- Department of Ophthalmology, Visual and Anatomical Sciences, Wayne State University School of Medicine, Detroit, MI, United States
The retina and the olfactory bulb are the gateways to the visual and olfactory systems, respectively, similarly using neural networks to initiate sensory signal processing. Sensory receptors receive signals that are transmitted to neural networks before projecting to primary cortices. These networks filter sensory signals based on their unique features and adjust their sensitivities by gain control systems. Interestingly, dopamine modulates sensory signal transduction in both systems. In the retina, dopamine adjusts the retinal network for daylight conditions (“light adaptation”). In the olfactory system, dopamine mediates lateral inhibition between the glomeruli, resulting in odorant signal decorrelation and discrimination. While dopamine is essential for signal discrimination in the olfactory system, it is not understood whether dopamine has similar roles in visual signal processing in the retina. To elucidate dopaminergic effects on visual processing, we conducted patch-clamp recording from second-order retinal bipolar cells, which exhibit multiple types that can convey different temporal features of light. We recorded excitatory postsynaptic potentials (EPSPs) evoked by various frequencies of sinusoidal light in the absence and presence of a dopamine receptor 1 (D1R) agonist or antagonist. Application of a D1R agonist, SKF-38393, shifted the peak temporal responses toward higher frequencies in a subset of bipolar cells. In contrast, a D1R antagonist, SCH-23390, reversed the effects of SKF on these types of bipolar cells. To examine the mechanism of dopaminergic modulation, we recorded voltage-gated currents, hyperpolarization-activated cyclic nucleotide-gated (HCN) channels, and low-voltage activated (LVA) Ca2+ channels. SKF modulated HCN and LVA currents, suggesting that these channels are the target of D1R signaling to modulate visual signaling in these bipolar cells. Taken together, we found that dopamine modulates the temporal tuning of a subset of retinal bipolar cells. Consequently, we determined that dopamine plays a role in visual signal processing, which is similar to its role in signal decorrelation in the olfactory bulb.
Introduction
Continuous integration of our sensory perceptions gives rise to our daily experience of the world, and this experience is made possible by specialized neuronal “antennae,” such as the retina and the olfactory bulb. Interestingly, the retina and the olfactory bulb utilize similar neural network architecture despite processing different signals. In both systems, sensory signals stimulate sensory receptor neurons, which facilitate information transfer through specific networks formed by interneurons and then project to the cerebral cortex by output neurons.
In the retina, rod and cone photoreceptors transduce light inputs into electrochemical signals. Second-order neurons, bipolar cells, converge photoreceptor input, and begin the process of extracting abstract visual features such as luminance, contrast, chromaticity, and spatiotemporal properties of light signals. This information is modulated by horizontal and amacrine cells and relayed to third-order retinal ganglion cells (RGCs), the output neurons of the retina (Wässle, 2004; Dowling, 2012). Similarly, in the olfactory system, olfactory receptor neurons (ORNs) in the nasal cavity convey odorant signals to the glomeruli structures in the olfactory bulb, where signals are modulated by juxtaglomerular cells before being sent out to the olfactory cortex by the olfactory output neurons, mitral and tufted cells (Astic et al., 1987; Stewart and Pedersen, 1987; Ressler et al., 1994; Gire et al., 2012).
Besides similarities in circuitry, the visual and olfactory processing systems also comparably use dopamine as a principal neuromodulator. Five types of dopamine receptors have been identified and are classified as D1-like (D1 and D5 receptors) and D2-like (D2, D3, and D4 receptors) receptors. D1-like receptor signaling stimulates a protein kinase A (PKA) pathway in which cAMP is increased, while D2-like receptor signaling decreases cAMP levels (Witkovsky, 2004; Iuphar, 2020). In the olfactory bulb, dopamine facilitates a gain control system, which presynaptically suppresses ORN transmission to the glomeruli, and also decorrelates the signals between glomeruli (Wachowiak and Cohen, 1999; Banerjee et al., 2015; Vaaga et al., 2017). Consequently, dopamine neuromodulation mediates odor signal discrimination, as shown by behavioral studies (Kruzich and Grandy, 2004; Tillerson et al., 2006; Wei et al., 2006).
In the retina, dopamine is released by dopaminergic amacrine cells (DACs) in response to light or circadian time (Kramer, 1971; Iuvone et al., 1978; Pourcho, 1982; Mariani and Hokoc, 1988; Kirsch and Wagner, 1989; Witkovsky et al., 1993; Weiler et al., 1997; Megaw et al., 2006). Retinal dopamine enables the retina to adapt from dark to daytime light conditions and has accordingly been shown to lower the light sensitivity across photoreceptor, horizontal cell, and RGC populations as light levels increase (Jensen and Daw, 1986; Vaquero et al., 2001; Hayashida and Ishida, 2004; Hayashida et al., 2009; Blasic et al., 2012; Ogata et al., 2012; Liu et al., 2016b; Nikolaeva et al., 2019). Furthermore, retinal dopamine uncouples horizontal cell and amacrine cell gap junctions with light adaptation, narrowing the receptive field size of downstream neurons (McMahon and Mattson, 1996; He et al., 2000). However, whether dopamine contributes to visual signal decorrelation—much like in the olfactory system—remains unclear.
Previously, our lab has shown that subsets of retinal bipolar cells express the dopamine receptor D1 (D1Rs) in a type-dependent manner (Farshi et al., 2016). Bipolar cells are known to perform parallel processing in the visual system (Wässle, 2004), where each type conveys distinct aspects of image features (Borghuis et al., 2013; Euler et al., 2014; Ichinose et al., 2014; Ichinose and Hellmer, 2016). Therefore, we examined whether dopamine signaling differentially modulated visual signaling in each type of bipolar cell. As the retina and olfactory bulb have extensive similarities in their signal processing, including the general role of dopamine in neuromodulation and sensory discrimination, a deeper understanding of dopamine’s function in the retina may translate to more significant insights into olfactory processing and vice versa.
Materials and Methods
Ethical Approval
All animal procedures were approved by the Institutional Animal Care and Use Committee at Wayne State University (protocol no. A05-03-15). All the necessary steps were taken to minimize animal suffering. The tissues were harvested immediately after the animal was euthanized by CO2 inhalation and cervical dislocation.
Retinal Preparation
The experimental techniques were similar to previously described (Ichinose and Lukasiewicz, 2012; Ichinose et al., 2014). Briefly, the mice (4–12 weeks old; male or female, C57BL/6J strain; Jackson Laboratory, Bar Harbor, ME, USA or Kcng4-cre strain; a gift from Dr. Sanes, Harvard University, Cambridge, MA, USA; Duan et al., 2014) were dark-adapted overnight, euthanized, and eyes were enucleated. Using a stereo microscope, the retina was isolated and cut into slice preparations (250 μm thick). Some ganglion cell recordings were conducted using the wholemount retinal preparations. Only the dorsal retina was used for recordings. All procedures were performed in dark-adapted conditions under infrared illumination using infrared viewers. The dissecting medium was cooled and continuously oxygenated. Retinal preparations were stored in an oxygenated dark box at room temperature.
Whole-Cell Recordings
Whole-cell patch-clamp recordings were made from the bipolar cell or ganglion cell somas in the retinal preparations by viewing them with an upright microscope (Slicescope Pro 2000, Scientifica, UK) equipped with a CCD camera (Retiga-2000R, Q-Imaging, Canada). The light-evoked postsynaptic potentials and currents (L-EPSPs and L-EPSCs) were recorded at the resting membrane potential and the equilibrium potential for chloride ions (ECl; −60 mV), respectively. All recordings were performed at 30–34°C. The liquid junction potentials were corrected after each recording. Whole-cell recordings from bipolar cells usually lasted 20–30 min without significant rundown (Ichinose et al., 2014). The electrodes were pulled from borosilicate glass (1B150F-4; WPI, FL, USA) with a P1000 Puller (Sutter Instruments, Novato, CA, USA) and had resistances of 8–12 MΩ. Clampex and MultiClamp 700B (Molecular Devices, San Jose, CA, USA) were used to generate the waveforms, acquire the data, and control light stimuli by a light-emitting diode (LED; Cool LED, UK). The data were digitized and stored on a personal computer using Axon Digidata 1440A (Molecular Devices). The responses were filtered at 1 kHz with the four-pole Bessel filter on the MultiClamp 700B and sampled at 2–5 kHz.
Solutions and Drugs
The retinal dissections were performed in HEPES-buffered extracellular solution containing the following (in mM):115 NaCl, 2.5 KCl, 2.5 CaCl2, 1.0 MgCl2, 10 HEPES, and 28 glucose, adjusted to pH 7.4 with NaOH. Physiological recordings were performed in Ames’ medium buffered with NaHCO3 (Millipore–Sigma, St. Louis, MO, USA) and bubbled with 95% O2 and 5% CO2; the pH was 7.4 at 30–33°C. The intracellular solution contained the following (in mM):111 potassium gluconate, 1.0 CaCl2, 10 HEPES, 1.1 EGTA, 10 NaCl, 1.0 MgCl2, 5 ATP-Mg, and 1.0 GTP-Na, adjusted to pH 7.2 with KOH. The potassium gluconate was replaced with cesium gluconate for the recording in voltage-clamp mode. A cocktail of inhibitory receptor antagonists, including a glycine receptor antagonist, strychnine (1 μM, Sigma), a GABAA receptor antagonist, (−)-bicuculline methobromide (50 μM; Axxora, NY, USA), and a GABAC receptor antagonist, (1,2,5,6-tetrahydropyridin-4-yl) methylphosphinic acid hydrate (TPMPA; 50 μm; Bio-Techne Company, Devens, MN, USA), were bath applied throughout all recordings to suppress the network effect. For pharmacological experiments either SKF-38393, a D1R agonist (10 μM; Bio-Techne Company, Devens, MA, USA), or SCH-23390, a D1R antagonist (10 μM; Bio-Techne Company, Devens, MA, USA), were bath applied. SKF-38393 was applied alone after control recordings, and then SCH-23390 was subsequently applied alone during the washout of SKF-38393.
Light Stimulation
Green light (500 nm) was projected through a 60× objective lens onto the photoreceptors in the vicinity of the recorded bipolar cells with a spot diameter of 100 μm, which is slightly larger than the size of the receptive field center for a bipolar cell (Berntson and Taylor, 2000). The preparations were adapted to a background light at the rod-saturated level, 4.35 × 104 photons/μm2/s for a minimum of 10 min before recording. The same average luminance was used for subsequent sinewave stimuli. A series of sinewave stimuli discloses the temporal features of a neuron (Figures 1A,D). However, this stimulus requires a long recording time, which hampers stable pharmacological experiments. Therefore, two other equivalent light stimuli were compared: chirp and sum-of-sines (a series of stimulus takes 90 s for individual sines, 30 s for chirp, and 20 s for sum-of-sines). The chirp and sum-of-sines stimuli evoked generally similar light responses compared to the original stimulus, but the sum-of-sines better replicated the results, especially at low frequencies (Figures 1B–D). A representative bipolar cell sum-of-sines recording and resulting power spectrum are shown (Figures 1E,F).
