- 1Division of Genetics and Development, Krembil Research Institute, University Health Network, Toronto, ON, Canada
- 2Institute of Medical Science, University of Toronto, Toronto, ON, Canada
- 3Faculty of Medicine, University of Toronto, Toronto, ON, Canada
- 4Division of Neurosurgery, University of Toronto, Toronto, ON, Canada
Traumatic spinal cord injury (SCI) impedes signal transmission by disrupting both the local neurons and their surrounding synaptic connections. Although the majority of SCI patients retain spared neural tissue at the injury site, they predominantly suffer from complete autonomic and sensorimotor dysfunction. While there have been significant advances in the characterization of the spared neural tissue following SCI, the functional role of injury-induced interneuronal plasticity remains elusive. In healthy individuals, spinal interneurons are responsible for relaying signals to coordinate both sympathetic and parasympathetic functions. However, the spontaneous synaptic loss following injury alters these intricate interneuronal networks in the spinal cord. Here, we propose the synaptopathy hypothesis of SCI based on recent findings regarding the maladaptive role of synaptic changes amongst the interneurons. These maladaptive consequences include circuit inactivation, neuropathic pain, spasticity, and autonomic dysreflexia. Recent preclinical advances have uncovered the therapeutic potential of spinal interneurons in activating the dormant relay circuits to restore sensorimotor function. This review will survey the diverse role of spinal interneurons in SCI pathogenesis as well as treatment strategies to target spinal interneurons.
Introduction
Spinal cord injury (SCI) is a heterogeneous disorder that has devastating consequences on the patient’s well-being, independence, and quality of life (National Spinal Cord Injury Statistical Center, 2020). Initiated by either traumatic or non-traumatic aetiologies, SCI constitutes the second leading cause of paralysis worldwide (Armour et al., 2016; Ahuja et al., 2017; Badhiwala et al., 2020). Traumatic SCI results from the compression, contusion, and laceration of the spinal cord due to an external mechanical force. This initial shock produces toxic cellular debris and disrupts the local vasculature, leading to maladaptive consequences, including hypoxia, swelling, and inflammation (Fleming et al., 2006). Referred to as secondary injury, these pathologies exacerbate the spinal cord damage and present a barrier to the patient’s recovery (Tator and Fehlings, 1991). In the clinic, the manifestation of traumatic SCI is classified as either complete or incomplete injury (Blesch and Tuszynski, 2009). Complete SCI leads to the loss of both sensorimotor and autonomic function distal to the injury site. In contrast, incomplete SCI cases continue to possess partial connectivity across the spinal cord, with varying degrees of functional deficits in surrounding neural circuits (Raineteau and Schwab, 2001; Sekhon and Fehlings, 2001).
One of the ambiguous hallmarks of secondary SCI pathophysiology is the induced neuroplasticity in the perilesional neural tissue following complete and incomplete injuries (Hutson and Di Giovanni, 2019). Spinal neuroplasticity refers to the ability of spinal neural circuits to make physiological, anatomical, and functional changes in response to a stimulus (Baker-Herman et al., 2004; Cadotte et al., 2012). The neuroplastic nature of spinal synapses is crucial for the development of neural relay circuits during the embryonic and adolescence stage (Ladle et al., 2007). However, irregular synaptic alterations following injury or disease can lead to pain and spasticity (Cadotte et al., 2012; Cadotte and Fehlings, 2013). A crucial element of neuroplasticity in both healthy and injured spinal cords are interneurons, as they relay signals between different types of neurons to coordinate complex neurotransmission. Although the full extent of neuroplasticity in the injured spinal cord remains unknown, interneurons undergo dramatic changes after injury, which further complicates the injury progression (Harkema, 2008).
The investigation of spinal neural circuits and the underlying interneurons dates back to 1906, when the first paradigm of spinal reflexes was elucidated by Charles Sherrington (Burke, 2006). Further studies by Graham Brown, John C. Eccles, Anders Lundberg, and Elzbieta Jankowska in cat models defined the fundamental basis of spinal circuits and connectivity (Brown, 1914; Eccles, 1968; Jankowska, 2001; Goulding, 2009). These pioneering studies were crucial for describing spinal reflexes and locomotion. Additionally, the concept of a central pattern generator (CPG), which is a connected network of interneurons that function as local executive units to generate neural oscillations and subsequent rhythmic motor activity, was described in these studies (Guertin, 2013).
Current investigations of spinal neural circuits utilize leading-edge technologies to examine neuronal cytoarchitecture, electrophysiological properties, synaptic connectivity, single-cell transcriptional profile, and cell lineage. Emerging technologies, such as RNA sequencing, viral tracing, in vivo microscopy, and transgenic manipulations, have revolutionized our understanding of spinal neural circuits. Advances in single-cell RNA-sequencing can determine the cellular identity, molecular markers, and expressional zonation in the spinal cord (Sathyamurthy et al., 2018; Zeisel et al., 2018; Delile et al., 2019). Importantly, targeted genetic manipulations based on these transcriptional profiles elucidate the role of interneuronal subpopulations (Moran-Rivard et al., 2001; Wilson et al., 2008; Talpalar et al., 2013). In parallel, viral tracing provides crucial information regarding connectivity and pathways of individual neurons. Lastly, advanced imaging techniques allow the characterization of cell morphology, location, and lineage in live animals (Sekiguchi et al., 2016). These technologies have characterized the function of different cells involved in neural circuits both in healthy and injured spinal cords. However, the neuron-to-neuron as well as neural-glial connectivity and plasticity during both normal state and injury remains obscure. The vital role of interneurons in both healthy and injured spinal cords is of high interest. This article aims to provide a concise overview of the spinal interneurons heterogeneity and their role following traumatic injury.
Spinal Interneurons
Healthy spinal neural circuits are comprised of four main classes of local neurons including motoneurons, preganglionic neurons, ascending projection neurons, and spinal interneurons (Zholudeva et al., 2018). Recent single-cell transcriptional analysis has characterized 43 distinct neuronal populations in the rodent lumbar spinal cord (Sathyamurthy et al., 2018). These neurons are surrounded by various glial and vascular cells including mural cells, smooth muscle cells, oligodendrocytes, fibroblasts, endothelial cells, and astrocytes (Vanlandewijck et al., 2018). In addition, the spinal cord houses a variety of multipotent stem cells in the ependymal layer, including oligodendrocyte progenitor cells (OPC) and neural progenitor cells (NPC) (Meletis et al., 2008; Barnabé-Heider et al., 2010).
In healthy individuals, spinal interneurons relay sensorimotor input, transduce sensorimotor information sent from the spinal cord to supraspinal centers by ascending tract neurons, modulate motoneuron activity, transmit information between near and distant spinal cord segments, and provide a transmission line to the opposite side of the spinal cord (Figure 1A; Zholudeva et al., 2018). Although the full functional role of spinal interneurons continues to be investigated, they are known to play crucial roles in vital functions, such as breathing through the phrenic circuit or locomotion through CPGs (Guertin, 2013).
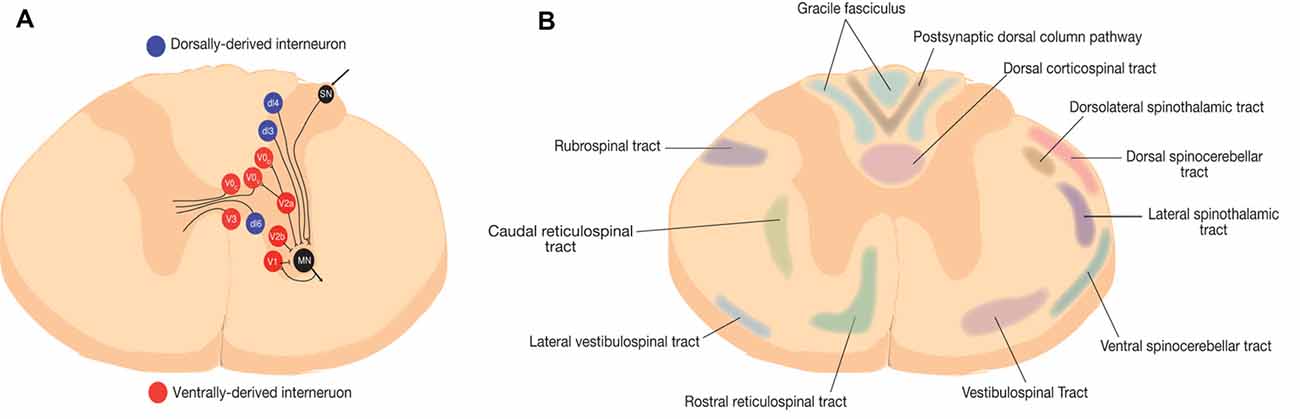
Figure 1. Representative schematic of spinal neural circuits in rodents. (A) Spinal interneurons are represented at the thoracic level. Red represents ventrally-derived interneurons and blue represent dorsally derived interneurons (Zholudeva et al., 2018). (B) Ascending and descending white matter tracts in rodents (Saliani et al., 2017).
