- 1Brain and Cognitive Science, Scranton College, Ewha Womans University, Seoul, South Korea
- 2Department of Medical Science, College of Medicine, Catholic Kwandong University, Incheon, South Korea
- 3Translational Brain Research Center, Catholic Kwandong University, International St. Mary’s Hospital, Incheon, South Korea
Astrocytes, once thought to be passive cells merely filling the space between neurons in the nervous system, are receiving attention as active modulators of the brain and spinal cord physiology by providing nutrients, maintaining homeostasis, and modulating synaptic transmission. Accumulating evidence indicates that astrocytes are critically involved in chronic pain regulation. Injury induces astrocytes to become reactive, and recent studies suggest that reactive astrocytes can have either neuroprotective or neurodegenerative effects. While the exact mechanisms underlying the transition from resting astrocytes to reactive astrocytes remain unknown, astrocytic calcium increase, coordinated by inflammatory molecules, has been suggested to trigger this transition. In this mini review article, we will discuss the roles of astrocytic calcium, channels contributing to calcium dynamics in astrocytes, astrocyte activations along the pain pathway, and possible relationships between astrocytic calcium dynamics and chronic pain.
Introduction
Astrocytes play critical roles in the central nervous system (CNS). Under physiological conditions, astrocytes occupy non-overlapping regions and function in discrete areas (Nedergaard et al., 2003). Nonetheless, astrocytes are joined to adjacent astrocytes by gap junctions (Dermietzel et al., 1991), which make them apt for coordinating activity. Although several types of voltage-gated ion channels have been reported in astrocytes, there are not enough voltage-gated Na+ channels expressed to generate action potentials; thus, astrocytes are not considered electrically excitable (Pappalardo et al., 2016). However, astrocytes are reported to respond to external stimuli with internal Ca2+ elevation (Wang et al., 2006; Agulhon et al., 2008; Rusakov, 2015; Bazargani and Attwell, 2016; Guerra-Gomes et al., 2017), and this suggests that Ca2+ dynamics are an important signaling mechanism in astrocytes. Furthermore, a stimulated astrocyte with increased intracellular Ca2+ could subsequently trigger intracellular Ca2+ rise in non-stimulated astrocytes and propagate Ca2+ signals, a phenomenon called an astrocytic Ca2+ wave (Cornell-Bell et al., 1990; Dani et al., 1992; Charles, 1998; Scemes and Giaume, 2006). Astrocytic Ca2+ waves enable astrocytes to communicate over long distances. It is now generally accepted that astrocytic Ca2+ waves usually do not occur in physiological conditions (Agulhon et al., 2008). Accordingly, astrocytic Ca2+ waves are commonly observed after injury or in pathological conditions. Whether Ca2+ signal propagation between astrocytes in these conditions provides protective or detrimental effect is not clear-cut. It is, however, clear that the Ca2+ dynamics of astrocytes change in several pathological conditions, including neuropathic pain (Shigetomi et al., 2019). Therefore, understanding changes in astrocytic Ca2+ signaling related to pathological pain may provide insight into developing better methods for pain control. In this review, we delved to provide an overview of the relationship between astrocytic Ca2+ and chronic pain.
Roles of Astrocytic Calcium
Astrocytic Ca2+ has been implicated in various supportive and active roles within the CNS, from regulating synaptic transmission to modifying behavior.
At the synaptic level, astrocytes provide many supportive roles that modulate synaptic transmission between neurons. One way astrocytes regulate neuronal excitability is by buffering extracellular ions and maintaining optimal concentrations. Clearing increased K+ from the extracellular space is vital for limiting the hyper-excitability of neurons, and dysfunction in astrocytic K+ buffering capability underlies several neurological diseases such as epilepsy and Huntington’s disease (Bellot-Saez et al., 2017). K+ uptake from the extracellular space by astrocyte results in astrocytic Ca2+ dependently (Quandt and MacVicar, 1986; Wang et al., 2012), showing the importance of astrocytic Ca2+ in regulating K+ homeostasis.
Astrocytes also play a key role in buffering neurotransmitters, which is in part regulated by astrocytic Ca2+. A single astrocyte can cover both excitatory and inhibitory neurons and buffer both neurotransmitters: excitatory glutamate (Glu) and inhibitory γ-aminobutyric acid (GABA). The majority of Glu in the extracellular space is absorbed (80–90%) by excitatory amino-acid transporter (EAAT)-1 and 2, which are predominantly expressed in astrocytes (Mahmoud et al., 2019). Whether astrocytic Ca2+ regulates the activity of EAAT is unknown, but EAAT activity raises intracellular Ca2+ in astrocytes (Mahmoud et al., 2019), which would lead to subsequent Ca2+ signaling cascades. GABA transporter (GAT) activity, on the other hand, is directly regulated by astrocytic Ca2+. Transient receptor potential (TRP) channel subfamily A (TRPA), a cation channel shown to be important in maintaining basal astrocytic Ca2+ concentration in astrocytes, controls the expression of GAT in a Ca2+-dependent manner (Shigetomi et al., 2011). In addition to basal Ca2+ concentration, changes in astrocytic Ca2+, such as Ca2+ influx into astrocytes or Ca2+ reduction in astrocytes, influence the activity and functional expression of GAT in astrocytes (Zhang et al., 2017; Yu et al., 2018). Both types of transporters, EAAT and GAT, are populated around astrocytic processes that wrap around synapses (Minelli et al., 1996; Voutsinos-Porche et al., 2003), making them ideal for buffering and maintaining an appropriate level of neurotransmitters. Accordingly, astrocytic Ca2+ has major supportive roles in maintaining an optimal level of excitatory and inhibitory balance of neurons.
Perisynaptic astrocytic process (PAP), a structure of fine astrocytic processes wrapped around neuronal synapses, affect astrocytic buffering ability and provide spine stability in an astrocytic Ca2+-dependent manner (Bernardinelli et al., 2014). PAPs are extremely fine structures on the scale of nanometers, and the extent of area covered by PAPs affects astrocytic neurotransmitter uptake, with more coverage leading to more neurotransmitter uptake (Pannasch et al., 2014). Uncaging astrocytic Ca2+ induced actin-dependent outgrowth of PAPs, whereas reduced Ca2+ signaling in astrocytes reduced synaptic coverage by astrocytes (Bernardinelli et al., 2014). Furthermore, in vivo whisker stimulation, which increases neuronal activity in the somatosensory cortex and elevates astrocytic Ca2+, also enhances the movement of PAPs, demonstrating that astrocytic Ca2+ has physiological roles in vivo (Bernardinelli et al., 2014).
Another way astrocytes support CNS function is by providing nutrients and oxygen for metabolism. Lactate, the main nutrient secreted by astrocytes, enables hippocampal long-term potentiation (LTP) between synapses (Suzuki et al., 2011), which is hypothesized to be the cellular basis for learning and memory. In accordance, specifically inhibiting lactate secretion from astrocytes in the hippocampus disrupted hippocampal-dependent learning and memory in mice (Suzuki et al., 2011). Whether Ca2+ affects astrocytic lactate secretion is unknown, but the control of vascular smooth muscle tone, which is another important way of providing nutrients and oxygen, is directly regulated by astrocytic Ca2+ (Winship et al., 2007; Otsu et al., 2015). Sensory stimulations were shown to trigger rapid astrocytic Ca2+ responses to regulate neurovascular coupling (Stobart et al., 2018a).
Astrocytes are also proposed to actively modulate synaptic signal transmission by releasing gliotransmitters, as part of the tripartite synapse (Halassa et al., 2007; Santello et al., 2012). Major gliotransmitters released by astrocytes are ATP, D-serine, GABA, and Glu. Whether Ca2+-dependent gliotransmitter release occurs in physiological conditions remains unresolved with debates for and against it (Fiacco and McCarthy, 2018; Savtchouk and Volterra, 2018). Opponents argue that evidence for Ca2+-dependent gliotransmitter release comes from cultured astrocytes or acute brain slice where tissue damage is inevitable (Agulhon et al., 2012; Fiacco and McCarthy, 2018). Inflammatory mediators such as tumor necrosis factor-α (TNF-α) and activated microglia resulting from tissue damage will transform resting astrocytes into reactive astrocytes, and reactive astrocytes are well-known to show astrocytic Ca2+-dependent gliotransmitter release. However, these cases do not negate Ca2+-independent gliotransmitter release, mediated by channels or transporters (Savtchouk and Volterra, 2018), and although inconclusive, Ca2+-dependent gliotransmitter release may also be present under physiological conditions.
Astrocytes, under physiological conditions, may or may not release gliotransmitters Ca2+ dependently, but many studies suggest that astrocytic Ca2+ clearly has physiological functions. Natural stimulations such as visual or whisker stimulation elicit astrocytic Ca2+ responses in vivo and modulation of astrocytic Ca2+ changed behavior (Wang et al., 2006; Stobart et al., 2018b). Astrocytic Ca2+ activity was found to be an important regulator of cortical oscillation switches in vivo (Poskanzer and Yuste, 2016). Various behaviors such as learning and memory, sleep patterns, and repetitive behaviors were altered by modulations of astrocytic Ca2+ (Srinivasan et al., 2015; Adamsky et al., 2018; Stobart et al., 2018b; Yu et al., 2018). Behaviors resembling neuropathic pain were also induced by selective optogenetic activation of astrocytes, which increased astrocytic Ca2+ in the spinal cord (Nam et al., 2016). Spontaneous astrocytic Ca2+ oscillation is also suggested to be important in generating brain rhythms (Buskila et al., 2019), and a human study suggested that alterations in Ca2+ could cause chronic pain (Alshelh et al., 2016, 2019).
