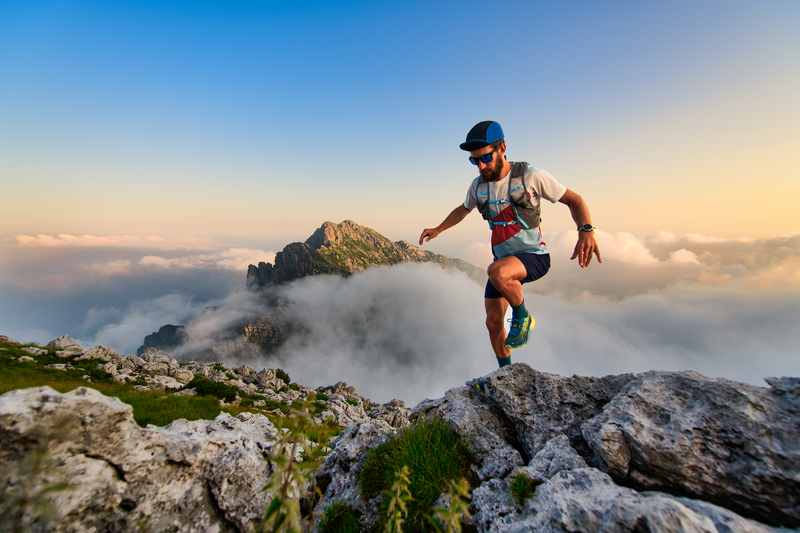
94% of researchers rate our articles as excellent or good
Learn more about the work of our research integrity team to safeguard the quality of each article we publish.
Find out more
MINI REVIEW article
Front. Cell. Neurosci. , 30 April 2020
Sec. Cellular Neurophysiology
Volume 14 - 2020 | https://doi.org/10.3389/fncel.2020.00111
This article is part of the Research Topic Novel Therapeutic Potential for Pituitary Adenylate Cyclase-Activating Polypeptide (PACAP), Vasoactive Intestinal Peptide (VIP) and related peptides in cognition deficits View all 10 articles
Pituitary adenylate cyclase-activating polypeptide (PACAP, ADCYAP1) dysregulation has been associated with multiple stress-related psychopathologies that may be related to altered hippocampal function. In coherence, PACAP- and PAC1 receptor (ADCYAP1R1)-null mice demonstrate changes in hippocampal-dependent behavioral responses, implicating the PACAPergic system function in this structure. Within the hippocampus, the dentate gyrus (DG) may play an important role in discerning the differences between similar contexts, and DG granule cells appear to both highly express PAC1 receptors and receive inputs from PACAP-expressing terminals. Here, we review the evidence from our laboratories and others that PACAP is an important regulator of activity within hippocampal circuits, particularly within the DG. These data are consistent with an increasing literature implicating PACAP circuits in stress-related pathologies such as post-traumatic stress disorder (PTSD) and implicate the hippocampus, and in particular the DG, as a critical site in which PACAP dysregulation can alter stress-related behaviors.
Pituitary adenylate cyclase-activating polypeptide (PACAP, ADCYAP1) dysregulation has been associated with multiple stress-related psychopathologies (Kormos and Gaszner, 2013; Hammack and May, 2015; Lutfy and Shankar, 2019) and we and others have reported that a single nucleotide polymorphism (SNP) in the PAC1 receptor gene (ADCYAP1R1 rs2267735) as well as circulating PACAP levels were associated with post-traumatic stress disorder (PTSD; Ressler et al., 2011). These findings were consistent with a growing literature demonstrating central PACAP signaling as critical for stress responding and fear- and anxiety-like behavior in rodents. PTSD is characterized by several features, which may represent dysregulation in several distinct neural circuits. Notably, in the original report, Ressler et al. (2011) reported that the risk allele of rs2267735 was associated with hyper-arousal and dark-enhanced startles, which are behaviors that have been closely associated with activation of subregions of the bed nucleus of the stria terminals (BNST). These observations have corroborated several years of work in our laboratories demonstrating that BNST PACAP activation is necessary and sufficient for many of the behavioral sequelae produced by chronic stress (Hammack et al., 2010; Hammack and May, 2015), as well as cocaine self-administration (Miles et al., 2018, 2019). However, dysregulated fear extinction and fear discrimination are also hallmark features of PTSD (see Jovanovic et al., 2012; Sangha et al., 2020), and rs2267735 was also associated with compromised extinction and reduced discrimination between fear-conditioned stimuli (Ressler et al., 2011; Pohlack et al., 2015; Mercer et al., 2016; Ramikie and Ressler, 2016). These data suggest that PACAP dysregulation has effects in multiple neural circuits associated with PTSD symptoms, which may include regions of the amygdala, medial prefrontal cortex (mPFC) and hippocampus. There are several lines of evidence suggesting that PACAP activation has distinct and interesting actions in the central nucleus of the amygdala (CeA; Missig et al., 2017; Meloni et al., 2019; Varodayan et al., 2020), basolateral amygdala (BLA; Cho et al., 2012; Schmidt et al., 2015), and mPFC (Kirry et al., 2018, 2019), and some of this work has been reviewed elsewhere (Miles and Maren, 2019). Here, we discuss potential roles of PACAP regulating activity within the hippocampus, as well as the behavioral consequences of such regulation.
PACAP is the archetypical member of the vasoactive intestinal peptide (VIP)-secretin-glucagon family of bioactive peptides and was isolated from ovine hypothalami based on its ability to stimulate adenylyl cyclase activity in anterior pituitary cells (Kimura et al., 1990; Miyata et al., 1990). Two α-amidated forms of PACAP arise from alternative posttranslational processing of the precursor molecule; PACAP38 has 38 amino acid residues [rat pro-PACAP(131-168)], while PACAP27 corresponds to the amino terminus of PACAP38 [proPACAP(131-157)]. Despite similarities in endoproteolytic processing by PC1 and PC2 (prohormone convertase 1 and 2, respectively) at dibasic amino acid processing sites, the levels of PACAP38 predominate in most tissues, although the ratio of the PACAP38: PACAP27 appears tissue-specific (Arimura et al., 1991). PACAP27 exhibits 68% amino acid identity with VIP (Kimura et al., 1990; Miyata et al., 1990); the 28-amino acid VIP peptide is also α-amidated but unlike PACAP, does not have alternatively processed forms. PACAP appears to represent the ancestral peptide and from gene duplications, VIP/PACAP and glucagon/GLP-1/GIP peptides appear to arise from different branches of the cladistic tree (Sherwood et al., 2000). PACAP peptides are well conserved among species and widely distributed among central and peripheral tissues to implicate their evolutionary importance in maintaining physiological homeostasis (Sherwood et al., 2000; Vaudry et al., 2009).
