular expression profiles (Molyneaux et al., 2007; Kwan et al., 2012; Greig et al., 2013; Narayanan et al., 2017; Gerfen et al., 2018). The mouse cortical pyramidal neurons are generated between E11 and E18, and the neurons with different birthdates migrate toward distinct layers with an inside-out manner (Molyneaux et al., 2007; Kwan et al., 2012; Greig et al., 2013).
Similarly, the timing of neurogenesis is a major contributor to producing diversity in the OB projection neurons. The earliest generated projection neurons in the mouse OB are the AOB mitral cells that emerge around embryonic day (E) 9 and 10 (Hinds, 1968; Imamura and Greer, 2015; Hirata et al., 2019). A recent study indicated that the AOB and MOB projection neurons are generated from different progenitor cells whereas a single progenitor cell can give rise to both MOB mitral and tufted cells in the developing mouse OB (Sanchez-Guardado and Lois, 2019). Nevertheless, MOB mitral and tufted cells are generated at different time points; mitral cells are generated between E9 and E13 having a peak at E11, while middle and external tufted cells are born during a later period between E12 and E18 (Hinds, 1968; Blanchart et al., 2006; Imamura et al., 2011; Hirata et al., 2019) (Figure 3B). Thymidine analog labeling and genetic tracing experiments have shown that middle tufted cells are generated earlier than external tufted cells (Hinds, 1968; Winpenny et al., 2011; Hirata et al., 2019). Therefore, similar to cortical pyramidal neurons, projection neurons in different layers are also generated at different time points with an inside-out manner in the MOB.
Using the thymidine analog labeling method, we also showed that mitral cells generated at E9 or E10 (early-generated mitral cells) were preferentially localized to the dorsomedial MCL, while mitral cells generated at E12 or E13 (late-generated mitral cells) were predominantly located in ventrolateral MCL in the mouse OB (Imamura et al., 2011). Later, we further revealed that early- and late-generated mitral cells extend their secondary dendrites in the dEPL and iEPL, respectively, indicating that late-generated mitral cells can be classified as the previously identified Type II mitral cells (Orona et al., 1984; Imamura and Greer, 2015). These results strongly suggest that neuronal birthdate is a significant contributor in the generation of morphological differences, not only between mitral and tufted cells, but also among subpopulations of mitral cells. Based on mitral cell location contributing to the projection pattern of target structures in the olfactory cortex, neuronal birthdate may also be considered an implication of a cells function. However, a critical next step is to determine whether there are differences in physiological and/or molecular properties between early- and late-generated mitral cells.
In summary, the olfactory system processes multiple aspects of olfactory information through parallel pathways. Similar to the visual system, the diversity of OB projection neurons provides the basis for the parallel pathways in the rodent OB, which has been established throughout the course of evolution. In the retina, distinct RGC types have been characterized by dendritic and axonal arborization patterns as well as physiological parameters. More recently, molecular expression patterns, including transcription factors, have been used to examine the diversity of RGCs (Sanes and Masland, 2015; Baden et al., 2016; Rheaume et al., 2018). In this review, we have summarized the morphological and physiological diversities of OB projection neurons. Although differing molecular expression profiles of OB projection neurons in the rodent OB have yet to be identified, the timing of neurogenesis seems to regulate the generation of different projection neuron subpopulations. Thus far, a large number of transcription factors expressed in developing projection neurons in the rodent OB have been reported (Winpenny et al., 2011; Imamura and Greer, 2013), and we and others showed that each transcription factor appears in the developing OB with a distinct spatiotemporal pattern (Williams et al., 2007; Campbell et al., 2011; Nguyen and Imamura, 2019). In addition, the results from large-scale analyses using omics approaches are available (Campbell et al., 2011; Kawasawa et al., 2016). A combination of cutting edge techniques including single-cell RNA-sequencing, tissue-clearing and whole-brain imaging, optical imaging, and electrophysiological recordings can now be used to reveal molecular, morphological, and physiological properties of OB projection neurons. The knowledge acquired by these techniques will further elucidate the functions and ramifications of each pathway.
Author Contributions
FI, AI, and BL reviewed the literatures and wrote the review. All authors contributed to the article and approved the submitted version.
Funding
This work was supported by National Institute on Deafness and Other Communication Disorders (NIDCD) R01DC016307 to FI.
Conflict of Interest
The authors declare that the research was conducted in the absence of any commercial or financial relationships that could be construed as a potential conflict of interest.
Acknowledgments
We thank Dr. Charles Greer for his helpful comments on the earlier version of this manuscript.
References
Adachi, N., Kohara, K., and Tsumoto, T. (2005). Difference in trafficking of brain-derived neurotrophic factor between axons and dendrites of cortical neurons, revealed by live-cell imaging. BMC Neurosci. 6:42. doi: 10.1186/1471-2202-6-42
Adam, Y., Livneh, Y., Miyamichi, K., Groysman, M., Luo, L., and Mizrahi, A. (2014). Functional transformations of odor inputs in the mouse olfactory bulb. Front. Neural Circuits 8:129. doi: 10.3389/fncir.2014.00129
Ahuja, G., Ivandic, I., Salturk, M., Oka, Y., Nadler, W., and Korsching, S. I. (2013). Zebrafish crypt neurons project to a single, identified mediodorsal glomerulus. Sci. Rep. 3:2063.
Angelo, K., and Margrie, T. W. (2011). Population diversity and function of hyperpolarization-activated current in olfactory bulb mitral cells. Sci. Rep. 1:50.
Angelo, K., Rancz, E. A., Pimentel, D., Hundahl, C., Hannibal, J., Fleischmann, A., et al. (2012). A biophysical signature of network affiliation and sensory processing in mitral cells. Nature 488, 375–378. doi: 10.1038/nature11291
Antal, M., Eyre, M., Finklea, B., and Nusser, Z. (2006). External tufted cells in the main olfactory bulb form two distinct subpopulations. Eur. J. Neurosci. 24, 1124–1136.
Arctander, S. (1969). Perfume and Flavor Chemicals: (aroma Chemicals). Carol Stream, IL: Allured Publishing Corporation.
Arenkiel, B. R., Hasegawa, H., Yi, J. J., Larsen, R. S., Wallace, M. L., Philpot, B. D., et al. (2011). Activity-induced remodeling of olfactory bulb microcircuits revealed by monosynaptic tracing. PLoS One 6:e29423. doi: 10.1371/journal.pone.0029423
Arnson, H. A., and Strowbridge, B. W. (2017). Spatial structure of synchronized inhibition in the olfactory bulb. J. Neurosci. 37, 10468–10480. doi: 10.1523/jneurosci.1004-17.2017
Aungst, J. L., Heyward, P. M., Puche, A. C., Karnup, S. V., Hayar, A., Szabo, G., et al. (2003). Centre-surround inhibition among olfactory bulb glomeruli. Nature 426, 623–629.
Baden, T., Berens, P., Franke, K., Roman Roson, M., Bethge, M., and Euler, T. (2016). The functional diversity of retinal ganglion cells in the mouse. Nature 529, 345–350. doi: 10.1038/nature16468
Baden, T., Euler, T., and Berens, P. (2020). Understanding the retinal basis of vision across species. Nat. Rev. Neurosci. 21, 5–20. doi: 10.1038/s41583-019-0242-1
Baker, K. L., Vasan, G., Gumaste, A., Pieribone, V. A., and Verhagen, J. V. (2019). Spatiotemporal dynamics of odor responses in the lateral and dorsal olfactory bulb. PLoS Biol. 17:e3000409. doi: 10.1371/journal.pbio.3000409
Baltanas, F. C., Curto, G. G., Gomez, C., Diaz, D., Murias, A. R., Crespo, C., et al. (2011). Types of cholecystokinin-containing periglomerular cells in the mouse olfactory bulb. J. Neurosci. Res. 89, 35–43. doi: 10.1002/jnr.22521
Banerjee, A., Marbach, F., Anselmi, F., Koh, M. S., Davis, M. B., Garcia Da Silva, P., et al. (2015). An interglomerular circuit gates glomerular output and implements gain control in the mouse olfactory bulb. Neuron 87, 193–207. doi: 10.1016/j.neuron.2015.06.019
Barber, P. C., Parry, D. M., Field, P. M., and Raisman, G. (1978). Electron microscope autoradiographic evidence for specific transneuronal transport in the mouse accessory olfactory bulb. Brain Res. 152, 283–302. doi: 10.1016/0006-8993(78)90256-1
Barnes, D. C., Hofacer, R. D., Zaman, A. R., Rennaker, R. L., and Wilson, D. A. (2008). Olfactory perceptual stability and discrimination. Nat. Neurosci. 11, 1378–1380. doi: 10.1038/nn.2217
Bassett, E. A., and Wallace, V. A. (2012). Cell fate determination in the vertebrate retina. Trends Neurosci. 35, 565–573. doi: 10.1016/j.tins.2012.05.004
Bear, D. M., Lassance, J. M., Hoekstra, H. E., and Datta, S. R. (2016). The evolving neural and genetic architecture of vertebrate olfaction. Curr. Biol. 26, R1039–R1049.