As described above, astrocytics Ca2+ signals are crucial for maintaining CNS function. Astrocytic Ca2+ signals may not be as fast as neuronal signals (second vs. millisecond range, respectively), but they could be more appropriate for providing sustained signaling in cases such as protecting tissues after an injury during recovery. While some alterations in astrocytic Ca2+ signals have been associated with dysfunctions in the CNS such as chronic pain, what kind of astrocytic Ca2+ changes lead to pathological conditions is still unclear. Since astrocytic Ca2+ signals triggered by different sources seem to have different functions, different sources of astrocytic Ca2+ are discussed in the next section.
Source of Calcium in Astrocytes
Several Ca2+ sources contribute to astrocytic Ca2+ dynamics. Ca2+ releases from intracellular stores and influx from the extracellular space both contribute to astrocytic Ca2+ changes, albeit with different patterns. Intracellular sources of astrocytic Ca2+ include inositol triphosphate receptor (IP3R), ryanodine receptor (RyR), and mitochondrial permeability transition pore (mPTP). Ca2+ Channels that contribute to Ca2+ influx into astrocytes from the extracellular space include Orai channels, ligand-gated ion channels (AMPA, NMDA, P2X, nicotinic acetylcholine, and 5-HT3 receptors), TRP channels, sodium/calcium exchangers (NCX), and voltage-gated Ca2+ channels.
The major source of astrocytic Ca2+ increase occurs by activation of IP3R expressed on the endoplasmic reticulum (ER). Activation of IP3R leads to a robust Ca2+ increase in the cell body. Ca2+ dynamics become nearly absent in the cell body of astrocytes with inhibition of IP3R or knockout of IP3R2, a major type of IP3R in astrocytes, but some Ca2+ activity in astrocytic processes remains (Srinivasan et al., 2015). The remaining Ca2+ activity in astrocytic processes could be from mPTP (Agarwal et al., 2017), RyR (Matyash et al., 2002), or influx from extracellular space via various channels and exchangers (Rungta et al., 2016). Astrocytic IP3R may be targeted to regulate chronic pain since direct and indirect reductions of IP3R activity were correlated with reductions in pathological pain symptoms associated with neuropathic pain in mice (Kim et al., 2016; Ishikawa et al., 2018).
RyR, another type of calcium channel expressed on the ER, is another intracellular source of astrocytic Ca2+. Although not a major contributor to astrocytic Ca2+ in hippocampal slices (Beck et al., 2004), it is reported to have supportive roles in generating Ca2+ oscillation in ventrobasal thalamic slices (Parri and Crunelli, 2003). RyR may also contribute to recovery after an injury, since RyR3 was shown to control astrocyte motility, an important component of healing and regeneration after brain injury (Matyash et al., 2002).
mPTP, a non-selective Ca2+-permeable channel expressed on mitochondria, is another possible contributor of intracellular Ca2+ increase in astrocytes. Unlike the IP3R, which triggers strong Ca2+ increases throughout the cell body, mPTP was shown to be responsible for local Ca2+ changes in astrocytic processes (Agarwal et al., 2017). Mitochondria located in astrocytic processes will provide energy and Ca2+ signals in microdomains under physiological conditions. However, in the presence of reactive oxygen species (ROS) generated by mitochondria and the increase in Ca2+ released from the ER, the mitochondrial membrane depolarizes via the opening of the mPTP, which ultimately leads to the necrotic death of astrocytes (Jacobson and Duchen, 2002). Both ROS and ER Ca2+ were required for this event to occur, and the transient opening of mPTP itself was innocuous, leading to no changes in apoptosis nor necrosis. These studies suggest that mPTP contributes to cellular signaling in physiological conditions and contributes to necrosis in the presence of ROS and ER Ca2+ release.
The major route of Ca2+ influx into astrocyte from extracellular space is by Orai channels. Orai channels are store-operated channels regulated by intracellular Ca2+ stores such as the ER (Smyth et al., 2006; Hewavitharana et al., 2007). When Ca2+ in the ER is depleted, stromal interaction molecules (STIMs) on the ER, which sense Ca2+, cluster to activate Orai channels (Zhang et al., 2005; Wang et al., 2009). Activated Orai channels not only replenish internal Ca2+ stores but also induce Ca2+-dependent gliotransmitter release and regulate inhibition by increasing GABAergic interneuron activity (Toth et al., 2019). Of the Orai channels expressed in astrocytes (Orai 1/2/3), activation of Orai1 was shown to promote the production of inflammatory cytokines in spinal astrocytes (Gao et al., 2016). Orai1 is also upregulated with neuroinflammation induced by lipopolysaccharide (LPS; Wei et al., 2019), suggesting that Orai1 could contribute to increased astrocytic Ca2+ following inflammation.
Several types of ligand-gated non-selective cation channels are also sources of Ca2+ elevation in astrocytes. ATP-gated purinergic receptor P2X and inotropic glutamate receptors such as AMPA and NMDA receptors are found in astrocytes. P2X7 could contribute to Ca2+ influx into astrocytes because a marked increase in astrocytic Ca2+ is observed with a P2X7 agonist application, but no changes were observed with a P2X1/P2X3 agonist application (Fumagalli et al., 2003). Most AMPA receptors are Ca2+ impermeable, but astrocytes in some brain regions, such as those expressed in olfactory astrocytes or Bergmann glia in the cerebellum, express GluA2 lacking AMPA receptors, which are Ca2+ permeable (Iino et al., 2001; Matthias et al., 2003). NMDA receptors are also expressed in some brain regions such as the neocortex, but not in the hippocampus, and they contribute to the regional heterogeneity of Ca2+ influx (Dzamba et al., 2013). These regional differences in expression patterns will likely contribute to different roles of astrocytes played in each region.
TRP channels are also an important source of astrocytic Ca2+ influx. TRPA1 has high Ca2+ conductance and has been shown to be an important regulator of resting Ca2+ concentration in astrocytes. Blocking TRPA1 not only reduced astrocytic Ca2+ concentration, but it also reduced the efficiency of inhibitory synapses by reducing GAT-3 activity in astrocytes (Shigetomi et al., 2013). TRPV1 and TRPV4 are also Ca2+-permeable channels expressed in astrocytes. Ca2+ entry through TRPV1 channel, but not Ca2+ entry through P2X4, induced strong Ca2+-dependent inactivation of Orai channels, thereby reducing Orai mediated Ca2+ entry, which also reduced wound healing measured by scratch-wound assay. This suggests that astrocytic Ca2+ signals induced by different channels would have different functions (Bastian-Eugenio et al., 2019). TRPV4 expression was also shown to be upregulated in ischemia, and it may be one underlying factor of astrocytic Ca2+ elevation following middle cerebral artery occlusion (Rakers et al., 2017). TRPC is another contributor to the astrocytic Ca2+ influx (Belkacemi et al., 2017). TRPC is a stretch-activated channel that mediates store-operated Ca2+ entry, similar to Orai channels. Orai and TRPC form complexes and participate in Ca2+ entry into astrocytes (Liao et al., 2009; Verkhratsky et al., 2014). However, the activation mechanism of the two channels slightly differs: Orai is STIM dependent, while TRPC could be either STIM dependent or independent. TRPC also has been implicated in the gliotransmitter release, showing that blocking TRPC1 reduced mechanically induced Ca2+-dependent Glu release (Malarkey et al., 2008). In the TRC3 knockout mice, TRPC3 mediated Ca2+ entry into astrocytes, and injury-induced astrogliosis was reduced (Belkacemi et al., 2017).
NCX working in reverse mode could also elevate astrocytic Ca2+. NCX usually works in a forward mode, extruding Ca2+ and transporting Na+ into the cell. When intracellular Na+ concentration increases, NCX extrudes Na+ and brings Ca2+ in Brazhe et al. (2018) and Wade et al. (2019). Several mechanisms are reported to increase intracellular Na+ concentration in astrocytes. NMDA mediated Na+ influx into neocortical astrocytes drive Ca2+ influx through NCX (Rose et al., 2020). Neurotransmitter uptake mediated by transporters such as GLT-1 or GAT-3 increase Na2+ in astrocytes, driving NCX to operate in reverse mode (Bazargani and Attwell, 2016). NCX working in reverse mode could be an important source of Ca2+ elevation in fine astrocytic processes, such as at PAPs that ensheath synapse. NCX was found to be enriched in PAPs, and the extremely fine nanometer volume of PAPs impedes the diffusion of Ca2+ (Rusakov et al., 2011; Rusakov, 2015). ER and mitochondria, which are intracellular sources of Ca2+, are absent in the fine astrocytic process likely due to physical constraints. Therefore, it is reasonable to assume that Ca2+ activity in fine astrocytic processes is segregated from Ca2+ activity in the soma or thick processes and that the major source of Ca2+ increase in fine astrocytic processes is an influx from the extracellular space.