Although various classification systems exist to describe the diversity of interneurons present in the spinal cord, they can be broadly classified into local and propriospinal interneurons. Local interneurons encompass short projections, which are crucial for neural oscillations and reflexes. In contrast, propriospinal interneurons are defined as neurons that project to a different spinal segment from which their cell bodies are located (Flynn et al., 2017). The propriospinal interneurons are further subdivided into short propriospinal interneurons and long propriospinal interneurons. Short propriospinal interneurons provide intersegmental connections, whereas long propriospinal interneurons connect distant regions, for instance between cervical and lumbar spinal regions. These propriospinal interneurons are crucial for interlimb coordination and play an important role in generating new circuits following injury (Flynn et al., 2011; Laliberte et al., 2019).
Local interneurons project either ipsilaterally or contralaterally. The interneurons that project to the opposite side of the spinal cord are referred to as contralateral, whereas interneurons that project into the same side are referred to as ipsilateral. The interneurons that project through the midline are referred to as commissural interneurons. Inhibitory interneurons release inhibitory neurotransmitters, such as γ-aminobutyric acid (GABA) and glycine. Whereas, excitatory interneurons release excitatory neurotransmitters, such as glutamate (Goulding, 2009).
Spinal interneurons are also classified based on their location and transcriptional profile during development, which arise from seven dorsal and four ventral progenitor cells (Table 1). Further characterization of the subtypes for each of these neuronal populations enables a reliable identification scheme to define their projection patterns, released neurotransmitters, and connectivity. During gestation, neuroepithelial cells at the ventricular zone of the neural tube are under the influence of a two-dimensional morphogen gradient system between the floor plate and the roof plate, which lead to the formation of several progenitor cells with differential expression of homeodomain transcription factors. These crucial morphogens include sonic hedgehog (Shh) and bone morphogenic proteins (BMP). In addition, the opposing fibroblast growth factor (FGF) and retinoic acid (RA) signalling molecules guide the rostral and caudal characterization of cells respectively (Jessell, 2000; Lu et al., 2015; Ogura et al., 2018). The result is the morphogenesis of 23 types of neurons through a series of cell divisions, which includes eight dorsally-derived interneurons, 13 ventrally-derived interneurons, and the connected motor neurons (Figure 1).
Dorsally-Derived Interneurons
There are eight classes of dorsally-derived interneurons, dI1 to dI6 as well as dILA and dILB, arising from seven progenitor cells (Lu et al., 2015). Although these interneurons originate from the dorsal region, some migrate ventrally during development. These cells are generated sequentially in early, mid, and late phases. The early phased interneurons include dI1 to dI3 and are generated from pd1 to pd3 progenitor cells, respectively. These cells migrate ventrally after development. The mid phase dorsal dI4 to dI6 are generated from pd4 to pd6 cells. Lastly, the late phase dILA and dILB are generated from pdIL progenitors cells (Gross et al., 2002; Lu et al., 2015).
The early-phased pd1, pd2, and pd3 progenitor cells express Atoh1, Ngn1/2, and Nash1 basic-helix-loop-helix (bHLH) transcription factors, respectively (Gowan et al., 2001; Avraham et al., 2009; Duval et al., 2014). The differentiated interneurons from these progenitors are characterized based on their LIM-homeodomain (LIM-HD) transcription factors, which mediate their axonal guidance and projection (Avraham et al., 2009). Specifically, the dI1 interneurons express Lhx2/9, while dI2 and dI3 interneurons express Lhx1/5 and Isl1, respectively (Gross et al., 2002; Avraham et al., 2009). The dI1 interneurons are located in the dorsal horn and integrate proprioceptive signals from the peripheral organs and project rostrally to the spinocerebellar tract (Gross et al., 2002; Avraham et al., 2009). These neurons can be further subdivided into contralaterally-projecting dI1c and ipsilaterally-projecting dI1i interneurons (Wilson et al., 2008). Lhx2/9 mutations result in loss of mid-line crossing in dI1c interneurons, which demonstrates their role in defining subtype identity in these neurons (Wilson et al., 2008). The dI2 interneurons are located in the intermediate spinal cord and the ventral horn, which are speculated to project contralaterally to transmit sensory input to the thalamus via the spinothalamic tract (Figures 1A,B; Gross et al., 2002). The dI3 interneurons are located in the intermediate spinal cord and the dorsal horn (Bui et al., 2013). These are excitatory neurons that project rostrally, ipsilaterally, and longitudinally through monosynaptic connections (Stepien et al., 2010; Bui et al., 2013; Lu et al., 2015).
The mid-phased dI4 to dl6 interneurons express Lbx1. In Lbx1 knock out animals, there is disrupted sensory transmission and excessive generation of commissural neurons (Gross et al., 2002). The dI4 interneurons are inhibitory GABAergic neurons, which are located in the dorsal horn and project ipsilaterally to convey somatosensory information (Gross et al., 2002). The dI5 interneurons are located at the dorsal horn, which are glutamatergic and contralaterally projecting neurons (Gross et al., 2002). These neurons express Brn3a, Tlx1, Tlx3, and Lmx1b transcription factors (Gross et al., 2002). The dI6 interneurons are located in the ventromedial spinal cord and are subdivided to neurons with either Wt1 or Dmrt3 expression (Gosgnach et al., 2017). Wt1 eletion alters forelimb hindlimb coordination in mice (Schnerwitzki et al., 2018). In contrast, Dmrt3 knock out mice exhibit altered stride length and swing time (Andersson et al., 2012).
The dILA and dILB develop later from a common progenitor cell, and their cell fate is significantly influenced by Achaete-Scute Family BHLH Transcription Factor 1 (ASCL1; Mizuguchi et al., 2006). These interneurons are mainly located in the superficial dorsal horn and carry out different roles in spinal circuits. The dILA interneurons are GABAergic, whereas dILb are glutamatergic and demonstrate a differential transcriptional profile from each other (Mizuguchi et al., 2006; Lu et al., 2015).
Ventrally-Derived Interneurons
The ventrally-derived interneurons arise from four progenitor cells called p0, p1, p2, and p3. Further maturation of these three lines of interneurons gives rise to a series of interneurons crucial for locomotion (Kiehn, 2016). V0 interneurons are characterized by their expression of Dbx1 (Pierani et al., 2001; Lanuza et al., 2004). These neurons are comprised of four subpopulations, including ventral (V0V), dorsal (V0D), cholinergic (V0C), and glutamatergic (V0G; Zagoraiou et al., 2009). The ablation of V0 interneurons or the deletion of Dbx1 leads to altered left-right coordination (Lanuza et al., 2004; Talpalar et al., 2013). The V0V and V0D are distinguished based on the expression of Evx1 and Pax7 factors (Talpalar et al., 2013). V0V neurons are excitatory and project commissural and play an important role in controlling locomotion (Talpalar et al., 2013).
The V1 interneurons are a large heterogenous class of inhibitory neurons, which include the vital renshaw and Ia interneurons (Bikoff et al., 2016). These neurons project ipsilaterally, express Engrailed1 (En1), and are crucial for executing of rhythmic activity via their function in recurrent and reciprocal inhibition (Falgairolle and O’Donovan, 2019). Recurrent inhibition (also known as Renshaw inhibition) refers to the suppressive effect of Renshaw cells back on the motoneurons that initiated their activation in a negative feedback mechanism (Özyurt et al., 2019). In contrast, reciprocal inhibition refers to the action of Ia interneurons to inhibit of antagonist neurons during the activation of the agonist neuron (Crone et al., 1987; Katz et al., 1991). Genetic inhibition of En1 expressing interneurons in mice demonstrates the importance of these neurons to generate rhythmic activity and quick locomotion (Gosgnach et al., 2006). A subsequent genetic ablation study in zebrafish echoed these results by demonstrating that V1 interneurons are crucial for suppressing the activities of V2a and motor neurons (Kimura and Higashijima, 2019).