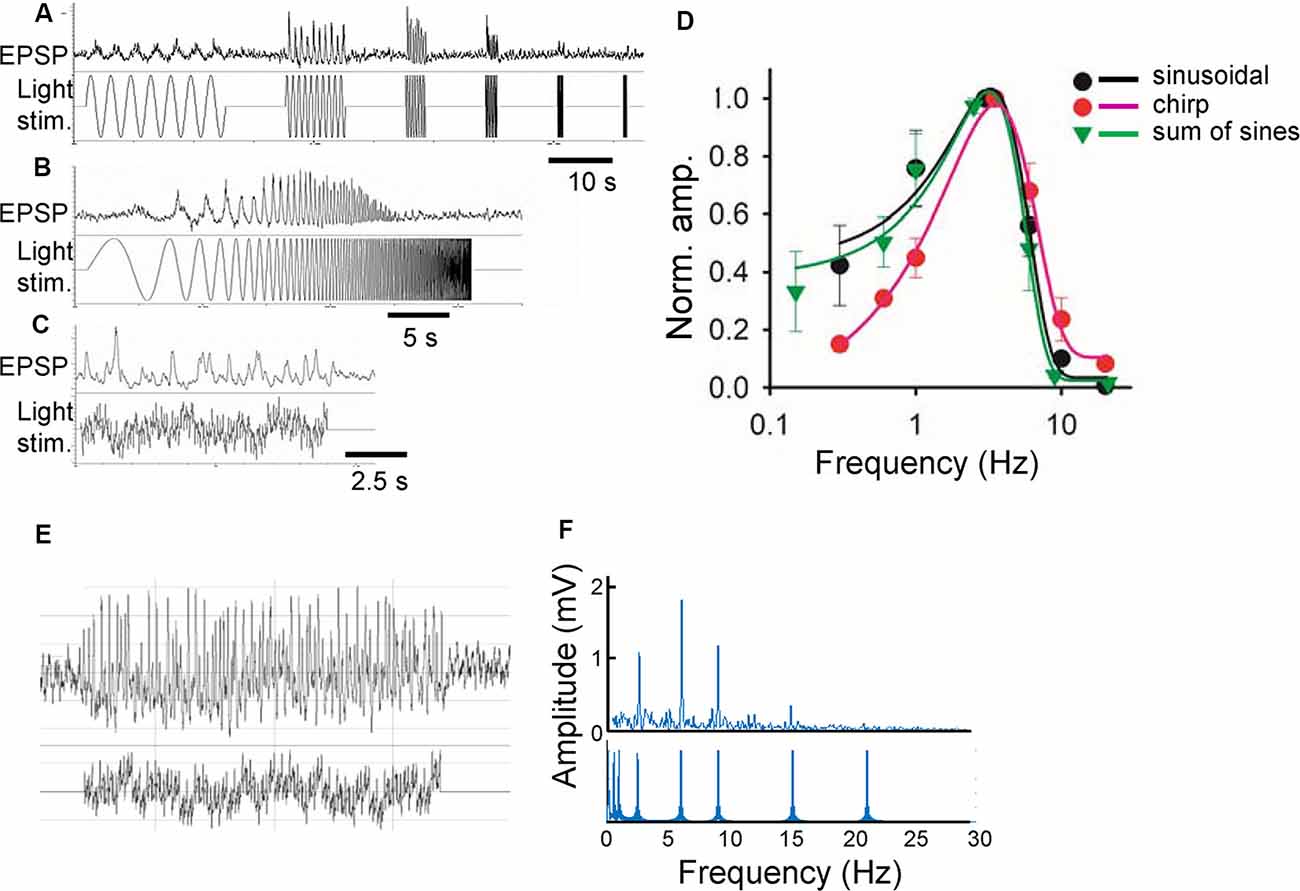
Figure 1. Comparison of three stimulus functions. (A) Sinusoidal light stimuli from 0.3 to 20 Hz (lower) and a representative response (light-evoked postsynaptic potentials; L-EPSP; upper). (B) Chirp light stimuli and L-EPSP with the same frequencies from the same cell. (C) Sum of sinusoidal light stimuli of similar frequencies and L-EPSP. (D) Comparison of L-EPSPs evoked by three functions (n = 3 ganglion layer cells). (E) A representative L-EPSP from bipolar cells (upper) evoked by a sum of sinusoidal light stimulation (lower). (F) An fast fourier transformation (FFT) analysis of the traces (E) revealed the different frequencies of light stimuli (lower) and the amplitude of L-EPSP for each frequency.
Voltage-Gated Channel Recording
Hyperpolarization-activated cyclic nucleotide-gated (HCN) channels and low-voltage activated (LVA) Ca2+ channels were recorded by voltage-clamp mode. HCN currents were activated by hyperpolarization (–60 to –130 mV) for 1 s followed by holding the potential at –70 mV (Figure 5A; Cangiano et al., 2007; Hellmer et al., 2016). Voltage-gated Ca2+ channels were evoked by a ramp voltage change from –90 to +44 mV at a speed of 134 mV/s (Hu et al., 2009). HCN currents were isolated based on our previous pharmacological experiments (Hellmer et al., 2016). LVA currents were also isolated by including potassium channel blockers (Cs and TEA) in the pipette solution (Hu et al., 2009).
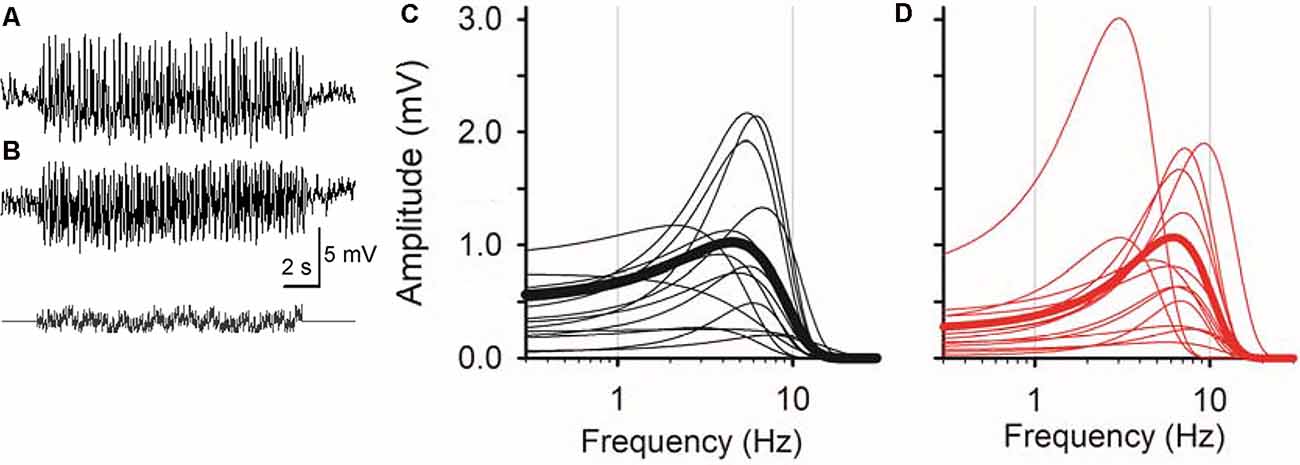
Figure 2. Sinusoidal L-EPSPs were changed by dopamine receptor 1 (D1R) signaling in bipolar cells. (A) A representative L-EPSP recorded from an XBC cell in response to a sum-of-sines light stimulus (bottom). (B) L-EPSPs in the presence of 10 μM SKF38393. (C) Bipolar cell temporal responses were fit to the exponential curves. Each curve exhibits a frequency-response relation for each bipolar cell (black) and the average population response (thick black) in the control solution. (D) The temporal responses for each bipolar cell (red) and the average response (thick red) in the presence of 10 μM SKF38393. The peak frequency response shifted to the right in some bipolar cells, indicating that D1R signaling increased the sensitivity to higher frequencies.
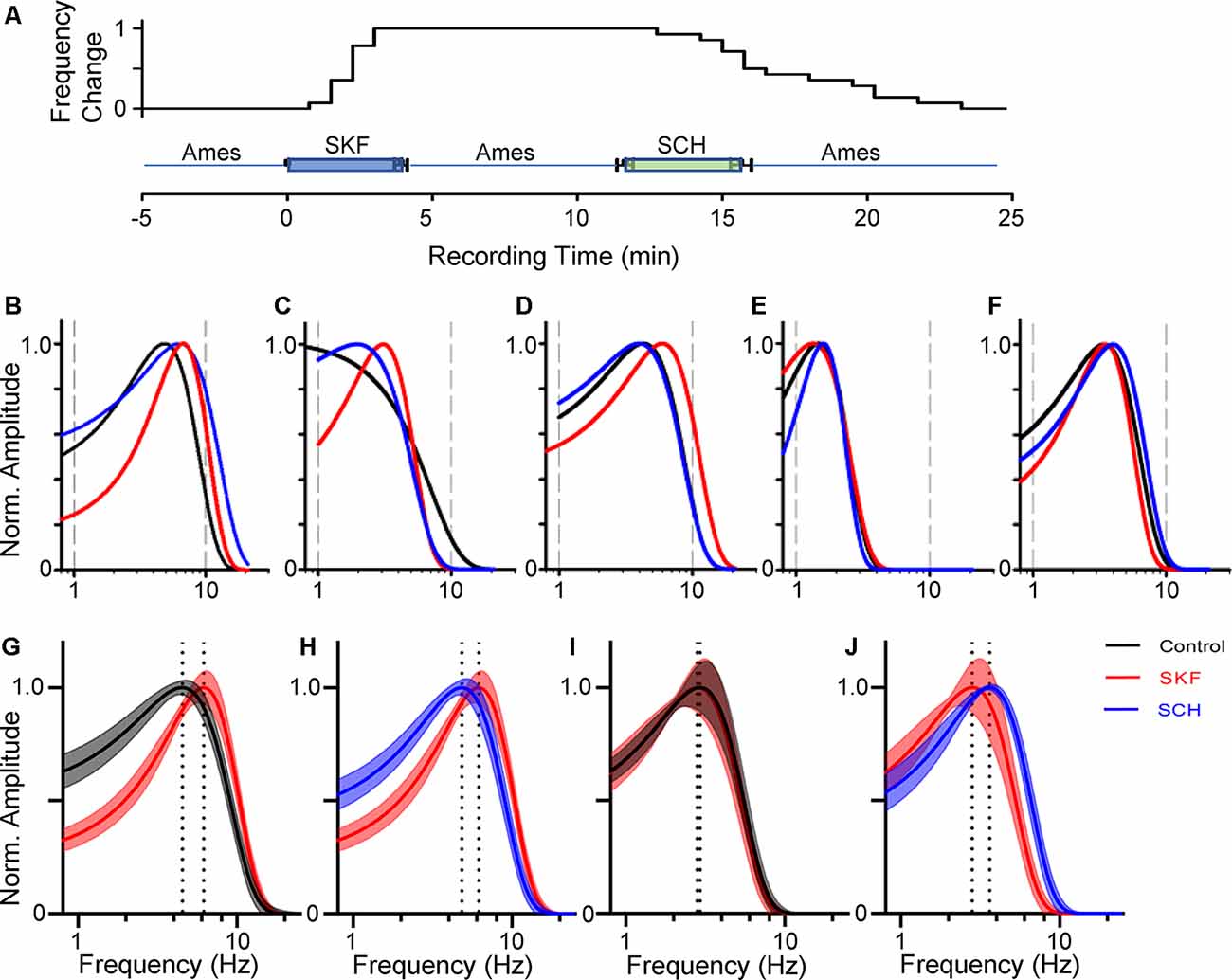
Figure 3. Dopamine receptor 1 (D1R) signaling increased the peak temporal tuning in some bipolar cells. (A) The time course of SKF-SCH applications and the normalized peak frequency change. The peak frequency is indicated 0 at the control level, and 1 when shifted to a higher frequency. The plot shows the individual response from the 14 bipolar cells. (B–D) The frequency-response curves for a transient OFF (B), sustained OFF (C), and transient ON (D) SKF sensitive bipolar cell in control (black), SKF (red), and SCH (blue). SKF showed an increase in the peak temporal frequency for all three of the cells. Subsequent SCH application reduced the peak temporal frequency. (E,F) The frequency response curves for an OFF (E) and ON SKF insensitive bipolar cell in control (black), SKF (red), and SCH (blue) conditions respectively. SKF or SCH application did not change the temporal tuning for these two cells. (G) The average frequency-response curves from 14 bipolar cells (including ON and OFF cells) in control (black, average ± SEM), and in the presence of SKF (red). The peak frequencies are indicated by dotted lines. SKF shifted the peak to the right, indicating that D1R signaling increased the temporal tuning for these cells towards higher frequencies. (H) The frequency-response curves from the same set of cells in the presence of SKF (red) and subsequent SCH (blue) application. SCH shifted the curve back to the control level. (I) The average frequency-response curves from six bipolar cells in control (black) and SKF (red) solutions. SKF did not change the curve for these bipolar cells. (J) The curves from the same set of bipolar cells in SKF (red) and SCH (blue) solutions.