PACAP can bind to three Class B heptahelical G protein-coupled receptors (GPCR). The PAC1 receptor is selective for the two PACAP isoforms (PACAP27/PACAP38); the VPAC1 and VPAC2 receptors exhibit similar high affinities for PACAP and VIP peptides (Harmar et al., 2012; Blechman and Levkowitz, 2013). Unlike the VPAC1 and VPAC2 GPCRs which are preferentially coupled to Gαs and adenylyl cyclase activity, the PAC1 receptors can be dually coupled to Gαs and Gαq/11 to engage adenylyl cyclase and phospholipase C activities, respectively. In addition to these classical plasma membranes delimited signaling mechanisms, the PAC1 receptors have also been shown to internalize and transduce long term endosomal signaling, especially β-arrestin-mediated ERK activation, to deliver second messengers to intracellular sites with high spatial and temporal resolution (Calebiro et al., 2010; Scita and Di Fiore, 2010; McMahon and Boucrot, 2011; Irannejad et al., 2013). From these studies, the PAC1 receptor can activate a multitude and integrated sequelae of downstream signaling events for cellular responses. Adding to the complexity, PAC1 receptors are unique among the Class B receptors in that multiple receptor variants depend on the absence or presence of two 84-bp Hip and Hop cassettes that encode inserts into the third cytoplasmic loop of the GPCR. Hence the PAC1 receptor can be Null with neither Hip nor Hop inserts, just Hip alone, just Hop or HipHop (Spengler et al., 1993; Harmar et al., 2012; Blechman and Levkowitz, 2013). Depending on the cell type, the different PAC1 receptor isoforms can be differentially coupled to the diverse downstream signaling cascades. From receptor isoform analyses, all regions of the mammalian central nervous system, including humans, preferentially express the PAC1Null and PAC1Hop receptor variants; only postganglionic sympathetic neurons appear unique in the expression of just the PAC1Hop receptor variant (Braas and May, 1999).
In our work related to stress- and pain-responding, only BNST and CeA infusions with PACAP altered anxiety- or pain-related behaviors (Hammack et al., 2010; Missig et al., 2014, 2017; Roman et al., 2014); parallel studies with VIP infusions had no apparent effects suggesting PAC1 receptor signaling in these behaviors. In accord, BNST PACAP and/or PAC1 receptor expression was upregulated by chronic stress or pain states (Hammack et al., 2009, 2010; Lezak et al., 2014; Missig et al., 2017) whereas VIP or VPAC1/VPAC2 transcript levels in these regions were not sensitive to these conditions. The PACAP effects could be blocked by the PAC1 receptor antagonist PACAP(6-38); moreover, many of the effects of PACAP infusion in these areas were mimicked by the specific PAC1 receptor agonist maxadilan (Missig et al., 2014, 2017; Roman et al., 2014) to demonstrate definitively roles for PAC1 receptor signaling in these responses.
PAC1 receptors are abundant and widely distributed in the CNS (Hashimoto et al., 1996; Jaworski and Proctor, 2000). Within the hippocampus, early in situ hybridization studies demonstrated high levels of PAC1 receptor transcripts in the granule cell layer (GCL) of the dentate gyrus (DG). Indeed, DG PAC1 receptor transcript expression can be striking compared to other brain regions (Hashimoto et al., 1996; Jaworski and Proctor, 2000). Complementary studies using PACAP-Cre mice (Bradford Lowell, McLean Hospital, Harvard University) demonstrated PACAP fiber projections from hippocampal hilar mossy cells to the DG inner molecular layer (IML), which implicated the formation of short PACAPergic synaptic circuits between PACAP-expressing hilar mossy cells and the proximal dendrites on PAC1 receptor-expressing DG granule cells (see below, Condro et al., 2016). PACAP may also bind to DG VPAC1 receptors; there is little VPAC2 receptor expression in this region (Vertongen et al., 1997). In sum, these observations implicated roles for PACAP/PAC1 receptor signaling in modulating hippocampal functions, including cognition, and learning and memory with potential consequences for contextually-mediated fear behaviors.
The functional anatomy of the hippocampus has been extensively reviewed (Schultz and Engelhardt, 2014). The axons from the entorhinal cortex (EC) layer 2 neurons project via the perforant pathway to the dendrites of granule cells in the outer molecular layers of the DG (Dolorfo and Amaral, 1998a,b). The mossy fibers of DG granule cells in turn project to the hippocampal CA3 region (Amaral et al., 2007; Blaabjerg and Zimmer, 2007), which then project to the CA1 region via Schaeffer Collaterals (Ishizuka et al., 1990). Pyramidal cells from CA1 then project back to layer 5 of the EC (Amaral and Witter, 1989). In the dorsal hippocampus, these circuits have been extensively studied for their role in the processing of contextual information. For example, several studies have determined distinct functional roles for the DG in contextual learning processes. Besnard et al. (2014) demonstrated enhanced DG activity following contextual conditioning as well as during the retrieval of contextual conditioning. Moreover, precise pharmacological inhibition of DG activity demonstrated the necessity of DG function in the expression of contextual fear at short time intervals (Hernández-Rabaza et al., 2008). Given that the overall activity of DG neurons is low under basal conditions, the DG may be preferentially activated in the acquisition and retrieval of contextual memories.
Using a transgenic mouse model where the expression of channelrhodopsin is under the control of the immediate-early gene c-fos promoter, Liu et al. (2012) reactivated the same population of DG neurons that were active at the time of contextual memory encoding. The reactivation of these DG neurons was able to enhance freezing behavior, presumably by stimulating the retrieval of the original contextual fear memory (Liu et al., 2012). Since this original report, many others have demonstrated that the reactivation of DG neuronal activity, hypothesized to be the encoding of context, is sufficient to retrieve contextual memories (Redondo et al., 2014; Ryan et al., 2015; Roy et al., 2016). Conversely, silencing DG neurons originally activated during memory encoding reduced freezing responses to a fearful context (Denny et al., 2014), suggesting that DG neuronal activity is necessary for retrieval. Hence, the population of DG neurons activated during the encoding of a contextual memory may represent the trace of that memory, also termed the memory engram (Ghandour et al., 2019). Bernier et al. (2017) further clarified the role of DG activity by demonstrating that DG inactivation significantly reduced contextual discrimination between similar contexts, while not affecting discrimination between very different contexts. As the DG may be critically involved in discerning the differences between similar contexts, altered hippocampal function may result in fear generalization across multiple contexts, which is a hallmark symptom of PTSD.