The V2 interneurons are derived from P2 progenitor cells and express LIM Homeobox 3 (Lhx3) transcription factor. These neurons project ipsilaterally and consist of four subpopulations, including V2a, V2b, V2c, and V2d (Dougherty et al., 2013; Harris et al., 2019). V2a are excitatory neurons and are characterized by the expression of Chx10 and are important in locomotion and left-right coordination. The V2a ablation disrupts left-right coordination (Crone et al., 2008, 2009; Dougherty and Kiehn, 2010). These interneurons are further classified into type 1 and type 2 groups, with varying projections and transmission patterns (Hayashi et al., 2018; Menelaou and McLean, 2019). Single cell RNA-seq experiments reveal that these subtypes each encompass multiple subpopulations (Hayashi et al., 2018). The V2b interneurons are inhibitory and express Gata2/3 (Zhang et al., 2014). The V2c function is still unknown, but these cells have been shown to express Sox1 and do not express Gata3 (Lu et al., 2015; Harris et al., 2019). The V2d cells are newly characterized excitatory interneurons (Dougherty et al., 2013; Harris et al., 2019).
The V3 interneurons are glutamatergic, predominantly commissural, and necessary for locomotion (Zhang et al., 2008). They originate from the ventral Nkx2.2 + p3 progenitor domain, which is defined by a marked expression of the transcription factor Sim1. A recent study (Deska-Gauthier et al., 2020) elucidated that V3 interneurons are organized into early and late-born neurogenic waves, where late-born neurons displayed restricted sub-population fates (Deska-Gauthier et al., 2020). Further, the Sim1 transcription factor, while expressed on all post-mitotic V3 interneurons, is critical only for the diversification of early-born, but not late-born, V3 interneurons. This suggests that the timing of neurogenesis can alter the way definitive transcription factors regulate subpopulation cell fate specification. To date, several studies have characterized the function of V3 interneurons and concluded that they play a significant role in spinal locomotor circuitry and gait transitions through direct connections with the rhythm-generating circuits (Rybak et al., 2006; Danner et al., 2019). Strikingly, these studies have shown that V3 interneurons do not form synapses with motoneurons; however, contradicting data from anatomical studies have illustrated boutons from these neurons in apposition to motoneurons (Zhang et al., 2008). This, in conjunction with developmental studies that demonstrate the existence of several dorsal and ventral sub-populations of V3 interneurons, suggests these conflicting observations may be due to the functional diversity of V3 interneuron sub-populations. Indeed, a recent study using patch-clamp and glutamate uncaging demonstrated that V3 interneurons form functional layers (Chopek et al., 2018). The ventromedial V3 interneurons form synapses with each other, whereas the ventrolateral V3 interneurons form synapses with the ipsilateral motoneurons. These motoneurons then provide excitatory and glutamatergic feedback to the V3 interneurons. This distinct circuitry implicates that V3 interneurons can contribute to precise spatiotemporal movements.
Injury-Induced Neuroplasticity
Although traumatic SCI culminates in demyelination, cavitation, glial reactivity, and impaired neural connections, many patients demonstrate functional improvements following incomplete injury (Fawcett et al., 2007). Recent investigations on SCI pathogenesis have elucidated the mechanisms behind functional improvements and barriers to recovery (Courtine and Sofroniew, 2019). There are three distinct histological compartments formed after injury, including a non-neuronal lesion core, astroglial border, and reactive prelisional neural tissue (Figure 2; O’Shea et al., 2017). Each compartment consists of distinctive cellular composition and undergoes unique pathophysiological changes after injury (Sofroniew, 2018).
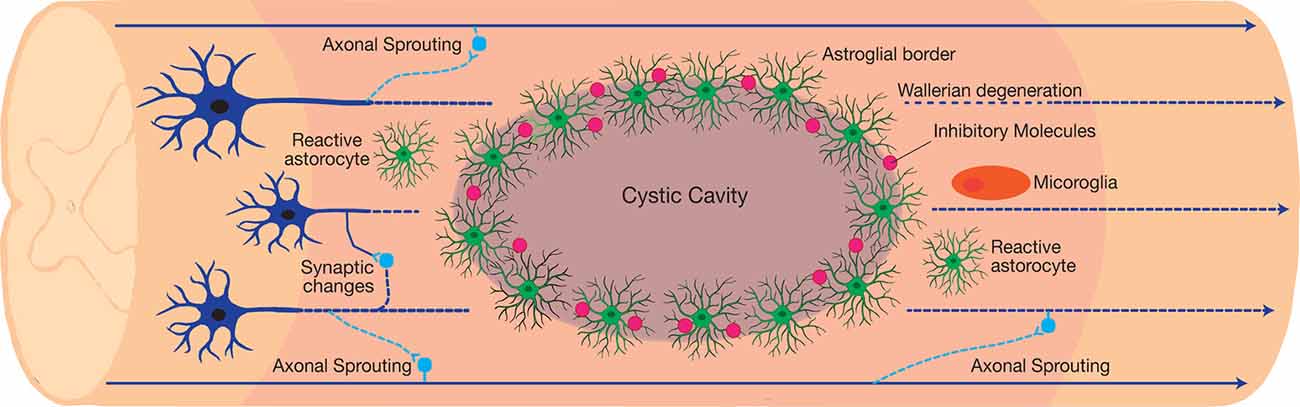
Figure 2. The neuroplastic nature of the spinal cord following traumatic incomplete spinal cord injury (SCI). The injury results in the formation of three distinct histological compartments: a non-neuronal lesion core, an astroglial scar, and a spared reactive perilesional neural tissue. Each compartment consists of a unique cellular composition and exhibits a distinct pathophysiology after injury. In the perilesional zone, reactive glia and the induced neuroplasticity alters the neuronal connections. Axonal sprouting and plasticity form bypass routes around the lesion, resulting in limited functional recovery after injury. However, maladaptive synaptic changes contribute to the dormancy of the spared neural circuits around the lesion, which introduces a barrier to recovery.
In the injury epicenter, injury-induced cell death introduces toxic debris into the spinal cord, which recruits both the local microglia and the circulatory immune cells to clear the introduced debris. Although this immune reaction initially plays a beneficial role, the extended reactivity of immune cells will further damage the neural tissue. The astroglial border is crucial for controlling the spread of immune cells. Serum proteins and local cell markers including ATP, Shh, BMPs, thrombin, FGF, and endothelin, promote astrocyte proliferation. This is in parallel to the synthesis and secretion of chondroitin sulfate proteoglycans (CSPGs) via NG2 OPC (NG2-OPC) and macrophages into the extracellular matrix (Jones et al., 2002). The newly formed astrocytes migrate and intermingle with CSPGs in order to organize into a barrier immediately adjacent to the non-neural lesion core, dubbed the astroglial scar (Silver and Silver, 2014). While the functional role of this barrier and the reactive glial cells after injury continues to be under investigation, the barrier is known to actively inhibit axonal regeneration and repair through the covalently attached chondroitin sulfate glycosaminoglycan (CS-GAG) side-chains of CSPGs (Anderson et al., 2016; Burnside et al., 2018; Bradbury and Burnside, 2019).
The glial reaction to trauma extends rostro-caudal from the astroglial scar into the perilesional zone of spared neural tissue. Within this region, astrocytes, microglia, and NG2-OPC induce spontaneous synapse and circuit reorganization. In contrast to the newly formed astrocytes in the astroglial scar border, hypertrophic reactive astrocytes in the perilesional zone maintain their connections with local neurons (Khakh and Sofroniew, 2015). The activity of reactive glial cells gradually reduces and transitions into healthy spinal cord tissue. Synapse loss in the perilesional zone spontaneously leads to formation of new synapses. Damaged axons either retract or sprout and form new synapses around the lesion core through the spared neural tissue (Figure 2). Axonal sprouting in propriospinal interneurons has the ability to form a detour around the lesion core, and is a likely mechanism for endogenous behavioural improvement following incomplete traumatic SCI (Bareyre et al., 2004; Ballermann and Fouad, 2006; Courtine et al., 2008; Takeoka et al., 2014). This is particularly evident in the C2 hemisection SCI model aimed at investigating respiratory dysfunction after injury. Within this model, respiration is restored via the activity of propriospinal interneurons that form new connections, referred to as crossed phrenic phenomenon (CPP).
Synaptopathy Hypothesis
The International Campaign for Cures of Spinal Cord Injury Paralysis (ICCP)—tasked at reviewing SCI clinical trials in 2007—concluded that most SCI patients suffer from a complete functional loss distal to the injury level (Grade A by the American Spinal Injury Association; ASIA). These patients show minimal recovery after 1 year, despite having a rim of preserved neural tissue around the injury site. This suggests that either remyelination or synaptic readjustment can elicit improved recovery. Surprisingly, all other ASIA classifications (Grades B, C, and D) demonstrate behavioral improvements, but the majority of Grade A patients do not exhibit any improvements 1-year post-injury (Adams and Cavanagh, 2004; Fawcett et al., 2007; Steeves et al., 2007; Tuszynski et al., 2007). In the post-ICCP era, the Spinal Trials Understanding, Design, and Implementation (STUDI) group—summoned in 2018—identified the role of combinatorial and rehabilitative trainings in ongoing clinical trials (Curt, 2019). This highlights the potential for neuroplasticity following injury and illustrates the growing interest in utilizing the neuroplastic potential of the spinal cord to treat SCI patients.