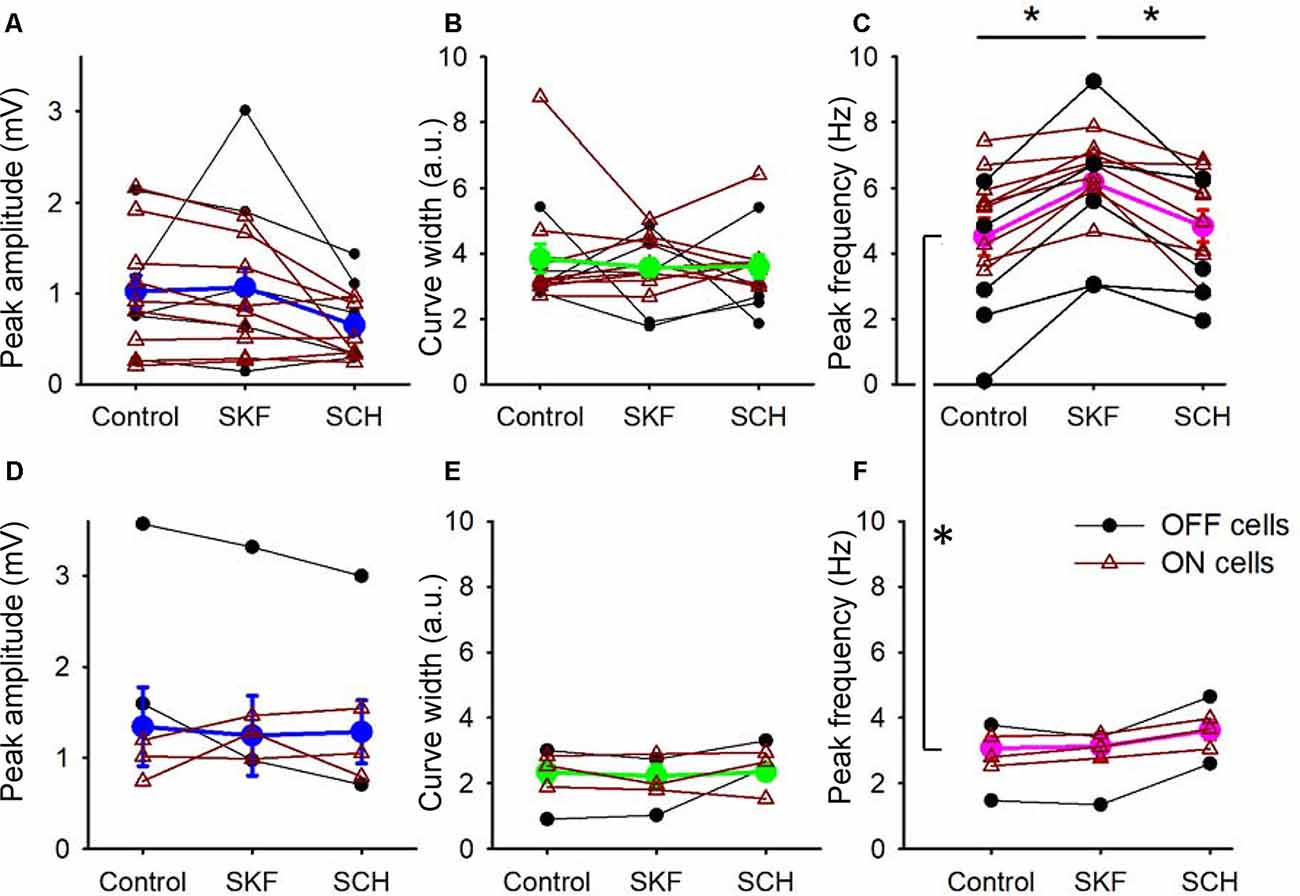
Figure 4. D1R signaling modulated the peak frequency, but not for the peak amplitude and the tuning curve width. (A) The peak amplitude of the frequency-response curves for the SKF-sensitive bipolar cells. Each dot-line represents one cell, and the average is shown in thick blue. ON and OFF cells are color-coded in brown and black, respectively. Both SKF and SCH did not change the peak amplitude. (B) The tuning curve width of the frequency response curves for the SKF-sensitive bipolar cells. Individual cells (brown and black) and the average (green). Neither SKF nor SCH changed the average tuning curve widths. (C) The peak frequency of the frequency-response curves for the SKF-sensitive bipolar cells. Individual cells are shown in brown and black, and the average is shown in magenta. SKF significantly increased the peak frequency (p < 0.01), which was reversed by SCH application (p < 0.01). (D–F) The same set of parameters for SKF-insensitive bipolar cells. The D1R agonist and antagonist changed none of the three parameters. (C,F) The peak frequencies in control solutions for the SKF-sensitive and -non-responsive bipolar cells are significantly different (p < 0.01, *p < 0.05).
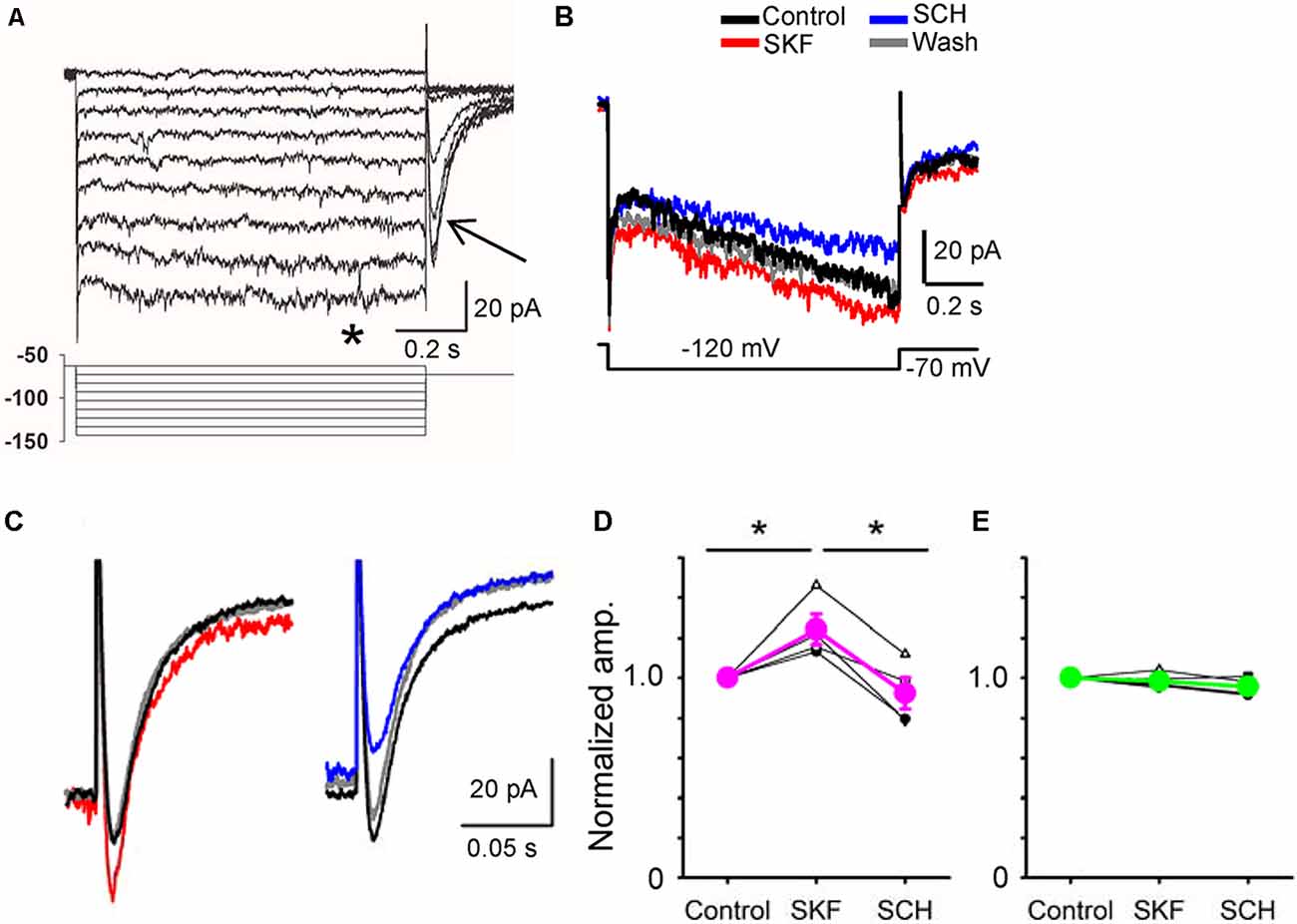
Figure 5. A D1R agonist and an antagonist modulated hyperpolarization-activated cyclic nucleotide-gated (HCN) currents in a bipolar cell. (A) Voltage steps (lower panel) evoked inward currents in a type 5-2 bipolar cell. Steady-state currents (*) and tail currents (arrow). (B) HCN currents were evoked in a type 5-2 bipolar cell. SKF increased the HCN steady-state current, whereas SCH decreased the current. (C) The tail current was also increased by SKF38393 (red). SCH23390 decreased the tail current (blue) in the same cell. (D) A summary graph shows that SKF increased the HCN tail current in four bipolar cells (p < 0.05), and SCH decreased the current (p < 0.01, *p < 0.05.). (E) In five bipolar cells, SKF and SCH did not change the HCN tail current (p > 0.1).
Morphological Identification
A fluorescent dye, sulforhodamine B (0.005%, Sigma), and Neurobiotin (0.5%, Vector Lab, Burlingame, CA, USA) were included in the patch-clamp pipette. Immediately after electrophysiological recordings, sulforhodamine B images were captured using the CCD camera. For Neurobiotin visualization, the slice preparation was fixed with 4% paraformaldehyde for 30 min, incubated with streptavidin-conjugated Alexa 488 (1:200, Thermo Fisher Scientific, Waltham, MA, USA) and an anti-choline acetyltransferase (ChAT) antibody (1:200, AB144P, Millipore, Danvers, MA, USA) overnight, and then incubated with the secondary antibody for 2 h at room temperature. The preparation was viewed with a confocal microscope (TCS SP8, Leica, Germany). We determined bipolar cell types according to previous descriptions (Ghosh et al., 2004; Ichinose et al., 2014; Ichinose and Hellmer, 2016).
Data Analysis and Statistics
For sinusoidal responses, MatLab (MathWorks, MA, USA) and pClamp were used to measure amplitude (in mV) by Fast Fourier Transformation (FFT) analysis. Fundamental and multiple harmonics amplitudes were added to achieve accurate amplitude measurements. After FFT analysis, the frequency-responses were fitted with curves using the equation:
Where a = peak amplitude, b = tuning curve width, x0 = frequency at the peak. Hereafter, we refer to x0 as the peak frequency or the frequency where cells responded with the largest response amplitude. Correspondingly, the peak amplitude indicates the amplitude response at the peak frequency. Finally, tuning curve width indicates the range of frequencies over which the cell responds, and is equivalent to the full width at half-maximum (FWHM) variable reported in other literature.
For HCN recordings, the tail current amplitude was analyzed to decrease contamination from other currents (Horwitz et al., 2011; He et al., 2014). The values are presented as the mean ± SEM. Voltage-gated currents and L-EPSCs were normalized to the control level because of large variations of individual currents between cells. A repeated-measures ANOVA was conducted to compare the response in control, SKF, and SCH solutions (Prism v.8, GraphPad Software, CA, USA). The ANOVA was run with a Geisser-Greenhouse correction to account for possible violations of the assumption of circularity/sphericity, followed by a Tukey’s multiple comparisons test to obtain the adjusted p-values. A paired t-test was conducted to compare the voltage-gated currents in control and SKF solutions. The differences were considered significant if p < 0.05.