Voltage-gated Ca2+ channels (VGCCs) could also contribute to astrocytic Ca2+ elevation. Several types of voltage-gated Ca2+ channels—L, N, R, and T types—are expressed in astrocytes. Although astrocytes are not electrically excitable, cultured astrocytes responded with an increase in intracellular Ca2+ to electrical stimulation (Latour et al., 2003; D’Ascenzo et al., 2004). Astrocytic Ca2+ changes mediated by VGCCs were also reported in slice experiments in the subventricular zone and ventrobasal thalamus (Parri et al., 2001; Parri and Crunelli, 2003; Young et al., 2010). Whether these channels are functional in resting astrocytes is unclear (Carmignoto et al., 1998), but L-type Ca2+ channel in astrocytes was significantly upregulated with inflammation or injury and contribute to the consequent increase in astrocytic Ca2+ (Westenbroek et al., 1998; Cheli et al., 2016). Blocking L- and N-type Ca2+ channel antagonist reduced the death of neurons and astrocytes (Gurkoff et al., 2013), and specific knockdown/knockout of L-type Ca2+ channels (Cav1.2) in astrocytes prevented astrocyte activation, reduced inflammation in the brain, and promoted myelin regeneration (Zamora et al., 2020), suggesting that astrocytic VGCCs could be therapeutic targets to reduce inflammation and promote recovery.
As described above, various channels lead to different Ca2+ dynamics in astrocytes. An increase in astrocytic Ca2+ is not a uniform process. Rather Ca2+ increases mediated by different channels having diverse consequences. Astrocyte diversity among different brain regions has been well characterized (Matias et al., 2019). Even within the same brain region, diverse populations of astrocytes with different functions have been reported (Yu et al., 2018). Sexual dimorphism of astrocytes has also been reported (Chen et al., 2018), and this could be one of the factors underlying the difference in pain prevalence between males and females. Therefore, the multi-faceted sources and consequence of astrocytic Ca2+ dynamics should be considered when investigating astrocyte physiology.
Astrocyte Activation Along the Pain Pathway With Chronic Pain
Astrocytes react to noxious stimulation and injury and become reactive astrocytes. There are mixed opinions on what defines a reactive astrocyte (Escartin et al., 2019), but reactive astrocytes are often characterized by morphological changes (hypertrophy), proliferation, gene expression changes, receptor changes, and functional changes. Functions of reactive astrocytes are completely different from astrocytes in a non-reactive state called resting astrocytes. Resting astrocytes have been suggested to attenuate pain, while reactive astrocytes have been suggested to contribute to chronic pain (Ji et al., 2019). Chronic pain discussed in this review will mainly include long-lasting pathological pain caused by inflammation or nerve damage. Dual effects of reactive astrocytes are gaining attention with the finding of two different types of reactive astrocytes named the A1 and A2, which have neurodegenerative and neuroprotective effects, respectively (Liddelow and Barres, 2017; Liddelow et al., 2017). Complement component 3 (C3) is the most highly upregulated gene in the A1 subtype, while the S100A10 was pointed out to be A2 subtype specific (Liddelow et al., 2017). Although the presence of A1 and A2 reactive astrocytes has been identified, whether they play different roles in chronic pain is uninvestigated. It is speculated, however, that the A1 subtype would cause chronic pain while the A2 subtype will reduce chronic pain (Li et al., 2019). Reactive astrocytes have been suggested as one of the factors contributing to the maintenance of chronic pain. Expression of glial fibrillary acidic protein (GFAP), a common marker of reactive astrocytes, was enhanced for 9 months in rats following spinal nerve injury (Gwak et al., 2012). Studies showing that inhibition of GFAP expression reduces neuropathic pain behaviors suggest that GFAP upregulation could lead to chronic pain (Ji et al., 2013). Moreover, injecting reactive astrocytes into the spinal cord induced mechanical allodynia in naïve mice, while drugs inhibiting astrocyte activation reversed mechanical allodynia (Gao et al., 2010), strongly supporting that reactive astrocytes contribute to triggering and maintaining chronic pain symptoms. These studies suggest a potential causative relationship between GFAP upregulation in reactive astrocytes and chronic pain.
Pain has both sensory discriminative (location and intensity) and affective (emotional unpleasantness) aspects, which are separately processed by discriminatory and the affective pain pathways. Interestingly, in chronic pain models, reactive astrocytes are found not only at the site of noxious stimulation or injury but also along both pain pathways (Figure 1). Astrocytes were activated in brain regions associated with the discriminative aspect of pain (the spinal cord, thalamus, and primary sensory cortex) and with the affective-cognitive aspect of pain [the anterior cingulate cortex (ACC), amygdala, medial prefrontal cortex, and hippocampus] (to be discussed in subsequent paragraphs). Similar glial activation patterns were reported in a human in vivo study, suggesting that finding in animal studies could be translated to humans. Using PET/MRI scanners and a marker of glial activation, Loggia et al. (2015) found that glial activation was higher in the thalamus, somatosensory cortex, cingulate cortex, ventromedial prefrontal cortex, and insular cortex in patients suffering from chronic back pain compared with control subjects (Loggia et al., 2015).
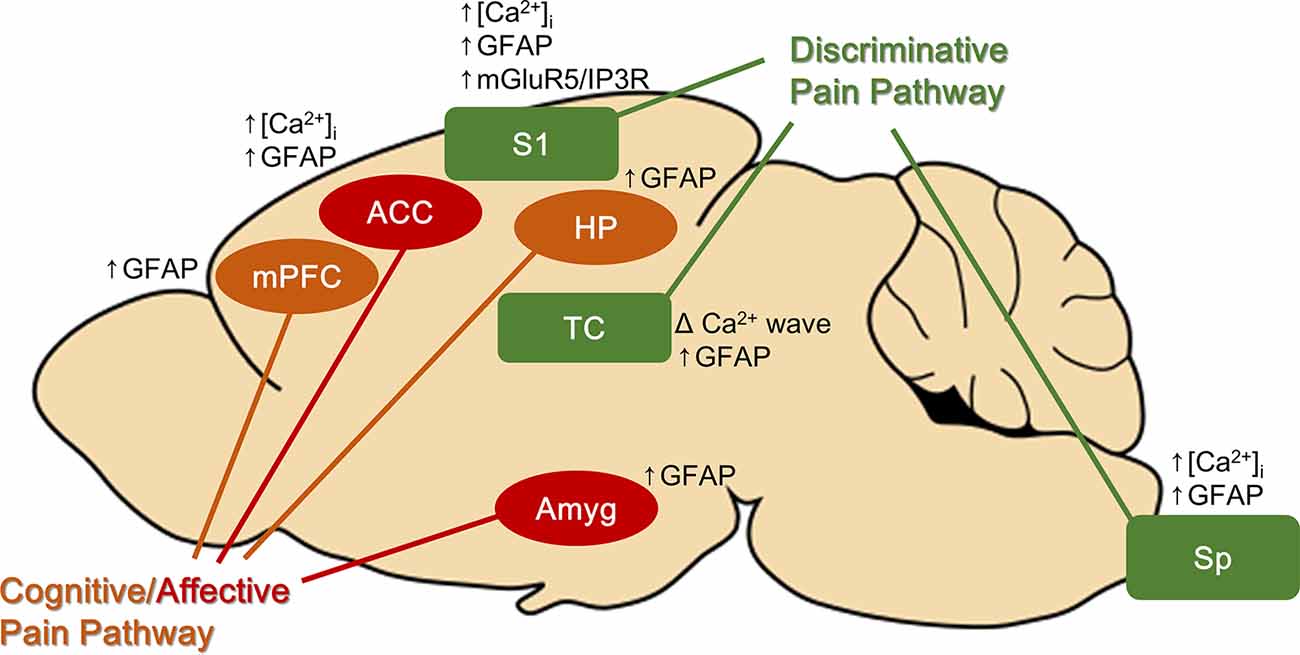
Figure 1. Brain areas found to have reactive astrocytes with chronic pain. Astrocytes in both discriminative and cognitive/affective pain pathways are activated with chronic pain. Some of the brain regions have astrocytic Ca2+ signal changes. ACC, anterior cingulate cortex; Amyg, amygdala; HP, hippocampus; mPFC, medial prefrontal cortex; S1, primary somatosensory cortex; Sp, spinal cord.
Numerous studies have suggested that reactive astrocytes in the spinal cord are associated with chronic pain. Astrocyte activation, indicated by GFAP upregulation, is reported in various pain models (Garrison et al., 1991; Raghavendra et al., 2004; Zhuang et al., 2006; Shi et al., 2012). Reducing GFAP in the spinal cord, by intrathecal injection of GFAP antisense oligonucleotide, reduced pain behaviors associated with spinal nerve ligation injury in rats (Kim et al., 2009). Also, direct activation of astrocytes in the spinal cord in naïve mice, using an optogenetic technique, induced symptoms of neuropathic pain such as mechanical and thermal hypersensitivity (Nam et al., 2016), supporting that activating astrocytes alone is enough to generate neuropathic pain-like behaviors.
Astrocytes in the thalamus, part of the discriminative pain pathway, were also found to be reactive under chronic pain conditions. Chronic constriction injury, which causes neuropathic pain, significantly enhanced GFAP expression in the thalamus (Giardini et al., 2017). Spinal nerve ligation, another neuropathic pain model, also increased the GFAP-immunostained area in the ventral posterolateral thalamus (VPL), while the number of GFAP/S100b+ astrocytes remained unchanged (Blaszczyk et al., 2018). This indicates that astrocytes in VPL increased their volume, indicative of hypertrophy, while their number did not change (no astrocyte proliferation). However, another study that investigated supra-spinal astrocyte reactivity, using the spared nerve injury model, did not find any differences in GFAP expression in the thalamus (Marcello et al., 2013). This discrepancy may be due to a slight difference in pain models used or method of analysis. The involvement of thalamic astrocytes in chronic pain is less clear than reactive microglia in the thalamus, which are consistently found, but a human study suggested that reactive astrocytes in the thalamus may have clinical implications. Patients with neuropathic pain associated with aberrant thalamocortical rhythms (thalamocortical dysrhythmia) exhibited brain rhythms that oscillated in a frequency similar to the frequency of Ca2+ waves displayed by activated astrocytes (<0.1 Hz), suggesting that reactive astrocytes in the thalamus may be the underlying cause (Alshelh et al., 2016). Accordingly, treatment with palmitoylethanolamide reduced glial reactivity and reduced oscillatory activity in the pain pathway only in patients who experienced pain reduction (Alshelh et al., 2019).