Bekkers, J. M., and Suzuki, N. (2013). Neurons and circuits for odor processing in the piriform cortex. Trends Neurosci. 36, 429–438. doi: 10.1016/j.tins.2013.04.005
Belluscio, L., Koentges, G., Axel, R., and Dulac, C. (1999). A map of pheromone receptor activation in the mammalian brain. Cell 97, 209–220. doi: 10.1016/s0092-8674(00)80731-x
Belluscio, L., Lodovichi, C., Feinstein, P., Mombaerts, P., and Katz, L. C. (2002). Odorant receptors instruct functional circuitry in the mouse olfactory bulb. Nature 419, 296–300. doi: 10.1038/nature01001
Beshel, J., Kopell, N., and Kay, L. M. (2007). Olfactory bulb gamma oscillations are enhanced with task demands. J. Neurosci. 27, 8358–8365. doi: 10.1523/jneurosci.1199-07.2007
Biechl, D., Tietje, K., Gerlach, G., and Wullimann, M. F. (2016). Crypt cells are involved in kin recognition in larval zebrafish. Sci. Rep. 6:24590.
Biechl, D., Tietje, K., Ryu, S., Grothe, B., Gerlach, G., and Wullimann, M. F. (2017). Identification of accessory olfactory system and medial amygdala in the zebrafish. Sci. Rep. 7:44295.
Blanchart, A., De Carlos, J. A., and López-Mascaraque, L. (2006). Time frame of mitral cell development in the mice olfactory bulb. J. Comp. Neurol. 496, 529–543. doi: 10.1002/cne.20941
Blazing, R. M., and Franks, K. M. (2020). Odor coding in piriform cortex: mechanistic insights into distributed coding. Curr. Opin. Neurobiol. 64, 96–102. doi: 10.1016/j.conb.2020.03.001
Bolding, K. A., and Franks, K. M. (2017). Complementary codes for odor identity and intensity in olfactory cortex. eLife 6:e22630.
Bolding, K. A., and Franks, K. M. (2018). Recurrent cortical circuits implement concentration-invariant odor coding. Science 361:eaat6904. doi: 10.1126/science.aat6904
Boschat, C., Pelofi, C., Randin, O., Roppolo, D., Luscher, C., Broillet, M. C., et al. (2002). Pheromone detection mediated by a V1r vomeronasal receptor. Nat. Neurosci. 5, 1261–1262. doi: 10.1038/nn978
Buck, L., and Axel, R. (1991). A novel multigene family may encode odorant receptors: a molecular basis for odor recognition. Cell 65, 175–187. doi: 10.1016/0092-8674(91)90418-x
Burton, S. D. (2017). Inhibitory circuits of the mammalian main olfactory bulb. J. Neurophysiol. 118, 2034–2051. doi: 10.1152/jn.00109.2017
Burton, S. D., Larocca, G., Liu, A., Cheetham, C. E., and Urban, N. N. (2017). Olfactory bulb deep short-axon cells mediate widespread inhibition of tufted cell apical dendrites. J. Neurosci. 37, 1117–1138. doi: 10.1523/jneurosci.2880-16.2016
Burton, S. D., and Urban, N. N. (2014). Greater excitability and firing irregularity of tufted cells underlies distinct afferent-evoked activity of olfactory bulb mitral and tufted cells. J. Physiol. 592, 2097–2118. doi: 10.1113/jphysiol.2013.269886
Byrd, C. A., and Burd, G. D. (1991). Development of the olfactory bulb in the clawed frog, Xenopus laevis: a morphological and quantitative analysis. J. Comp. Neurol. 314, 79–90. doi: 10.1002/cne.903140108
Campbell, G. R., Baudhuin, A., Vranizan, K., and Ngai, J. (2011). Transcription factors expressed in olfactory bulb local progenitor cells revealed by genome-wide transcriptome profiling. Mol. Cell Neurosci. 46, 548–561. doi: 10.1016/j.mcn.2010.12.012
Cavarretta, F., Burton, S. D., Igarashi, K. M., Shepherd, G. M., Hines, M. L., and Migliore, M. (2018). Parallel odor processing by mitral and middle tufted cells in the olfactory bulb. Sci. Rep. 8:7625.
Chapuis, J., and Wilson, D. A. (2011). Bidirectional plasticity of cortical pattern recognition and behavioral sensory acuity. Nat. Neurosci. 15, 155–161. doi: 10.1038/nn.2966
Chess, A., Simon, I., Cedar, H., and Axel, R. (1994). Allelic inactivation regulates olfactory receptor gene expression. Cell 78, 823–834. doi: 10.1016/s0092-8674(94)90562-2
Choi, G. B., Stettler, D. D., Kallman, B. R., Bhaskar, S. T., Fleischmann, A., and Axel, R. (2011). Driving opposing behaviors with ensembles of piriform neurons. Cell 146, 1004–1015. doi: 10.1016/j.cell.2011.07.041
Davis, B. J., Macrides, F., Youngs, W. M., Schneider, S. P., and Rosene, D. L. (1978). Efferents and centrifugal afferents of the main and accessory olfactory bulbs in the hamster. Brain Res. Bull. 3, 59–72. doi: 10.1016/0361-9230(78)90062-x
Del Punta, K., Leinders-Zufall, T., Rodriguez, I., Jukam, D., Wysocki, C. J., Ogawa, S., et al. (2002). Deficient pheromone responses in mice lacking a cluster of vomeronasal receptor genes. Nature 419, 70–74. doi: 10.1038/nature00955
Dewan, A., Pacifico, R., Zhan, R., Rinberg, D., and Bozza, T. (2013). Non-redundant coding of aversive odours in the main olfactory pathway. Nature 497, 486–489. doi: 10.1038/nature12114
Dhawale, A. K., Hagiwara, A., Bhalla, U. S., Murthy, V. N., and Albeanu, D. F. (2010). Non-redundant odor coding by sister mitral cells revealed by light addressable glomeruli in the mouse. Nat. Neurosci. 13, 1404–1412. doi: 10.1038/nn.2673
Diaz-Quesada, M., Youngstrom, I. A., Tsuno, Y., Hansen, K. R., Economo, M. N., and Wachowiak, M. (2018). Inhalation frequency controls reformatting of mitral/tufted cell odor representations in the olfactory bulb. J. Neurosci. 38, 2189–2206. doi: 10.1523/jneurosci.0714-17.2018
Dluzen, D. E., Muraoka, S., Engelmann, M., and Landgraf, R. (1998a). The effects of infusion of arginine vasopressin, oxytocin, or their antagonists into the olfactory bulb upon social recognition responses in male rats. Peptides 19, 999–1005. doi: 10.1016/s0196-9781(98)00047-3
Dluzen, D. E., Muraoka, S., and Landgraf, R. (1998b). Olfactory bulb norepinephrine depletion abolishes vasopressin and oxytocin preservation of social recognition responses in rats. Neurosci. Lett. 254, 161–164. doi: 10.1016/s0304-3940(98)00691-0
Dryer, L., and Graziadei, P. P. (1994). Projections of the olfactory bulb in an elasmobranch fish, Sphyrna tiburo: segregation of inputs in the telencephalon. Anat. Embryol. 190, 563–572.
Economo, M. N., Hansen, K. R., and Wachowiak, M. (2016). Control of Mitral/Tufted cell output by selective inhibition among olfactory bulb glomeruli. Neuron 91, 397–411. doi: 10.1016/j.neuron.2016.06.001
Eisthen, H., and Polese, G. (2010). Evolution of vertebrate olfactory subsystems. evolution of nervous systems. 2. Non Mammalian Vertebrates 2, 355–406. doi: 10.1016/b0-12-370878-8/00142-7
Ennis, M., and Hayar, A. (2008). “Physiology of the main olfactory bulb,” in The Senses: A Comprehensive Reference, eds R. H. Masland, T. D. Albright, T. D. Albright, R. H. Masland, P. Dallos, D. Oertel, et al. (New York, NY: Academic Press), 641–686. doi: 10.1016/b978-012370880-9.00118-3
Ennis, M., Puche, A. C., Holy, T., and Shipley, M. T. (2015). “Chapter 27 - the olfactory system,” in The Rat Nervous System, 4th Edn, ed. G. Paxinos (San Diego: Academic Press), 761–803.