Results
D1R Signaling Modulates Temporal Features in a Subset of Bipolar Cells
Whole-cell recordings were conducted from bipolar cells using retinal slices prepared in the dark under infrared illumination. After adapting the preparations at a mesopic light level for more than 10 min, light-evoked excitatory postsynaptic potentials (L-EPSPs) were recorded in response to the sum-of-sines stimuli in the presence of inhibitory receptor blockers (see “Materials and Methods” section; Figure 2A). L-EPSPs for individual cells were analyzed by FFT (Figure 1), which revealed the frequencies of sinewave stimuli and L-EPSP amplitudes for those frequencies. The response amplitude was plotted as a function of frequency and fitted by an exponential equation (see “Materials and Methods” section) for each cell. The frequency-response curves from 14 bipolar cells, including representative recordings from types 3, 4, 5, XBC, and 6, are plotted in Figure 2C (black lines). The peak amplitude, tuning curve width, and peak frequency were diverse among cells because multiple types of bipolar cells exhibit distinct temporal features (Ichinose et al., 2014; Ichinose and Hellmer, 2016).
Previously, we found that the dopamine receptor 1 (D1R) is expressed by bipolar cells in a type-dependent manner (Farshi et al., 2016). D1Rs are expressed by types 1, 3b, 4, 5-2, XBC, 6, and 7 bipolar cells, whereas types 2, 3a, 5-1, and 9 did not possess the receptor. While morphological evidence has suggested that dopaminergic signaling plays a distinct role in visual processing in each type of bipolar cell, physiological investigations have not been performed. We examined the dopaminergic effect on the temporal features in bipolar cells by the application of a D1R agonist, SKF38393 (10 μM; Figure 2B). In some bipolar cells, SKF shifted the peak frequency to higher frequencies (Figures 2C,D, compare average traces shown by thick black and red lines, 14/20 cells), suggesting that D1R signaling modulated the temporal tuning.
We have examined the dopaminergic effect on temporal features in both ON and OFF bipolar cells. We recorded L-EPSPs in the control solution, applied SKF38393 (10 μM), for 4 min, washed-out approximately for 8 min, and then applied an antagonist, SCH23390 (10 μM; Figure 3A). In 14 ON and OFF bipolar cells, the peak frequencies shifted toward higher frequencies by SKF (Figures 3B–D,G), and the subsequent application of SCH moved the peak frequency back to the control level (Figures 3B–D,H). The time course of SKF-SCH applications and the responses from 14 bipolar cells (Figure 3A) shows that the peak frequencies changed during the SKF application (at 2.1 ± 0.2 min) and changed back to the control level after the SCH application (at 15.2 ± 0.8 min), indicating that D1R signaling modulated the temporal responses. Furthermore, we observed that SCH-only application shifted the peak frequency to lower frequencies (n = 2), or no change (n = 2), suggesting that some ambient dopamine may already be present in our light-adapted condition. In the other six bipolar cells, the peak frequency responses were not shifted by SKF (Figures 3D,E,H,I); therefore, we categorized them as SKF-insensitive cells. The former group included type 3 (n = 4), type 4 (N = 1), type 5 (n = 7), and type 6 (n = 2), whereas the latter contained type 2 (n = 1), type 3 (n = 1), type 5 (n = 3), and type 6 (n = 1). Although the number of recordings from individual types was low and no further type-specific morphological features (such as type 3a and 3b) were determined in this study, the observed cell types were consistent with our previous findings of D1R-expressing and non-expressing bipolar cells (Farshi et al., 2016).
We compared the parameters of frequency-response curves between SKF-sensitive and insensitive bipolar cells by repeated measures ANOVA (see “Materials and Methods” section). The peak amplitude, tuning curve width, and the peak frequency of SKF-sensitive bipolar cells were plotted (n = 14; Figures 4A–C). The former two factors were not affected by SKF, nor SCH. However, the peak frequency was shifted to higher frequencies by SKF (p < 0.01) and returned to the control level after SCH (p < 0.01; Figure 4C). For the SKF-insensitive bipolar cells, none of these parameters were modulated by SKF, nor by SCH (p > 0.1 for all combinations; Figures 4D–F). We also compared the peak frequency for SKF-sensitive ON and OFF bipolar cells separately (n = 9 for ON, n = 5 for OFF); SKF shifted the peak frequency to a higher frequency (p < 0.01 both for ON and OFF) and SCH decreased it (p = 0.05 for OFF, p < 0.01 for ON). Furthermore, in control conditions, the peak frequency of SKF-sensitive cells was higher than for the SKF insensitive cells (p < 0.01; Figures 4C,F). One possible explanation for this is that due to slicing, these cells may have been damaged in some way and therefore respond slower as a result; however, we note that the mean peak frequency of SKF-insensitive cells (2.8 ± 0.3 Hz; Figure 4F, control) are similar to those found in previous slice studies (Burkhardt et al., 2007; Ichinose and Hellmer, 2016). These results indicated that D1R signaling enables a subset of bipolar cells to respond to higher frequency stimuli during light-adapted conditions.
Voltage-Gated Channels in Bipolar Cells Are Targets for Dopaminergic Modulation
The temporal properties of bipolar cells are shaped by multiple factors, including ligand or voltage-gated channel diversity, the mGluR6 complex, and amacrine cells (DeVries, 2000; Ma et al., 2003; Müller et al., 2003; Cao et al., 2012; Baden et al., 2013; Puthussery et al., 2013; Lindstrom et al., 2014; Ray et al., 2014; Franke et al., 2017). Among these factors, voltage-gated channels, such as HCN and voltage-gated Ca2+ channels, are also known-targets of dopamine (Pfeiffer-Linn and Lasater, 1993; Surmeier et al., 1995; Fan and Yazulla, 2001; Robinson and Siegelbaum, 2003; Hayashida and Ishida, 2004). Therefore, we investigated whether D1R signaling modulated hyperpolarization-activated cyclic nucleotide-gated (HCN) channels and low voltage-activated (LVA) Ca2+ channels in D1R-expressing bipolar cells.
Whole-cell recordings were conducted from bipolar cells, and HCN currents were evoked in response to a series of step pulses (Figure 5A; Cangiano et al., 2007; Hellmer et al., 2016). Steady-state and tail currents were recorded. After steady recordings were obtained, we applied SKF, followed by SCH in the bath solution. In four bipolar cells, including type 1 (n = 1), type 3 (n = 1), and type 5 (n = 2), SKF increased the tail current, and SCH reduced the current (Figures 5B–D, P < 0.05). In contrast, SKF and SCH did not affect the tail current in five other bipolar cells, including type 2 (n = 1), type 4 (n = 1), type 5 (n = 1), type 8 (n = 1), and the rod bipolar cell (n = 1). The SKF-sensitive bipolar cell types likely correspond with the types of bipolar cells that exhibited D1Rs (Farshi et al., 2016). These results suggest that D1R-signaling increased the peak temporal tuning in a subset of bipolar cells by increasing HCN currents.
Furthermore, we examined the effect of D1R signaling on LVA Ca2+ channels in bipolar cells. The LVA current was evoked by a ramp voltage stimulation (Figure 6). SKF reduced the LVA currents (Figure 6A) in a subset of bipolar cells. The SKF-sensitive cells were type 4 (n = 1), type 5 (n = 3), and type 6 (n = 2), whereas SKF insensitive cells were type 5 (n = 1), and rod bipolar cells (n = 3; Figures 6B,C). These types were consistent with the previous morphological analysis (Farshi et al., 2016). These results suggest that D1R-signaling modulates LVA currents, which may modulate temporal tuning in a subset of bipolar cells.
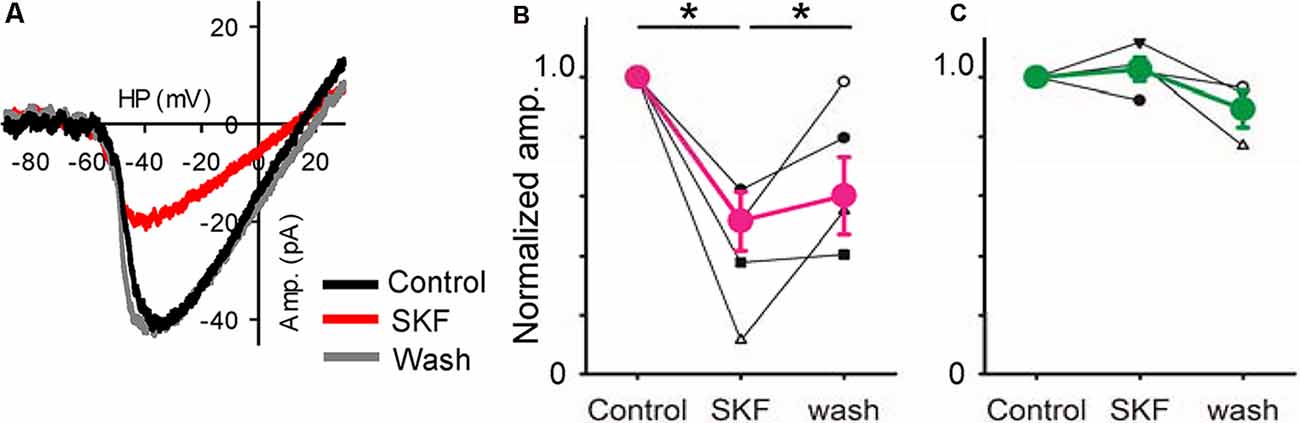
Figure 6. A D1R agonist modulated low-voltage activated (LVA) Ca2+ currents. (A) A voltage ramp evoked LVA Ca2+ currents in an XBC. SKF reduced it, an effect that recovered after SKF was washed from the solution clause; HP = holding potential. (B) Normalized amplitudes from six bipolar cells (3 type 5, 1 type 4, and 2 type 6) in response to SKF. SKF reduced the current (p < 0.01, *p < 0.05.). (C) Normalized amplitudes from four bipolar cells (1 type 5, and 3 rod-bipolar cells). SKF did not change the currents.
Discussion
Using retinal slice preparations from the mouse, we examined the effect of a D1R agonist and antagonist on the temporal features of bipolar cell signaling. In a subset of ON and OFF bipolar cells, the D1R agonist SKF38393 increased sensitivity to higher frequency responses, which was reversed by the application of a D1R antagonist, SCH23390. SKF38393 also increased HCN and decreased LVA Ca2+ currents in a subset of bipolar cells, suggesting that voltage-gated channels may be the underlying mechanism involved in D1R activation. The types of SKF-sensitive and SKF insensitive bipolar cells were consistent with those we previously identified as D1R-expressing and D1R-lacking bipolar cells. As the retina and olfactory bulb signal processing structures are similar, we incorporated our findings to examine similarities between retinal and olfactory dopamine neuromodulation.
Dopamine and Signal Decorrelation in the Olfactory System
The mouse olfactory system is capable of discerning more than 1012 odors, resulting from over 1,000 unique odorant receptors expressed by ORNs in the olfactory epithelium (Zhang and Firestein, 2002; Bushdid et al., 2014). ORN receptors are sensitive to odorant size, shape, charge, and odorant molecule functional groups; therefore, a single odorant will activate a unique combination of odorant receptors that requires decoding by higher-order neurons (Malnic et al., 1999). Each population of unique ORNs project to distinct glomeruli in the mouse olfactory bulb, where nearly 1,800 glomeruli operate in parallel (Ressler et al., 1994; Mombaerts et al., 1996).
Within a single glomerulus, odorant information output is controlled primarily by mitral cells which balance excitation from glutamatergic ORNs and external tufted cells (Tatti et al., 2014) as well as inhibition from GABAergic periglomerular cells (PGs). To decode information from multiple odorants that may have overlapping receptor activation, glomeruli utilize lateral inhibition through another subset of GABAergic juxtaglomerular cells, the superficial short axon (sSA) cells. sSAs are excited by input within a strongly activated glomerulus and inhibit the output of other, more weakly activated glomeruli by using a combination of dopamine and GABAergic mechanisms (Aungst et al., 2003; Parrish-Aungst et al., 2007). The balance of excitation and inhibition both within and between glomeruli gates which information is sent to higher cortical areas.