The activity of astrocytes in the primary somatosensory cortex (S1), the region important for identifying the intensity and location of pain, is also enhanced by nerve injury. GFAP expression and frequency of astrocytic Ca2+ activity of astrocytes were enhanced in S1 (Kim et al., 2016; Ishikawa et al., 2018). Increased astrocytic Ca2+ activity in S1 after nerve injury was reportedly due to the resurgence in expression of the metabotropic Glu receptor 5 (mGluR5; Kim et al., 2016), which is almost absent in adult mice and humans under physiological conditions (Sun et al., 2013). mGluR5 activates IP3R, which leads to Ca2+ release from the ER. Inhibiting the resurgence of mGluR5 reduced allodynia while enhancing mGluR5 expression induced allodynia in naïve mice (Kim et al., 2016), strongly supporting that increased astrocytic Ca2+ signals by mGluR5 trigger chronic pain symptoms. Consistently, inhibiting S1 astrocyte metabolism with fluoroacetate, which also blocks astrocytic Ca2+ activity, reduced mirror pain (pain occurring in the unflicked contralateral side) resulting from nerve injury (Ishikawa et al., 2018).
Astrocytes in brain regions known to process the cognitive-affective aspect of pain are also activated with chronic pain. Sciatic nerve ligation significantly enhanced GFAP expression in the cingulate cortex (Kuzumaki et al., 2007; Yamashita et al., 2014). Injection of Complete Freund’s Adjuvant (CFA), which induces chronic inflammatory pain, also enhanced GFAP expression in the ACC bilaterally (Chen et al., 2012). Interestingly, L-alpha-aminoadipate (astrocyte toxin) injection into the ACC inhibited place escape/avoidance behavior, which measures aversive emotion associated with nociceptive stimuli, but paw withdrawal threshold was unaffected, suggesting that astrocyte activation in the ACC only regulates the affective component of pain (Chen et al., 2012). GFAP expression was also increased in the amygdala (brain structure implicated in processing negative emotion) and the medial prefrontal cortex (structure implicated in cognitive processing; Alvarado et al., 2013; Marcello et al., 2013). Enhanced GFAP expression is also reported in the hippocampus (structure important for learning and memory; Zhu et al., 2009).
The mechanism underlying the activation of astrocytes along the pain processing pathway—structures not directly affected by injury—during chronic pain remains unknown. One possible mechanism could be through the propagation of Ca2+ waves between astrocytes connected by gap junctions. As described in the “Introduction” section, reactive astrocytes exhibit prominent Ca2+ waves, which enable long-distance communication. Astrocytes are physically connected via gap junctions, composed mainly of connexin (Cx) subtype Cx43 (Nagy et al., 1999). Ca2+ waves propagate by diffusion of IP3 through gap junctions (short distance signaling) or diffusion of extracellular ATP (long-distance signaling; Fujii et al., 2017). Connexins could also act as hemichannels releasing various molecules such as ATP, Glu, and chemokines, which can contribute to astrocyte activation. Cx43 is upregulated in reactive astrocytes and implicated to have important roles in chronic pain development and maintenance (Xing et al., 2019). In particular, mirror pain induced by nerve injury or inflammation was reduced by carbenoxolone, a non-selective gap junction inhibitor (Spataro et al., 2004; Choi et al., 2017), suggesting that astrocytic gap junctions could contribute to astrocyte activation along the pain pathway. However, Cx43 alone may not regulate pain sensitivity, as suggested by a recent finding that downregulation of spinal astrocytic Cx43 induced mechanical hypersensitivity in naïve mice (Morioka et al., 2018).
Relationship Between Astrocytic Calcium and Pain
While some studies suggest that aberrant changes in astrocytic Ca2+ underlie pathological pain, detailed mechanisms remain to be determined. Generally, increased astrocytic Ca2+ has been associated with increased pain, while reduced astrocytic Ca2+ has been associated with reduced pain. We can begin to understand these mechanisms by taking into consideration the various sources and consequences of astrocytic Ca2+ changes (as described in “Source of Calcium in Astrocytes” section above).
Astrocytic Ca2+ increased in the S1 and the ACC following nerve injury (Yamashita et al., 2014; Kim et al., 2016; Ishikawa et al., 2018). Astrocytic Ca2+ changes were also shown to be associated with synaptogenic thrombospondin (TSP-1) release and synapse formation, indicating that rewired circuits could cause neuropathic pain (Kim et al., 2016). Directly activating spinal astrocytes with optogenetic [channelrhodopsin-2 (ChR2)] stimulation, which increased astrocytic Ca2+, also triggered mechanical and thermal hypersensitivity in naïve animals (Nam et al., 2016), much like that seen in animals inflicted with chronic pain, suggesting that an increase in astrocytic Ca2+ can contribute to the induction of pain. However, since ChR2 activation of spinal astrocytes also induced the release of ATP and pro-inflammatory mediators along with enhanced GFAP expression (Nam et al., 2016), enhanced astrocytic Ca2+ alone may not be enough to induce pain hypersensitivity. An increase in Ca2+ waves generated by reactive astrocytes is also suggested to contribute to pathological pain symptoms in patients with neuropathic pain (Alshelh et al., 2016).
Conversely, reduction in astrocytic Ca2+ has been associated with attenuated pain responses. Blocking astrocytic Ca2+ with fluoroacetate, astrocyte metabolism inhibitor, reduced mirror pain responses that accompany neuropathic pain (Ishikawa et al., 2018). IP3R2 knockout mice, which have significantly reduced astrocytic Ca2+ signals, especially the prominent astrocytic Ca2+ signals in the cell body as discussed in “Source of Calcium in Astrocytes” section, also displayed reduced mirror pain following nerve injury (Ishikawa et al., 2018).
Up until now, only a few studies have investigated the relationship between astrocytic Ca2+ and chronic pain, but these studies consistently suggest that a global, not local, increase in astrocytic Ca2+ may cause chronic pain. Activation of both the IP3R and the ChR2 induce global Ca2+ change in astrocytes. The specific type of Ca2+ channels involved in ChR-2 mediated changes in astrocytic Ca2+ is uncertain. However given that ChR2 is a light-activated non-selective cation channel, ChR2 activation will have induced Ca2+ influx, along with other cations, from extracellular space. Influx, or efflux, of cations via ChR2 may further activate other Ca2+ channels that could increase astrocytic Ca2+, leading into a chain reaction of intracellular Ca2+ increase. Spreading Ca2+ increase between astrocytes by ChR2 activation may also contribute to the neuropathic pain-like symptoms since photo-stimulation of astrocytes also released ATP, a molecule important for generating astrocytic Ca2+ waves. Furthermore, photo-stimulation of the ChR2 expressing astrocyte increases intracellular Ca2+ even in astrocytes that do not express ChR2, reportedly via NMDA receptor activation (Berlinguer-Palmini et al., 2014). These studies support the possibility that propagation of Ca2+ waves between astrocytes by photo-stimulation could have caused pro-inflammatory mediator release and enhanced GFAP expression, which eventually could have caused neuropathic pain-like symptoms in naïve mice.
How the increase in astrocytic Ca2+ leads to chronic pain is unclear, but changes in the interaction between neuron–astrocyte may be one factor. Kim et al. (2016) showed that astrocytic Ca2+ changes regulate the release of the synaptogenic molecule thrombospondin (TSP-1), which remodel synapses. Altered connectivity between neurons and associated hyperactivity may be the underlying cause of chronic pain. Since reactive astrocyte exhibits prominent Ca2+-dependent gliotransmitter release, enhanced Glu released by reactive astrocytes that excessively excite neurons may also contribute to chronic pain. Changes in neurotransmitter buffering activity could also contribute to the changes in neuron–astrocyte interaction ensuing chronic pain. Buffering of the inhibitory transmitter—GABA—by astrocytes via GAT is directly affected by astrocytic Ca2+, with more GABA taken up with increasing Ca2+. Although GAT activity was shown to enhance the synaptic efficacy of an inhibitory synapse by reducing ambient GABA that desensitize the inhibitory synapse (Shigetomi et al., 2011), this condition may not apply to reactive astrocytes. Increased internal Ca2+ of reactive astrocytes may greatly reduce the synaptic GABA concentration to a level that reduces the efficacy of inhibitory synapses.