Eyre, M. D., Antal, M., and Nusser, Z. (2008). Distinct deep short-axon cell subtypes of the main olfactory bulb provide novel intrabulbar and extrabulbar GABAergic connections. J. Neurosci. 28, 8217–8229. doi: 10.1523/jneurosci.2490-08.2008
Eyre, M. D., Kerti, K., and Nusser, Z. (2009). Molecular diversity of deep short-axon cells of the rat main olfactory bulb. Eur. J. Neurosci. 29, 1397–1407. doi: 10.1111/j.1460-9568.2009.06703.x
Fantana, A. L., Soucy, E. R., and Meister, M. (2008). Rat olfactory bulb mitral cells receive sparse glomerular inputs. Neuron 59, 802–814. doi: 10.1016/j.neuron.2008.07.039
Figueres-Onate, M., Gutierrez, Y., and Lopez-Mascaraque, L. (2014). Unraveling Cajal’s view of the olfactory system. Front. Neuroanat. 8:55. doi: 10.3389/fnana.2014.00055
Fletcher, M. L. (2011). Analytical processing of binary mixture information by olfactory bulb glomeruli. PLoS One 6:e29360. doi: 10.1371/journal.pone.0029360
Frederick, D. E., Brown, A., Brim, E., Mehta, N., Vujovic, M., and Kay, L. M. (2016). Gamma and beta oscillations define a sequence of neurocognitive modes present in odor processing. J. Neurosci. 36, 7750–7767. doi: 10.1523/jneurosci.0569-16.2016
Fujimoto, S., Leiwe, M. N., Sakaguchi, R., Muroyama, Y., Kobayakawa, R., Kobayakawa, K., et al. (2019). Spontaneous activity generated within the olfactory bulb establishes the discrete wiring of mitral cell dendrites. bioRxiv [Preprint]. doi: 10.1101/625616
Fujita, I., Satou, M., and Ueda, K. (1988). Morphology of physiologically identified mitral cells in the carp olfactory bulb: a light microscopic study after intracellular staining with horseradish peroxidase. J. Comp. Neurol. 267, 253–268. doi: 10.1002/cne.902670208
Fukunaga, I., Berning, M., Kollo, M., Schmaltz, A., and Schaefer, A. T. (2012). Two distinct channels of olfactory bulb output. Neuron 75, 320–329. doi: 10.1016/j.neuron.2012.05.017
Fukunaga, I., Herb, J. T., Kollo, M., Boyden, E. S., and Schaefer, A. T. (2014). Independent control of gamma and theta activity by distinct interneuron networks in the olfactory bulb. Nat. Neurosci. 17, 1208–1216. doi: 10.1038/nn.3760
Fuller, C. L., and Byrd, C. A. (2005). Ruffed cells identified in the adult zebrafish olfactory bulb. Neurosci. Lett. 379, 190–194. doi: 10.1016/j.neulet.2004.12.062
Fuller, C. L., Yettaw, H. K., and Byrd, C. A. (2006). Mitral cells in the olfactory bulb of adult zebrafish (Danio rerio): morphology and distribution. J. Comp. Neurol. 499, 218–230. doi: 10.1002/cne.21091
Gadziola, M. A., Tylicki, K. A., Christian, D. L., and Wesson, D. W. (2015). The olfactory tubercle encodes odor valence in behaving mice. J. Neurosci. 35, 4515–4527. doi: 10.1523/jneurosci.4750-14.2015
Gautam, S. H., Short, S. M., and Verhagen, J. V. (2014). Retronasal odor concentration coding in glomeruli of the rat olfactory bulb. Front. Integr. Neurosci. 8:81. doi: 10.3389/fnint.2014.00081
Geramita, M., and Urban, N. N. (2017). Differences in glomerular-layer-mediated feedforward inhibition onto mitral and tufted cells lead to distinct modes of intensity coding. J. Neurosci. 37, 1428–1438. doi: 10.1523/jneurosci.2245-16.2016
Geramita, M. A., Burton, S. D., and Urban, N. N. (2016). Distinct lateral inhibitory circuits drive parallel processing of sensory information in the mammalian olfactory bulb. eLife 5:e16039.
Gerfen, C. R., Economo, M. N., and Chandrashekar, J. (2018). Long distance projections of cortical pyramidal neurons. J. Neurosci. Res. 96, 1467–1475. doi: 10.1002/jnr.23978
Gerlach, G., Tietje, K., Biechl, D., Namekawa, I., Schalm, G., and Sulmann, A. (2019). Behavioural and neuronal basis of olfactory imprinting and kin recognition in larval fish. J. Exp. Biol. 222(Pt Suppl. 1):jeb189746. doi: 10.1242/jeb.189746
Ghosh, S., Larson, S. D., Hefzi, H., Marnoy, Z., Cutforth, T., Dokka, K., et al. (2011). Sensory maps in the olfactory cortex defined by long-range viral tracing of single neurons. Nature 472, 217–220. doi: 10.1038/nature09945
Gilbert, L. A., Larson, M. H., Morsut, L., Liu, Z., Brar, G. A., Torres, S. E., et al. (2013). CRISPR-mediated modular RNA-guided regulation of transcription in eukaryotes. Cell 154, 442–451. doi: 10.1016/j.cell.2013.06.044
Godfrey, P. A., Malnic, B., and Buck, L. B. (2004). The mouse olfactory receptor gene family. Proc. Natl. Acad. Sci. U.S.A. 101, 2156–2161. doi: 10.1073/pnas.0308051100
Gorin, M., Tsitoura, C., Kahan, A., Watznauer, K., Drose, D. R., Arts, M., et al. (2016). Interdependent conductances drive infraslow intrinsic rhythmogenesis in a subset of accessory olfactory bulb projection neurons. J. Neurosci. 36, 3127–3144. doi: 10.1523/jneurosci.2520-15.2016
Greer, C. A., Whitman, M. C., Rela, L., Imamura, F., and Rodriguez Gil, D. (2008). “4.36 - architecture of the olfactory bulb,” in The Senses: A Comprehensive Reference, eds R. H. Masland, T. D. Albright, T. D. Albright, R. H. Masland, P. Dallos, D. Oertel, et al. (New York, NY: Academic Press), 623–640.
Greig, L. C., Woodworth, M. B., Galazo, M. J., Padmanabhan, H., and Macklis, J. D. (2013). Molecular logic of neocortical projection neuron specification, development and diversity. Nat. Rev. Neurosci. 14, 755–769. doi: 10.1038/nrn3586
Gutierrez-Mecinas, M., Crespo, C., Blasco-Ibanez, J. M., Gracia-Llanes, F. J., Marques-Mari, A. I., and Martinez-Guijarro, F. J. (2005). Characterization of somatostatin- and cholecystokinin-immunoreactive periglomerular cells in the rat olfactory bulb. J. Comp. Neurol. 489, 467–479. doi: 10.1002/cne.20649
Haberly, L. B., and Price, J. L. (1977). The axonal projection patterns of the mitral and tufted cells of the olfactory bulb in the rat. Brain Res. 129, 152–157. doi: 10.1016/0006-8993(77)90978-7
Haddad, R., Lanjuin, A., Madisen, L., Zeng, H., Murthy, V. N., and Uchida, N. (2013). Olfactory cortical neurons read out a relative time code in the olfactory bulb. Nat. Neurosci. 16, 949–957. doi: 10.1038/nn.3407
Halpern, M. (1976). The efferent connections of the olfactory bulb and accessory olfactory bulb in the snakes, Thamnophis sirtalis and Thamnophis radix. J. Morphol. 150, 553–578. doi: 10.1002/jmor.1976.150.2.553
Hassenklover, T., and Manzini, I. (2013). Olfactory wiring logic in amphibians challenges the basic assumptions of the unbranched axon concept. J. Neurosci. 33, 17247–17252. doi: 10.1523/jneurosci.2755-13.2013
Hayar, A., Karnup, S., Ennis, M., and Shipley, M. T. (2004a). External tufted cells: a major excitatory element that coordinates glomerular activity. J. Neurosci. 24, 6676–6685. doi: 10.1523/jneurosci.1367-04.2004
Hayar, A., Karnup, S., Shipley, M. T., and Ennis, M. (2004b). Olfactory bulb glomeruli: external tufted cells intrinsically burst at theta frequency and are entrained by patterned olfactory input. J. Neurosci. 24, 1190–1199. doi: 10.1523/jneurosci.4714-03.2004
Herrick, C. J. (1924). The amphibian forebrain. II. The olfactory bulb of Amblystoma. J. Comp. Neurol. 37, 373–396. doi: 10.1002/cne.900370303
Heyward, P., Ennis, M., Keller, A., and Shipley, M. T. (2001). Membrane bistability in olfactory bulb mitral cells. J. Neurosci. 21, 5311–5320. doi: 10.1523/jneurosci.21-14-05311.2001
Hinds, J. W. (1968). Autoradiographic study of histogenesis in the mouse olfactory bulb. I. Time of origin of neurons and neuroglia. J. Comp. Neurol. 134, 287–304. doi: 10.1002/cne.901340304
Hintiryan, H., Gou, L., Zingg, B., Yamashita, S., Lyden, H. M., Song, M. Y., et al. (2012). Comprehensive connectivity of the mouse main olfactory bulb: analysis and online digital atlas. Front. Neuroanat. 6:30. doi: 10.3389/fnana.2012.00030
Hirata, T., Shioi, G., Abe, T., Kiyonari, H., Kato, S., Kobayashi, K., et al. (2019). A novel birthdate-labeling method reveals segregated parallel projections of mitral and external tufted cells in the main olfactory system. eNeuro 6:ENEURO.0234-19.2019. doi: 10.1523/ENEURO.0234-19.2019
Holy, T. E. (2018). The accessory olfactory system: innately specialized or microcosm of mammalian circuitry? Annu. Rev. Neurosci. 41, 501–525. doi: 10.1146/annurev-neuro-080317-061916
Huang, L., Garcia, I., Jen, H. I., and Arenkiel, B. R. (2013). Reciprocal connectivity between mitral cells and external plexiform layer interneurons in the mouse olfactory bulb. Front. Neural. Circ. 7:32. doi: 10.3389/fncir.2013.00032
Huang, L., Ung, K., Garcia, I., Quast, K. B., Cordiner, K., Saggau, P., et al. (2016). Task learning promotes plasticity of interneuron connectivity maps in the olfactory bulb. J. Neurosci. 36, 8856–8871. doi: 10.1523/jneurosci.0794-16.2016
Igarashi, K. M., Ieki, N., An, M., Yamaguchi, Y., Nagayama, S., Kobayakawa, K., et al. (2012). Parallel mitral and tufted cell pathways route distinct odor information to different targets in the olfactory cortex. J. Neurosci. 32, 7970–7985. doi: 10.1523/jneurosci.0154-12.2012
Ikemoto, S. (2007). Dopamine reward circuitry: two projection systems from the ventral midbrain to the nucleus accumbens-olfactory tubercle complex. Brain Res. Rev. 56, 27–78. doi: 10.1016/j.brainresrev.2007.05.004
Illig, K. R., and Eudy, J. D. (2008). Contralateral projections of the rat anterior olfactory nucleus. J. Comp. Neurol. 512, 115–123. doi: 10.1002/cne.21900
Imai, T. (2014). Construction of functional neuronal circuitry in the olfactory bulb. Semin. Cell Dev. Biol. 35, 180–188. doi: 10.1016/j.semcdb.2014.07.012
Imamura, F., Ayoub, A. E., Rakic, P., and Greer, C. A. (2011). Timing of neurogenesis is a determinant of olfactory circuitry. Nat. Neurosci. 14, 331–337. doi: 10.1038/nn.2754
Imamura, F., and Greer, C. A. (2013). Pax6 regulates Tbr1 and Tbr2 expressions in olfactory bulb mitral cells. Mol. Cell Neurosci. 54, 58–70. doi: 10.1016/j.mcn.2013.01.002
Imamura, F., and Greer, C. A. (2015). Segregated labeling of olfactory bulb projection neurons based on their birthdates. Eur. J. Neurosci. 41, 147–156. doi: 10.1111/ejn.12784
Imamura, F., Nagao, H., Naritsuka, H., Murata, Y., Taniguchi, H., and Mori, K. (2006). A leucine-rich repeat membrane protein, 5T4, is expressed by a subtype of granule cells with dendritic arbors in specific strata of the mouse olfactory bulb. J. Comp. Neurol. 495, 754–768. doi: 10.1002/cne.20896
Inaki, K., Nishimura, S., Nakashiba, T., Itohara, S., and Yoshihara, Y. (2004). Laminar organization of the developing lateral olfactory tract revealed by differential expression of cell recognition molecules. J. Comp. Neurol. 479, 243–256. doi: 10.1002/cne.20270
Ingram, S. M., Krause, R. G. II, Baldino, F. Jr., Skeen, L. C., and Lewis, M. E. (1989). Neuronal localization of cholecystokinin mRNA in the rat brain by using in situ hybridization histochemistry. J. Comp. Neurol. 287, 260–272. doi: 10.1002/cne.902870209
Inokuchi, K., Imamura, F., Takeuchi, H., Kim, R., Okuno, H., Nishizumi, H., et al. (2017). Nrp2 is sufficient to instruct circuit formation of mitral-cells to mediate odour-induced attractive social responses. Nat. Commun. 8:15977.