Several studies have demonstrated that PACAP systems regulate hippocampal activity. For example, the fEPSP response upon perforant pathway stimulation is enhanced in the presence of PACAP (Kondo et al., 1997), and in accord, Matsuyama et al. (2003) observed in both PACAP and PAC1 receptor knockout mice (Otto et al., 2001) a significant decrement in long term potentiation (LTP) following high-frequency stimulation of the perforant path. Otto et al. (2001) interpreted from receptor immunocytochemical data that the PAC1 receptors were presynaptic on DG mossy fibers and suggested an impairment of LTP at the mossy fiber/CA3 synapse following afferent stimulation in PAC1 receptor knockout mice. A few other studies have attempted to determine the role of PACAP in specific hippocampal subregions, especially the CA1. Hence, synaptic strength at the Schaeffer collateral synapse is sensitive to PACAP (Kondo et al., 1997; Roberto and Brunelli, 2000; Roberto et al., 2001; Ciranna and Cavallaro, 2003), which may be mediated by PACAP effects on AMPA currents (Costa et al., 2009; Toda and Huganir, 2015), NMDA currents (Macdonald et al., 2005), or other intrinsic channel mechanisms (Taylor et al., 2014). Behaviorally, PAC1 receptor knockout mice demonstrated decrements in several hippocampal-dependent tasks, such as foreground contextual fear conditioning (Sauvage et al., 2000), and background contextual fear conditioning (Otto et al., 2001). Moreover, PACAP infusions into CA1 enhanced the consolidation of contextual fear conditioning, and antagonism of the PAC1 receptor at CA1 impaired fear consolidation as well as subsequent fear extinction (Schmidt et al., 2015). Despite these studies, there is limited evidence of PACAP innervation of CA1 or CA3. Recent studies with PACAP-EGFP mice demonstrated sparse PACAPergic innervation of CA1 or CA3 (Condro et al., 2016), which appeared to complement the in situ hybridization data showing proportionately much less PAC1 receptor transcript expression in these areas than other regions of the hippocampus (Hashimoto et al., 1996; Jaworski and Proctor, 2000). Hence, whether the early observations on PACAP-mediated hippocampal function reflected VIP or PACAP signaling on VPAC receptors remained unclear. However, as a result of the availability of more recent tools and models, there has been a refocus of PACAP roles in the hippocampus.
In aggregate, the recent data from studies using the PACAP-EGFP and PACAP-Cre mice have been in alignment with previous PACAP and PAC1 receptor in situ hybridization studies, demonstrating that hippocampal PACAP expression is largely from hilar mossy cells which send projections predominantly to PAC1 receptor-expressing DG granule cell dendrites at the IML (Condro et al., 2016). From in situ hybridization data, PAC1 receptor mRNA expression is extensive in DG granule cells suggesting a role for PACAP in regulating DG activity. Previous in situ hybridization studies also identified PACAP-expressing neurons in DG hilar cells, and in coherence from PACAP-EGFP and PACAP-Cre mouse data, we and others have observed dense PACAP-positive axonal projections to the DG IML, where PACAP afferents likely synapse onto the dendrites of PAC1 receptor-expressing DG granule cells (Figure 1, Condro et al., 2016). Together, the anatomy suggests that a primary role of hippocampal PACAP signaling appears to be in the regulation of DG granule cell activity, and DG-related behaviors.
Figure 1. Neuronal pituitary adenylate cyclase-activating polypeptide (PACAP) expression and projection in the dentate gyrus (DG). The DG in PACAP-Cre mice was infused with Cre-dependent mCherry reporter. In coherence with work in PACAP-EGFP mice and previous PACAP and PAC1 receptor in situ hybridization studies, PACAP expression was in apparent hilar mossy cells which elaborated axonal projections predominantly to the inner molecular dendritic layer of granule cells. GCL, granule cell layer; IML, inner molecular layer.
Using whole-cell recordings in current-clamp mode, Johnson et al. (2020) have observed that PACAP enhances the excitability of DG granule cells assessed by determining the number of action potentials elicited by 1-s depolarizing current steps of increasing intensity (Figure 2). Thus, PACAP increased the slope of the excitability curve, an effect of PACAP that was observed in other neuron types (Cho et al., 2012; Gupte et al., 2016; May and Parsons, 2017). Moreover, this increase in excitability was associated with a negative shift in the threshold for action potential generation, observed in nearly all DG granule cell neurons, due to post-synaptic actions of PACAP, and appeared to be independent of changes in input resistance and changes in resting membrane potential (Johnson et al., 2020). Importantly, while DG neurons demonstrate VPAC1 and low levels of VPAC2 receptor expression, the excitatory actions of PACAP were not mimicked by VIP, suggesting that the PACAP regulation of DG activity was PAC1 receptor-dependent. From these observations, we propose that PACAP release could serve to increase the excitability of DG granules cells that have been activated by other means, i.e., PACAP alone would likely not have independent neuronal effects without concurrent synergistic excitatory inputs. Hence the primary consequence of DG PAC1 receptor signaling would be to amplify the responses of activated neurons.
Figure 2. PACAP enhances the excitability of DG neurons. Trace (A), representative patch-clamp recording in current-clamp mode from DG neuron demonstrating action potential generation elicited by a 1 s, 50 pA current step. Trace (B), same DG neuron as in (A) demonstrating increased action potential generation under the same conditions but in the presence of PACAP. Dentate granule cell spiking upon PACAP infusion was greater than that elicited before peptide exposure. (C) Excitability curves produced by plotting the number of action potentials elicited by 1-s current steps of increasing strength. Note that the excitability curve for neurons treated with PACAP (filled circles) is steeper than that for untreated control cells (open circles). The hashed circle indicated no action potentials were elicited by a 30 pA step.
Following previous work, we investigated the signaling mechanisms and intrinsic membrane currents potentially contributing to the PACAP/PAC1 receptor enhancement of hippocampal neuronal excitability (Johnson et al., 2020). As noted above, there are many second messenger cascades downstream of PAC1 receptor activation, including plasma membrane delimited Gαs-mediated AC/cAMP and Gαq/11-mediated PLC/DAG/IP3 signaling. Also, both AC/cAMP and PLC/DAG/IP3 signaling, along with β-arrestin-mediated endosomal signaling following receptor internalization, can activate MEK/ERK signaling cascades.
In peripheral parasympathetic postganglionic cardiac neurons, all of these signaling pathways appeared to contribute to neuronal excitability by modulating different ionic conductances (Parsons et al., 2016; Tompkins et al., 2016; May and Parsons, 2017). Cyclic AMP generation was shown to gate the nonselective cationic H-current (Merriam et al., 2004; Tompkins et al., 2009), cAMP-activation of PKA was implicated in the activation of T-type calcium currents (Tompkins et al., 2015) and ERK signaling was shown to enhance a voltage-dependent sodium current possibly through phosphorylation of Nav1.7 (Tompkins et al., 2016). However, conditions that blunt clathrin-mediated receptor endocytosis, including treatment with the clathrin inhibitor Pitstop2 or the dynamin inhibitor dynasore, as well as decreasing ambient temperature, essentially eliminated the PACAP effect on cardiac neuron excitability (Merriam et al., 2013; May et al., 2014; Tompkins et al., 2018; Parsons and May, 2019). Thus receptor internalization and recruitment of endosomal signaling was a critical mechanism. While receptor internalization has been associated primarily with desensitization mechanisms, these views are being reconsidered; these PACAP/PAC1 receptors signaling studies were unique and among those implicating receptor endosomal signaling mechanisms as important drivers of neuronal excitability.