The release of inflammatory mediators by elevated astrocytic Ca2+ may also contribute to chronic pain symptoms. Activation of STIM1 and Orai1 channels, which are major channels for Ca2+ influx from the extracellular space, promoted the production of pro-inflammatory mediators such as TNFα and IL-6 in spinal astrocytes (Gao et al., 2016). The secretion of IL-6 was also shown to be regulated by astrocytic Ca2+ (Codeluppi et al., 2014). Inflammatory mediators are well-known to enhance astrocytic Ca2+ (Agulhon et al., 2012) and will further amplify astrocytic Ca2+ signaling. Overall, these studies suggest that astrocytic Ca2+ and chronic pain have a close interrelationship, but further studies need to be carried out to reveal more detailed mechanisms. Multiple interrelated events triggered by astrocytic Ca2+, some occurring sequentially as astrocytic cytokine expression precedes increased GFAP expression (Norden et al., 2016), will likely contribute to the development of chronic pain. Although few studies have directly investigated the relationship between astrocytic Ca2+ and pain, numerous studies report that various channels, discussed in “Source of Calcium in Astrocytes” section, contribute to an increase in astrocytic Ca2+ after injury or inflammation, conditions that could cause pain. Other than the IP3R, which was shown to be related to chronic pain, RyR3, STIM1, Orai1, TRPs, and VGCCs also have been implicated in injury/inflammation and ensuing astrocyte activation. Since astrocytic Ca2+ signals induced by different channels have different effects and functions, it would be interesting to investigate how each of these channels differentially contributes to chronic pain.
Conclusion
Astrocytic Ca2+ is integral in maintaining CNS function. Following an injury, astrocytes become reactive and go under morphological, chemical, and functional changes. Astrocytic Ca2+ dynamics also change with injury, inflammation, or pain. Astrocyte Ca2+ channels shown to increase expression/activity with inflammation or injury include IP3R, Orai, Ca2+-permeable TRP channels, and VGCCs. Gap junction channels are also Ca2+ permeable, and Cx43 was reported to be upregulated with injury, but the diffusion of Ca2+ through gap junctions is slow and their contribution to Ca2+ signaling would be relatively small. These changes all enhance intracellular astrocytic Ca2+ concentration, so an increase in astrocytic Ca2+ appears to underlie pathological pain. However, it is not clear what potentiated astrocytic Ca2+ signal does to cause pathological pain. Does it act to protect or damage the CNS? The answer may not be clear-cut, as in the example of glial scars. Glial scars were viewed as an obstacle to functional recovery after damage because it hinders axon regeneration; however, preventing glial scar formation results in greater damage (Sofroniew, 2015). Likewise, potentiated astrocytic Ca2+ will likely have both protective and detrimental effects. On the other hand, different types of reactive astrocytes, the A1 (neurotoxic) and the A2 (neuroprotective; Liddelow and Barres, 2017; Liddelow et al., 2017), may have different effects on chronic pain. Although the presence or contribution of A2 reactive astrocytes to chronic pain is not yet validated, the protective effect neuroprotective effect exerted by reactive astrocytes after an injury is speculated to be attributed by the A2 subtype, which are induced following injuries such as ischemia (Li et al., 2019). Whether and how the two different types of reactive astrocytes lead to distinct changes in astrocytic Ca2+ is unknown. It is possible that astrocytic Ca2+ induced by different channels may drive reactive astrocytes to be one type or the other. Therefore, investigating how different astrocytic Ca2+ channels contribute to different types of reactive astrocytes in regard to chronic pain development will provide a deeper understanding of the roles of astrocyte in pain and may offer better targets for pain control.
Author Contributions
JC and YH contributed equally to the manuscript. All authors contributed to the article and approved the submitted version.
Funding
This research was funded by the Ministry of Science ICT through the National Research Foundation of Korea (NRF) grants: NRF-2018M3C7A1024736, NRF-2018R1C1B6002210, and NRF-2019R1A2C2088377.
Conflict of Interest
The authors declare that the research was conducted in the absence of any commercial or financial relationships that could be construed as a potential conflict of interest.
Acknowledgments
We thank Frances Cho for proofreading the manuscript.
References
Adamsky, A., Kol, A., Kreisel, T., Doron, A., Ozeri-Engelhard, N., Melcer, T., et al. (2018). Astrocytic activation generates de novo neuronal potentiation and memory enhancement. Cell 174, 59.e14–71.e14. doi: 10.1016/j.cell.2018.05.002
Agarwal, A., Wu, P. H., Hughes, E. G., Fukaya, M., Tischfield, M. A., Langseth, A. J., et al. (2017). Transient opening of the mitochondrial permeability transition pore induces microdomain calcium transients in astrocyte processes. Neuron 93, 587.e7–605.e7. doi: 10.1016/j.neuron.2016.12.034
Agulhon, C., Petravicz, J., McMullen, A. B., Sweger, E. J., Minton, S. K., Taves, S. R., et al. (2008). What is the role of astrocyte calcium in neurophysiology? Neuron 59, 932–946. doi: 10.1016/j.neuron.2008.09.004
Agulhon, C., Sun, M. Y., Murphy, T., Myers, T., Lauderdale, K., and Fiacco, T. A. (2012). Calcium signaling and gliotransmission in normal vs. reactive astrocytes. Front. Pharmacol 3:139. doi: 10.3389/fphar.2012.00139
Alshelh, Z., Di Pietro, F., Youssef, A. M., Reeves, J. M., Macey, P. M., Vickers, E. R., et al. (2016). Chronic neuropathic pain: it’s about the rhythm. J. Neurosci. 36, 1008–1018. doi: 10.1523/JNEUROSCI.2768-15.2016
Alshelh, Z., Mills, E. P., Kosanovic, D., Di Pietro, F., Macey, P. M., Vickers, E. R., et al. (2019). Effects of the glial modulator palmitoylethanolamide on chronic pain intensity and brain function. J. Pain Res. 12, 2427–2439. doi: 10.2147/JPR.S209657
Alvarado, S., Tajerian, M., Millecamps, M., Suderman, M., Stone, L. S., and Szyf, M. (2013). Peripheral nerve injury is accompanied by chronic transcriptome-wide changes in the mouse prefrontal cortex. Mol. Pain 9:21. doi: 10.1186/1744-8069-9-21
Bastian-Eugenio, C. E., Bohorquez-Hernandez, A., Pacheco, J., Sampieri, A., Asanov, A., Ocelotl-Oviedo, J. P., et al. (2019). Heterologous calcium-dependent inactivation of Orai1 by neighboring TRPV1 channels modulates cell migration and wound healing. Commun. Biol. 2:88. doi: 10.1186/1744-8069-9-21
Bazargani, N., and Attwell, D. (2016). Astrocyte calcium signaling: the third wave. Nat. Neurosci. 19, 182–189. doi: 10.1038/nn.4201
Beck, A., Nieden, R. Z., Schneider, H. P., and Deitmer, J. W. (2004). Calcium release from intracellular stores in rodent astrocytes and neurons in situ. Cell Calcium 35, 47–58. doi: 10.1016/s0143-4160(03)00171-4
Belkacemi, T., Niermann, A., Hofmann, L., Wissenbach, U., Birnbaumer, L., Leidinger, P., et al. (2017). TRPC1- and TRPC3-dependent Ca2+ signaling in mouse cortical astrocytes affects injury-evoked astrogliosis in vivo. Glia 65, 1535–1549. doi: 10.1002/glia.23180
Bellot-Saez, A., Kekesi, O., Morley, J. W., and Buskila, Y. (2017). Astrocytic modulation of neuronal excitability through K+ spatial buffering. Neurosci. Biobehav. Rev. 77, 87–97. doi: 10.1016/j.neubiorev.2017.03.002