Isosaka, T., Matsuo, T., Yamaguchi, T., Funabiki, K., Nakanishi, S., Kobayakawa, R., et al. (2015). Htr2a-expressing cells in the central amygdala control the hierarchy between innate and learned fear. Cell 163, 1153–1164. doi: 10.1016/j.cell.2015.10.047
Iurilli, G., and Datta, S. R. (2017). Population coding in an innately relevant olfactory area. Neuron 93, 1180.e7–1197.e7.
Iwahori, N., Nakamura, K., and Mameya, C. (1989). A Golgi study on the main olfactory bulb in the snake Elaphe quadrivirgata. Neurosci. Res. 6, 411–425. doi: 10.1016/0168-0102(89)90003-5
Jiang, T., and Holley, A. (1992). Morphological variations among output neurons of the olfactory bulb in the frog (Rana ridibunda). J. Comp. Neurol. 320, 86–96. doi: 10.1002/cne.903200106
Johnson, B. A., and Leon, M. (2000). Modular representations of odorants in the glomerular layer of the rat olfactory bulb and the effects of stimulus concentration. J. Comp. Neurol. 422, 496–509. doi: 10.1002/1096-9861(20000710)422:4<496::aid-cne2>3.0.co;2-4
Johnson, B. A., and Leon, M. (2007). Chemotopic odorant coding in a mammalian olfactory system. J. Comp. Neurol. 503, 1–34. doi: 10.1002/cne.21396
Kang, N., Baum, M. J., and Cherry, J. A. (2009). A direct main olfactory bulb projection to the ‘vomeronasal’ amygdala in female mice selectively responds to volatile pheromones from males. Eur. J. Neurosci. 29, 624–634. doi: 10.1111/j.1460-9568.2009.06638.x
Kato, H. K., Gillet, S. N., Peters, A. J., Isaacson, J. S., and Komiyama, T. (2013). Parvalbumin-expressing interneurons linearly control olfactory bulb output. Neuron 80, 1218–1231. doi: 10.1016/j.neuron.2013.08.036
Kawasawa, Y. I., Salzberg, A. C., Li, M., Sestan, N., Greer, C. A., and Imamura, F. (2016). RNA-seq analysis of developing olfactory bulb projection neurons. Mol. Cell Neurosci. 74, 78–86. doi: 10.1016/j.mcn.2016.03.009
Kay, L. M. (2003). Two species of gamma oscillations in the olfactory bulb: dependence on behavioral state and synaptic interactions. J. Integr. Neurosci. 2, 31–44. doi: 10.1142/s0219635203000196
Kay, L. M., Beshel, J., Brea, J., Martin, C., Rojas-Libano, D., and Kopell, N. (2009). Olfactory oscillations: the what, how and what for. Trends Neurosci. 32, 207–214. doi: 10.1016/j.tins.2008.11.008
Kay, L. M., Crk, T., and Thorngate, J. (2005). A redefinition of odor mixture quality. Behav. Neurosci. 119, 726–733. doi: 10.1037/0735-7044.119.3.726
Kermen, F., Franco, L. M., Wyatt, C., and Yaksi, E. (2013). Neural circuits mediating olfactory-driven behavior in fish. Front. Neural. Circuits 7:62. doi: 10.3389/fncir.2013.00062
Kikuta, S., Fletcher, M. L., Homma, R., Yamasoba, T., and Nagayama, S. (2013). Odorant response properties of individual neurons in an olfactory glomerular module. Neuron 77, 1122–1135. doi: 10.1016/j.neuron.2013.01.022
Kikuta, S., Sato, K., Kashiwadani, H., Tsunoda, K., Yamasoba, T., and Mori, K. (2010). Neurons in the anterior olfactory nucleus pars externa detect right or left localization of odor sources. Proc. Natl. Acad. Sci. U.S.A. 107, 12363–12368. doi: 10.1073/pnas.1003999107
Kirillova, V., and Lin, J. W. (1998). A whole-cell clamp study of dendrodendritic synaptic activities in mitral cells of turtle olfactory bulb slices. Neuroscience 87, 255–264. doi: 10.1016/s0306-4522(98)00146-8
Kishi, K., Mori, K., and Ojima, H. (1984). Distribution of local axon collaterals of mitral, displaced mitral, and tufted cells in the rabbit olfactory bulb. J. Comp. Neurol. 225, 511–526. doi: 10.1002/cne.902250404
Klenoff, J. R., and Greer, C. A. (1998). Postnatal development of olfactory receptor cell axonal arbors. J. Comp. Neurol. 390, 256–267. doi: 10.1002/(sici)1096-9861(19980112)390:2<256::aid-cne8>3.0.co;2-0
Kobayakawa, K., Kobayakawa, R., Matsumoto, H., Oka, Y., Imai, T., Ikawa, M., et al. (2007). Innate versus learned odour processing in the mouse olfactory bulb. Nature 450, 503–508. doi: 10.1038/nature06281
Kolb, H., Linberg, K. A., and Fisher, S. K. (1992). Neurons of the human retina: a Golgi study. J. Comp. Neurol. 318, 147–187. doi: 10.1002/cne.903180204
Kollo, M., Schmaltz, A., Abdelhamid, M., Fukunaga, I., and Schaefer, A. T. (2014). ‘Silent’ mitral cells dominate odor responses in the olfactory bulb of awake mice. Nat. Neurosci. 17, 1313–1315. doi: 10.1038/nn.3768
Kondoh, D., Wada, A., Endo, D., Nakamuta, N., and Taniguchi, K. (2013). Histological and lectin histochemical studies on the main and accessory olfactory bulbs in the Japanese striped snake. Elaphe quadrivirgata. J. Vet. Med. Sci. 75, 567–574. doi: 10.1292/jvms.12-0484
Kondoh, K., Lu, Z., Ye, X., Olson, D. P., Lowell, B. B., and Buck, L. B. (2016). A specific area of olfactory cortex involved in stress hormone responses to predator odours. Nature 532, 103–106. doi: 10.1038/nature17156
Kosaka, T. (1980). Ruffed cell: a new type of neuron with a distinctive initial unmyelinated portion of the axon in the olfactory bulb of the goldfish (Carassius auratus): II. Fine structure of the ruffed cell. J. Comp. Neurol. 193, 119–145. doi: 10.1002/cne.901930109
Kosaka, T., and Hama, K. (1979). Ruffed cell: a new type of neuron with a distinctive initial unmyelinated portion of the axon in the olfactory bulb of the goldfish (Carassius auratus) I. Golgi impregnation and serial thin sectioning studies. J. Comp. Neurol. 186, 301–319. doi: 10.1002/cne.901860302
Kosaka, T., and Hama, K. (1982). Structure of the mitral cell in the olfactory bulb of the goldfish (Carassius auratus). J. Comp. Neurol. 212, 365–384. doi: 10.1002/cne.902120405
Kratskin, I. L., Rio, J. P., Kenigfest, N. B., Doty, R. L., and Reperant, J. (2000). A light and electron microscopic study of taurine-like immunoreactivity in the main olfactory bulb of frogs. J. Chem. Neuroanat. 18, 87–101. doi: 10.1016/s0891-0618(99)00055-1
Kwan, K. Y., Sestan, N., and Anton, E. S. (2012). Transcriptional co-regulation of neuronal migration and laminar identity in the neocortex. Development 139, 1535–1546. doi: 10.1242/dev.069963
Lanuza, E., and Halpern, M. (1998). Efferents and centrifugal afferents of the main and accessory olfactory bulbs in the snake Thamnophis sirtalis. Brain Behav. Evol. 51, 1–22. doi: 10.1159/000006525
Larriva-Sahd, J. (2008). The accessory olfactory bulb in the adult rat: a cytological study of its cell types, neuropil, neuronal modules, and interactions with the main olfactory system. J. Comp. Neurol. 510, 309–350. doi: 10.1002/cne.21790
Lemons, K., Fu, Z., Aoude, I., Ogura, T., Sun, J., Chang, J., et al. (2017). Lack of TRPM5-expressing microvillous cells in mouse main olfactory epithelium leads to impaired odor-evoked responses and olfactory-guided behavior in a challenging chemical environment. eNeuro 4:ENEURO.0135-17.2017. doi: 10.1523/ENEURO.0135-17.2017
Lepousez, G., Csaba, Z., Bernard, V., Loudes, C., Videau, C., Lacombe, J., et al. (2010). Somatostatin interneurons delineate the inner part of the external plexiform layer in the mouse main olfactory bulb. J. Comp. Neurol. 518, 1976–1994. doi: 10.1002/cne.22317
Lepousez, G., and Lledo, P. M. (2013). Odor discrimination requires proper olfactory fast oscillations in awake mice. Neuron 80, 1010–1024. doi: 10.1016/j.neuron.2013.07.025
Li, A., Gire, D. H., and Restrepo, D. (2015). Upsilon spike-field coherence in a population of olfactory bulb neurons differentiates between odors irrespective of associated outcome. J. Neurosci. 35, 5808–5822. doi: 10.1523/jneurosci.4003-14.2015
Lin, D. M., Wang, F., Lowe, G., Gold, G. H., Axel, R., Ngai, J., et al. (2000). Formation of precise connections in the olfactory bulb occurs in the absence of odorant-evoked neuronal activity. Neuron 26, 69–80.