The signaling mechanisms underlying PACAP-enhanced DG granule cell excitability appeared different from those in peripheral cardiac neurons. Interestingly, from inhibitor studies, neither the AC/cAMP/PKA nor PLC/DAG/IP3 signaling appeared to contribute to PACAP modulation of DG cell excitability. In contrast, treatment with the MEK inhibitor, PD98059, virtually eliminated PACAP-enhanced induced excitability of DG granule cells (Johnson et al., 2020). Also, like cardiac neurons, the excitatory effects of PACAP on DG granule cells were essentially eliminated by treatment with the cell-permeable clathrin-mediated endocytosis inhibitor Pitstop2. Thus the results in aggregate suggested that for hippocampal DG cells, PAC1 receptor endosomal recruitment of MEK/ERK signaling represents the primary second messenger mechanism contributing to the PACAP modulation of neuronal excitability in DG granule cells (Johnson et al., 2020). These results appeared consistent with our other reports investigating PAC1 receptor signaling in other CNS pathways (Merriam et al., 2004; May et al., 2014; Missig et al., 2017; Miles et al., 2019). GPCR endosomal signaling, as opposed to membrane-bound activation of G-protein pathways, has been described to produced sustained long-lasting activation that is less sensitive to extracellular events that normally regulate signaling, such as transmitter diffusion and/or inactivation. Perhaps not surprisingly, the excitatory effects of PACAP in the ex vivo electrophysiological preparation were long-lasting even after peptide ligand washout. This mechanism for producing long-lasting changes in neuronal excitability may have important behavioral consequences, although the temporal dynamics of the behavioral effects of PACAP are largely unexplored. PACAP has been shown to alter the activity of several ion channels that regulate the excitability of neurons and ongoing studies focus on the identification of ionic currents in DG cells that potentially are the target of ERK phosphorylation.
As noted earlier, DG granule cells play important roles in contextual fear conditioning, acquisition and retrieval, and given that PACAP may produce long-lasting changes in neuronal excitability of DG neurons (Johnson et al., 2020), PACAP may play a role in regulating these processes. As PACAP appears to augment DG neuronal activity to excitatory inputs, PACAP administration before training may facilitate contextual fear acquisition by enhancing the rate of conditioning or altering freezing behaviors during extinction testing on subsequent days. Alternatively, DG PACAP infusions before re-exposure to conditioning context may enhance fear expression thereby decreasing the rate of extinction. Whether DG PACAP signaling affects fear conditioning acquisition and/or extinction is under study but preliminary results suggest PACAP has a more pronounced effect on the latter. In this particular paradigm, DG PACAP/PAC1 receptor activation before extinction may lead to persistent neuronal activity in the neurons that make up the contextual fear memory engram to delay extinction processes.
As noted above, a polymorphism in the PAC1 receptor has been associated with PTSD symptoms (Ressler et al., 2011) and carriers of the risk allele exhibit differential hippocampal-dependent function in a contextual conditioning task compared to subjects with the normal allele (Pohlack et al., 2015). A hallmark feature of PTSD is the compromised ability to extinguish fear memories, which may be consistent with the DG PACAP behavioral effects described above. Not surprisingly, the processing of contextual information is highly associated with fear extinction, where the context may be used to disambiguate between the original fear memory and the extinction memory. Hence, should PACAP alter the processing of contextual information, the original fear memory may persist contributing to the disorder. Hippocampal dysfunction has also been associated with fear generalization, a feature of PTSD where fear responses are expressed in situations or environments unrelated to the acquired context (Dunsmoor and Paz, 2015). DG PACAP signaling may also participate in this process. For example, if chronic stress-induced DG PACAP neuroplasticity and altered DG function were to obfuscate engrams encoding fear memories with non-threatening or safe engram representations, then a consequence may be behavioral abnormalities that participate in PTSD. As an illustration, if behavioral engram representations for celebratory fireworks were obfuscated or supplanted, from stress-mediated PACAP neuroplasticity, by engrams encoding fear from combat barrages, then the changes in DG function may reinforce other maladapted stress circuits leading to psychopathologies. These data, combined with data implicating the role of stress-related PACAPergic dysregulation in other limbic regions in the regulation of emotional behavior, continue to implicate maladaptations to central PACAP/PAC1 receptor systems in the development and expression of fear and anxiety-related behavioral disorders.
Neural circuits in the hippocampus have been highly implicated in the processing of contextual information, including paradigms associated with emotional (fear) learning. In particular, processing in the hippocampal DG may be particularly critical for processing subtle differences between similar contexts. Anatomical data suggest that DG granule cells highly express PAC1 receptors and receive dense input from PACAP-expressing axon terminals in the IML. And, physiological data suggest that PACAP enhances the excitability of DG granule cells via PAC1 receptor endosomal signaling that activates MEK/ERK pathways to regulate the function of intrinsic membrane currents (Johnson et al., 2020). Consistent with this anatomy and physiology, PACAP infusions into the hippocampal DG appear to enhance the retention of a contextual fear memory. These data are consistent with previous reports implicating dysregulations in PACAP systems with PTSD symptoms and suggest that the hippocampal DG may be an important target for the effects of PACAP dysregulation. These data support an expanding literature that also implicates bed nucleus of the stria terminalis (BNST), central nucleus of the amygdala (CeA), hypothalamic subregions as other key structures where PACAP activation regulates emotional behavior. Together these data suggest that central PACAP activation is a key event that coordinates the activity of many circuits associated with the behavioral response to threatening stimuli; hence, PACAP systems may also play a key role when severe or chronic stress produces emotional pathology. These systems may be key targets for future therapeutic approaches to stress-related psychiatric disorders.
GJ performed some of the studies described in the manuscript. GJ, RP, VM, and SH contributed to some of the experimental designs, writing and editing of the manuscript.
This work was supported by National Institute of Mental Health (NIH) grants MH097988 and National Institute of General Medical Sciences (GM)129431, University of Vermont (UVM) Vice President for Research Office, and UVM College of Arts and Sciences.
The authors declare that the research was conducted in the absence of any commercial or financial relationships that could be construed as a potential conflict of interest.