Berlinguer-Palmini, R., Narducci, R., Merhan, K., Dilaghi, A., Moroni, F., Masi, A., et al. (2014). Arrays of microLEDs and astrocytes: biological amplifiers to optogenetically modulate neuronal networks reducing light requirement. PLoS One 9:e108689. doi: 10.1371/journal.pone.0108689
Bernardinelli, Y., Randall, J., Janett, E., Nikonenko, I., König, S., Jones, E. V., et al. (2014). Activity-dependent structural plasticity of perisynaptic astrocytic domains promotes excitatory synapse stability. Curr. Biol. 24, 1679–1688. doi: 10.1016/j.cub.2014.06.025
Blaszczyk, L., Maitre, M., Leste-Lasserre, T., Clark, S., Cota, D., Oliet, S. H. R., et al. (2018). Sequential alteration of microglia and astrocytes in the rat thalamus following spinal nerve ligation. J. Neuroinflammation 15:349. doi: 10.1186/s12974-018-1378-z
Brazhe, A. R., Verisokin, A. Y., Verveyko, D. V., and Postnov, D. E. (2018). Sodium-calcium exchanger can account for regenerative Ca2+ entry in thin astrocyte processes. Front. Cell. Neurosci. 12:250. doi: 10.3389/fncel.2018.00250
Buskila, Y., Bellot-Saez, A., and Morley, J. W. (2019). Generating brain waves, the power of astrocytes. Front. Neurosci. 13:1125. doi: 10.3389/fnins.2019.01125
Carmignoto, G., Pasti, L., and Pozzan, T. (1998). On the role of voltage-dependent calcium channels in calcium signaling of astrocytes in situ. J. Neurosci. 18, 4637–4645. doi: 10.1523/JNEUROSCI.18-12-04637.1998
Charles, A. (1998). Intercellular calcium waves in glia. Glia 24, 39–49. doi: 10.1002/(sici)1098-1136(199809)24:1<39::aid-glia5>3.0.co;2-w
Cheli, V. T., Santiago Gonzalez, D. A., Smith, J., Spreuer, V., Murphy, G. G., and Paez, P. M. (2016). L-type voltage-operated calcium channels contribute to astrocyte activation in vitro. Glia 64, 1396–1415. doi: 10.1002/glia.23013
Chen, F. L., Dong, Y. L., Zhang, Z. J., Cao, D. L., Xu, J., Hui, J., et al. (2012). Activation of astrocytes in the anterior cingulate cortex contributes to the affective component of pain in an inflammatory pain model. Brain Res. Bull. 87, 60–66. doi: 10.1016/j.brainresbull.2011.09.022
Chen, G., Luo, X., Qadri, M. Y., Berta, T., and Ji, R. R. (2018). Sex-dependent glial signaling in pathological pain: distinct roles of spinal microglia and astrocytes. Neurosci. Bull. 34, 98–108. doi: 10.1007/s12264-017-0145-y
Choi, H. S., Roh, D. H., Yoon, S. Y., Kwon, S. G., Choi, S. R., Kang, S. Y., et al. (2017). The role of spinal interleukin-1β and astrocyte connexin 43 in the development of mirror-image pain in an inflammatory pain model. Exp. Neurol. 287, 1–13. doi: 10.1016/j.expneurol.2016.10.012
Codeluppi, S., Fernandez-Zafra, T., Sandor, K., Kjell, J., Liu, Q., Abrams, M., et al. (2014). Interleukin-6 secretion by astrocytes is dynamically regulated by PI3K-mTOR-calcium signaling. PLoS One 9:e92649. doi: 10.1371/journal.pone.0092649
Cornell-Bell, A. H., Finkbeiner, S. M., Cooper, M. S., and Smith, S. J. (1990). Glutamate induces calcium waves in cultured astrocytes: long-range glial signaling. Science 247, 470–473. doi: 10.1126/science.1967852
Dani, J. W., Chernjavsky, A., and Smith, S. J. (1992). Neuronal activity triggers calcium waves in hippocampal astrocyte networks. Neuron 8, 429–440. doi: 10.1016/0896-6273(92)90271-e
D’Ascenzo, M., Vairano, M., Andreassi, C., Navarra, P., Azzena, G. B., and Grassi, C. (2004). Electrophysiological and molecular evidence of L-(Cav1), N- (Cav2.2) and R- (Cav2.3) type Ca2+ channels in rat cortical astrocytes. Glia 45, 354–363. doi: 10.1002/glia.10336
Dermietzel, R., Hertberg, E. L., Kessler, J. A., and Spray, D. C. (1991). Gap junctions between cultured astrocytes: immunocytochemical, molecular and electrophysiological analysis. J. Neurosci. 11, 1421–1432. doi: 10.1523/JNEUROSCI.11-05-01421.1991
Dzamba, D., Honsa, P., and Anderova, M. (2013). NMDA receptors in glial cells: pending questions. Curr. Neuropharmacol. 11, 250–262. doi: 10.2174/1570159X11311030002
Escartin, C., Guillemaud, O., and Carrillo-de Sauvage, M. A. (2019). Questions and (some) answers on reactive astrocytes. Glia 67, 2221–2247. doi: 10.1002/glia.23687
Fiacco, T. A., and McCarthy, K. D. (2018). Multiple lines of evidence indicate that gliotransmission does not occur under physiological conditions. J. Neurosci. 38, 3–13. doi: 10.1523/JNEUROSCI.0016-17.2017
Fujii, Y., Maekawa, S., and Morita, M. (2017). Astrocyte calcium waves propagate proximally by gap junction and distally by extracellular diffusion of ATP released from volume-regulated anion channels. Sci. Rep. 7:13115. doi: 10.1038/s41598-017-13243-0
Fumagalli, M., Brambilla, R., D’Ambrosi, N., Volonte, C., Matteoli, M., Verderio, C., et al. (2003). Nucleotide-mediated calcium signaling in rat cortical astrocytes: role of P2X and P2Y receptors. Glia 43, 218–230. doi: 10.1002/glia.10248
Gao, X., Xia, J., Munoz, F. M., Manners, M. T., Pan, R., Meucci, O., et al. (2016). STIMs and Orai1 regulate cytokine production in spinal astrocytes. J. Neuroinflammation 13:126. doi: 10.1186/s12974-016-0594-7
Gao, Y. J., Zhang, L., and Ji, R. R. (2010). Spinal injection of TNF-alpha-activated astrocytes produces persistent pain symptom mechanical allodynia by releasing monocyte chemoattractant protein-1. Glia 58, 1871–1880. doi: 10.1002/glia.21056
Garrison, C. J., Dougherty, P. M., Kajander, K. C., and Carlton, S. M. (1991). Staining of glial fibrillary acidic protein (GFAP) in lumbar spinal cord increases following a sciatic nerve constriction injury. Brain Res. 565, 1–7. doi: 10.1016/0006-8993(91)91729-k
Giardini, A. C., Dos Santos, F. M., da Silva, J. T., de Oliveira, M. E., Martins, D. O., and Chacur, M. (2017). Neural mobilization treatment decreases glial cells and brain-derived neurotrophic factor expression in the central nervous system in rats with neuropathic pain induced by CCI in rats. Pain Res. Manag. 2017:7429761. doi: 10.1155/2017/7429761
Guerra-Gomes, S., Sousa, N., Pinto, L., and Oliveira, J. F. (2017). Functional roles of astrocyte calcium elevations: from synapses to behavior. Front. Cell. Neurosci. 11:427. doi: 10.3389/fncel.2017.00427
Gurkoff, G., Shahlaie, K., Lyeth, B., and Berman, R. (2013). Voltage-gated calcium channel antagonists and traumatic brain injury. Pharmaceuticals 6, 788–812. doi: 10.3390/ph6070788
Gwak, Y. S., Kang, J., Unabia, G. C., and Hulsebosch, C. E. (2012). Spatial and temporal activation of spinal glial cells: role of gliopathy in central neuropathic pain following spinal cord injury in rats. Exp. Neurol. 234, 362–372. doi: 10.1016/j.expneurol.2011.10.010
Halassa, M. M., Fellin, T., and Haydon, P. G. (2007). The tripartite synapse: roles for gliotransmission in health and disease. Trends Mol. Med. 13, 54–63. doi: 10.1016/j.molmed.2006.12.005
Hewavitharana, T., Deng, X., Soboloff, J., and Gill, D. L. (2007). Role of STIM and Orai proteins in the store-operated calcium signaling pathway. Cell Calcium 42, 173–182. doi: 10.1016/j.ceca.2007.03.009
Iino, M., Goto, K., Kakegawa, W., Okado, H., Sudo, M., Ishiuchi, S., et al. (2001). Glia-synapse interaction through Ca2+-permeable AMPA receptors in Bergmann glia. Science 292, 926–929. doi: 10.1126/science.1058827
Ishikawa, T., Eto, K., Kim, S. K., Wake, H., Takeda, I., Horiuchi, H., et al. (2018). Cortical astrocytes prime the induction of spine plasticity and mirror image pain. Pain 159, 1592–1606. doi: 10.1097/j.pain.0000000000001248
Jacobson, J., and Duchen, M. R. (2002). Mitochondrial oxidative stress and cell death in astrocytes—requirement for stored Ca2+ and sustained opening of the permeability transition pore. J. Cell Sci. 115, 1175–1188. Available online at: https://jcs.biologists.org/content/115/6/1175.