Lin, W., Margolskee, R., Donnert, G., Hell, S. W., and Restrepo, D. (2007). Olfactory neurons expressing transient receptor potential channel M5 (TRPM5) are involved in sensing semiochemicals. Proc. Natl. Acad. Sci. U.S.A. 104, 2471–2476. doi: 10.1073/pnas.0610201104
Liu, A., Papale, A. E., Hengenius, J., Patel, K., Ermentrout, B., and Urban, N. N. (2020). Mouse navigation strategies for odor source localization. Front. Neurosci. 14:218. doi: 10.3389/fnins.2020.00218
Liu, P., Cao, T., Xu, J., Mao, X., Wang, D., and Li, A. (2020). Plasticity of sniffing pattern and neural activity in the olfactory bulb of behaving mice during odor sampling. Anticipation, and reward. Neurosci. Bull. 36, 598–610. doi: 10.1007/s12264-019-00463-9
Liu, G., Froudarakis, E., Patel, J. M., Kochukov, M. Y., Pekarek, B., Hunt, P. J., et al. (2019). Target specific functions of EPL interneurons in olfactory circuits. Nat. Commun. 10:3369.
Liu, S., and Shipley, M. T. (2008). Multiple conductances cooperatively regulate spontaneous bursting in mouse olfactory bulb external tufted cells. J. Neurosci. 28, 1625–1639. doi: 10.1523/jneurosci.3906-07.2008
Liu, W. L., and Shipley, M. T. (1994). Intrabulbar associational system in the rat olfactory bulb comprises cholecystokinin-containing tufted cells that synapse onto the dendrites of GABAergic granule cells. J. Comp. Neurol. 346, 541–558. doi: 10.1002/cne.903460407
Liu, X., and Liu, S. (2018). Cholecystokinin selectively activates short axon cells to enhance inhibition of olfactory bulb output neurons. J. Physiol. 596, 2185–2207. doi: 10.1113/jp275511
Llahi, S., Farinas, I., and Garcia Verdugo, J. M. (1985). Mitral cells of the olfactory bulb of the lizardPodarcis Hispanica. SCIENTIA Gerundensis 11, 17–28.
Lodovichi, C., Belluscio, L., and Katz, L. C. (2003). Functional topography of connections linking mirror-symmetric maps in the mouse olfactory bulb. Neuron 38, 265–276. doi: 10.1016/s0896-6273(03)00194-6
Lohman, A. H., and Smeets, W. J. (1993). Overview of the main and accessory olfactory bulb projections in reptiles. Brain Behav. Evol. 41, 147–155. doi: 10.1159/000113832
Losacco, J., Ramirez-Gordillo, D., Gilmer, J., and Restrepo, D. (2020). Learning improves decoding of odor identity with phase-referenced oscillations in the olfactory bulb. eLife 9, e52583. doi: 10.7554/eLife.52583
Lukas, M., Suyama, H., and Egger, V. (2019). Vasopressin cells in the rodent olfactory bulb resemble non-bursting superficial tufted cells and are primarily inhibited upon olfactory nerve stimulation. eNeuro 6:ENEURO.0431-18.2019.
MacNeil, M. A., and Masland, R. H. (1998). Extreme diversity among amacrine cells: implications for function. Neuron 20, 971–982. doi: 10.1016/s0896-6273(00)80478-x
Macrides, F., and Schneider, S. P. (1982). Laminar organization of mitral and tufted cells in the main olfactory bulb of the adult hamster. J. Comp. Neurol. 208, 419–430. doi: 10.1002/cne.902080410
Malnic, B., Godfrey, P. A., and Buck, L. B. (2004). The human olfactory receptor gene family. Proc. Natl. Acad. Sci. U.S.A. 101, 2584–2589.
Malnic, B., Hirono, J., Sato, T., and Buck, L. B. (1999). Combinatorial receptor codes for odors. Cell 96, 713–723. doi: 10.1016/s0092-8674(00)80581-4
Malun, D., and Brunjes, P. C. (1996). Development of olfactory glomeruli: temporal and spatial interactions between olfactory receptor axons and mitral cells in opossums and rats. J. Comp. Neurol. 368, 1–16. doi: 10.1002/(sici)1096-9861(19960422)368:1<1::aid-cne1>3.0.co;2-7
Manabe, H., and Mori, K. (2013). Sniff rhythm-paced fast and slow gamma-oscillations in the olfactory bulb: relation to tufted and mitral cells and behavioral states. J. Neurophysiol. 110, 1593–1599. doi: 10.1152/jn.00379.2013
Martin, C., Gervais, R., Hugues, E., Messaoudi, B., and Ravel, N. (2004). Learning modulation of odor-induced oscillatory responses in the rat olfactory bulb: a correlate of odor recognition? J. Neurosci. 24, 389–397. doi: 10.1523/jneurosci.3433-03.2004
Martin, C., and Ravel, N. (2014). Beta and gamma oscillatory activities associated with olfactory memory tasks: different rhythms for different functional networks? Front. Behav. Neurosci. 8:218. doi: 10.3389/fnbeh.2014.00218
Martinez-Garcia, F., Olucha, F. E., Teruel, V., Lorente, M. J., and Schwerdtfeger, W. K. (1991). Afferent and efferent connections of the olfactory bulbs in the lizard Podarcis hispanica. J. Comp. Neurol. 305, 337–347. doi: 10.1002/cne.903050214
Martínez-Marcos, A., and Halpern, M. (2009). “Evolution of olfactory and vomeronasal systems,” in Encyclopedia of Neuroscience, eds M. D. Binder, N. Hirokawa, and U. Windhorst (Berlin: Springer), 1264–1269. doi: 10.1007/978-3-540-29678-2_3135
Masland, R. H. (2012). The neuronal organization of the retina. Neuron 76, 266–280. doi: 10.1016/j.neuron.2012.10.002
Matsumoto, H., Kashiwadani, H., Nagao, H., Aiba, A., and Mori, K. (2009). Odor-induced persistent discharge of mitral cells in the mouse olfactory bulb. J. Neurophysiol. 101, 1890–1900. doi: 10.1152/jn.91019.2008
Matsuno, T., Kiyokage, E., and Toida, K. (2017). Synaptic distribution of individually labeled mitral cells in the external plexiform layer of the mouse olfactory bulb. J. Comp. Neurol. 525:2611. doi: 10.1002/cne.24224
Meissner-Bernard, C., Dembitskaya, Y., Venance, L., and Fleischmann, A. (2019). Encoding of odor fear memories in the mouse olfactory cortex. Curr. Biol. 29:e364.
Merkle, F. T., Mirzadeh, Z., and Alvarez-Buylla, A. (2007). Mosaic organization of neural stem cells in the adult brain. Science 317, 381–384. doi: 10.1126/science.1144914
Miyamichi, K., Amat, F., Moussavi, F., Wang, C., Wickersham, I., Wall, N. R., et al. (2010). Cortical representations of olfactory input by trans-synaptic tracing. Nature 472, 191–196. doi: 10.1038/nature09714
Miyamichi, K., Shlomai-Fuchs, Y., Shu, M., Weissbourd, B. C., Luo, L., and Mizrahi, A. (2013). Dissecting local circuits: parvalbumin interneurons underlie broad feedback control of olfactory bulb output. Neuron 80, 1232–1245. doi: 10.1016/j.neuron.2013.08.027
Miyasaka, N., Arganda-Carreras, I., Wakisaka, N., Masuda, M., Sumbul, U., Seung, H. S., et al. (2014). Olfactory projectome in the zebrafish forebrain revealed by genetic single-neuron labelling. Nat. Commun. 5:3639.