The synaptopathy hypothesis of SCI combines the recent findings from our lab and others, which suggests that traumatic SCI disrupts the preserved synaptic connections amongst the spinal interneurons. Despite the adaptive role of axonal sprouting in endogenous recovery, overwhelming evidence suggests neurotransmitter imbalance following SCI in the perilesional area results in an imbalanced excitatory/inhibitory (E/I) ratio, leading to the functional inactivation of preserved tissue (Chen et al., 2018). Synaptopathy is defined as maladaptive synaptic alterations, which lead to dysfunctional neurocircuitry. Various neurological conditions, such as autism spectrum disorder (ASD), schizophrenia, epilepsy, and Alzheimer’s disease have been linked to synaptic alterations (Ko et al., 2015).
Electrophysiological analyses using in vivo whole-cell patch clamp in a rat hemisection model demonstrate elevated spontaneous action potential firing in caudal substantia gelatinosa neurons following injury (Kozuka et al., 2016). While the mechanism remains to be fully elucidated, this hyperexcitation suggests the elimination of tonic descending control of inhibitory spinal interneurons (Kozuka et al., 2016). Interestingly, rehabilitative and neuromodulatory treatments aimed at exploiting synaptic changes after injury can activate dormant neural pathways (Petruska et al., 2007; Darrow et al., 2019; Kobayakawa et al., 2019). Transcriptional analysis in injured rats demonstrates the differential expression of synaptic genes following rehabilitative training (Kobayakawa et al., 2019). A recent study utilizing combinatorial rehabilitative training and Epidural stimulation (EDS) demonstrated that complete SCI patients, classified as grade A under ASIA score, were able to regain walking ability following several treatment sessions. Surprisingly, the patients lose their functional improvements following the cessation of the EDS. This is indicative of the beneficial role of neuromodulatory treatments to balance neurotransmission and activate dormant circuits after injury (Angeli et al., 2018).
Irregular neurotransmitter production, clearance, and sensitivity are potential mechanisms for maladaptive synaptic changes following traumatic SCI (Liu et al., 2004; Huang et al., 2016). For instance, altered serotonin regulation following injury is attributed to functional deficits, such as spasms (Thaweerattanasinp et al., 2020). Additionally, increased clustering of 5-HT2C receptors on V2a interneurons results in the super-sensitivity of neurons to serotonin following SCI (Husch et al., 2012).
A subtype of synaptopathy is channelopathy—the improper function of ion channels—which are critical for synaptic transmission and signal propagation in neural tissue (Hanna, 2006). Traumatic SCI alters the expression of ligand-gated channels, which in turn fluctuates the physiology of synapses in spinal interneurons. For instance, K+/Cl− cotransporter type 2 (KCC2) is a neuron specific transmembrane protein responsible for maintaining chloride and potassium ion gradients in the synaptic cleft (Moore et al., 2019). Previous studies have demonstrated the involvement of KCC2 in SCI pathogenesis. Expressed by SLC12A5, reduced KCC2 expression after injury lowers the concentration of chloride ions in the extracellular matrix (Boulenguez et al., 2010). The opening of chloride channels by GABA will induce chloride outflux from cells, which induces an excitatory effect. Such synaptic change upstream of inhibitory interneurons results in increased inhibition after SCI, which impairs signal transduction across spared neural tissue (Chen et al., 2018).
Spinal Interneurons as a Therapeutic Target After SCI
Therapeutic interneuronal modulation seeks to either reverse adverse maladaptive synaptic changes following injury or form new circuits to bypass the damaged injury site. The clinical translation of such therapeutics can restore vital autonomic functions, such as respiration, after high cervical injuries (Golder and Mitchell, 2005; Satkunendrarajah et al., 2018). In preclinical studies, the staggered double hemisections (SDH) SCI model enables the examination of interneuronal connections in the absence of supraspinal inputs (Figure 3). This model suspends all supraspinal inputs by two contralateral hemisection incisions, but spares relay circuits in between the incision sites (Laliberte et al., 2019). Various types of studies on the SDH model have developed therapeutics to target relay circuits and interneurons. Thus far, these include EDS, rehabilitative training, as well as molecular interventions via pharmacological and gene therapy approaches.
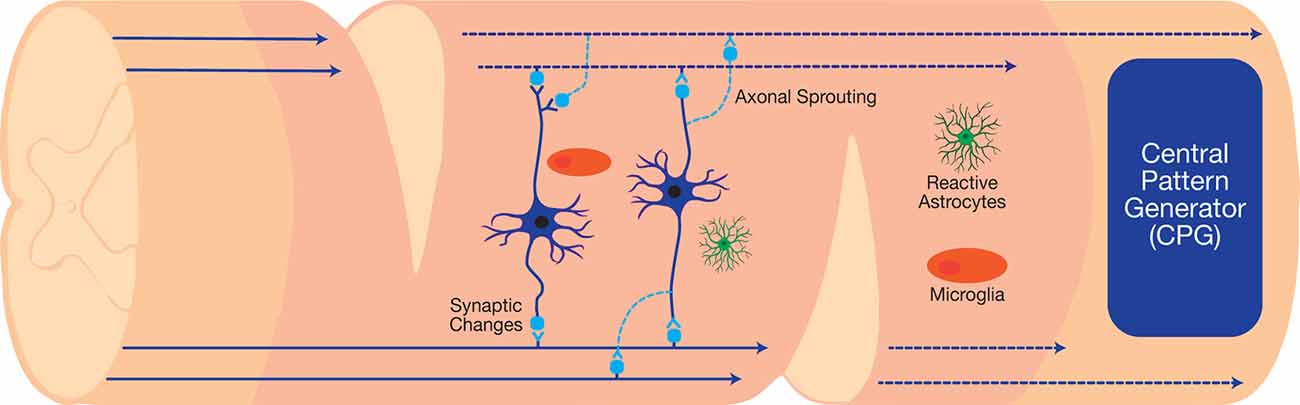
Figure 3. The staggered double hemisections (SDH) model interrupts all supraspinal connections while sparing spinal interneurons, which can be used to investigate neuroplasticity after SCI.
Epidural Stimulation and Physical Rehabilitation
EDS refers to the introduction of electrical current via an electrode implanted over dura. Combined electrochemical neuroprosthesis and rehabilitation through treadmill-aid/robotic postural interface enabled the cortical control of locomotion through spared neural tissue (Lavrov et al., 2008; Angeli et al., 2018; Capogrosso et al., 2018). While effective in reactivating locomotor circuitry, this stimulation is not exclusive to spinal interneurons—but recent work has shown that the activation of sensory afferents recruits interneurons and motoneurons to produce coordinated hindlimb stepping (Formento et al., 2018). This, in conjunction with the functional recovery gained after SDH from the use of a pharmacological agent—quipazine—that directly targets propriospinal interneurons, is compelling evidence that propriospinal relay is critical for EDS-mediated functional recovery (Gerasimenko et al., 2007).
Pharmacological and Gene Therapy Approaches
The currently evolving gene therapeutic techniques, as well as pharmacological interventions, demonstrate new ways to influence neuroplastic change in spinal interneurons (Dimidschstein et al., 2016; Wang et al., 2019). These techniques are particularly crucial for restoring breathing following damage to the phrenic circuit. For instance, the pharmacogenetic stimulation of mid-cervical excitatory interneurons demonstrates improved breathing in mice after C2 hemisection injury with disrupted ipsilateral bulbospinal connections (Satkunendrarajah et al., 2018). In parallel, an alternative approach is to restore E/I balance amongst the interneurons to mitigate maladaptive synaptic alterations after injury. Particularly, virally-induced episomal expression of KCC2 in GABAergic neurons significantly improves the activity of propriospinal interneurons between the SDH sites and augments hindlimb functional recovery (Chen et al., 2018). Similarly, the administration of either the glycinergic antagonist strychnine or the GABAA receptor antagonist bicuculline improves stepping in the absence of rehabilitative training (Robinson and Goldberger, 1986; de Leon et al., 1999). While these aforementioned studies targeted the cervical and thoracic regions of the spinal cord, studies that investigated the role of interneurons below in the lumbar region using optogenetics demonstrated that V2A (Ljunggren et al., 2014) and Shox2+ (Dougherty et al., 2013) interneurons are critical for stepping and rhythmogenesis within the lumbar locomotor CPGs. Taken together, these studies demonstrate that both activation of excitatory and modulation of inhibitory interneurons may be critical for functional recovery.