Ji, R. R., Berta, T., and Nedergaard, M. (2013). Glia and pain: is chronic pain a gliopathy? Pain 154, S10–S28. doi: 10.1016/j.pain.2013.06.022
Ji, R.-R., Donnelly, C. R., and Nedergaard, M. (2019). Astrocytes in chronic pain and itch. Nat. Rev. Neurosci. 20, 667–685. doi: 10.1038/s41583-019-0218-1
Kim, D. S., Figueroa, K. W., Li, K. W., Boroujerdi, A., Yolo, T., and Luo, Z. D. (2009). Profiling of dynamically changed gene expression in dorsal root ganglia post peripheral nerve injury and a critical role of injury-induced glial fibrillary acidic protein in maintenance of pain behaviors [corrected]. Pain 143, 114–122. doi: 10.1016/j.pain.2009.02.006
Kim, S. K., Hayashi, H., Ishikawa, T., Shibata, K., Shigetomi, E., Shinozaki, Y., et al. (2016). Cortical astrocytes rewire somatosensory cortical circuits for peripheral neuropathic pain. J. Clin. Invest. 126, 1983–1997. doi: 10.1172/JCI82859
Kuzumaki, N., Narita, M., Narita, M., Hareyama, N., Niikura, K., Nagumo, Y., et al. (2007). Chronic pain-induced astrocyte activation in the cingulate cortex with no change in neural or glial differentiation from neural stem cells in mice. Neurosci. Lett. 415, 22–27. doi: 10.1016/j.neulet.2006.12.057
Latour, I., Hamid, J., Beedle, A. M., Zamponi, G. W., and Macvicar, B. A. (2003). Expression of voltage-gated Ca2+ channel subtypes in cultured astrocytes. Glia 41, 347–353. doi: 10.1002/glia.10162
Li, T., Chen, X., Zhang, C., Zhang, Y., and Yao, W. (2019). An update on reactive astrocytes in chronic pain. J. Neuroinflammation 16:140. doi: 10.1186/s12974-019-1524-2
Liao, Y., Plummer, N. W., George, M. D., Abramowitz, J., Zhu, M. X., and Birnbaumer, L. (2009). A role for Orai in TRPC-mediated Ca2+ entry suggests that a TRPC:Orai complex may mediate store and receptor operated Ca2+ entry. Proc. Natl. Acad. Sci. U S A 106, 3202–3206. doi: 10.1073/pnas.0813346106
Liddelow, S. A., and Barres, B. A. (2017). Reactive astrocytes: production, function, and therapeutic potential. Immunity 46, 957–967. doi: 10.1016/j.immuni.2017.06.006
Liddelow, S. A., Guttenplan, K. A., Clarke, L. E., Bennett, F. C., Bohlen, C. J., Schirmer, L., et al. (2017). Neurotoxic reactive astrocytes are induced by activated microglia. Nature 541, 481–487. doi: 10.1038/nature21029
Loggia, M. L., Chonde, D. B., Akeju, O., Arabasz, G., Catana, C., Edwards, R. R., et al. (2015). Evidence for brain glial activation in chronic pain patients. Brain 138, 604–615. doi: 10.1093/brain/awu377
Mahmoud, S., Gharagozloo, M., Simard, C., and Gris, D. (2019). Astrocytes maintain glutamate homeostasis in the CNS by controlling the balance between glutamate uptake and release. Cells 8:184. doi: 10.3390/cells8020184
Malarkey, E. B., Ni, Y., and Parpura, V. (2008). Ca2+ entry through TRPC1 channels contributes to intracellular Ca2+ dynamics and consequent glutamate release from rat astrocytes. Glia 56, 821–835. doi: 10.1002/glia.20656
Marcello, L., Cavaliere, C., Colangelo, A. M., Bianco, M. R., Cirillo, G., Alberghina, L., et al. (2013). Remodelling of supraspinal neuroglial network in neuropathic pain is featured by a reactive gliosis of the nociceptive amygdala. Eur. J. Pain 17, 799–810. doi: 10.1002/j.1532-2149.2012.00255.x
Matias, I., Morgado, J., and Gomes, F. C. A. (2019). Astrocyte heterogeneity: impact to brain aging and disease. Front. Aging Neurosci. 11:59. doi: 10.3389/fnagi.2019.00059
Matthias, K., Kirchhoff, F., Seifert, G., Huttmann, K., Matyash, M., Kettenmann, H., et al. (2003). Segregated expression of AMPA-type glutamate receptors and glutamate transporters defines distinct astrocyte populations in the mouse hippocampus. J. Neurosci. 23, 1750–1758. doi: 10.1523/JNEUROSCI.23-05-01750.2003
Matyash, M., Matyash, V., Nolte, C., Sorrentino, V., and Kettenmann, H. (2002). Requirement of functional ryanodine receptor type 3 for astrocyte migration. FASEB J. 16, 84–86. doi: 10.1096/fj.01-0380fje
Minelli, A., DeBiasi, S., Brecha, N. C., Zuccarello, L. V., and Conti, F. (1996). GAT-3, a high-affinity GABA plasma membrane transporter, is localized to astrocytic processes and it is not confined to the vicinity of GABAergic synapses in the cerebral cortex. J. Neurosci. 16, 6255–6264. doi: 10.1523/JNEUROSCI.15-11-07734.1995
Morioka, N., Fujii, S., Kondo, S., Zhang, F. F., Miyauchi, K., Nakamura, Y., et al. (2018). Downregulation of spinal astrocytic connexin43 leads to upregulation of interleukin-6 and cyclooxygenase-2 and mechanical hypersensitivity in mice. Glia 66, 428–444. doi: 10.1002/glia.23255
Nagy, J. I., Patel, D., Ochalski, P. A., and Stelmack, G. L. (1999). Connexin30 in rodent, cat and human brain: selective expression in gray matter astrocytes, co-localization with connexin43 at gap junctions and late developmental appearance. Neuroscience 88, 447–468. doi: 10.1016/s0306-4522(98)00191-2
Nam, Y., Kim, J. H., Kim, J. H., Jha, M. K., Jung, J. Y., Lee, M. G., et al. (2016). Reversible induction of pain hypersensitivity following optogenetic stimulation of spinal astrocytes. Cell Rep. 17, 3049–3061. doi: 10.1016/j.celrep.2016.11.043
Nedergaard, M., Ransom, B., and Goldman, S. A. (2003). New roles for astrocytes: redefining the functional architecture of the brain. Trends Neurosci. 26, 523–530. doi: 10.1016/j.tins.2003.08.008
Norden, D. M., Trojanowski, P. J., Villanueva, E., Navarro, E., and Godbout, J. P. (2016). Sequential activation of microglia and astrocyte cytokine expression precedes increased Iba-1 or GFAP immunoreactivity following systemic immune challenge. Glia 64, 300–316. doi: 10.1002/glia.22930
Otsu, Y., Couchman, K., Lyons, D. G., Collot, M., Agarwal, A., Mallet, J. M., et al. (2015). Calcium dynamics in astrocyte processes during neurovascular coupling. Nat. Neurosci. 18, 210–218. doi: 10.1038/nn.3906
Pannasch, U., Freche, D., Dallerac, G., Ghezali, G., Escartin, C., Ezan, P., et al. (2014). Connexin 30 sets synaptic strength by controlling astroglial synapse invasion. Nat. Neurosci. 17, 549–558. doi: 10.1038/nn.3662
Pappalardo, L. W., Black, J. A., and Waxman, S. G. (2016). Sodium channels in astroglia and microglia. Glia 64, 1628–1645. doi: 10.1002/glia.22967
Parri, H. R., and Crunelli, V. (2003). The role of Ca2+ in the generation of spontaneous astrocytic Ca2+ oscillations. Neuroscience 120, 979–992. doi: 10.1016/s0306-4522(03)00379-8
Parri, H. R., Gould, T. M., and Crunelli, V. (2001). Spontaneous astrocytic Ca2+ oscillations in situ drive NMDAR-mediated neuronal excitation. Nat. Neurosci. 4, 803–812. doi: 10.1038/90507
Poskanzer, K. E., and Yuste, R. (2016). Astrocytes regulate cortical state switching in vivo. Proc. Natl. Acad. Sci. U S A 113, E2675–E2684. doi: 10.1073/pnas.1520759113
Quandt, F. N., and MacVicar, B. A. (1986). Calcium activated potassium channels in cultured astrocytes. Neuroscience 19, 29–41. doi: 10.1016/0306-4522(86)90003-5
Raghavendra, V., Tanga, F. Y., and DeLeo, J. A. (2004). Complete Freunds adjuvant-induced peripheral inflammation evokes glial activation and proinflammatory cytokine expression in the CNS. Eur. J. Neurosci. 20, 467–473. doi: 10.1111/j.1460-9568.2004.03514.x
Rakers, C., Schmid, M., and Petzold, G. C. (2017). TRPV4 channels contribute to calcium transients in astrocytes and neurons during peri-infarct depolarizations in a stroke model. Glia 65, 1550–1561. doi: 10.1002/glia.23183
Rose, C. R., Ziemens, D., and Verkhratsky, A. (2020). On the special role of NCX in astrocytes: translating Na+-transients into intracellular Ca2+ signals. Cell Calcium 86:102154. doi: 10.1016/j.ceca.2019.102154
Rungta, R. L., Bernier, L. P., Dissing-Olesen, L., Groten, C. J., LeDue, J. M., Ko, R., et al. (2016). Ca2+ transients in astrocyte fine processes occur via Ca2+ influx in the adult mouse hippocampus. Glia 64, 2093–2103. doi: 10.1002/glia.23042
Rusakov, D. A. (2015). Disentangling calcium-driven astrocyte physiology. Nat. Rev. Neurosci. 16, 226–233. doi: 10.1038/nrn3878
Rusakov, D. A., Zheng, K., and Henneberger, C. (2011). Astrocytes as regulators of synaptic function: a quest for the Ca2+ master key. Neuroscientist 17, 513–523. doi: 10.1177/1073858410387304
Santello, M., Cali, C., and Bezzi, P. (2012). Gliotransmission and the tripartite synapse. Adv. Exp. Med. Biol. 970, 307–331. doi: 10.1007/978-3-7091-0932-8_14
Savtchouk, I., and Volterra, A. (2018). Gliotransmission: beyond black-and-white. J. Neurosci. 38, 14–25. doi: 10.1523/JNEUROSCI.0017-17.2017
Scemes, E., and Giaume, C. (2006). Astrocyte calcium waves: what they are and what they do. Glia 54, 716–725. doi: 10.1002/glia.20374