Miyasaka, N., Morimoto, K., Tsubokawa, T., Higashijima, S., Okamoto, H., and Yoshihara, Y. (2009). From the olfactory bulb to higher brain centers: genetic visualization of secondary olfactory pathways in zebrafish. J. Neurosci. 29, 4756–4767. doi: 10.1523/jneurosci.0118-09.2009
Mohrhardt, J., Nagel, M., Fleck, D., Ben-Shaul, Y., and Spehr, M. (2018). signal detection and coding in the accessory olfactory system. Chem. Senses 43, 667–695. doi: 10.1093/chemse/bjy061
Molyneaux, B. J., Arlotta, P., Menezes, J. R., and Macklis, J. D. (2007). Neuronal subtype specification in the cerebral cortex. Nat. Rev. Neurosci. 8, 427–437. doi: 10.1038/nrn2151
Mombaerts, P., Wang, F., Dulac, C., Chao, S. K., Nemes, A., Mendelsohn, M., et al. (1996). Visualizing an olfactory sensory map. Cell 87, 675–686. doi: 10.1016/s0092-8674(00)81387-2
Monahan, K., and Lomvardas, S. (2015). Monoallelic expression of olfactory receptors. Annu. Rev. Cell Dev. Biol. 31, 721–740. doi: 10.1146/annurev-cellbio-100814-125308
Mori, K., Kishi, K., and Ojima, H. (1983). Distribution of dendrites of mitral, displaced mitral, tufted, and granule cells in the rabbit olfactory bulb. J. Comp. Neurol. 219, 339–355. doi: 10.1002/cne.902190308
Mori, K., Nagao, H., and Yoshihara, Y. (1999). The olfactory bulb: coding and processing of odor molecule information. Science 286, 711–715. doi: 10.1126/science.286.5440.711
Mori, K., Nowycky, M. C., and Shepherd, G. M. (1981). Electrophysiological analysis of mitral cells in the isolated turtle olfactory bulb. J. Physiol. 314, 281–294. doi: 10.1113/jphysiol.1981.sp013707
Mori, K., and Sakano, H. (2011). How is the olfactory map formed and interpreted in the mammalian brain? Annu. Rev. Neurosci. 34, 467–499. doi: 10.1146/annurev-neuro-112210-112917
Mori, K., Takahashi, Y. K., Igarashi, K. M., and Yamaguchi, M. (2006). Maps of odorant molecular features in the Mammalian olfactory bulb. Physiol. Rev. 86, 409–433. doi: 10.1152/physrev.00021.2005
Mouradian, L. E., and Scott, J. W. (1988). Cytochrome oxidase staining marks dendritic zones of the rat olfactory bulb external plexiform layer. J. Comp. Neurol. 271, 507–518. doi: 10.1002/cne.902710404
Murata, K., Kanno, M., Ieki, N., Mori, K., and Yamaguchi, M. (2015). Mapping of learned odor-induced motivated behaviors in the mouse olfactory tubercle. J. Neurosci. 35, 10581–10599. doi: 10.1523/jneurosci.0073-15.2015
Nagao, H., Yoshihara, Y., Mitsui, S., Fujisawa, H., and Mori, K. (2000). Two mirror-image sensory maps with domain organization in the mouse main olfactory bulb. Neuroreport 11, 3023–3027. doi: 10.1097/00001756-200009110-00039
Nagayama, S., Enerva, A., Fletcher, M. L., Masurkar, A. V., Igarashi, K. M., Mori, K., et al. (2010). Differential axonal projection of mitral and tufted cells in the mouse main olfactory system. Front. Neural. Circuits 4:120. doi: 10.3389/fncir.2010.00120
Nagayama, S., Homma, R., and Imamura, F. (2014). Neuronal organization of olfactory bulb circuits. Front. Neural Circuits 8:98. doi: 10.3389/fncir.2014.00098
Nagayama, S., Takahashi, Y. K., Yoshihara, Y., and Mori, K. (2004). Mitral and tufted cells differ in the decoding manner of odor maps in the rat olfactory bulb. J. Neurophysiol. 91, 2532–2540. doi: 10.1152/jn.01266.2003
Narayanan, R. T., Udvary, D., and Oberlaender, M. (2017). Cell type-specific structural organization of the six layers in rat barrel cortex. Front. Neuroanat. 11:91. doi: 10.3389/fnana.2017.00091
Nassi, J. J., and Callaway, E. M. (2009). Parallel processing strategies of the primate visual system. Nat. Rev. Neurosci. 10, 360–372. doi: 10.1038/nrn2619
Nezlin, L. P., Heermann, S., Schild, D., and Rossler, W. (2003). Organization of glomeruli in the main olfactory bulb of Xenopus laevis tadpoles. J. Comp. Neurol. 464, 257–268. doi: 10.1002/cne.10709
Nezlin, L. P., and Schild, D. (2000). Structure of the olfactory bulb in tadpoles of Xenopus laevis. Cell Tissue Res. 302, 21–29. doi: 10.1007/s004410000208
Nguyen, U. P., and Imamura, F. (2019). Regional differences in mitral cell development in mouse olfactory bulb. J. Comp. Neurol. 527, 2233–2244.
Northcutt, R. G. (2002). Understanding vertebrate brain evolution. Integr. Comp. Biol. 42, 743–756. doi: 10.1093/icb/42.4.743
Ojima, H., Mori, K., and Kishi, K. (1984). The trajectory of mitral cell axons in the rabbit olfactory cortex revealed by intracellular HRP injection. J. Comp. Neurol. 230, 77–87. doi: 10.1002/cne.902300107
Oka, Y. (1983). Golgi, electron-microscopic and combined Golgi-electron-microscopic studies of the mitral cells in the goldfish olfactory bulb. Neuroscience 8, 723–742. doi: 10.1016/0306-4522(83)90006-4
Orona, E., Rainer, E. C., and Scott, J. W. (1984). Dendritic and axonal organization of mitral and tufted cells in the rat olfactory bulb. J. Comp. Neurol. 226, 346–356. doi: 10.1002/cne.902260305
Orona, E., Scott, J. W., and Rainer, E. C. (1983). Different granule cell populations innervate superficial and deep regions of the external plexiform layer in rat olfactory bulb. J. Comp. Neurol. 217, 227–237. doi: 10.1002/cne.902170209
Padmanabhan, K., and Urban, N. N. (2010). Intrinsic biophysical diversity decorrelates neuronal firing while increasing information content. Nat. Neurosci. 13, 1276–1282. doi: 10.1038/nn.2630
Panzanelli, P., Perazzini, A. Z., Fritschy, J. M., and Sassoe-Pognetto, M. (2005). Heterogeneity of gamma-aminobutyric acid type A receptors in mitral and tufted cells of the rat main olfactory bulb. J. Comp. Neurol. 484, 121–131. doi: 10.1002/cne.20440
Parrish-Aungst, S., Shipley, M. T., Erdelyi, F., Szabo, G., and Puche, A. C. (2007). Quantitative analysis of neuronal diversity in the mouse olfactory bulb. J. Comp. Neurol. 501, 825–836. doi: 10.1002/cne.21205
Pashkovski, S. L., Iurilli, G., Brann, D., Chicharro, D., Drummey, K., Franks, K., et al. (2020). Structure and flexibility in cortical representations of odour space. Nature 583, 253–258. doi: 10.1038/s41586-020-2451-1
Peng, Y. R., Shekhar, K., Yan, W., Herrmann, D., Sappington, A., Bryman, G. S., et al. (2019). Molecular classification and comparative taxonomics of foveal and peripheral cells in primate retina. Cell 176, 1222.e7–1237.e7.
Pinato, G., and Midtgaard, J. (2003). Regulation of granule cell excitability by a low-threshold calcium spike in turtle olfactory bulb. J. Neurophysiol. 90, 3341–3351. doi: 10.1152/jn.00560.2003
Pinching, A. J., and Powell, T. P. (1971a). The neuron types of the glomerular layer of the olfactory bulb. J. Cell Sci. 9, 305–345.
Pinching, A. J., and Powell, T. P. (1971b). The neuropil of the glomeruli of the olfactory bulb. J. Cell Sci. 9, 347–377.
Pressler, R. T., and Strowbridge, B. W. (2006). Blanes cells mediate persistent feedforward inhibition onto granule cells in the olfactory bulb. Neuron 49, 889–904. doi: 10.1016/j.neuron.2006.02.019
Price, J. L., and Powell, T. P. (1970a). The mitral and short axon cells of the olfactory bulb. J. Cell Sci. 7, 631–651.
Price, J. L., and Powell, T. P. (1970b). The synaptology of the granule cells of the olfactory bulb. J. Cell Sci. 7, 125–155.
Rall, W., Shepherd, G. M., Reese, T. S., and Brightman, M. W. (1966). Dendrodendritic synaptic pathway for inhibition in the olfactory bulb. Exp. Neurol. 14, 44–56. doi: 10.1016/0014-4886(66)90023-9
Rheaume, B. A., Jereen, A., Bolisetty, M., Sajid, M. S., Yang, Y., Renna, K., et al. (2018). Single cell transcriptome profiling of retinal ganglion cells identifies cellular subtypes. Nat. Commun. 9:2759.