Conclusions
The current clinical practice for traumatic SCI is limited to prevention and early neuroprotection (Chio et al., 2019). Several emerging treatment strategies, such as anti-NOGO treatment (currently at phase 2/3 clinical trial) are being developed (Kucher et al., 2018). These treatments are able to improve behavioral outcome measures, but not fully recover lost function. The heterogeneity of SCI warrants the development of combinatorial approaches to treat SCI patients. An important consideration in such studies is the role of interneurons and synaptic modifications to activate preserved host tissue. Further investigations into the mechanisms behind synaptic changes in the injured spinal cord will improve the available treatment strategies for SCI patients.
Author Contributions
M-MZ and JH: literature review, manuscript writing, editing, and finalizing, approval of the final manuscript. MF: framing the concept and structure of the review, editing, and finalizing, approval of the final manuscript.
Funding
This work was supported by the Cervical Spine Research Society (MF). M-MZ was awarded the Ontario Graduate Scholarship (OGS).
Conflict of Interest
The authors declare that the research was conducted in the absence of any commercial or financial relationships that could be construed as a potential conflict of interest.
Acknowledgments
We are appreciative of Dr. Tim Worden for copyediting the manuscript. MF would like to acknowledge support from the Halbert Chair in Neural Repair and Regeneration and the Krembil Foundation.
References
Adams, M., and Cavanagh, J. F. R. (2004). International Campaign for Cures of Spinal Cord Injury Paralysis (ICCP): another step forward for spinal cord injury research. Spinal Cord 42, 273–280. doi: 10.1038/sj.sc.3101597
Ahuja, C. S., Wilson, J. R., Nori, S., Kotter, M. R. N., Druschel, C., Curt, A., et al. (2017). Traumatic spinal cord injury. Nat. Rev. Dis. Primers 3:17018. doi: 10.1038/nrdp.2017.18
Anderson, M. A., Burda, J. E., Ren, Y., Ao, Y., O’Shea, T. M., Kawaguchi, R., et al. (2016). Astrocyte scar formation aids central nervous system axon regeneration. Nature 532, 195–200. doi: 10.1038/nature17623
Andersson, L. S., Larhammar, M., Memic, F., Wootz, H., Schwochow, D., Rubin, C.-J., et al. (2012). Mutations in DMRT3 affect locomotion in horses and spinal circuit function in mice. Nature 488, 642–646. doi: 10.1038/nature11399
Angeli, C. A., Boakye, M., Morton, R. A., Vogt, J., Benton, K., Chen, Y., et al. (2018). Recovery of over-ground walking after chronic motor complete spinal cord injury. N. Engl. J. Med. 379, 1244–1250. doi: 10.1056/NEJMoa1803588
Armour, B. S., Courtney-Long, E. A., Fox, M. H., Fredine, H., and Cahill, A. (2016). Prevalence and causes of paralysis—united states, 2013. Am. J. Public Health 106, 1855–1857. doi: 10.2105/AJPH.2016.303270
Avraham, O., Hadas, Y., Vald, L., Zisman, S., Schejter, A., Visel, A., et al. (2009). Transcriptional control of axonal guidance and sorting in dorsal interneurons by the Lim-HD proteins Lhx9 and Lhx1. Neural Dev. 4:21. doi: 10.1186/1749-8104-4-21
Badhiwala, J. H., Ahuja, C. S., Akbar, M. A., Witiw, C. D., Nassiri, F., Furlan, J. C., et al. (2020). Degenerative cervical myelopathy—update and future directions. Nat. Rev. Neurol. 16, 108–124. doi: 10.1038/s41582-019-0303-0
Baker-Herman, T. L., Fuller, D. D., Bavis, R. W., Zabka, A. G., Golder, F. J., Doperalski, N. J., et al. (2004). BDNF is necessary and sufficient for spinal respiratory plasticity following intermittent hypoxia. Nat. Neurosci. 7, 48–55. doi: 10.1038/nn1166
Ballermann, M., and Fouad, K. (2006). Spontaneous locomotor recovery in spinal cord injured rats is accompanied by anatomical plasticity of reticulospinal fibers. Eur. J. Neurosci. 23, 1988–1996. doi: 10.1111/j.1460-9568.2006.04726.x
Bareyre, F. M., Kerschensteiner, M., Raineteau, O., Mettenleiter, T. C., Weinmann, O., and Schwab, M. E. (2004). The injured spinal cord spontaneously forms a new intraspinal circuit in adult rats. Nat. Neurosci. 7, 269–277. doi: 10.1038/nn1195
Barnabé-Heider, F., Göritz, C., Sabelström, H., Takebayashi, H., Pfrieger, F. W., Meletis, K., et al. (2010). Origin of new glial cells in intact and injured adult spinal cord. Cell Stem Cell 7, 470–482. doi: 10.1016/j.stem.2010.07.014
Bikoff, J. B., Gabitto, M. I., Rivard, A. F., Drobac, E., Machado, T. A., Miri, A., et al. (2016). Spinal inhibitory interneuron diversity delineates variant motor microcircuits. Cell 165, 207–219. doi: 10.1016/j.cell.2016.01.027
Blesch, A., and Tuszynski, M. H. (2009). Spinal cord injury: plasticity, regeneration and the challenge of translational drug development. Trends Neurosci. 32, 41–47. doi: 10.1016/j.tins.2008.09.008
Boulenguez, P., Liabeuf, S., Bos, R., Bras, H., Jean-Xavier, C., Brocard, C., et al. (2010). Down-regulation of the potassium-chloride cotransporter KCC2 contributes to spasticity after spinal cord injury. Nat. Med. 16, 302–307. doi: 10.1038/nm.2107
Bradbury, E. J., and Burnside, E. R. (2019). Moving beyond the glial scar for spinal cord repair. Nat. Commun. 10:3879. doi: 10.1038/s41467-019-11707-7
Brown, G. (1914). On the nature of the fundamental activity of the nervous centres. J. Physiol. 48, 18–46. doi: 10.1113/jphysiol.1914.sp001646
Bui, T. V., Akay, T., Loubani, O., Hnasko, T. S., Jessell, T. M., and Brownstone, R. M. (2013). Circuits for grasping: spinal dI3 interneurons mediate cutaneous control of motor behavior. Neuron 78, 191–204. doi: 10.1016/j.neuron.2013.02.007
Burke, R. E. (2006). Sir Charles Sherrington’s the integrative action of the nervous system: a centenary appreciation. Brain 130, 887–894. doi: 10.1093/brain/awm022
Burnside, E. R., De Winter, F., Didangelos, A., James, N. D., Andreica, E.-C., Layard-Horsfall, H., et al. (2018). Immune-evasive gene switch enables regulated delivery of chondroitinase after spinal cord injury. Brain 141, 2362–2381. doi: 10.1093/brain/awy158
Cadotte, D. W., Bosma, R., Mikulis, D., Nugaeva, N., Smith, K., Pokrupa, R., et al. (2012). Plasticity of the injured human spinal cord: insights revealed by spinal cord functional MRI. PLoS One 7:e45560. doi: 10.1371/journal.pone.0045560
Cadotte, D. W., and Fehlings, M. G. (2013). Visualizing plasticity and repair in the injured CNS. Nat. Rev. Neurol. 9, 546–547. doi: 10.1038/nrneurol.2013.190
Capogrosso, M., Wagner, F. B., Gandar, J., Moraud, E. M., Wenger, N., Milekovic, T., et al. (2018). Configuration of electrical spinal cord stimulation through real-time processing of gait kinematics. Nat. Protoc. 13, 2031–2061. doi: 10.1038/s41596-018-0030-9
Chen, B., Li, Y., Yu, B., Zhang, Z., Brommer, B., Williams, P. R., et al. (2018). Reactivation of dormant relay pathways in injured spinal cord by KCC2 manipulations. Cell 174, 521.e13–535.e13. doi: 10.1016/j.cell.2018.06.005