Dopamine plays a critical role in odorant discrimination by contributing to the lateral inhibition between glomeruli. First, dopaminergic sSAs within a single glomerulus mediate lateral inhibition to other less-activated glomeruli in part by activating inhibitory D2Rs on the neighboring ORN axon terminals, reducing afferent input (Hsia et al., 1999; Ennis et al., 2001; Vaaga et al., 2017). Dopamine also indirectly inhibits mitral cell output by activating excitatory D1Rs in external tufted cells; external tufted cells release glutamate onto PG neurons which then locally inhibit mitral cell output (Liu et al., 2013, 2016a). Besides sSAs, dopaminergic subsets of PGs are thought to contribute to local inhibition of ORN afferent inputs, but this effect has yet to be shown directly (Maher and Westbrook, 2008). Ultimately, olfactory dopamine modulation serves to decorrelate odorant information by enhancing odorant dissimilarities and allowing the animal to discriminate multiple different odors in its environment (Wei et al., 2006; Escanilla et al., 2009; Banerjee et al., 2015).
Dopamine and Retinal Visual Processing
In the retina, dopamine release is evoked by light stimulation from tyrosine hydroxylase (TH)-positive amacrine cells (DACs), and is thought to facilitate the transition from dim to bright ambient light conditions, such as in dawn to early morning (Krizaj, 2000; Witkovsky, 2004; Zhang et al., 2007). Therefore, dopamine is thought to contribute to light adaptation and circadian rhythm. Dopamine receptors are expressed throughout the retina; D1 receptors are expressed by a broad range of retinal network neurons, whereas D2-like receptors are present in photoreceptors and DACs (Cohen et al., 1992; Harsanyi and Mangel, 1992; Veruki and Wässle, 1996; Derouiche and Asan, 1999; Mora-Ferrer et al., 1999; Stella and Thoreson, 2000; Witkovsky, 2004). Dopamine release has a broad range of effects on retinal neurons.
Rod and cone photoreceptors have high and low light sensitivities, respectively, covering all ranges of light conditions from night to daylight. Rods and cones are furthermore coupled with homologous and heterologous gap junctions (DeVries et al., 2002; Hornstein et al., 2005). The rod-rod coupling is critical in low light conditions to integrate small inputs from multiple rods, thereby averaging signals across rods to improve the signal to noise ratio (Fain, 1975; Hornstein et al., 2005; Li et al., 2012). As light levels increase, released dopamine acts on D4Rs in photoreceptors to decouple them, transitioning retinal signaling from rod to cone dominance (Derouiche and Asan, 1999; Ribelayga et al., 2008; Jin et al., 2015).
Dopamine similarly reduces coupling in the retinal network. Horizontal cell homologous coupling is reduced by dopamine and by light in a variety of species (Dong and McReynolds, 1991; Xin and Bloomfield, 1999; Packer and Dacey, 2005), which reduces the receptive field size of horizontal cells. The functional significance of this well-known fact has not been clearly understood. One plausible example is that horizontal cells contribute to the receptive field surround of a subset of primate ganglion cells, and dopamine modulates the surround inhibition to those ganglion cells (Mcmahon et al., 2004; Zhang et al., 2011) [but see mouse ganglion cells (Dedek et al., 2008)]. The homologous coupling of AII amacrine cells is also regulated by dopamine. AII amacrine cells are a critical component of the rod-signaling pathway, and accordingly, dopamine works as it does in rod photoreceptors. Individual AIIs can pass small signals within a wide network of AIIs through gap junction coupling in low light conditions, whereas this coupling is closed by light. In this way, dopamine in bright ambient conditions contributes to the dominance of cone-mediated signaling (Pourcho, 1982; McMahon and Mattson, 1996; He et al., 2000; Zhang et al., 2011; Hirasawa et al., 2012).
In addition to its effects on gap junctions, dopamine also reduces the response gain across cell populations, preventing saturation as ambient light levels increase. The gain control by retinal dopamine is primarily mediated by modulating voltage-gated channels, similar to its effects seen elsewhere in the CNS (Surmeier et al., 1995; Carr et al., 2003; Rosenkranz and Johnston, 2006). At the level of photoreceptors, dopamine works on D4Rs to decrease the responsivity of rod photoreceptors by suppressing an Ih current required for rod repolarization (Kawai et al., 2011). Dopamine furthermore modulates horizontal cells and subsets of RGCs via D1R inhibition of voltage-gated Ca2+ or Na+ currents to decrease visual signaling (Jensen and Daw, 1986; Vaquero et al., 2001; Hayashida and Ishida, 2004; Hayashida et al., 2009; Blasic et al., 2012; Ogata et al., 2012; Liu et al., 2016b). Lastly, previous studies have shown that light-adaptation or activation of D1Rs in bipolar cells leads to increased GABAergic feedback from horizontal cells, increasing the strength of surround inhibition (Cook and McReynolds, 1998; Chaffiol et al., 2017). Taken together, dopamine mediates light adaptation in retinal cell populations through gap junction regulation and by controlling neuronal response gain control.
Finally, dopaminergic amacrine cells exhibit a specific connection to the intrinsically photosensitive retinal ganglion cells (ipRGCs) that are key neurons for the circadian rhythm via their entrainment of the suprachiasmatic nucleus (Berson et al., 2002; Qiu et al., 2005). Dopaminergic cells receive excitatory retrograde visual signaling from M1 ipRGCs (Zhang et al., 2012; Prigge et al., 2016). In turn, released dopamine regulates melanopsin mRNA expression in ipRGCs and reduces their light responses (Sakamoto et al., 2005; Van Hook et al., 2012). In this way, dopamine also appears to modulate the maintenance of circadian rhythms.
A Novel Role for Dopamine in Retinal Signal Processing: Visual Signal Decorrelation
As we described, the olfactory bulb and retina exhibit various similarities. Both systems utilize comparable neural networks to process incoming receptor signals before projecting to the cortices. Moreover, both systems contain neuromodulators that tune signal processing. One such modulator is dopamine, which coordinates D1R and D2R signaling among neurons residing in each system. However, several dissimilarities are also present. In the olfactory bulb, dopamine plays a role in odor signal decorrelation. In the retina, dopamine plays a role in light adaptation and circadian rhythm; however, visual signal decorrelation has not previously been attributed to dopamine modulation. Our findings in the present study demonstrate a novel role for dopamine in the retina, increasing the peak temporal tuning of some bipolar cell types, and regulating temporal overlap between bipolar cell types.
In the retina, different features of images such as color, motion, and shape are encoded through distinct neural pathways, which are sent out to the brain in parallel (Enroth-Cugell and Robson, 1966; Boycott and Wässle, 1974; Livingstone and Hubel, 1987, 1988; Awatramani and Slaughter, 2000; Wässle, 2004). Parallel processing starts as early as the second-order neurons in the retina, bipolar cells, which extract distinct features of image signaling from photoreceptors and encode them across approximately 15 types of bipolar cells (Wu et al., 2000; Ghosh et al., 2004; Wässle et al., 2009; Helmstaedter et al., 2013; Shekhar et al., 2016). Distinct functions for each type have been gradually understood (Euler et al., 2014). Type 1 through 4 bipolar cells are classified as OFF bipolar cells, encoding light offset, while types 5–9 and rod bipolar cells (ON-bipolar cells) encode the onset of light. Rod and cone signaling is also encoded through distinct types of bipolar cells. Additionally, chromatic information is also uniquely separated, where bipolar cells types 1 and 9 carry mid- and short-wavelength light information, respectively (Breuninger et al., 2011). Furthermore, each bipolar cell type exhibits distinct temporal tuning. Types 2, 3, 5, 7 bipolar cells encode fast-changing light stimuli (e.g., object motion, object edge detection), while the others likely encode more stationary features of light (e.g., color, shape; Ichinose et al., 2014; Ichinose and Hellmer, 2016). Previously, we found that D1Rs are expressed by bipolar cells in a type-dependent manner (Farshi et al., 2016). If dopamine modulates visual signal processing only in a subset of bipolar cells, this will demonstrate temporal signal decorrelation, a new role of dopamine in the retina.
We found that a D1R agonist, SKF38393, shifted peak frequency responses towards higher frequencies in a subset of bipolar cells (Figures 3, 4). In ON-bipolar cells, D1Rs are expressed by type 5-2, XBC, 6, and 7 bipolar cells (Farshi et al., 2016), which exhibit mid to high-frequency tuning (Ichinose et al., 2014). We found that SKF increased the peak frequency responses in type 5, 6, and XBC that were consistent with the D1R-expressing bipolar cell types. There are no known markers for subsets of type 5 bipolar cells, and thus, we were not able to confirm that type 5 cells we recorded were D1R-expressing types. However, we found that the frequency tuning of SKF-sensitive cells was higher than that of the SKF insensitive cells (Figure 4). Taken together, our data suggest that dopamine enables high-temporal tuning ON-bipolar cells to respond to higher frequencies through D1Rs.
For a subset of OFF bipolar cells, dopamine also shifted the peak frequency response to higher frequencies (Figures 3, 4). In the present study, we had only a limited number of OFF cells for each type and could not correlate morphological types and SKF sensitivity. However, only a subset of OFF bipolar cells was SKF-sensitive, suggesting type-specific dopaminergic modulation. We previously showed that D1Rs are expressed by type 1, 3b, and 4 OFF bipolar cells which are low-frequency tuning cells (Farshi et al., 2016; Ichinose and Hellmer, 2016). Therefore, this suggests that dopamine may boost the temporal response of previously low-frequency tuning bipolar cells towards higher frequencies, such that all OFF bipolar cells would become sensitive to higher frequencies in contrast to the ON pathway bipolar cell types.
To explore the underlying mechanisms, we examined the dopaminergic effect on voltage-gated Ca2+ currents and HCN currents. HCN currents were increased by SKF in a subset of bipolar cells (Figure 5). HCN currents are critical for rhythmic activities in the heart pacemaker cells and neurons in the central nervous system (Baker et al., 1997; Day et al., 2005; Knop et al., 2008). In contrast, in the retina HCN channels have been associated with transient signaling as well as higher-frequency tuning (Cangiano et al., 2007; Della Santina et al., 2012; Puthussery et al., 2013; Bemme et al., 2017). These results suggest that HCN currents shape the high-frequency responses. Voltage-gated Ca2+ currents were examined because it may increase the membrane excitability. Contrary to our expectation, LVA currents were reduced by SKF in a subset of bipolar cells (Figure 6). LVA Ca2+ currents support the burst spiking activity in neurons (Fan et al., 2000; Pellegrini et al., 2016); however, its effect on graded synaptic responses has not been understood. Future investigation will need to elucidate the mechanism of temporal response modulation in bipolar cells by dopamine.
Alternatively, the observed effects of SKF could come from other D1R containing neurons. While we could not directly rule out possible contributions from D1Rs in other neurons such as AII amacrine or horizontal cells, we minimized this possibility through our experimental conditions. For example, we adapted the preparations with a rod-saturating light background before recordings; therefore, D1R-mediated AII amacrine or horizontal cell uncoupling and amplitude reductions likely were already present before recording. Moreover, the 100 μm diameter spot size of the stimulus would likely be small enough to mitigate horizontal cell feedback that was not already blocked by bicuculline. Interestingly, AII-AII gap junction coupling within the AII network can act as a low-pass filter (Veruki and Hartveit, 2002; Veruki et al., 2008) in a similar frequency range to signals observed in this study. However, it has been shown that primarily sustained currents pass between cone bipolar cells through the AII network (Kuo et al., 2016), which would be unlikely to affect the change in high-frequency tuning that we observed. Furthermore, it does not explain why a subset of ON bipolar cells was SKF-insensitive. Therefore, we concluded that our results of dopaminergic temporal response modulation were attributable to direct bipolar cell activation.
