- 1Center for Physiology and Pharmacology, Department of Neurophysiology and Neuropharmacology, Medical University of Vienna, Vienna, Austria
- 2Center for Physiology and Pharmacology, Institute for Physiology, Medical University of Vienna, Vienna, Austria
- 3Department of Medical Physiology and Biophysics, Institute of Biomedicine of Seville (IBiS)/University of Seville/CIBERCV, Seville, Spain
- 4Center for Physiology and Pharmacology, Department of Vascular Biology and Thrombosis Research, Medical University of Vienna, Vienna, Austria
Podoplanin (Pdpn), a brain-tumor-related glycoprotein identified in humans and animals, is endogenously expressed in several organs critical for life support such as kidney, lung, heart and brain. In the brain, Pdpn has been identified in proliferative nestin-positive adult neural progenitor cells and in neurons of the neurogenic hippocampal dentate gyrus (DG), a structure associated to anxiety, critical for learning and memory functions and severely damaged in people with Alzheimer’s Disease (AD). The in vivo role of Pdpn in adult neurogenesis and anxiety-like behavior remained however unexplored. Using mice with disrupted Pdpn gene as a model organism and applying combined behavioral, molecular biological and electrophysiological assays, we here show that the absence of Pdpn selectively impairs long-term synaptic depression in the neurogenic DG without affecting the CA3-Schaffer’s collateral-CA1 synapses. Pdpn deletion also enhanced the proliferative capacity of DG neural progenitor cells and diminished survival of differentiated neuronal cells in vitro. In addition, mice with podoplanin gene disruption showed increased anxiety-like behaviors in experimentally validated behavioral tests as compared to wild type littermate controls. Together, these findings broaden our knowledge on the molecular mechanisms influencing hippocampal synaptic plasticity and neurogenesis in vivo and reveal Pdpn as a novel molecular target for future studies addressing general anxiety disorder and synaptic depression-related memory dysfunctions.
Introduction
Podoplanin (Pdpn), also known as AGGRUS; GP36; Gp38; GP40; HT1A-1; OTS8; PA2.26; T1A; TI1A; T1A2; T1A-2, is a small (~40 kDa) transmembrane cell-surface sialomucin-like glycoprotein present in humans and several other species, which has been implicated in severe pathophysiological processes in some of the organs where it is constitutively expressed (Rishi et al., 1995; Williams et al., 1996; Breiteneder-Geleff et al., 1997; Williams, 2003; Shibahara et al., 2006; Gittenberger-de Groot et al., 2007; Vanderbilt et al., 2008; Uhrin et al., 2010; Astarita et al., 2012; Kolar et al., 2015). In particular, Pdpn is expressed in the developmental and adult mammalian brain, where aberrant and exacerbated cellular Pdpn expression has been associated with the genesis and expansion of malignant brain tumors (Cortez et al., 2010; Astarita et al., 2012). Under physiological conditions, however, Pdpn is profusely expressed in the hippocampal dentate gyrus (DG; Kotani et al., 2003; Cicvaric et al., 2016), a region where neurogenesis takes place. The endogenous function of Pdpn in the adult DG and its involvement in in vivo neurogenesis remained however unexplored.