Chio, J. C. T., Wang, J., Badner, A., Hong, J., Surendran, V., and Fehlings, M. G. (2019). The effects of human immunoglobulin G on enhancing tissue protection and neurobehavioral recovery after traumatic cervical spinal cord injury are mediated through the neurovascular unit. J. Neuroinflammation 16:141. doi: 10.1186/s12974-019-1518-0
Chopek, J. W., Nascimento, F., Beato, M., Brownstone, R. M., and Zhang, Y. (2018). Sub-populations of spinal V3 interneurons form focal modules of layered pre-motor microcircuits. Cell Rep. 25, 146.e3–156.e3. doi: 10.1016/j.celrep.2018.08.095
Courtine, G., and Sofroniew, M. V. (2019). Spinal cord repair: advances in biology and technology. Nat. Med. 25, 898–908. doi: 10.1038/s41591-019-0475-6
Courtine, G., Song, B., Roy, R. R., Zhong, H., Herrmann, J. E., Ao, Y., et al. (2008). Recovery of supraspinal control of stepping via indirect propriospinal relay connections after spinal cord injury. Nat. Med. 14, 69–74. doi: 10.1038/nm1682
Crone, C., Hultborn, H., Jespersen, B., and Nielsen, J. (1987). Reciprocal Ia inhibition between ankle flexors and extensors in man. J. Physiol. 389, 163–185. doi: 10.1113/jphysiol.1987.sp016652
Crone, S. A., Quinlan, K. A., Zagoraiou, L., Droho, S., Restrepo, C. E., Lundfald, L., et al. (2008). Genetic ablation of V2a ipsilateral interneurons disrupts left-right locomotor coordination in mammalian spinal cord. Neuron 60, 70–83. doi: 10.1016/j.neuron.2008.08.009
Crone, S. A., Zhong, G., Harris-Warrick, R., and Sharma, K. (2009). In mice lacking V2a interneurons, gait depends on speed of locomotion. J. Neurosci. 29, 7098–7109. doi: 10.1523/JNEUROSCI.1206-09.2009
Danner, S. M., Zhang, H., Shevtsova, N. A., Borowska-Fielding, J., Deska-Gauthier, D., Rybak, I. A., et al. (2019). Spinal V3 interneurons and left-right coordination in mammalian locomotion. Front. Cell. Neurosci. 13:516. doi: 10.3389/fncel.2019.00516
Darrow, D., Balser, D., Netoff, T. I., Krassioukov, A., Phillips, A., Parr, A., et al. (2019). Epidural spinal cord stimulation facilitates immediate restoration of dormant motor and autonomic supraspinal pathways after chronic neurologically complete spinal cord injury. J. Neurotrauma 36, 2325–2336. doi: 10.1089/neu.2018.6006
de Leon, R. D., Tamaki, H., Hodgson, J. A., Roy, R. R., and Edgerton, V. R. (1999). Hindlimb locomotor and postural training modulates glycinergic inhibition in the spinal cord of the adult spinal cat. J. Neurophysiol. 82, 359–369. doi: 10.1152/jn.1999.82.1.359
Delile, J., Rayon, T., Melchionda, M., Edwards, A., Briscoe, J., and Sagner, A. (2019). Single cell transcriptomics reveals spatial and temporal dynamics of gene expression in the developing mouse spinal cord. Development 146:dev173807. doi: 10.1242/dev.173807
Deska-Gauthier, D., Borowska-Fielding, J., Jones, C. T., and Zhang, Y. (2020). The temporal neurogenesis patterning of spinal p3–V3 interneurons into divergent subpopulation assemblies. J. Neurosci. 40, 1440–1452. doi: 10.1523/JNEUROSCI.1518-19.2019
Dimidschstein, J., Chen, Q., Tremblay, R., Rogers, S. L., Saldi, G.-A., Guo, L., et al. (2016). A viral strategy for targeting and manipulating interneurons across vertebrate species. Nat. Neurosci. 19, 1743–1749. doi: 10.1038/nn.4430
Dougherty, K. J., and Kiehn, O. (2010). Firing and cellular properties of V2a interneurons in the rodent spinal cord. J. Neurosci. 30, 24–37. doi: 10.1523/JNEUROSCI.4821-09.2010
Dougherty, K. J., Zagoraiou, L., Satoh, D., Rozani, I., Doobar, S., Arber, S., et al. (2013). Locomotor rhythm generation linked to the output of spinal shox2 excitatory interneurons. Neuron 80, 920–933. doi: 10.1016/j.neuron.2013.08.015
Duval, N., Daubas, P., Bourcier de Carbon, C., St Cloment, C., Tinevez, J.-Y., Lopes, M., et al. (2014). Msx1 and Msx2 act as essential activators of Atoh1 expression in the murine spinal cord. Development 141, 1726–1736. doi: 10.1242/dev.099002
Falgairolle, M., and O’Donovan, M. J. (2019). V1 interneurons regulate the pattern and frequency of locomotor-like activity in the neonatal mouse spinal cord. PLoS Biol. 17:e3000447. doi: 10.1371/journal.pbio.3000447
Fawcett, J. W., Curt, A., Steeves, J. D., Coleman, W. P., Tuszynski, M. H., Lammertse, D., et al. (2007). Guidelines for the conduct of clinical trials for spinal cord injury as developed by the ICCP panel: spontaneous recovery after spinal cord injury and statistical power needed for therapeutic clinical trials. Spinal Cord 45, 190–205. doi: 10.1038/sj.sc.3102007
Fleming, J. C., Norenberg, M. D., Ramsay, D. A., Dekaban, G. A., Marcillo, A. E., Saenz, A. D., et al. (2006). The cellular inflammatory response in human spinal cords after injury. Brain 129, 3249–3269. doi: 10.1093/brain/awl296
Flynn, J. R., Conn, V. L., Boyle, K. A., Hughes, D. I., Watanabe, M., Velasquez, T., et al. (2017). Anatomical and molecular properties of long descending propriospinal neurons in mice. Front. Neuroanat. 11:5. doi: 10.3389/fnana.2017.00005
Flynn, J. R., Graham, B. A., Galea, M. P., and Callister, R. J. (2011). The role of propriospinal interneurons in recovery from spinal cord injury. Neuropharmacology 60, 809–822. doi: 10.1016/j.neuropharm.2011.01.016
Formento, E., Minassian, K., Wagner, F., Mignardot, J. B., Le Goff-Mignardot, C. G., Rowald, A., et al. (2018). Electrical spinal cord stimulation must preserve proprioception to enable locomotion in humans with spinal cord injury. Nat. Neurosci. 21, 1728–1741. doi: 10.1038/s41593-018-0262-6
Gerasimenko, Y. P., Ichiyama, R. M., Lavrov, I. A., Courtine, G., Cai, L., Zhong, H., et al. (2007). Epidural spinal cord stimulation plus quipazine administration enable stepping in complete spinal adult rats. J. Neurophysiol. 98, 2525–2536. doi: 10.1152/jn.00836.2007
Golder, F. J., and Mitchell, G. S. (2005). Spinal synaptic enhancement with acute intermittent hypoxia improves respiratory function after chronic cervical spinal cord injury. J. Neurosci. 25, 2925–2932. doi: 10.1523/JNEUROSCI.0148-05.2005
Gosgnach, S., Bikoff, J. B., Dougherty, K. J., El Manira, A., Lanuza, G. M., and Zhang, Y. (2017). Delineating the diversity of spinal interneurons in locomotor circuits. J. Neurosci. 37, 10835–10841. doi: 10.1523/JNEUROSCI.1829-17.2017
Gosgnach, S., Lanuza, G. M., Butt, S. J. B., Saueressig, H., Zhang, Y., Velasquez, T., et al. (2006). V1 spinal neurons regulate the speed of vertebrate locomotor outputs. Nature 440, 215–219. doi: 10.1038/nature04545
Goulding, M. (2009). Circuits controlling vertebrate locomotion: moving in a new direction. Nat. Rev. Neurosci. 10, 507–518. doi: 10.1038/nrn2608
Gowan, K., Helms, A. W., Hunsaker, T. L., Collisson, T., Ebert, P. J., Odom, R., et al. (2001). Crossinhibitory activities of Ngn1 and Math1 allow specification of distinct dorsal Interneurons. Neuron 31, 219–232. doi: 10.1016/s0896-6273(01)00367-1
Gross, M. K., Dottori, M., and Goulding, M. (2002). Lbx1 specifies somatosensory association interneurons in the dorsal spinal cord. Neuron 34, 535–549. doi: 10.1016/s0896-6273(02)00690-6