Shi, Y., Gelman, B. B., Lisinicchia, J. G., and Tang, S. J. (2012). Chronic-pain-associated astrocytic reaction in the spinal cord dorsal horn of human immunodeficiency virus-infected patients. J. Neurosci. 32, 10833–10840. doi: 10.1523/JNEUROSCI.5628-11.2012
Shigetomi, E., Jackson-Weaver, O., Huckstepp, R. T., O’Dell, T. J., and Khakh, B. S. (2013). TRPA1 channels are regulators of astrocyte basal calcium levels and long-term potentiation via constitutive D-serine release. J. Neurosci. 33, 10143–10153. doi: 10.1523/JNEUROSCI.5779-12.2013
Shigetomi, E., Saito, K., Sano, F., and Koizumi, S. (2019). Aberrant calcium signals in reactive astrocytes: a key process in neurological disorders. Int. J. Mol. Sci. 20:996. doi: 10.3390/ijms20040996
Shigetomi, E., Tong, X., Kwan, K. Y., Corey, D. P., and Khakh, B. S. (2011). TRPA1 channels regulate astrocyte resting calcium and inhibitory synapse efficacy through GAT-3. Nat. Neurosci. 15, 70–80. doi: 10.1038/nn.3000
Smyth, J. T., Dehaven, W. I., Jones, B. F., Mercer, J. C., Trebak, M., Vazquez, G., et al. (2006). Emerging perspectives in store-operated Ca2+ entry: roles of Orai, Stim and TRP. Biochim. Biophys. Acta 1763, 1147–1160. doi: 10.1016/j.bbamcr.2006.08.050
Sofroniew, M. V. (2015). Astrocyte barriers to neurotoxic inflammation. Nat. Rev. Neurosci. 16, 249–263. doi: 10.1038/nrn3898
Spataro, L. E., Sloane, E. M., Milligan, E. D., Wieseler-Frank, J., Schoeniger, D., Jekich, B. M., et al. (2004). Spinal gap junctions: potential involvement in pain facilitation. J. Pain 5, 392–405. doi: 10.1016/j.jpain.2004.06.006
Srinivasan, R., Huang, B. S., Venugopal, S., Johnston, A. D., Chai, H., Zeng, H., et al. (2015). Ca2+ signaling in astrocytes from Ip3r2−/− mice in brain slices and during startle responses in vivo. Nat. Neurosci. 18, 708–717. doi: 10.1038/nn.4001
Stobart, J. L., Ferrari, K. D., Barrett, M. J. P., Gluck, C., Stobart, M. J., Zuend, M., et al. (2018a). Cortical circuit activity evokes rapid astrocyte calcium signals on a similar timescale to neurons. Neuron 98, 726.e4–735.e4. doi: 10.1016/j.neuron.2018.03.050
Stobart, J. L., Ferrari, K. D., Barrett, M. J. P., Stobart, M. J., Looser, Z. J., Saab, A. S., et al. (2018b). Long-term in vivo calcium imaging of astrocytes reveals distinct cellular compartment responses to sensory stimulation. Cereb. Cortex 28, 184–198. doi: 10.1093/cercor/bhw366
Sun, W., McConnell, E., Pare, J. F., Xu, Q., Chen, M., Peng, W., et al. (2013). Glutamate-dependent neuroglial calcium signaling differs between young and adult brain. Science 339, 197–200. doi: 10.1126/science.1226740
Suzuki, A., Stern, S. A., Bozdagi, O., Huntley, G. W., Walker, R. H., Magistretti, P. J., et al. (2011). Astrocyte-neuron lactate transport is required for long-term memory formation. Cell 144, 810–823. doi: 10.1016/j.cell.2011.02.018
Toth, A. B., Hori, K., Novakovic, M. M., Bernstein, N. G., Lambot, L., and Prakriya, M. (2019). CRAC channels regulate astrocyte Ca2+ signaling and gliotransmitter release to modulate hippocampal GABAergic transmission. Sci. Signal. 12:eaaw5450. doi: 10.1126/scisignal.aaw5450
Verkhratsky, A., Reyes, R. C., and Parpura, V. (2014). TRP channels coordinate ion signalling in astroglia. Rev. Physiol. Biochem. Pharmacol. 166, 1–22. doi: 10.1007/112_2013_15
Voutsinos-Porche, B., Knott, G., Tanaka, K., Quairiaux, C., Welker, E., and Bonvento, G. (2003). Glial glutamate transporters and maturation of the mouse somatosensory cortex. Cereb. Cortex 13, 1110–1121. doi: 10.1093/cercor/13.10.1110
Wade, J. J., Breslin, K., Wong-Lin, K., Harkin, J., Flanagan, B., Van Zalinge, H., et al. (2019). Calcium microdomain formation at the perisynaptic cradle due to NCX reversal: a computational study. Front. Cell. Neurosci. 13:185. doi: 10.3389/fncel.2019.00185
Wang, Y., Deng, X., Zhou, Y., Hendron, E., Mancarella, S., Ritchie, M. F., et al. (2009). STIM protein coupling in the activation of Orai channels. Proc. Natl. Acad. Sci. U S A 106, 7391–7396. doi: 10.1073/pnas.0900293106
Wang, X., Lou, N., Xu, Q., Tian, G. F., Peng, W. G., Han, X., et al. (2006). Astrocytic Ca2+ signaling evoked by sensory stimulation in vivo. Nat. Neurosci. 9, 816–823. doi: 10.1038/nn1703
Wang, F., Smith, N. A., Xu, Q., Fujita, T., Baba, A., Matsuda, T., et al. (2012). Astrocytes modulate neural network activity by Ca2+-dependent uptake of extracellular K+. Sci. Signal. 5:ra26. doi: 10.1126/scisignal.2002334
Wei, T., Wang, Y., Xu, W., Liu, Y., Chen, H., and Yu, Z. (2019). KCa3.1 deficiency attenuates neuroinflammation by regulating an astrocyte phenotype switch involving the PI3K/AKT/GSK3beta pathway. Neurobiol. Dis. 132:104588. doi: 10.1016/j.nbd.2019.104588
Westenbroek, R. E., Bausch, S. B., Lin, R. C., Franck, J. E., Noebels, J. L., and Catterall, W. A. (1998). Upregulation of L-type Ca2+ channels in reactive astrocytes after brain injury, hypomyelination, and ischemia. J. Neurosci. 18, 2321–2334. doi: 10.1523/JNEUROSCI.18-07-02321.1998
Winship, I. R., Plaa, N., and Murphy, T. H. (2007). Rapid astrocyte calcium signals correlate with neuronal activity and onset of the hemodynamic response in vivo. J. Neurosci. 27, 6268–6272. doi: 10.1523/JNEUROSCI.4801-06.2007
Xing, L., Yang, T., Cui, S., and Chen, G. (2019). Connexin hemichannels in astrocytes: role in CNS disorders. Front. Mol. Neurosci. 12:23. doi: 10.3389/fnmol.2019.00023
Yamashita, A., Hamada, A., Suhara, Y., Kawabe, R., Yanase, M., Kuzumaki, N., et al. (2014). Astrocytic activation in the anterior cingulate cortex is critical for sleep disorder under neuropathic pain. Synapse 68, 235–247. doi: 10.1002/syn.21733
Young, S. Z., Platel, J. C., Nielsen, J. V., Jensen, N. A., and Bordey, A. (2010). GABAA increases calcium in subventricular zone astrocyte-like cells through L- and T-type voltage-gated calcium channels. Front. Cell. Neurosci. 4:8. doi: 10.3389/fncel.2010.00008
Yu, X., Taylor, A. M. W., Nagai, J., Golshani, P., Evans, C. J., Coppola, G., et al. (2018). Reducing astrocyte calcium signaling in vivo alters striatal microcircuits and causes repetitive behavior. Neuron 99, 1170.e9–1187.e9. doi: 10.1016/j.neuron.2018.08.015
Zamora, N. N., Cheli, V. T., Santiago Gonzalez, D. A., Wan, R., and Paez, P. M. (2020). Deletion of voltage-gated calcium channels in astrocytes during demyelination reduces brain inflammation and promotes myelin regeneration in mice. J. Neurosci. 40, 3332–3347. doi: 10.1523/JNEUROSCI.1644-19.2020
Zhang, Y. V., Ormerod, K. G., and Littleton, J. T. (2017). Astrocyte Ca2+ influx negatively regulates neuronal activity. eNeuro 4:ENEURO.0340-16.2017. doi: 10.1523/ENEURO.0340-16.2017
Zhang, S. L., Yu, Y., Roos, J., Kozak, J. A., Deerinck, T. J., Ellisman, M. H., et al. (2005). STIM1 is a Ca2+ sensor that activates CRAC channels and migrates from the Ca2+ store to the plasma membrane. Nature 437, 902–905. doi: 10.1038/nature04147
Zhu, J., Wei, X., Liu, J., Hu, Y., and Xu, J. (2009). Interaction of glia activation and neurotransmission in hippocampus of neuropathic rats treated with mirtazapine. Exp. Clin. Psychopharmacol. 17, 198–203. doi: 10.1037/a0016033
Zhuang, Z. Y., Wen, Y. R., Zhang, D. R., Borsello, T., Bonny, C., Strichartz, G. R., et al. (2006). A peptide c-Jun N-terminal kinase (JNK) inhibitor blocks mechanical allodynia after spinal nerve ligation: respective roles of JNK activation in primary sensory neurons and spinal astrocytes for neuropathic pain development and maintenance. J. Neurosci. 26, 3551–3560. doi: 10.1523/JNEUROSCI.5290-05.2006
Keywords: astrocyte, calcium, calcium channels, pain pathway, chronic pain, neuropathic pain, inflammatory pain
Citation: Cho J and Huh Y (2020) Astrocytic Calcium Dynamics Along the Pain Pathway. Front. Cell. Neurosci. 14:594216. doi: 10.3389/fncel.2020.594216
Received: 12 August 2020; Accepted: 14 September 2020;
Published: 16 October 2020.
Edited by:
Divya P. Kumar, JSS Academy of Higher Education and Research, IndiaReviewed by:
Alessandro Michelucci, Luxembourg Institute of Health, LuxembourgKyung Seok Han, Dongguk University Gyeongju, South Korea
Copyright © 2020 Cho and Huh. This is an open-access article distributed under the terms of the Creative Commons Attribution License (CC BY). The use, distribution or reproduction in other forums is permitted, provided the original author(s) and the copyright owner(s) are credited and that the original publication in this journal is cited, in accordance with accepted academic practice. No use, distribution or reproduction is permitted which does not comply with these terms.
*Correspondence: Yeowool Huh, aHVoMDZAY2t1LmFjLmty; Jeiwon Cho, amVsZWN0cm8yMUBld2hhLmFjLmty