Inspired by a comparison between the olfactory bulb and the retina, we found that a general function of dopamine is to mediate signal decorrelation in both systems, despite using unique mechanisms in each. Ultimately, this study adds to a growing body of evidence that the intrinsic signaling properties regulating bipolar cell output are subject to extrinsic tuning both via inhibition as well as neuromodulation (Ayoub and Matthews, 1992; Tooker et al., 2013; Franke et al., 2017; Hall et al., 2019) to shape parallel signal processing in the retina.
Data Availability Statement
Requests to access the datasets should be directed to dGljaGlub3NAbWVkLndheW5lLmVkdQ==.
Ethics Statement
The animal study was reviewed and approved by the Institutional Animal Care and Use Committee at Wayne State University (protocol no. A05-03-15).
Author Contributions
TI designed the study, collected experimental data, and performed statistical analysis. CH, JB, LH, CK, and TI wrote the manuscript. All authors contributed to the article and approved the submitted version.
Funding
The present study was supported by the National Institutes of Health (NIH) project grant (R01 EY020533; R01 EY028915) to TI, co-financed by Research to Prevent Blindness (RPB), RPB for Medical Student LH, and the Rumble Fellowship to CH by the Wayne State University Graduate School.
Conflict of Interest
The authors declare that the research was conducted in the absence of any commercial or financial relationships that could be construed as a potential conflict of interest.
References
Astic, L., Saucier, D., and Holley, A. (1987). Topographical relationships between olfactory receptor cells and glomerular foci in the rat olfactory bulb. Brain Res. 424, 144–152. doi: 10.1016/0006-8993(87)91204-2
Aungst, J. L., Heyward, P. M., Puche, A. C., Karnup, S. V., Hayar, A., Szabo, G., et al. (2003). Centre-surround inhibition among olfactory bulb glomeruli. Nature 426, 623–629. doi: 10.1038/nature02185
Awatramani, G. B., and Slaughter, M. M. (2000). Origin of transient and sustained responses in ganglion cells of the retina. J. Neurosci. 20, 7087–7095. doi: 10.1523/jneurosci.20-18-07087.2000
Ayoub, G. S., and Matthews, G. (1992). Substance P modulates calcium current in retinal bipolar neurons. Vis. Neurosci. 8, 539–544. doi: 10.1017/s0952523800005630
Baden, T., Berens, P., Bethge, M., and Euler, T. (2013). Spikes in mammalian bipolar cells support temporal layering of the inner retina. Curr. Biol. 23, 48–52. doi: 10.1016/j.cub.2012.11.006
Baker, K., Warren, K. S., Yellen, G., and Fishman, M. C. (1997). Defective “pacemaker” current (Ih) in a zebrafish mutant with a slow heart rate. Proc. Natl. Acad. Sci. U S A 94, 4554–4559. doi: 10.1073/pnas.94.9.4554
Banerjee, A., Marbach, F., Anselmi, F., Koh, M. S., Davis, M. B., Garcia da silva, P., et al. (2015). An interglomerular circuit gates glomerular output and implements gain control in the mouse olfactory bulb. Neuron 87, 193–207. doi: 10.1016/j.neuron.2015.06.019
Bemme, S., Weick, M., and Gollisch, T. (2017). Differential effects of HCN channel block on on and off pathways in the retina as a potential cause for medication-induced phosphene perception. Invest. Ophthalmol. Vis. Sci. 58, 4754–4767. doi: 10.1167/iovs.17-21572
Berntson, A., and Taylor, W. R. (2000). Response characteristics and receptive field widths of on-bipolar cells in the mouse retina. J. Physiol. 524, 879–889. doi: 10.1111/j.1469-7793.2000.00879.x
Berson, D. M., Dunn, F. A., and Takao, M. (2002). Phototransduction by retinal ganglion cells that set the circadian clock. Science 295, 1070–1073. doi: 10.1126/science.1067262
Blasic, J. R. Jr., Brown, R. L., and Robinson, P. R. (2012). Phosphorylation of mouse melanopsin by protein kinase A. PLoS One 7:e45387. doi: 10.1371/journal.pone.0045387
Borghuis, B. G., Marvin, J. S., Looger, L. L., and Demb, J. B. (2013). Two-photon imaging of nonlinear glutamate release dynamics at bipolar cell synapses in the mouse retina. J. Neurosci. 33, 10972–10985. doi: 10.1523/jneurosci.1241-13.2013
Boycott, B. B., and Wässle, H. (1974). The morphological types of ganglion cells of the domestic cat’s retina. J. Physiol. 240, 397–419. doi: 10.1113/jphysiol.1974.sp010616
Breuninger, T., Puller, C., Haverkamp, S., and Euler, T. (2011). Chromatic bipolar cell pathways in the mouse retina. J. Neurosci. 31, 6504–6517. doi: 10.1523/jneurosci.0616-11.2011
Burkhardt, D. A., Fahey, P. K., and Sikora, M. A. (2007). Retinal bipolar cells: temporal filtering of signals from cone photoreceptors. Vis. Neurosci. 24, 765–774. doi: 10.1017/s0952523807070630
Bushdid, C., Magnasco, M. O., Vosshall, L. B., and Keller, A. (2014). Humans can discriminate more than 1 trillion olfactory stimuli. Science 343, 1370–1372. doi: 10.1126/science.1249168
Cangiano, L., Gargini, C., Della Santina, L., Demontis, G. C., and Cervetto, L. (2007). High-pass filtering of input signals by the Ih current in a non-spiking neuron, the retinal rod bipolar cell. PLoS One 2:e1327. doi: 10.1371/journal.pone.0001327
Cao, Y., Pahlberg, J., Sarria, I., Kamasawa, N., Sampath, A. P., and Martemyanov, K. A. (2012). Regulators of G protein signaling RGS7 and RGS11 determine the onset of the light response in ON bipolar neurons. Proc. Natl. Acad. Sci. U S A 109, 7905–7910. doi: 10.1073/pnas.1202332109
Carr, D. B., Day, M., Cantrell, A. R., Held, J., Scheuer, T., Catterall, W. A., et al. (2003). Transmitter modulation of slow, activity-dependent alterations in sodium channel availability endows neurons with a novel form of cellular plasticity. Neuron 39, 793–806. doi: 10.1016/s0896-6273(03)00531-2
Chaffiol, A., Ishii, M., Cao, Y., and Mangel, S. C. (2017). Dopamine regulation of GABAA receptors contributes to light/dark modulation of the ON-cone bipolar cell receptive field surround in the retina. Curr. Biol. 27, 2600.e4–2609.e4. doi: 10.1016/j.cub.2017.07.063
Cohen, A. I., Todd, R. D., Harmon, S., and O’Malley, K. L. (1992). Photoreceptors of mouse retinas possess D4 receptors coupled to adenylate cyclase. Proc. Natl. Acad. Sci. U S A 89, 12093–12097. doi: 10.1073/pnas.89.24.12093
Cook, P. B., and McReynolds, J. S. (1998). Modulation of sustained and transient lateral inhibitory mechanisms in the mudpuppy retina during light adaptation. J. Neurophysiol. 79, 197–204. doi: 10.1152/jn.1998.79.1.197
Day, M., Carr, D. B., Ulrich, S., Ilijic, E., Tkatch, T., and Surmeier, D. J. (2005). Dendritic excitability of mouse frontal cortex pyramidal neurons is shaped by the interaction among HCN, Kir2 and Kleak channels. J. Neurosci. 25, 8776–8787. doi: 10.1523/jneurosci.2650-05.2005
Dedek, K., Pandarinath, C., Alam, N. M., Wellershaus, K., Schubert, T., Willecke, K., et al. (2008). Ganglion cell adaptability: does the coupling of horizontal cells play a role? PLoS One 3:e1714. doi: 10.1371/journal.pone.0001714
Della Santina, L., Piano, I., Cangiano, L., Caputo, A., Ludwig, A., Cervetto, L., et al. (2012). Processing of retinal signals in normal and HCN deficient mice. PLoS One 7:e29812. doi: 10.1371/journal.pone.0029812
Derouiche, A., and Asan, E. (1999). The dopamine D2 receptor subfamily in rat retina: ultrastructural immunogold and in situ hybridization studies. Eur. J. Neurosci. 11, 1391–1402. doi: 10.1046/j.1460-9568.1999.00557.x
DeVries, S. H. (2000). Bipolar cells use kainate and AMPA receptors to filter visual information into separate channels. Neuron 28, 847–856. doi: 10.1016/s0896-6273(00)00158-6
DeVries, S. H., Qi, X., Smith, R., Makous, W., and Sterling, P. (2002). Electrical coupling between mammalian cones. Curr. Biol. 12, 1900–1907. doi: 10.1016/s0960-9822(02)01261-7
Dong, C. J., and McReynolds, J. S. (1991). The relationship between light, dopamine release and horizontal cell coupling in the mudpuppy retina. J. Physiol. 440, 291–309. doi: 10.1113/jphysiol.1991.sp018709
Dowling, J. E. (2012). The Retina: An Approachable Part of the Brain. Cambridge, MA: Belknap Press of Harvard University Press.