Amaral, D. G., Scharfman, H. E., and Lavenex, P. (2007). The dentate gyrus: fundamental neuroanatomical organization (dentate gyrus for dummies). Prog. Brain Res. 163, 3–22. doi: 10.1016/s0079-6123(07)63001-5
Amaral, D. G., and Witter, M. P. (1989). The three-dimensional organization of the hippocampal formation: a review of anatomical data. Neuroscience 31, 571–591. doi: 10.1016/0306-4522(89)90424-7
Arimura, A., Somogyvári-Vigh, A., Miyata, A., Mizuno, K., Coy, D. H., and Kitada, C. (1991). Tissue distribution of PACAP as determined by RIA: highly abundant in the rat brain and testes. Endocrinology 129, 2787–2789. doi: 10.1210/endo-129-5-2787
Bernier, B. E., Lacagnina, A. F., Ayoub, A., Shue, F., Zemelman, B. V., Krasne, F. B., et al. (2017). Dentate gyrus contributes to retrieval as well as encoding: evidence from context fear conditioning, recall, and extinction. J. Neurosci. 37, 6359–6371. doi: 10.1523/JNEUROSCI.3029-16.2017
Besnard, A., Laroche, S., and Caboche, J. (2014). Comparative dynamics of MAPK/ERK signalling components and immediate early genes in the hippocampus and amygdala following contextual fear conditioning and retrieval. Brain Struct. Funct. 219, 415–430. doi: 10.1007/s00429-013-0505-y
Blaabjerg, M., and Zimmer, J. (2007). The dentate mossy fibers: structural organization, development and plasticity. Prog. Brain Res. 163, 85–107. doi: 10.1016/s0079-6123(07)63005-2
Blechman, J., and Levkowitz, G. (2013). Alternative splicing of the pituitary adenylate cyclase-activating polypeptide receptor PAC1: mechanisms of fine tuning of brain activity. Front. Endocrinol. 4:55. doi: 10.3389/fendo.2013.00055
Braas, K. M., and May, V. (1999). Pituitary adenylate cyclase-activating polypeptides directly stimulate sympathetic neuron neuropeptide Y release through PAC1 receptor isoform activation of specific intracellular signaling pathways. J. Biol. Chem. 274, 27702–27710. doi: 10.1074/jbc.274.39.27702
Calebiro, D., Nikolaev, V. O., Persani, L., and Lohse, M. J. (2010). Signaling by internalized G-protein-coupled receptors. Trends Pharmacol. Sci. 31, 221–228. doi: 10.1016/j.tips.2010.02.002
Cho, J. H., Zushida, K., Shumyatsky, G. P., Carlezon, W. A. Jr., Meloni, E. G., and Bolshakov, V. Y. (2012). Pituitary adenylate cyclase-activating polypeptide induces postsynaptically expressed potentiation in the intra-amygdala circuit. J. Neurosci. 32, 14165–14177. doi: 10.1523/JNEUROSCI.1402-12.2012
Ciranna, L., and Cavallaro, S. (2003). Opposing effects by pituitary adenylate cyclase-activating polypeptide and vasoactive intestinal peptide on hippocampal synaptic transmission. Exp. Neurol. 184, 778–784. doi: 10.1016/s0014-4886(03)00300-5
Condro, M. C., Matynia, A., Foster, N. N., Ago, Y., Rajbhandari, A. K., Van, C., et al. (2016). High-resolution characterization of a PACAP-EGFP transgenic mouse model for mapping PACAP-expressing neurons. J Comp Neurol. 524, 3827–3848. doi: 10.1002/cne.24035
Costa, L., Santangelo, F., Li Volsi, G., and Ciranna, L. (2009). Modulation of AMPA receptor-mediated ion current by pituitary adenylate cyclase-activating polypeptide (PACAP) in CA1 pyramidal neurons from rat hippocampus. Hippocampus 19, 99–109. doi: 10.1002/hipo.20488
Denny, C. A., Kheirbek, M. A., Alba, E. L., Tanaka, K. F., Brachman, R. A., Laughman, K. B., et al. (2014). Hippocampal memory traces are differentially modulated by experience, time, and adult neurogenesis. Neuron 83, 189–201. doi: 10.1016/j.neuron.2014.05.018
Dolorfo, C. L., and Amaral, D. G. (1998a). Entorhinal cortex of the rat: organization of intrinsic connections. J. Comp. Neurol. 398, 49–82. doi: 10.1002/(sici)1096-9861(19980817)398:1<49::aid-cne4>3.0.co;2-9
Dolorfo, C. L., and Amaral, D. G. (1998b). Entorhinal cortex of the rat: topographic organization of the cells of origin of the perforant path projection to the dentate gyrus. J. Comp. Neurol. 398, 25–48. doi: 10.1002/(sici)1096-9861(19980817)398:1<25::aid-cne3>3.0.co;2-b
Dunsmoor, J. E., and Paz, R. (2015). Fear generalization and anxiety: behavioral and neural mechanisms. Biol. Psychiatry 78, 336–343. doi: 10.1016/j.biopsych.2015.04.010
Ghandour, K., Ohkawa, N., Fung, C. C. A., Asai, H., Saitoh, Y., Takekawa, T., et al. (2019). Orchestrated ensemble activities constitute a hippocampal memory engram. Nat. Commun. 10:2637. doi: 10.1038/s41467-019-10683-2
Gupte, R. P., Kadunganattil, S., Shepherd, A. J., Merrill, R., Planer, W., Bruchas, M. R., et al. (2016). Convergent phosphomodulation of the major neuronal dendritic potassium channel Kv4.2 by pituitary adenylate cyclase-activating polypeptide. Neuropharmacology 101, 291–308. doi: 10.1016/j.neuropharm.2015.10.006
Hammack, S. E., Cheung, J., Rhodes, K. M., Schutz, K. C., Falls, W. A., Braas, K. M., et al. (2009). Chronic stress increases pituitary adenylate cyclase-activating peptide (PACAP) and brain-derived neurotrophic factor (BDNF) mRNA expression in the bed nucleus of the stria terminalis (BNST): roles for PACAP in anxiety-like behavior. Psychoneuroendocrinology 34, 833–843. doi: 10.1016/j.psyneuen.2008.12.013