Guertin, P. A. (2013). Central pattern generator for locomotion: anatomical, physiological, and pathophysiological considerations. Front. Neurol. 3:183. doi: 10.3389/fneur.2012.00183
Hanna, M. G. (2006). Genetic neurological channelopathies. Nat. Rev. Neurol. 2, 252–263. doi: 10.1038/ncpneuro0178
Harkema, S. J. (2008). Plasticity of interneuronal networks of the functionally isolated human spinal cord. Brain Res. Rev. 57, 255–264. doi: 10.1016/j.brainresrev.2007.07.012
Harris, A., Masgutova, G., Collin, A., Toch, M., Hidalgo-Figueroa, M., Jacob, B., et al. (2019). Onecut factors and Pou2f2 regulate the distribution of V2 interneurons in the mouse developing spinal cord. Front. Cell. Neurosci. 13:184. doi: 10.3389/fncel.2019.00184
Hayashi, M., Hinckley, C. A., Driscoll, S. P., Moore, N. J., Levine, A. J., Hilde, K. L., et al. (2018). Graded arrays of spinal and supraspinal V2a interneuron subtypes underlie forelimb and hindlimb motor control. Neuron 97, 869.e5–884.e5. doi: 10.1016/j.neuron.2018.01.023
Huang, Y.-J., Lee, K. H., Murphy, L., Garraway, S. M., and Grau, J. W. (2016). Acute spinal cord injury (SCI) transforms how GABA affects nociceptive sensitization. Exp. Neurol. 285, 82–95. doi: 10.1016/j.expneurol.2016.09.005
Husch, A., Van Patten, G. N., Hong, D. N., Scaperotti, M. M., Cramer, N., and Harris-Warrick, R. M. (2012). Spinal cord injury induces serotonin supersensitivity without increasing intrinsic excitability of mouse V2a interneurons. J. Neurosci. 32, 13145–13154. doi: 10.1523/JNEUROSCI.2995-12.2012
Hutson, T. H., and Di Giovanni, S. (2019). The translational landscape in spinal cord injury: focus on neuroplasticity and regeneration. Nat. Rev. Neurol. 15, 732–745. doi: 10.1038/s41582-019-0280-3
Jankowska, E. (2001). Spinal interneuronal systems: identification, multifunctional character and reconfigurations in mammals. J. Physiol. 533, 31–40. doi: 10.1111/j.1469-7793.2001.0031b.x
Jessell, T. M. (2000). Neuronal specification in the spinal cord: inductive signals and transcriptional codes. Nat. Rev. Genet. 1, 20–29. doi: 10.1038/35049541
Jones, L. L., Yamaguchi, Y., Stallcup, W. B., and Tuszynski, M. H. (2002). NG2 is a major chondroitin sulfate proteoglycan produced after spinal cord injury and is expressed by macrophages and oligodendrocyte progenitors. J. Neurosci. 22, 2792–2803. doi: 10.1523/JNEUROSCI.22-07-02792.2002
Katz, R., Penicaud, A., and Rossi, A. (1991). Reciprocal Ia inhibition between elbow flexors and extensors in the human. J. Physiol. 437, 269–286. doi: 10.1113/jphysiol.1991.sp018595
Khakh, B. S., and Sofroniew, M. V. (2015). Diversity of astrocyte functions and phenotypes in neural circuits. Nat. Neurosci. 18, 942–952. doi: 10.1038/nn.4043
Kiehn, O. (2016). Decoding the organization of spinal circuits that control locomotion. Nat. Rev. Neurosci. 17, 224–238. doi: 10.1038/nrn.2016.9
Kimura, Y., and Higashijima, S. (2019). Regulation of locomotor speed and selection of active sets of neurons by V1 neurons. Nat. Commun. 10:2268. doi: 10.1038/s41467-019-09871-x
Ko, J., Choii, G., and Um, J. W. (2015). The balancing act of GABAergic synapse organizers. Trends Mol. Med. 21, 256–268. doi: 10.1016/j.molmed.2015.01.004
Kobayakawa, K., DePetro, K. A., Zhong, H., Pham, B., Hara, M., Harada, A., et al. (2019). Locomotor training increases synaptic structure with high NGL-2 expression after spinal cord hemisection. Neurorehabil. Neural Repair 33, 225–231. doi: 10.1177/1545968319829456
Kozuka, Y., Kawamata, M., Furue, H., Ishida, T., Tanaka, S., Namiki, A., et al. (2016). Changes in synaptic transmission of substantia gelatinosa neurons after spinal cord hemisection revealed by analysis using in vivo patch-clamp recording. Mol. Pain 12:174480691666582. doi: 10.1177/1744806916665827
Kucher, K., Johns, D., Maier, D., Abel, R., Badke, A., Baron, H., et al. (2018). First-in-man intrathecal application of neurite growth-promoting anti-nogo-a antibodies in acute spinal cord injury. Neurorehabil. Neural Repair 32, 578–589. doi: 10.1177/1545968318776371
Ladle, D. R., Pecho-Vrieseling, E., and Arber, S. (2007). Assembly of motor circuits in the spinal cord: driven to function by genetic and experience-dependent mechanisms. Neuron 56, 270–283. doi: 10.1016/j.neuron.2007.09.026
Laliberte, A. M., Goltash, S., Lalonde, N. R., and Bui, T. V. (2019). Propriospinal neurons: essential elements of locomotor control in the intact and possibly the injured spinal cord. Front. Cell. Neurosci. 13:512. doi: 10.3389/fncel.2019.00512
Lanuza, G. M., Gosgnach, S., Pierani, A., Jessell, T. M., and Goulding, M. (2004). Genetic identification of spinal interneurons that coordinate left-right locomotor activity necessary for walking movements. Neuron 42, 375–386. doi: 10.1016/s0896-6273(04)00249-1
Lavrov, I., Courtine, G., Dy, C. J., van den Brand, R., Fong, A. J., Gerasimenko, Y., et al. (2008). Facilitation of stepping with epidural stimulation in spinal rats: role of sensory input. J. Neurosci. 28, 7774–7780. doi: 10.1523/JNEUROSCI.1069-08.2008
Liu, J., Wolfe, D., Hao, S., Huang, S., Glorioso, J. C., Mata, M., et al. (2004). Peripherally delivered glutamic acid decarboxylase gene therapy for spinal cord injury pain. Mol. Ther. 10, 57–66. doi: 10.1016/j.ymthe.2004.04.017
Ljunggren, E. E., Haupt, S., Ausborn, J., Ampatzis, K., and Manira, A. E. (2014). Optogenetic activation of excitatory premotor interneurons is sufficient to generate coordinated locomotor activity in larval zebrafish. J. Neurosci. 34, 134–139. doi: 10.1523/JNEUROSCI.4087-13.2014
Lu, D. C., Niu, T., and Alaynick, W. A. (2015). Molecular and cellular development of spinal cord locomotor circuitry. Front. Mol. Neurosci. 8:25. doi: 10.3389/fnmol.2015.00025
Meletis, K., Barnabé-Heider, F., Carlén, M., Evergren, E., Tomilin, N., Shupliakov, O., et al. (2008). Spinal cord injury reveals multilineage differentiation of ependymal cells. PLoS Biol. 6:e182. doi: 10.1371/journal.pbio.0060182
Menelaou, E., and McLean, D. L. (2019). Hierarchical control of locomotion by distinct types of spinal V2a interneurons in zebrafish. Nat. Commun. 10:4197. doi: 10.1038/s41467-019-12240-3
Mizuguchi, R., Kriks, S., Cordes, R., Gossler, A., Ma, Q., and Goulding, M. (2006). Ascl1 and Gsh1/2 control inhibitory and excitatory cell fate in spinal sensory interneurons. Nat. Neurosci. 9, 770–778. doi: 10.1038/nn1706
Moore, Y. E., Conway, L. C., Wobst, H. J., Brandon, N. J., Deeb, T. Z., and Moss, S. J. (2019). Developmental regulation of KCC2 phosphorylation has long-term impacts on cognitive function. Front. Mol. Neurosci. 12:173. doi: 10.3389/fnmol.2019.00173
Moran-Rivard, L., Kagawa, T., Saueressig, H., Gross, M. K., Burrill, J., and Goulding, M. (2001). Evx1 is a postmitotic determinant of V0 interneuron identity in the spinal cord. Neuron 29, 385–399. doi: 10.1016/s0896-6273(01)00213-6
National Spinal Cord Injury Statistical Center. (2020). Spinal Cord Injury, Facts and Figures at a Glance. Birmingham: University of Alabama at Birmingham.