Duan, X., Krishnaswamy, A., De La Huerta, I., and Sanes, J. R. (2014). Type II cadherins guide assembly of a direction-selective retinal circuit. Cell 158, 793–807. doi: 10.1016/j.cell.2014.06.047
Ennis, M., Zhou, F.-M., Ciombor, K. J., Aroniadou-Anderjaska, V., Hayar, A., Borrelli, E., et al. (2001). Dopamine D2 receptor-mediated presynaptic inhibition of olfactory nerve terminals. J. Neurophysiol. 86, 2986–2997. doi: 10.1152/jn.2001.86.6.2986
Enroth-Cugell, C., and Robson, J. G. (1966). The contrast sensitivity of retinal ganglion cells of the cat. J. Physiol. 187, 517–552. doi: 10.1113/jphysiol.1966.sp008107
Escanilla, O., Yuhas, C., Marzan, D., and Linster, C. (2009). Dopaminergic modulation of olfactory bulb processing affects odor discrimination learning in rats. Behav. Neurosci. 123, 828–833. doi: 10.1037/a0015855
Euler, T., Haverkamp, S., Schubert, T., and Baden, T. (2014). Retinal bipolar cells: elementary building blocks of vision. Nat. Rev. Neurosci. 15, 507–519. doi: 10.1038/nrn3783
Fain, G. L. (1975). Quantum sensitivity of rods in the toad retina. Science 187, 838–841. doi: 10.1126/science.1114328
Fan, S. F., and Yazulla, S. (2001). Dopamine depletion with 6-OHDA enhances dopamine D1-receptor modulation of potassium currents in retinal bipolar cells. Vis. Neurosci. 18, 327–337. doi: 10.1017/s0952523801182167
Fan, Y. P., Horn, E. M., and Waldrop, T. G. (2000). Biophysical characterization of rat caudal hypothalamic neurons: calcium channel contribution to excitability. J. Neurophysiol. 84, 2896–2903. doi: 10.1152/jn.2000.84.6.2896
Farshi, P., Fyk-Kolodziej, B., Krolewski, D. M., Walker, P. D., and Ichinose, T. (2016). Dopamine D1 receptor expression is bipolar cell type-specific in the mouse retina. J. Comp. Neurol. 524, 2059–2079. doi: 10.1002/cne.23932
Franke, K., Berens, P., Schubert, T., Bethge, M., Euler, T., and Baden, T. (2017). Inhibition decorrelates visual feature representations in the inner retina. Nature 542, 439–444. doi: 10.1038/nature21394
Ghosh, K. K., Bujan, S., Haverkamp, S., Feigenspan, A., and Wässle, H. (2004). Types of bipolar cells in the mouse retina. J. Comp. Neurol. 469, 70–82. doi: 10.1002/cne.10985
Gire, D. H., Franks, K. M., Zak, J. D., Tanaka, K. F., Whitesell, J. D., Mulligan, A. A., et al. (2012). Mitral cells in the olfactory bulb are mainly excited through a multistep signaling path. J. Neurosci. 32, 2964–2975. doi: 10.1523/JNEUROSCI.5580-11.2012
Hall, L. M., Hellmer, C. B., Koehler, C. C., and Ichinose, T. (2019). Bipolar cell Type-specific expression and conductance of α-7 nicotinic acetylcholine receptors in the mouse retina. Invest. Ophthalmol. Vis. Sci. 60, 1353–1361. doi: 10.1167/iovs.18-25753
Harsanyi, K., and Mangel, S. C. (1992). Activation of a D2 receptor increases electrical coupling between retinal horizontal cells by inhibiting dopamine release. Proc. Natl. Acad. Sci. U S A 89, 9220–9224. doi: 10.1073/pnas.89.19.9220
Hayashida, Y., and Ishida, A. T. (2004). Dopamine receptor activation can reduce voltage-gated Na+ current by modulating both entry into and recovery from inactivation. J. Neurophysiol. 92, 3134–3141. doi: 10.1152/jn.00526.2004
Hayashida, Y., Rodriguez, C. V., Ogata, G., Partida, G. J., Oi, H., Stradleigh, T. W., et al. (2009). Inhibition of adult rat retinal ganglion cells by D1-type dopamine receptor activation. J. Neurosci. 29, 15001–15016. doi: 10.1523/jneurosci.3827-09.2009
He, C., Chen, F., Li, B., and Hu, Z. (2014). Neurophysiology of HCN channels: from cellular functions to multiple regulations. Prog. Neurobiol. 112, 1–23. doi: 10.1016/j.pneurobio.2013.10.001
He, S., Weiler, R., and Vaney, D. I. (2000). Endogenous dopaminergic regulation of horizontal cell coupling in the mammalian retina. J. Comp. Neurol. 418, 33–40. doi: 10.1002/(sici)1096-9861(20000228)418:1<33::aid-cne3>3.0.co;2-j
Hellmer, C. B., Zhou, Y., Fyk-Kolodziej, B., Hu, Z., and Ichinose, T. (2016). Morphological and physiological analysis of type-5 and other bipolar cells in the mouse retina. Neuroscience 315, 246–258. doi: 10.1016/j.neuroscience.2015.12.016
Helmstaedter, M., Briggman, K. L., Turaga, S. C., Jain, V., Seung, H. S., and Denk, W. (2013). Connectomic reconstruction of the inner plexiform layer in the mouse retina. Nature 500, 168–174. doi: 10.1038/nature12346
Hirasawa, H., Betensky, R. A., and Raviola, E. (2012). Corelease of dopamine and GABA by a retinal dopaminergic neuron. J. Neurosci. 32, 13281–13291. doi: 10.1523/jneurosci.2213-12.2012
Hornstein, E. P., Verweij, J., Li, P. H., and Schnapf, J. L. (2005). Gap-junctional coupling and absolute sensitivity of photoreceptors in macaque retina. J. Neurosci. 25, 11201–11209. doi: 10.1523/JNEUROSCI.3416-05.2005
Horwitz, G. C., Risner-Janiczek, J. R., Jones, S. M., and Holt, J. R. (2011). HCN channels expressed in the inner ear are necessary for normal balance function. 31, 16814–16825. doi: 10.1523/jneurosci.3064-11.2011
Hsia, A. Y., Vincent, J.-D., and Lledo, P.-M. (1999). Dopamine depresses synaptic inputs into the olfactory bulb. J. Neurophysiol. 82, 1082–1085. doi: 10.1152/jn.1999.82.2.1082
Hu, C., Bi, A., and Pan, Z. H. (2009). Differential expression of three T-type calcium channels in retinal bipolar cells in rats. Vis. Neurosci. 26, 177–187. doi: 10.1017/s0952523809090026
Ichinose, T., and Hellmer, C. B. (2016). Differential signalling and glutamate receptor compositions in the OFF bipolar cell types in the mouse retina. J. Physiol. 594, 883–894. doi: 10.1113/jp271458
Ichinose, T., and Lukasiewicz, P. D. (2012). The mode of retinal presynaptic inhibition switches with light intensity. J. Neurosci. 32, 4360–4371. doi: 10.1523/jneurosci.5645-11.2012
Ichinose, T., Fyk-Kolodziej, B., and Cohn, J. (2014). Roles of ON cone bipolar cell subtypes in temporal coding in the mouse retina. J. Neurosci. 34, 8761–8771. doi: 10.1523/jneurosci.3965-13.2014
Iuphar. (2020). Guide to Pharmacology. Available online at: https://www.guidetopharmacology.org/.
Iuvone, P. M., Galli, C. L., Garrison-Gund, C. K., and Neff, N. H. (1978). Light stimulates tyrosine hydroxylase activity and dopamine synthesis in retinal amacrine neurons. Science 202, 901–902. doi: 10.1126/science.30997
Jensen, R. J., and Daw, N. W. (1986). Effects of dopamine and its agonists and antagonists on the receptive field properties of ganglion cells in the rabbit retina. Neuroscience 17, 837–855. doi: 10.1016/0306-4522(86)90049-7
Jin, N. G., Chuang, A. Z., Masson, P. J., and Ribelayga, C. P. (2015). Rod electrical coupling is controlled by a circadian clock and dopamine in mouse retina. J. Physiol. 593, 1597–1631. doi: 10.1113/jphysiol.2014.284919
Kawai, F., Horiguchi, M., and Miyachi, E. (2011). Dopamine modulates the voltage response of human rod photoreceptors by inhibiting the h current. Invest. Ophthalmol. Vis. Sci. 52, 4113–4117. doi: 10.1167/iovs.10-6983
Kirsch, M., and Wagner, H. J. (1989). Release pattern of endogenous dopamine in teleost retinae during light adaptation and pharmacological stimulation. Vision Res. 29, 147–154. doi: 10.1016/0042-6989(89)90120-x
Knop, G. C., Seeliger, M. W., Thiel, F., Mataruga, A., Kaupp, U. B., Friedburg, C., et al. (2008). Light responses in the mouse retina are prolonged upon targeted deletion of the HCN1 channel gene. Eur. J. Neurosci. 28, 2221–2230. doi: 10.1111/j.1460-9568.2008.06512.x
Kramer, S. G. (1971). Dopamine: a retinal neurotransmitter. I. Retinal uptake, storage and light-stimulated release of H3-dopamine in vivo. Invest. Ophthalmol. 10, 438–452.
Krizaj, D. (2000). Mesopic state: cellular mechanisms involved in pre- and post-synaptic mixing of rod and cone signals. Microsc. Res. Tech. 50, 347–359. doi: 10.1002/1097-0029(20000901)50:5<347::aid-jemt4>3.0.co;2-d
Kruzich, P. J., and Grandy, D. K. (2004). Dopamine D2 receptors mediate two-odor discrimination and reversal learning in C57BL/6 mice. BMC Neurosci. 5:12. doi: 10.1186/1471-2202-5-12
Kuo, S. P., Schwartz, G. W., and Rieke, F. (2016). Nonlinear spatiotemporal integration by electrical and chemical synapses in the retina. Neuron 90, 320–332. doi: 10.1016/j.neuron.2016.03.012
Li, P. H., Verweij, J., Long, J. H., and Schnapf, J. L. (2012). Gap-junctional coupling of mammalian rod photoreceptors and its effect on visual detection. J. Neurosci. 32, 3552–3562. doi: 10.1523/jneurosci.2144-11.2012
Lindstrom, S. H., Ryan, D. G., Shi, J., and Devries, S. H. (2014). Kainate receptor subunit diversity underlying response diversity in retinal off bipolar cells. J. Physiol. 592, 1457–1477. doi: 10.1113/jphysiol.2013.265033
Liu, S., Plachez, C., Shao, Z., Puche, A., and Shipley, M. T. (2013). Olfactory bulb short axon cell release of GABA and dopamine produces a temporally biphasic inhibition-excitation response in external tufted cells. J. Neurosci. 33, 2916–2926. doi: 10.1523/jneurosci.3607-12.2013
Liu, S., Puche, A. C., and Shipley, M. T. (2016a). The interglomerular circuit potently inhibits olfactory bulb output neurons by both direct and indirect pathways. J. Neurosci. 36, 9604–9617. doi: 10.1523/jneurosci.1763-16.2016
Liu, X., Grove, J. C., Hirano, A. A., Brecha, N. C., and Barnes, S. (2016b). Dopamine D1 receptor modulation of calcium channel currents in horizontal cells of mouse retina. J. Neurophysiol. 116, 686–697. doi: 10.1152/jn.00990.2015
Livingstone, M. S., and Hubel, D. H. (1987). Psychophysical evidence for separate channels for the perception of form, color, movement and depth. J. Neurosci. 7, 3416–3468. doi: 10.1523/jneurosci.07-11-03416.1987
Livingstone, M. S., and Hubel, D. H. (1988). Segregation of form, color, movement, and depth: anatomy, physiology and perception. Science 240, 740–749. doi: 10.1126/science.3283936
Ma, Y. P., Cui, J., Hu, H. J., and Pan, Z. H. (2003). Mammalian retinal bipolar cells express inwardly rectifying K+ currents (IKir) with a different distribution than that of Ih. J. Neurophysiol. 90, 3479–3489. doi: 10.1152/jn.00426.2003
Maher, B. J., and Westbrook, G. L. (2008). Co-transmission of dopamine and GABA in periglomerular cells. J. Neurophysiol. 99, 1559–1564. doi: 10.1152/jn.00636.2007
Malnic, B., Hirono, J., Sato, T., and Buck, L. B. (1999). Combinatorial receptor codes for odors. Cell 96. doi: 10.1016/s0092-8674(00)80581-4
Mariani, A. P., and Hokoc, J. N. (1988). Two types of tyrosine hydroxylase-immunoreactive amacrine cell in the rhesus monkey retina. J. Comp. Neurol. 276, 81–91. doi: 10.1002/cne.902760106
McMahon, D. G., and Mattson, M. P. (1996). Horizontal cell electrical coupling in the giant danio: synaptic modulation by dopamine and synaptic maintenance by calcium. Brain Res. 718, 89–96. doi: 10.1016/0006-8993(96)00043-1
Mcmahon, M. J., Packer, O. S., and Dacey, D. M. (2004). The classical receptive field surround of primate parasol ganglion cells is mediated primarily by a non-GABAergic pathway. J. Neurosci. 24, 3736–3745. doi: 10.1523/jneurosci.5252-03.2004
Megaw, P. L., Boelen, M. G., Morgan, I. G., and Boelen, M. K. (2006). Diurnal patterns of dopamine release in chicken retina. Neurochem. Int. 48, 17–23. doi: 10.1016/j.neuint.2005.08.004
Mombaerts, P., Wang, F., Dulac, C., Chao, S. K., Nemes, A., Mendelsohn, M., et al. (1996). Visualizing an olfactory sensory map. Cell 87, 675–686. doi: 10.1016/s0092-8674(00)81387-2
Mora-Ferrer, C., Yazulla, S., Studholme, K. M., and Haak-Frendscho, M. (1999). Dopamine D1-receptor immunolocalization in goldfish retina. J. Comp. Neurol. 411, 705–714. doi: 10.1002/(sici)1096-9861(19990906)411:4<705::aid-cne14>3.0.co;2-y
Müller, F., Scholten, A., Ivanova, E., Haverkamp, S., Kremmer, E., and Kaupp, U. B. (2003). HCN channels are expressed differentially in retinal bipolar cells and concentrated at synaptic terminals. Eur. J. Neurosci. 17, 2084–2096. doi: 10.1046/j.1460-9568.2003.02634.x
Nikolaeva, D. A., Astakhova, L. A., and Firsov, M. L. (2019). The effects of dopamine and dopamine receptor agonists on the phototransduction cascade of frog rods. Mol. Vis. 25, 400–414.