Hammack, S. E., and May, V. (2015). Pituitary adenylate cyclase activating polypeptide in stress-related disorders: data convergence from animal and human studies. Biol. Psychiatry 78, 167–177. doi: 10.1016/j.biopsych.2014.12.003
Hammack, S. E., Roman, C. W., Lezak, K. R., Kocho-Shellenberg, M., Grimmig, B., Falls, W. A., et al. (2010). Roles for pituitary adenylate cyclase-activating peptide (PACAP) expression and signaling in the bed nucleus of the stria terminalis (BNST) in mediating the behavioral consequences of chronic stress. J. Mol. Neurosci. 42, 327–340. doi: 10.1007/s12031-010-9364-7
Harmar, A. J., Fahrenkrug, J., Gozes, I., Laburthe, M., May, V., Pisegna, J. R., et al. (2012). Pharmacology and functions of receptors for vasoactive intestinal peptide and pituitary adenylate cyclase-activating polypeptide: IUPHAR review 1. Br. J. Pharmacol. 166, 4–17. doi: 10.1111/j.1476-5381.2012.01871.x
Hashimoto, H., Nogi, H., Mori, K., Ohishi, H., Shigemoto, R., Yamamoto, K., et al. (1996). Distribution of the mRNA for a pituitary adenylate cyclase-activating polypeptide receptor in the rat brain: an in situ hybridization study. J. Comp. Neurol. 371, 567–577. doi: 10.1002/(SICI)1096-9861(19960805)371:4<567::AID-CNE6>3.0.CO;2-2
Hernández-Rabaza, V., Hontecillas-Prieto, L., Velázquez-Sánchez, C., Ferragud, A., Pérez-Villaba, A., Arcusa, A., et al. (2008). The hippocampal dentate gyrus is essential for generating contextual memories of fear and drug-induced reward. Neurobiol. Learn. Mem. 90, 553–559. doi: 10.1016/j.nlm.2008.06.008
Irannejad, R., Tomshine, J. C., Tomshine, J. R., Chevalier, M., Mahoney, J. P., Steyaert, J., et al. (2013). Conformational biosensors reveal GPCR signalling from endosomes. Nature 495, 534–538. doi: 10.1038/nature12000
Ishizuka, N., Weber, J., and Amaral, D. G. (1990). Organization of intrahippocampal projections originating from CA3 pyramidal cells in the rat. J. Comp. Neurol. 295, 580–623. doi: 10.1002/cne.902950407
Jaworski, D. M., and Proctor, M. D. (2000). Developmental regulation of pituitary adenylate cyclase-activating polypeptide and PAC1 receptor mRNA expression in the rat central nervous system. Dev. Brain Res. 120, 27–39. doi: 10.1016/s0165-3806(99)00192-3
Johnson, G. C., Parsons, R. L., May, V., and Hammack, S. E. (2020). PACAP-induced PAC1 receptor internalization and recruitment of MEK/ERK signaling enhances excitability of dentate gyrus granule cells. Am. J. Physiol. doi: 10.1152/ajpcell.00065.2020 [Epub ahead of print].
Jovanovic, T., Kazama, A., Bachevalier, J., and Davis, M. (2012). Impaired safety signal learning may be a biomarker of PTSD. Neuropharmacology 62, 695–704. doi: 10.1016/j.neuropharm.2011.02.023
Kimura, C., Ohkubo, S., Ogi, K., Hosoya, M., Itoh, Y., Onda, H., et al. (1990). A novel peptide which stimulates adenylate cyclase: molecular cloning and characterization of the ovine and human cDNAs. Biochem. Biophys. Res. Commun. 166, 81–89. doi: 10.1016/0006-291x(90)91914-e
Kirry, A. J., Durigan, D. J., Twining, R. C., and Gilmartin, M. R. (2019). Estrous cycle stage gates sex differences in prefrontal muscarinic control of fear memory formation. Neurobiol. Learn. Mem. 161, 26–36. doi: 10.1016/j.nlm.2019.03.001
Kirry, A. J., Herbst, M. R., Poirier, S. E., Maskeri, M. M., Rothwell, A. C., Twining, R. C., et al. (2018). Pituitary adenylate cyclase-activating polypeptide (PACAP) signaling in the prefrontal cortex modulates cued fear learning, but not spatial working memory, in female rats. Neuropharmacology 133, 145–154. doi: 10.1016/j.neuropharm.2018.01.010
Kondo, T., Tominaga, T., Ichikawa, M., and Iijima, T. (1997). Differential alteration of hippocampal synaptic strength induced by pituitary adenylate cyclase activating polypeptide-38 (PACAP-38). Neurosci. Lett. 221, 189–192. doi: 10.1016/s0304-3940(96)13323-1
Kormos, V., and Gaszner, B. (2013). Role of neuropeptides in anxiety, stress, and depression: from animals to humans. Neuropeptides 47, 401–419. doi: 10.1016/j.npep.2013.10.014
Lezak, K. R., Roman, C. W., Braas, K. M., Schutz, K. C., Falls, W. A., Schulkin, J., et al. (2014). Regulation of bed nucleus of the stria terminalis PACAP expression by stress and corticosterone. J. Mol. Neurosci. 54, 477–484. doi: 10.1007/s12031-014-0269-8
Liu, X., Ramirez, S., Pang, P. T., Puryear, C. B., Govindarajan, A., Deisseroth, K., et al. (2012). Optogenetic stimulation of a hippocampal engram activates fear memory recall. Nature 484, 381–385. doi: 10.1038/nature11028
Lutfy, K., and Shankar, G. (2019). Emerging evidence for the role of pituitary adenylate cyclase-activating peptide in neuropsychiatric disorders. Prog. Mol. Biol. Transl. Sci. 167, 143–157. doi: 10.1016/bs.pmbts.2019.06.009
Macdonald, D. S., Weerapura, M., Beazely, M. A., Martin, L., Czerwinski, W., Roder, J. C., et al. (2005). Modulation of NMDA receptors by pituitary adenylate cyclase activating peptide in CA1 neurons requires G α q, protein kinase C, and activation of Src. J. Neurosci. 25, 11374–11384. doi: 10.1523/JNEUROSCI.3871-05.2005
Matsuyama, S., Matsumoto, A., Hashimoto, H., Shintani, N., and Baba, A. (2003). Impaired long-term potentiation in vivo in the dentate gyrus of pituitary adenylate cyclase-activating polypeptide (PACAP) or PACAP type 1 receptor-mutant mice. Neuroreport 14, 2095–2098. doi: 10.1097/00001756-200311140-00017