Rodriguez, I., Feinstein, P., and Mombaerts, P. (1999). Variable patterns of axonal projections of sensory neurons in the mouse vomeronasal system. Cell 97, 199–208. doi: 10.1016/s0092-8674(00)80730-8
Rodriguez-Gil, D. J., Bartel, D. L., Jaspers, A. W., Mobley, A. S., Imamura, F., and Greer, C. A. (2015). Odorant receptors regulate the final glomerular coalescence of olfactory sensory neuron axons. Proc. Natl. Acad. Sci. U.S.A. 112, 5821–5826. doi: 10.1073/pnas.1417955112
Roland, B., Deneux, T., Franks, K. M., Bathellier, B., and Fleischmann, A. (2017). Odor identity coding by distributed ensembles of neurons in the mouse olfactory cortex. eLife 6:e26337. doi: 10.7554/eLife.26337
Root, C. M., Denny, C. A., Hen, R., and Axel, R. (2014). The participation of cortical amygdala in innate, odour-driven behaviour. Nature 515, 269–273. doi: 10.1038/nature13897
Sakano, H. (2020). Developmental regulation of olfactory circuit formation in mice. Dev. Growth Differ. 62, 199–213. doi: 10.1111/dgd.12657
Sanchez-Guardado, L., and Lois, C. (2019). Lineage does not regulate the sensory synaptic input of projection neurons in the mouse olfactory bulb. eLife 8:e46675.
Sanes, J. R., and Masland, R. H. (2015). The types of retinal ganglion cells: current status and implications for neuronal classification. Annu. Rev. Neurosci. 38, 221–246. doi: 10.1146/annurev-neuro-071714-034120
Saraiva, L. R., Kondoh, K., Ye, X., Yoon, K. H., Hernandez, M., and Buck, L. B. (2016). Combinatorial effects of odorants on mouse behavior. Proc. Natl. Acad. Sci. U.S.A. 113, E3300–E3306.
Sato, T., Homma, R., and Nagayama, S. (2020). Direct comparison of odor responses of homologous glomeruli in the medial and lateral maps of the mouse olfactory bulb. eNeuro 7:ENEURO.0449-19.2020. doi: 10.1523/ENEURO.0449-19.2020
Satou, M. (1990). Synaptic organization, local neuronal circuitry, and functional segregation of the teleost olfactory bulb. Prog. Neurobiol. 34, 115–142. doi: 10.1016/0301-0082(90)90004-z
Scalia, F., Gallousis, G., and Roca, S. (1991a). A note on the organization of the amphibian olfactory bulb. J. Comp. Neurol. 305, 435–442. doi: 10.1002/cne.903050307
Scalia, F., Gallousis, G., and Roca, S. (1991b). Differential projections of the main and accessory olfactory bulb in the frog. J. Comp. Neurol. 305, 443–461. doi: 10.1002/cne.903050308
Scalia, F., and Winans, S. S. (1975). The differential projections of the olfactory bulb and accessory olfactory bulb in mammals. J. Comp. Neurol. 161, 31–55. doi: 10.1002/cne.901610105
Schaefer, A. T., and Margrie, T. W. (2007). Spatiotemporal representations in the olfactory system. Trends Neurosci. 30, 92–100. doi: 10.1016/j.tins.2007.01.001
Schaffer, E. S., Stettler, D. D., Kato, D., Choi, G. B., Axel, R., and Abbott, L. F. (2018). Odor perception on the two sides of the brain: consistency despite randomness. Neuron 98, 736.e3–742.e3.
Schiller, P. H. (2010). Parallel information processing channels created in the retina. Proc. Natl. Acad. Sci. U.S.A. 107, 17087–17094. doi: 10.1073/pnas.1011782107
Schneider, S. P., and Macrides, F. (1978). Laminar distributions of internuerons in the main olfactory bulb of the adult hamster. Brain Res. Bull. 3, 73–82. doi: 10.1016/0361-9230(78)90063-1
Schneider, S. P., and Scott, J. W. (1983). Orthodromic response properties of rat olfactory bulb mitral and tufted cells correlate with their projection patterns. J. Neurophysiol. 50, 358–378. doi: 10.1152/jn.1983.50.2.358
Schoenfeld, T. A., Marchand, J. E., and Macrides, F. (1985). Topographic organization of tufted cell axonal projections in the hamster main olfactory bulb: an intrabulbar associational system. J. Comp. Neurol. 235, 503–518. doi: 10.1002/cne.902350408
Scott, J. W., Mcbride, R. L., and Schneider, S. P. (1980). The organization of projections from the olfactory bulb to the piriform cortex and olfactory tubercle in the rat. J. Comp. Neurol. 194, 519–534. doi: 10.1002/cne.901940304
Scott, J. W., Ranier, E. C., Pemberton, J. L., Orona, E., and Mouradian, L. E. (1985). Pattern of rat olfactory bulb mitral and tufted cell connections to the anterior olfactory nucleus pars externa. J. Comp. Neurol. 242, 415–424. doi: 10.1002/cne.902420309
Seabrook, T. A., Burbridge, T. J., Crair, M. C., and Huberman, A. D. (2017). Architecture, function, and assembly of the mouse visual system. Annu. Rev. Neurosci. 40, 499–538. doi: 10.1146/annurev-neuro-071714-033842
Serizawa, S., Miyamichi, K., Nakatani, H., Suzuki, M., Saito, M., Yoshihara, Y., et al. (2003). Negative feedback regulation ensures the one receptor-one olfactory neuron rule in mouse. Science 302, 2088–2094. doi: 10.1126/science.1089122
Seroogy, K. B., Brecha, N., and Gall, C. (1985). Distribution of cholecystokinin-like immunoreactivity in the rat main olfactory bulb. J. Comp. Neurol. 239, 373–383. doi: 10.1002/cne.902390403
Shao, Z., Puche, A. C., Liu, S., and Shipley, M. T. (2012). Intraglomerular inhibition shapes the strength and temporal structure of glomerular output. J. Neurophysiol. 108, 782–793. doi: 10.1152/jn.00119.2012
Shao, Z., Puche, A. C., and Shipley, M. T. (2013). Intraglomerular inhibition maintains mitral cell response contrast across input frequencies. J. Neurophysiol. 110, 2185–2191. doi: 10.1152/jn.00023.2013
Shepherd, G. M., Chen, W. R., and Greer, C. A. (2004). “Olfactory bulb,” in The Synaptic Organization of the Brain, 5th Edn, ed. G. M. Shepherd (New York, NY: Oxford UP), 165–216.
Shmuel, R., Secundo, L., and Haddad, R. (2019). Strong, weak and neuron type dependent lateral inhibition in the olfactory bulb. Sci. Rep. 9:1602.
Short, S. M., and Wachowiak, M. (2019). Temporal dynamics of inhalation-linked activity across defined subpopulations of mouse olfactory bulb neurons imaged in vivo. eNeuro 6:ENEURO.0189-19.2019. doi: 10.1523/ENEURO.0189-19.2019
Silva, L., and Antunes, A. (2017). Vomeronasal receptors in vertebrates and the evolution of pheromone detection. Annu. Rev. Anim. Biosci. 5, 353–370. doi: 10.1146/annurev-animal-022516-022801
Sosulski, D. L., Lissitsyna Bloom, M., Cutforth, T., Axel, R., and Datta, S. R. (2011). Distinct representations of olfactory information in different cortical centres. Nature 472, 213–216. doi: 10.1038/nature09868
Spors, H., and Grinvald, A. (2002). Spatio-temporal dynamics of odor representations in the mammalian olfactory bulb. Neuron 34, 301–315. doi: 10.1016/s0896-6273(02)00644-x
Stettler, D. D., and Axel, R. (2009). Representations of odor in the piriform cortex. Neuron 63, 854–864. doi: 10.1016/j.neuron.2009.09.005
Takami, S., and Graziadei, P. P. (1991). Light microscopic Golgi study of mitral/tufted cells in the accessory olfactory bulb of the adult rat. J. Comp. Neurol. 311, 65–83. doi: 10.1002/cne.903110106
Tan, J., Savigner, A., Ma, M., and Luo, M. (2010). Odor information processing by the olfactory bulb analyzed in gene-targeted mice. Neuron 65, 912–926. doi: 10.1016/j.neuron.2010.02.011
Thomas-Danguin, T., Sinding, C., Romagny, S., El Mountassir, F., Atanasova, B., Le Berre, E., et al. (2014). The perception of odor objects in everyday life: a review on the processing of odor mixtures. Front. Psychol. 5:504. doi: 10.3389/fpsyg.2014.00504
Thompson, J. A., Salcedo, E., Restrepo, D., and Finger, T. E. (2012). Second-order input to the medial amygdala from olfactory sensory neurons expressing the transduction channel TRPM5. J. Comp. Neurol. 520, 1819–1830. doi: 10.1002/cne.23015
Tirindelli, R., Dibattista, M., Pifferi, S., and Menini, A. (2009). From pheromones to behavior. Physiol. Rev. 89, 921–956.