Ogata, G., Stradleigh, T. W., Partida, G. J., and Ishida, A. T. (2012). Dopamine and full-field illumination activate D1 and D2–D5-type receptors in adult rat retinal ganglion cells. J. Comp. Neurol. 520, 4032–4049. doi: 10.1002/cne.23159
Packer, O. S., and Dacey, D. M. (2005). Synergistic center-surround receptive field model of monkey H1 horizontal cells. J. Vis. 5, 1038–1054. doi: 10.1167/5.11.9
Parrish-Aungst, S., Shipley, M. T., Erdelyi, F., Szabo, G., and Puche, A. C. (2007). Quantitative analysis of neuronal diversity in the mouse olfactory bulb. J. Comp. Neurol. 501, 825–836. doi: 10.1002/cne.21205
Pellegrini, C., Lecci, S., Luthi, A., and Astori, S. (2016). Suppression of sleep spindle rhythmogenesis in mice with deletion of CaV3.2 and CaV3.3 T-type Ca2+ channels. Sleep 39, 875–885. doi: 10.5665/sleep.5646
Pfeiffer-Linn, C., and Lasater, E. M. (1993). Dopamine modulates in a differential fashion T- and L-type calcium currents in bass retinal horizontal cells. J. Gen. Physiol. 102, 277–294. doi: 10.1085/jgp.102.2.277
Pourcho, R. G. (1982). Dopaminergic amacrine cells in the cat retina. Brain Res. 252, 101–109. doi: 10.1016/0006-8993(82)90982-9
Prigge, C. L., Yeh, P. T., Liou, N. F., Lee, C. C., You, S. F., Liu, L. L., et al. (2016). M1 ipRGCs influence visual function through retrograde signaling in the retina. J. Neurosci. 36, 7184–7197. doi: 10.1523/JNEUROSCI.3500-15.2016
Puthussery, T., Venkataramani, S., Gayet-Primo, J., Smith, R. G., and Taylor, W. R. (2013). NaV1.1 channels in axon initial segments of bipolar cells augment input to magnocellular visual pathways in the primate retina. J. Neurosci. 33, 16045–16059. doi: 10.1523/JNEUROSCI.1249-13.2013
Qiu, X., Kumbalasiri, T., Carlson, S. M., Wong, K. Y., Krishna, V., Provencio, I., et al. (2005). Induction of photosensitivity by heterologous expression of melanopsin. Nature 433, 745–749. doi: 10.1038/nature03345
Ray, T. A., Heath, K. M., Hasan, N., Noel, J. M., Samuels, I. S., Martemyanov, K. A., et al. (2014). GPR179 is required for high sensitivity of the mGluR6 signaling cascade in depolarizing bipolar cells. J. Neurosci. 34, 6334–6343. doi: 10.1523/JNEUROSCI.4044-13.2014
Ressler, K. J., Sullivan, S. L., and Buck, L. B. (1994). Information coding in the olfactory system: evidence for a stereotyped and highly organized epitope map in the olfactory bulb. Cell 79, 1245–1255. doi: 10.1016/0092-8674(94)90015-9
Ribelayga, C., Cao, Y., and Mangel, S. C. (2008). The circadian clock in the retina controls rod-cone coupling. Neuron 59, 790–801. doi: 10.1016/j.neuron.2008.07.017
Robinson, R. B., and Siegelbaum, S. A. (2003). Hyperpolarization-activated cation currents: from molecules to physiological function. Annu. Rev. Physiol. 65, 453–480. doi: 10.1146/annurev.physiol.65.092101.142734
Rosenkranz, J. A., and Johnston, D. (2006). Dopaminergic regulation of neuronal excitability through modulation of Ih in layer V entorhinal cortex. J. Neurosci. 26, 3229–3244. doi: 10.1523/JNEUROSCI.4333-05.2006
Sakamoto, K., Liu, C., Kasamatsu, M., Pozdeyev, N. V., Iuvone, P. M., and Tosini, G. (2005). Dopamine regulates melanopsin mRNA expression in intrinsically photosensitive retinal ganglion cells. Eur. J. Neurosci. 22, 3129–3136. doi: 10.1111/j.1460-9568.2005.04512.x
Shekhar, K., Lapan, S. W., Whitney, I. E., Tran, N. M., Macosko, E. Z., Kowalczyk, M., et al. (2016). Comprehensive classification of retinal bipolar neurons by single-cell transcriptomics. Cell 166, 1308.e30–1323.e30. doi: 10.1016/j.cell.2016.07.054
Stella, S. L. Jr., and Thoreson, W. B. (2000). Differential modulation of rod and cone calcium currents in tiger salamander retina by D2 dopamine receptors and cAMP. Eur. J. Neurosci. 12, 3537–3548. doi: 10.1046/j.1460-9568.2000.00235.x
Stewart, W. B., and Pedersen, P. E. (1987). The spatial organization of olfactory nerve projections. Brain Res. 411, 248–258. doi: 10.1016/0006-8993(87)91076-6
Surmeier, D. J., Bargas, J., Hemmings, H. C. Jr., Nairn, A. C., and Greengard, P. (1995). Modulation of calcium currents by a D1 dopaminergic protein kinase/phosphatase cascade in rat neostriatal neurons. Neuron 14, 385–397. doi: 10.1016/0896-6273(95)90294-5
Tatti, R., Bhaukaurally, K., Gschwend, O., Seal, R. P., Edwards, R. H., Rodriguez, I., et al. (2014). A population of glomerular glutamatergic neurons controls sensory information transfer in the mouse olfactory bulb. Nat. Commun. 5:3791. doi: 10.1038/ncomms4791
Tillerson, J. L., Caudle, W. M., Parent, J. M., Gong, C., Schallert, T., and Miller, G. W. (2006). Olfactory discrimination deficits in mice lacking the dopamine transporter or the D2 dopamine receptor. Behav. Brain Res. 172, 97–105. doi: 10.1016/j.bbr.2006.04.025
Tooker, R. E., Lipin, M. Y., Leuranguer, V., Rozsa, E., Bramley, J. R., Harding, J. L., et al. (2013). Nitric oxide mediates activity-dependent plasticity of retinal bipolar cell output via S-nitrosylation. J. Neurosci. 33, 19176–19193. doi: 10.1523/JNEUROSCI.2792-13.2013
Vaaga, C. E., Yorgason, J. T., Williams, J. T., and Westbrook, G. L. (2017). Presynaptic gain control by endogenous cotransmission of dopamine and GABA in the olfactory bulb. J. Neurophysiol. 117, 1163–1170. doi: 10.1152/jn.00694.2016
Van Hook, M. J., Wong, K. Y., and Berson, D. M. (2012). Dopaminergic modulation of ganglion-cell photoreceptors in rat. Eur. J. Neurosci. 35, 507–518. doi: 10.1111/j.1460-9568.2011.07975.x
Vaquero, C. F., Pignatelli, A., Partida, G. J., and Ishida, A. T. (2001). A dopamine- and protein kinase A-dependent mechanism for network adaptation in retinal ganglion cells. J. Neurosci. 21, 8624–8635. doi: 10.1523/JNEUROSCI.21-21-08624.2001
Veruki, M. L., and Hartveit, E. (2002). AII (Rod) amacrine cells form a network of electrically coupled interneurons in the mammalian retina. Neuron 33, 935–946. doi: 10.1016/s0896-6273(02)00609-8
Veruki, M. L., and Wässle, H. (1996). Immunohistochemical localization of dopamine D1 receptors in rat retina. Eur. J. Neurosci. 8, 2286–2297. doi: 10.1111/j.1460-9568.1996.tb01192.x
Veruki, M. L., Oltedal, L., and Hartveit, E. (2008). Electrical synapses between AII amacrine cells: dynamic range and functional consequences of variation in junctional conductance. J. Neurophysiol. 100, 3305–3322. doi: 10.1152/jn.90957.2008
Wachowiak, M., and Cohen, L. B. (1999). Presynaptic inhibition of primary olfactory afferents mediated by different mechanisms in lobster and turtle. J. Neurosci. 19, 8808–8817. doi: 10.1523/JNEUROSCI.19-20-08808.1999
Wässle, H. (2004). Parallel processing in the mammalian retina. Nat. Rev. Neurosci. 5, 747–757. doi: 10.1038/nrn1497
Wässle, H., Puller, C., Muller, F., and Haverkamp, S. (2009). Cone contacts, mosaics, and territories of bipolar cells in the mouse retina. J. Neurosci. 29, 106–117. doi: 10.1523/JNEUROSCI.4442-08.2009
Wei, C. J., Linster, C., and Cleland, T. A. (2006). Dopamine D2 receptor activation modulates perceived odor intensity. Behav. Neurosci. 120, 393–400. doi: 10.1037/0735-7044.120.2.393
Weiler, R., Baldridge, W. H., Mangel, S. C., and Dowling, J. E. (1997). Modulation of endogenous dopamine release in the fish retina by light and prolonged darkness. Vis. Neurosci. 14, 351–356. doi: 10.1017/s0952523800011470
Witkovsky, P. (2004). Dopamine and retinal function. Doc. Ophthalmol. 108, 17–40. doi: 10.1023/b:doop.0000019487.88486.0a
Witkovsky, P., Nicholson, C., Rice, M. E., Bohmaker, K., and Meller, E. (1993). Extracellular dopamine concentration in the retina of the clawed frog, Xenopus laevis. Proc. Natl. Acad. Sci. U S A 90, 5667–5671. doi: 10.1073/pnas.90.12.5667
Wu, S. M., Gao, F., and Maple, B. R. (2000). Functional architecture of synapses in the inner retina: segregation of visual signals by stratification of bipolar cell axon terminals. J. Neurosci. 20, 4462–4470. doi: 10.1523/JNEUROSCI.20-12-04462.2000
Xin, D., and Bloomfield, S. A. (1999). Dark- and light-induced changes in coupling between horizontal cells in mammalian retina. J. Comp. Neurol. 405, 75–87. doi: 10.1002/(sici)1096-9861(19990301)405:1<75::aid-cne6>3.0.co;2-d
Zhang, A. J., Jacoby, R., and Wu, S. M. (2011). Light- and dopamine-regulated receptive field plasticity in primate horizontal cells. J. Comp. Neurol. 519, 2125–2134. doi: 10.1002/cne.22604
Zhang, D. Q., Belenky, M. A., Sollars, P. J., Pickard, G. E., and McMahon, D. G. (2012). Melanopsin mediates retrograde visual signaling in the retina. PLoS One 7:e42647. doi: 10.1371/journal.pone.0042647
Zhang, D. Q., Zhou, T. R., and Mcmahon, D. G. (2007). Functional heterogeneity of retinal dopaminergic neurons underlying their multiple roles in vision. J. Neurosci. 27, 692–699. doi: 10.1523/JNEUROSCI.4478-06.2007
Keywords: retina, dopamine, patch clamp, visual signal processing, temporal processing
Citation: Hellmer CB, Bohl JM, Hall LM, Koehler CC and Ichinose T (2020) Dopaminergic Modulation of Signal Processing in a Subset of Retinal Bipolar Cells. Front. Cell. Neurosci. 14:253. doi: 10.3389/fncel.2020.00253
Received: 22 April 2020; Accepted: 23 July 2020;
Published: 14 August 2020.
Edited by:
Wallace B. Thoreson, University of Nebraska Medical Center, United StatesReviewed by:
Stuart Mangel, The Ohio State University, United StatesWilliam Grimes, National Institutes of Health (NIH), United States
Erika Eggers, University of Arizona, United States
Copyright © 2020 Hellmer, Bohl, Hall, Koehler and Ichinose. This is an open-access article distributed under the terms of the Creative Commons Attribution License (CC BY). The use, distribution or reproduction in other forums is permitted, provided the original author(s) and the copyright owner(s) are credited and that the original publication in this journal is cited, in accordance with accepted academic practice. No use, distribution or reproduction is permitted which does not comply with these terms.
*Correspondence: Tomomi Ichinose, dGljaGlub3NAbWVkLndheW5lLmVkdQ==