May, V., Buttolph, T. R., Girard, B. M., Clason, T. A., and Parsons, R. L. (2014). PACAP-induced ERK activation in HEK cells expressing PAC1 receptors involves both receptor internalization and PKC signaling. Am. J. Physiol. Cell Physiol. 306, C1068–C1079. doi: 10.1152/ajpcell.00001.2014
May, V., and Parsons, R. L. (2017). G protein-coupled receptor endosomal signaling and regulation of neuronal excitability and stress responses: signaling options and lessons from the PAC1 receptor. J. Cell. Physiol. 232, 698–706. doi: 10.1002/jcp.25615
McMahon, H. T., and Boucrot, E. (2011). Molecular mechanism and physiological functions of clathrin-mediated endocytosis. Nat. Rev. Mol. Cell Biol. 12, 517–533. doi: 10.1038/nrm3151
Meloni, E. G., Kaye, K. T., Venkataraman, A., and Carlezon, W. A. Jr. (2019). PACAP increases Arc/Arg 3.1 expression within the extended amygdala after fear conditioning in rats. Neurobiol. Learn. Mem. 157, 24–34. doi: 10.1016/j.nlm.2018.11.011
Mercer, K. B., Dias, B., Shafer, D., Maddox, S. A., Mulle, J. G., Hu, P., et al. (2016). Functional evaluation of a PTSD-associated genetic variant: estradiol regulation and ADCYAP1R1. Transl. Psychiatry 6:e978. doi: 10.1038/tp.2016.241
Merriam, L. A., Baran, C. N., Girard, B. M., Hardwick, J. C., May, V., and Parsons, R. L. (2013). Pituitary adenylate cyclase 1 receptor internalization and endosomal signaling mediate the pituitary adenylate cyclase activating polypeptide-induced increase in guinea pig cardiac neuron excitability. J. Neurosci. 33, 4614–4622. doi: 10.1523/JNEUROSCI.4999-12.2013
Merriam, L. A., Barstow, K. L., and Parsons, R. L. (2004). Pituitary adenylate cyclase-activating polypeptide enhances the hyperpolarization-activated nonselective cationic conductance, Ih, in dissociated guinea pig intracardiac neurons. Regul. Pept. 123, 123–133. doi: 10.1016/j.regpep.2004.04.019
Miles, O. W., and Maren, S. (2019). Role of the bed nucleus of the stria terminalis in PTSD: insights from preclinical models. Front. Behav. Neurosci. 13:68. doi: 10.3389/fnbeh.2019.00068
Miles, O. W., May, V., and Hammack, S. E. (2019). Pituitary adenylate cyclase-activating peptide (PACAP) signaling and the dark side of addiction. J. Mol. Neurosci. 68, 453–464. doi: 10.1007/s12031-018-1147-6
Miles, O. W., Thrailkill, E. A., Linden, A. K., May, V., Bouton, M. E., and Hammack, S. E. (2018). Pituitary adenylate cyclase-activating peptide in the bed nucleus of the stria terminalis mediates stress-induced reinstatement of cocaine seeking in rats. Neuropsychopharmacology 43, 978–986. doi: 10.1038/npp.2017.135
Missig, G., Mei, L., Vizzard, M. A., Braas, K. M., Waschek, J. A., Ressler, K. J., et al. (2017). Parabrachial pituitary adenylate cyclase-activating polypeptide activation of amygdala endosomal extracellular signal-regulated kinase signaling regulates the emotional component of pain. Biol. Psychiatry 81, 671–682. doi: 10.1016/j.biopsych.2016.08.025
Missig, G., Roman, C. W., Vizzard, M. A., Braas, K. M., Hammack, S. E., and May, V. (2014). Parabrachial nucleus (PBn) pituitary adenylate cyclase activating polypeptide (PACAP) signaling in the amygdala: implication for the sensory and behavioral effects of pain. Neuropharmacology 86, 38–48. doi: 10.1016/j.neuropharm.2014.06.022
Miyata, A., Jiang, L., Dahl, R. R., Kikada, C., Kubo, K., Fujino, M., et al. (1990). Isolation of a neuropeptide corresponding to the N-terminal 27 residues of the pituitary adenylate cyclase activating polypeptide with 38 residues (PACAP38). Biochem. Biophys. Res. Commun. 170, 643–648. doi: 10.1016/0006-291x(90)92140-u
Otto, C., Kovalchuk, Y., Wolfer, D. P., Gass, P., Martin, M., Zuschratter, W., et al. (2001). Impairment of mossy fiber long-term potentiation and associative learning in pituitary adenylate cyclase activating polypeptide type I receptor-deficient mice. J. Neurosci. 21, 5520–5527. doi: 10.1523/JNEUROSCI.21-15-05520.2001
Parsons, R. L., and May, V. (2019). PACAP-induced PAC1 receptor internalization and recruitment of endosomal signaling regulate cardiac neuron excitability. J. Mol. Neurosci. Springer International Publishing, Switzerland 68, 340–347. doi: 10.1007/s12031-018-1127-x
Parsons, R. L., Tompkins, J. D., Hardwick, J. C., Merriam, L. A., Girard, B. M., and May, V. (2016). “Multiple mechanisms contribute to PAC1 modulation of parasympathetic cardiac neuron excitability,” in Pituitary Adenylate Cyclase Activating Polypeptide-PACAP, Current Topics in Neurotoxicity 11, eds D. Reglodi and A. Tamas (Switzerland: Springer International Publishing), 205–225.
Pohlack, S. T., Nees, F., Ruttorf, M., Cacciaglia, R., Winkelmann, T., Schad, L. R., et al. (2015). Neural mechanism of a sex-specific risk variant for posttraumatic stress disorder in the type I receptor of the pituitary adenylate cyclase activating polypeptide. Biol. Psychiatry 78, 840–847. doi: 10.1016/j.biopsych.2014.12.018
Ramikie, T. S., and Ressler, K. J. (2016). Stress-related disorders, pituitary adenylate cyclase-activating peptide (PACAP)ergic system, and sex differences. Dialogues Clin. Neurosci. 18, 403–413.