O’Shea, T. M., Burda, J. E., and Sofroniew, M. V. (2017). Cell biology of spinal cord injury and repair. J. Clin. Invest. 127, 3259–3270. doi: 10.1172/JCI90608
Ogura, T., Sakaguchi, H., Miyamoto, S., and Takahashi, J. (2018). Three-dimensional induction of dorsal, intermediate and ventral spinal cord tissues from human pluripotent stem cells. Development 145:dev162214. doi: 10.1242/dev.162214
Özyurt, M. G., Piotrkiewicz, M., Topkara, B., Weisskircher, H., and Türker, K. S. (2019). Motor units as tools to evaluate profile of human Renshaw inhibition. J. Physiol. 597, 2185–2199. doi: 10.1113/jp277129
Petruska, J. C., Ichiyama, R. M., Jindrich, D. L., Crown, E. D., Tansey, K. E., Roy, R. R., et al. (2007). Changes in motoneuron properties and synaptic inputs related to step training after spinal cord transection in rats. J. Neurosci. 27, 4460–4471. doi: 10.1523/JNEUROSCI.2302-06.2007
Pierani, A., Moran-Rivard, L., Sunshine, M. J., Littman, D. R., Goulding, M., and Jessell, T. M. (2001). Control of interneuron fate in the developing spinal cord by the progenitor homeodomain protein Dbx1. Neuron 29, 367–384. doi: 10.1016/s0896-6273(01)00212-4
Raineteau, O., and Schwab, M. E. (2001). Plasticity of motor systems after incomplete spinal cord injury. Nat. Rev. Neurosci. 2, 263–273. doi: 10.1038/35067570
Robinson, G. A., and Goldberger, M. E. (1986). The development and recovery of motor function in spinal cats. II. Pharmacological enhancement of recovery. Exp. Brain Res. 62, 387–400. doi: 10.1007/bf00238858
Rybak, I. A., Stecina, K., Shevtsova, N. A., and McCrea, D. A. (2006). Modelling spinal circuitry involved in locomotor pattern generation: insights from the effects of afferent stimulation. J. Physiol. 577, 641–658. doi: 10.1113/jphysiol.2006.118711
Saliani, A., Perraud, B., Duval, T., Stikov, N., Rossignol, S., and Cohen-Adad, J. (2017). Axon and myelin morphology in animal and human spinal cord. Front. Neuroanat. 11:129. doi: 10.3389/fnana.2017.00129
Sathyamurthy, A., Johnson, K. R., Matson, K. J. E., Dobrott, C. I., Li, L., Ryba, A. R., et al. (2018). Massively parallel single nucleus transcriptional profiling defines spinal cord neurons and their activity during behavior. Cell Rep. 22, 2216–2225. doi: 10.1016/j.celrep.2018.02.003
Satkunendrarajah, K., Karadimas, S. K., Laliberte, A. M., Montandon, G., and Fehlings, M. G. (2018). Cervical excitatory neurons sustain breathing after spinal cord injury. Nature 562, 419–422. doi: 10.1038/s41586-018-0595-z
Schnerwitzki, D., Perry, S., Ivanova, A., Caixeta, F. V., Cramer, P., Günther, S., et al. (2018). Neuron-specific inactivation of Wt1 alters locomotion in mice and changes interneuron composition in the spinal cord. Life Sci. Alliance 1:e201800106. doi: 10.26508/lsa.201800106
Sekhon, L. H. S., and Fehlings, M. G. (2001). Epidemiology, demographics, and pathophysiology of acute spinal cord injury. Spine 26, S2–S12. doi: 10.1097/00007632-200112151-00002
Sekiguchi, K. J., Shekhtmeyster, P., Merten, K., Arena, A., Cook, D., Hoffman, E., et al. (2016). Imaging large-scale cellular activity in spinal cord of freely behaving mice. Nat. Commun. 7:11450. doi: 10.1038/ncomms11450
Silver, D. J., and Silver, J. (2014). Contributions of chondroitin sulfate proteoglycans to neurodevelopment, injury, and cancer. Curr. Opin. Neurobiol. 27, 171–178. doi: 10.1016/j.conb.2014.03.016
Sofroniew, M. V. (2018). Dissecting spinal cord regeneration. Nature 557, 343–350. doi: 10.1038/s41586-018-0068-4
Steeves, J. D., Lammertse, D., Curt, A., Fawcett, J. W., Tuszynski, M. H., Ditunno, J. F., et al. (2007). Guidelines for the conduct of clinical trials for spinal cord injury (SCI) as developed by the ICCP panel: clinical trial outcome measures. Spinal Cord 45, 206–221. doi: 10.1038/sj.sc.3102010
Stepien, A. E., Tripodi, M., and Arber, S. (2010). Monosynaptic rabies virus reveals premotor network organization and synaptic specificity of cholinergic partition cells. Neuron 68, 456–472. doi: 10.1016/j.neuron.2010.10.019
Takeoka, A., Vollenweider, I., Courtine, G., and Arber, S. (2014). Muscle spindle feedback directs locomotor recovery and circuit reorganization after spinal cord injury. Cell 159, 1626–1639. doi: 10.1016/j.cell.2014.11.019
Talpalar, A. E., Bouvier, J., Borgius, L., Fortin, G., Pierani, A., and Kiehn, O. (2013). Dual-mode operation of neuronal networks involved in left-right alternation. Nature 500, 85–88. doi: 10.1038/nature12286
Tator, C., and Fehlings, M. G. (1991). Review of the secondary injury theory of acute spinal cord trauma with emphasis on vascular mechanisms. J. Neurosurg 75, 15–26. doi: 10.3171/jns.1991.75.1.0015
Thaweerattanasinp, T., Birch, D., Jiang, M. C., Tresch, M. C., Bennett, D. J., Heckman, C., et al. (2020). Bursting interneurons in the deep dorsal horn develop increased excitability and sensitivity to serotonin after chronic spinal injury. J. Neurophysiol. doi: 10.1152/jn.00701.2019 [Epub ahead of print].
Tuszynski, M. H., Steeves, J. D., Fawcett, J. W., Lammertse, D., Kalichman, M., Rask, C., et al. (2007). Guidelines for the conduct of clinical trials for spinal cord injury as developed by the ICCP Panel: clinical trial inclusion/exclusion criteria and ethics. Spinal Cord 45, 222–231. doi: 10.1038/sj.sc.3102009
Vanlandewijck, M., He, L., Mäe, M. A., Andrae, J., Ando, K., Del Gaudio, F., et al. (2018). A molecular atlas of cell types and zonation in the brain vasculature. Nature 554, 475–480. doi: 10.1038/nature25739
Wang, D., Tai, P. W. L., and Gao, G. (2019). Adeno-associated virus vector as a platform for gene therapy delivery. Nat. Rev. Drug Discov. 18, 358–378. doi: 10.1038/s41573-019-0012-9
Wilson, S. I., Shafer, B., Lee, K. J., and Dodd, J. (2008). A molecular program for contralateral trajectory: rig-1 control by LIM homeodomain transcription factors. Neuron 59, 413–424. doi: 10.1016/j.neuron.2008.07.020
Zagoraiou, L., Akay, T., Martin, J. F., Brownstone, R. M., Jessell, T. M., and Miles, G. B. (2009). A cluster of cholinergic premotor interneurons modulates mouse locomotor activity. Neuron 64, 645–662. doi: 10.1016/j.neuron.2009.10.017
Zeisel, A., Hochgerner, H., Lönnerberg, P., Johnsson, A., Memic, F., van der Zwan, J., et al. (2018). Molecular architecture of the mouse nervous system. Cell 174, 999.e22–1014.e22. doi: 10.1016/j.cell.2018.06.021
Zhang, J., Lanuza, G. M., Britz, O., Wang, Z., Siembab, V. C., Zhang, Y., et al. (2014). V1 and V2b interneurons secure the alternating flexor-extensor motor activity mice require for limbed locomotion. Neuron 82, 138–150. doi: 10.1016/j.neuron.2014.02.013
Zhang, Y., Narayan, S., Geiman, E., Lanuza, G. M., Velasquez, T., Shanks, B., et al. (2008). V3 spinal neurons establish a robust and balanced locomotor rhythm during walking. Neuron 60, 84–96. doi: 10.1016/j.neuron.2008.09.027
Keywords: spinal cord injury, interneurons, synaptopathy, synaptic connections, neuroplasticity
Citation: Zavvarian M-M, Hong J and Fehlings MG (2020) The Functional Role of Spinal Interneurons Following Traumatic Spinal Cord Injury. Front. Cell. Neurosci. 14:127. doi: 10.3389/fncel.2020.00127
Received: 06 March 2020; Accepted: 17 April 2020;
Published: 18 May 2020.
Edited by:
Junfang Wu, University of Maryland, Baltimore, United StatesReviewed by:
Shaoping Hou, Drexel University, United StatesTuan Vu Bui, University of Ottawa, Canada
Copyright © 2020 Zavvarian, Hong and Fehlings. This is an open-access article distributed under the terms of the Creative Commons Attribution License (CC BY). The use, distribution or reproduction in other forums is permitted, provided the original author(s) and the copyright owner(s) are credited and that the original publication in this journal is cited, in accordance with accepted academic practice. No use, distribution or reproduction is permitted which does not comply with these terms.
*Correspondence: Michael G. Fehlings, bWljaGFlbC5mZWhsaW5nc0B1aG4uY2E=