Tobin, V. A., Hashimoto, H., Wacker, D. W., Takayanagi, Y., Langnaese, K., Caquineau, C., et al. (2010). An intrinsic vasopressin system in the olfactory bulb is involved in social recognition. Nature 464, 413–417. doi: 10.1038/nature08826
Toida, K., Kosaka, K., Heizmann, C. W., and Kosaka, T. (1994). Synaptic contacts between mitral/tufted cells and GABAergic neurons containing calcium-binding protein parvalbumin in the rat olfactory bulb, with special reference to reciprocal synapses between them. Brain Res. 650, 347–352. doi: 10.1016/0006-8993(94)91804-x
Turner, D. L., and Cepko, C. L. (1987). A common progenitor for neurons and glia persists in rat retina late in development. Nature 328, 131–136. doi: 10.1038/328131a0
Ubeda-Banon, I., Pro-Sistiaga, P., Mohedano-Moriano, A., Saiz-Sanchez, D., De La Rosa-Prieto, C., Gutierrez-Castellanos, N., et al. (2011). Cladistic analysis of olfactory and vomeronasal systems. Front. Neuroanat. 5:3. doi: 10.3389/fnana.2011.00003
Uchida, N., Poo, C., and Haddad, R. (2014). Coding and transformations in the olfactory system. Annu. Rev. Neurosci. 37, 363–385. doi: 10.1146/annurev-neuro-071013-013941
Vaaga, C. E., and Westbrook, G. L. (2016). Parallel processing of afferent olfactory sensory information. J. Physiol. 594, 6715–6732. doi: 10.1113/jp272755
Vaaga, C. E., and Westbrook, G. L. (2017). Distinct temporal filters in mitral cells and external tufted cells of the olfactory bulb. J. Physiol. 595, 6349–6362. doi: 10.1113/jp274608
Voinescu, P. E., Kay, J. N., and Sanes, J. R. (2009). Birthdays of retinal amacrine cell subtypes are systematically related to their molecular identity and soma position. J. Comp. Neurol. 517, 737–750. doi: 10.1002/cne.22200
von Campenhausen, H., and Mori, K. (2000). Convergence of segregated pheromonal pathways from the accessory olfactory bulb to the cortex in the mouse. Eur. J. Neurosci. 12, 33–46. doi: 10.1046/j.1460-9568.2000.00879.x
Wachowiak, M., and Shipley, M. T. (2006). Coding and synaptic processing of sensory information in the glomerular layer of the olfactory bulb. Semin. Cell Dev Biol. 17, 411–423. doi: 10.1016/j.semcdb.2006.04.007
Wacker, D. W., Engelmann, M., Tobin, V. A., Meddle, S. L., and Ludwig, M. (2011). Vasopressin and social odor processing in the olfactory bulb and anterior olfactory nucleus. Ann. N. Y. Acad. Sci. 1220, 106–116. doi: 10.1111/j.1749-6632.2010.05885.x
Wagner, S., Gresser, A. L., Torello, A. T., and Dulac, C. (2006). A multireceptor genetic approach uncovers an ordered integration of VNO sensory inputs in the accessory olfactory bulb. Neuron 50, 697–709. doi: 10.1016/j.neuron.2006.04.033
Walz, A., Omura, M., and Mombaerts, P. (2006). Development and topography of the lateral olfactory tract in the mouse: imaging by genetically encoded and injected fluorescent markers. J. Neurobiol. 66, 835–846. doi: 10.1002/neu.20266
Wandell, B. A. (1995). Foundations of Vision, Vol. 21. Sunderland, MA: Sinauer Associates, Inc, 142–144.
Weiss, L., Jungblut, L. D., Pozzi, A. G., Zielinski, B. S., O’connell, L. A., Hassenklover, T., et al. (2020). Multi-glomerular projection of single olfactory receptor neurons is conserved among amphibians. J. Comp. Neurol. 528, 2239–2253. doi: 10.1002/cne.24887
Wesson, D. W., and Wilson, D. A. (2011). Sniffing out the contributions of the olfactory tubercle to the sense of smell: hedonics, sensory integration, and more? Neurosci. Biobehav. Rev. 35, 655–668. doi: 10.1016/j.neubiorev.2010.08.004
White, E. L. (1972). Synaptic organization in the olfactory glomerulus of the mouse. Brain Res. 37, 69–80. doi: 10.1016/0006-8993(72)90346-0
Whitesell, J. D., Sorensen, K. A., Jarvie, B. C., Hentges, S. T., and Schoppa, N. E. (2013). Interglomerular lateral inhibition targeted on external tufted cells in the olfactory bulb. J. Neurosci. 33, 1552–1563. doi: 10.1523/jneurosci.3410-12.2013
Williams, E. O., Xiao, Y., Sickles, H. M., Shafer, P., Yona, G., Yang, J. Y., et al. (2007). Novel subdomains of the mouse olfactory bulb defined by molecular heterogeneity in the nascent external plexiform and glomerular layers. BMC Dev. Biol. 7:48. doi: 10.1186/1471-213X-7-48
Wilson, C. D., Serrano, G. O., Koulakov, A. A., and Rinberg, D. (2017). A primacy code for odor identity. Nat. Commun. 8:1477.
Wilson, D. A. (2000). Odor specificity of habituation in the rat anterior piriform cortex. J. Neurophysiol. 83, 139–145. doi: 10.1152/jn.2000.83.1.139
Wilson, D. A., and Sullivan, R. M. (2011). Cortical processing of odor objects. Neuron 72, 506–519. doi: 10.1016/j.neuron.2011.10.027
Winpenny, E., Lebel-Potter, M., Fernandez, M. E., Brill, M. S., Götz, M., Guillemot, F., et al. (2011). Sequential generation of olfactory bulb glutamatergic neurons by Neurog2-expressing precursor cells. Neural. Dev. 6:12. doi: 10.1186/1749-8104-6-12
Yamaguchi, M. (2017). Functional sub-circuits of the olfactory system viewed from the olfactory bulb and the olfactory tubercle. Front. Neuroanat. 11:33. doi: 10.3389/fnana.2017.00033
Yamatani, H., Sato, Y., Fujisawa, H., and Hirata, T. (2004). Chronotopic organization of olfactory bulb axons in the lateral olfactory tract. J. Comp. Neurol. 475, 247–260. doi: 10.1002/cne.20155
Yan, Z., Tan, J., Qin, C., Lu, Y., Ding, C., and Luo, M. (2008). Precise circuitry links bilaterally symmetric olfactory maps. Neuron 58, 613–624. doi: 10.1016/j.neuron.2008.03.012
Yan, W., Laboulaye, M. A., Tran, N. M., Whitney, I. E., Benhar, I., and Sanes, J. R. (2020). Mouse retinal cell atlas: molecular identification of over sixty amacrine cell types. J. Neurosci. 40:5177–5195. doi: 10.1523/jneurosci.0471-20.2020
Yokoi, M., Mori, K., and Nakanishi, S. (1995). Refinement of odor molecule tuning by dendrodendritic synaptic inhibition in the olfactory bulb. Proc. Natl. Acad. Sci. U.S.A. 92, 3371–3375. doi: 10.1073/pnas.92.8.3371
Yokosuka, M. (2012). Histological properties of the glomerular layer in the mouse accessory olfactory bulb. Exp. Anim. 61, 13–24. doi: 10.1538/expanim.61.13
Yonekura, J., and Yokoi, M. (2007). Conditional genetic labeling of mitral cells of the mouse accessory olfactory bulb to visualize the organization of their apical dendritic tufts. Mol. Cell Neurosci. 37, 708–718. doi: 10.1016/j.mcn.2007.12.016
Yoshihara, Y., Mizuno, T., Nakahira, M., Kawasaki, M., Watanabe, Y., Kagamiyama, H., et al. (1999). A genetic approach to visualization of multisynaptic neural pathways using plant lectin transgene. Neuron 22, 33–41. doi: 10.1016/s0896-6273(00)80676-5
Zhang, X., and Firestein, S. (2002). The olfactory receptor gene superfamily of the mouse. Nat. Neurosci. 5, 124–133. doi: 10.1038/nn800
Zhang, Z., Liu, Q., Wen, P., Zhang, J., Rao, X., Zhou, Z., et al. (2017). Activation of the dopaminergic pathway from VTA to the medial olfactory tubercle generates odor-preference and reward. eLife 6:e25423. doi: 10.7554/eLife.25423
Zhou, Z., and Belluscio, L. (2008). Intrabulbar projecting external tufted cells mediate a timing-based mechanism that dynamically gates olfactory bulb output. J. Neurosci. 28, 9920–9928. doi: 10.1523/jneurosci.3082-08.2008
Zhou, Z., and Belluscio, L. (2012). Coding odorant concentration through activation timing between the medial and lateral olfactory bulb. Cell Rep. 2, 1143–1150. doi: 10.1016/j.celrep.2012.09.035
Zippel, H. P. (1999). “Two physiologically different types of relay neurons in the olfactory bulb of goldfish,” in Advances in Chemical Signals in Vertebrates, eds R. E. Johnston, D. Müller-Schwarze, and P. W. Sorensen (Boston, MA: Springer), 593–597. doi: 10.1007/978-1-4615-4733-4_53
Zippel, H. P., Gloger, M., Nasser, S., and Wilcke, S. (2000). Odour discrimination in the olfactory bulb of goldfish: contrasting interactions between mitral cells and ruffed cells. Philos. Trans. R. Soc. Lond. B Biol. Sci. 355, 1229–1232. doi: 10.1098/rstb.2000.0673
Keywords: olfactory bulb, projection neurons, heterogeneity, parallel pathways, mitral cell, tufted cell
Citation: Imamura F, Ito A and LaFever BJ (2020) Subpopulations of Projection Neurons in the Olfactory Bulb. Front. Neural Circuits 14:561822. doi: 10.3389/fncir.2020.561822
Received: 13 May 2020; Accepted: 12 August 2020;
Published: 28 August 2020.
Edited by:
Debra Ann Fadool, Florida State University, United StatesReviewed by:
Julian P. Meeks, The University of Texas Southwestern Medical Center, United StatesVeronica Egger, University of Regensburg, Germany
Copyright © 2020 Imamura, Ito and LaFever. This is an open-access article distributed under the terms of the Creative Commons Attribution License (CC BY). The use, distribution or reproduction in other forums is permitted, provided the original author(s) and the copyright owner(s) are credited and that the original publication in this journal is cited, in accordance with accepted academic practice. No use, distribution or reproduction is permitted which does not comply with these terms.
*Correspondence: Fumiaki Imamura, ZnVpMUBwc3UuZWR1