Redondo, R. L., Kim, J., Arons, A. L., Ramirez, S., Liu, X., and Tonegawa, S. (2014). Bidirectional switch of the valence associated with a hippocampal contextual memory engram. Nature 513, 426–430. doi: 10.1038/nature13725
Ressler, K. J., Mercer, K. B., Bradley, B., Jovanovic, T., Mahan, A., Kerley, K., et al. (2011). Post-traumatic stress disorder is associated with PACAP and the PAC1 receptor. Nature 470, 492–497. doi: 10.1038/nature09856
Roberto, M., and Brunelli, M. (2000). PACAP-38 enhances excitatory synaptic transmission in the rat hippocampal CA1 region. Learn. Mem. 7, 303–311. doi: 10.1101/lm.34200
Roberto, M., Scuri, R., and Brunelli, M. (2001). Differential effects of PACAP-38 on synaptic responses in rat hippocampal CA1 region. Learn. Mem. 8, 265–271. doi: 10.1101/lm.40501
Roman, C. W., Lezak, K. R., Hartsock, M. J., Falls, W. A., Braas, K. M., Howard, A. B., et al. (2014). PAC1 receptor antagonism in the bed nucleus of the stria terminalis (BNST) attenuates the endocrine and behavioral consequences of chronic stress. Psychoneuroendocrinology 47, 151–165. doi: 10.1016/j.psyneuen.2014.05.014
Roy, D. S., Arons, A., Mitchell, T. I., Pignatelli, M., Ryan, T. J., and Tonegawa, S. (2016). Memory retrieval by activating engram cells in mouse models of early Alzheimer’s disease. Nature 531, 508–512. doi: 10.1038/nature17172
Ryan, T. J., Roy, D. S., Pignatelli, M., Arons, A., and Tonegawa, S. (2015). Memory. Engram cells retain memory under retrograde amnesia. Science 348, 1007–1013. doi: 10.1126/science.aaa5542
Sangha, S., Diehl, M. M., Bergstrom, H. C., and Drew, M. R. (2020). Know safety, no fear. Neurosci. Biobehav. Rev. 108, 218–230. doi: 10.1016/j.neubiorev.2019.11.006
Sauvage, M., Brabet, P., Holsboer, F., Bockaert, J., and Steckler, T. (2000). Mild deficits in mice lacking pituitary adenylate cyclase-activating polypeptide receptor type 1 (PAC1) performing on memory tasks. Mol. Brain Res. 84, 79–89. doi: 10.1016/s0169-328x(00)00219-9
Schmidt, S. D., Myskiw, J. C., Furini, C. R., Schmidt, B. E., Cavalcante, L. E., and Izquierdo, I. (2015). PACAP modulates the consolidation and extinction of the contextual fear conditioning through NMDA receptors. Neurobiol. Learn. Mem. 118, 120–124. doi: 10.1016/j.nlm.2014.11.014
Schultz, C., and Engelhardt, M. (2014). Anatomy of the hippocampal formation. Front. Neurol Neurosci. 34, 6–17. doi: 10.1159/000360925
Scita, G., and Di Fiore, P. P. (2010). The endocytic matrix. Nature 463, 464–473. doi: 10.1038/nature08910
Sherwood, N. M., Krueckl, S. L., and McRory, J. E. (2000). The origin and function of the pituitary adenylate cyclase-activating polypeptide (PACAP)/glucagon superfamily. Endocr. Rev. 21, 619–670. doi: 10.1210/edrv.21.6.0414
Spengler, D., Waeber, C., Pantaloni, C., Holsboer, F., Bockaert, J., Seeburg, P. H., et al. (1993). Differential signal transduction by five splice variants of the PACAP receptor. Nature 365, 170–175. doi: 10.1038/365170a0
Taylor, R. D., Madsen, M. G., Krause, M., Sampedro-Castañeda, M., Stocker, M., and Pedarzani, P. (2014). Pituitary adenylate cyclase-activating polypeptide (PACAP) inhibits the slow afterhyperpolarizing current sIAHP in CA1 pyramidal neurons by activating multiple signaling pathways. Hippocampus 24, 32–43. doi: 10.1002/hipo.22201
Toda, A. M., and Huganir, R. L. (2015). Regulation of AMPA receptor phosphorylation by the neuropeptide PACAP38. Proc. Natl. Acad. Sci. U S A. 112, 6712–6717. doi: 10.1073/pnas.1507229112
Tompkins, J. D., Clason, T. A., Buttolph, T. R., Girard, B. M., Linden, A. K., Hardwick, J. C., et al. (2018). Src family kinase inhibitors blunt PACAP-induced PAC1 receptor endocytosis, phosphorylation of ERK, and the increase in cardiac neuron excitability. Am. J. Physiol. Cell Physiol. 314, C233–C241. doi: 10.1152/ajpcell.00223.2017
Tompkins, J. D., Clason, T. A., Hardwick, J. C., Girard, B. M., Merriam, L. A., May, V., et al. (2016). Activation of MEK/ERK signaling contributes to the PACAP-induced increase in guinea pig cardiac neuron excitability. Am. J. Physiol. Cell Physiol. 311, C643–C651. doi: 10.1152/ajpcell.00164.2016
Tompkins, J. D., Lawrence, Y. T., and Parsons, R. L. (2009). Enhancement of Ih, but not inhibition of IM, is a key mechanism underlying the PACAP-induced increase in excitability of guinea pig intrinsic cardiac neurons. Am. J. Physiol. Regul. Integr. Comp. Physiol. 297, R52–R59. doi: 10.1152/ajpregu.00039.2009
Tompkins, J. D., Merriam, L. A., Girard, B. M., May, V., and Parsons, R. L. (2015). Nickel suppresses the PACAP-induced increase in guinea pig cardiac neuron excitability. Am. J. Physiol. Cell Physiol. 308, C857–C866. doi: 10.1152/ajpcell.00403.2014
Varodayan, F. P., Minnig, M. A., Steinman, M. Q., Oleata, C. S., Riley, M. W., Sabino, V., et al. (2020). PACAP regulation of central amygdala GABAergic synapses is altered by restraint stress. Neuropharmacology 168:107752. doi: 10.1016/j.neuropharm.2019.107752
Vaudry, D., Falluel-Morel, A., Bourgault, S., Basille, M., Burel, D., Wurtz, O., et al. (2009). Pituitary adenylate cyclase-activating polypeptide and its receptors: 20 years after the discovery. Pharmacol. Rev. 61, 283–357. doi: 10.1124/pr.109.001370
Keywords: pituitary adenylate cyclase-activating polypeptide, PAC1 receptor, hippocampus, dentate gyrus, contextual fear conditioning
Citation: Johnson GC, Parsons R, May V and Hammack SE (2020) The Role of Pituitary Adenylate Cyclase-Activating Polypeptide (PACAP) Signaling in the Hippocampal Dentate Gyrus. Front. Cell. Neurosci. 14:111. doi: 10.3389/fncel.2020.00111
Received: 11 December 2019; Accepted: 08 April 2020;
Published: 30 April 2020.
Edited by:
Lucia Ciranna, University of Catania, ItalyReviewed by:
Takashi Tominaga, Tokushima Bunri University, JapanCopyright © 2020 Johnson, Parsons, May and Hammack. This is an open-access article distributed under the terms of the Creative Commons Attribution License (CC BY). The use, distribution or reproduction in other forums is permitted, provided the original author(s) and the copyright owner(s) are credited and that the original publication in this journal is cited, in accordance with accepted academic practice. No use, distribution or reproduction is permitted which does not comply with these terms.
*Correspondence: Sayamwong E. Hammack, c2hhbW1hY2tAdXZtLmVkdQ==
Disclaimer: All claims expressed in this article are solely those of the authors and do not necessarily represent those of their affiliated organizations, or those of the publisher, the editors and the reviewers. Any product that may be evaluated in this article or claim that may be made by its manufacturer is not guaranteed or endorsed by the publisher.
Research integrity at Frontiers
Learn more about the work of our research integrity team to safeguard the quality of each article we publish.