Adult neurogenesis is a form of plasticity comprising the formation of newly developed neurons that can be functionally integrated into pre-established synaptic circuits (Eriksson et al., 1998; Colucci-D’Amato et al., 2006; Costa et al., 2015; Baptista and Andrade, 2018). Few regions of the mammalian brain, including the hippocampal DG, contain dividing progenitor cells capable of giving rise to newly formed functional neurons (Liu and Martin, 2006; Hagg, 2009; Ming and Song, 2011; Walton, 2012; Dennis et al., 2016); indicative of a high degree of functional specificity. Newly generated neurons in the hippocampus play key roles in memory acquisition and maintenance (Anacker et al., 2015; Goncalves et al., 2016; Hollands et al., 2017; Toda et al., 2019). The mechanisms linking hippocampal neurogenesis to memory functions remain however poorly understood. Some of the neural plastic changes occurring during long-term potentiation (LTP) and long-term depression (LTD) are proposed as putative mechanisms participating in the in vivo formation of memories (Malenka and Bear, 2004; Sajikumar and Frey, 2004) and both LTP and LTD have been independently associated to neurogenesis (Staubli and Lynch, 1990; Jouvenceau et al., 2006; Saxe et al., 2006, 2007; Kemp and Manahan-Vaughan, 2007; Malleret et al., 2010). Alterations in hippocampal neurogenesis are additionally associated with psychiatric disorders including depression and anxiety (Abrous et al., 2005; Trejo et al., 2008; Llorens-Martin et al., 2010; Petrik et al., 2012; Nishijima et al., 2013; Toda et al., 2019) and to the onset and development of memory-related human brain neuropathologies, e.g., Alzheimer’s disease (AD; Chuang, 2010; Demars et al., 2010; Hong et al., 2010, 2011; Hollands et al., 2016; Lazarov and Hollands, 2016). However, the molecular elements linking neurogenesis to either LTP (Staubli and Lynch, 1990; Staubli et al., 1990; Tononi and Cirelli, 2006) or LTD (Zeng et al., 2001; Nakao et al., 2002; Malleret et al., 2010) and to memory dysfunctions and psychiatric disorders remain unclear.
Using a Pdpn knockout mouse line that was earlier utilized for studies on the function of Pdpn in the lymphatic vascular system (Uhrin et al., 2010), we previously reported that Pdpn gene disruption results in altered spatial reference memory and impaired synaptic strengthening specifically at the neurogenic DG (not at CA3-to-CA1 synapses), and further unveiled podoplanin as a promoter of neuritogenesis and synaptic activity (Cicvaric et al., 2016). The selective functional requirement of Pdpn to a specific sub-hippocampal region (the DG), for proper synaptic strengthening, suggested to us that Pdpn could be involved in additional DG-specific functions important for learning and memory. Here, we present experimental evidence unveiling a novel role for Pdpn in hippocampal neurogenesis, DG specific synaptic depression and mood-related behavior. We show that Pdpn disruption in vivo promotes neural progenitor cell proliferation, selectively impairs DG LTD and induces anxiety-like behaviors in mice. The identification of molecular elements concomitantly influencing neurogenesis, memory-related synaptic plasticity and mood behaviors is critical for a better understanding of the brain function in health and disease.
Materials and Methods
Animals
Male Pdpn knockout mice (Pdpn−/−) and their wild-type littermate mice (Pdpn+/+), 9–18 weeks old, in 129S/v: Swiss background were obtained by crossing of heterozygous mice and maintained in specific pathogen-free facilities of the Medical University of Vienna. Animals were housed in groups of 3–5 mice per cage in a temperature [(22 ± 1)°C] and light [(200 ± 20)lx] controlled colony room with food and water provided ad libitum. The illumination was kept on a 12 h light/dark cycle with the light period starting at 6:00 a.m. Experiments described in this study were approved by the national ethical committee on animal care and use (BMWFW-66.009/0201-WF/II/3b/2014, Bundesministerium für Wissenschaft, Forschung und Wirtschaft), and carried out according to the U.K. Animals Scientific Procedures Act, 1986 and associated guidelines, EU Directive 2010/63/EU for animal experiments and in compliance with ARRIVE guidelines.
Neurogenesis Analysis in vivo
BrdU Injections
In order to analyze different stages of neurogenesis two injection paradigms were used following previously published protocols (Meshi et al., 2006; Pollak et al., 2008; Khan et al., 2014). Briefly, naïve mice were i.p. injected with BrdU [(+)-5′ Bromo-2′-deoxyuridine, Cat. No. B5002, Sigma–Aldrich, Vienna, Austria] dissolved in 0.9% NaCl solution at 50 mg/kg, four times (every 2 h) and sacrificed 24 h after the last BrdU injection to assess proliferation of progenitor cells (“proliferation paradigm”). For the assessment of the survival of the newborn cells (“survival paradigm”), mice were injected with the same dosage of BrdU twice per day (every 8 h) for three consecutive days and sacrificed 14 days after the first injection (as shown in Figure 1A).
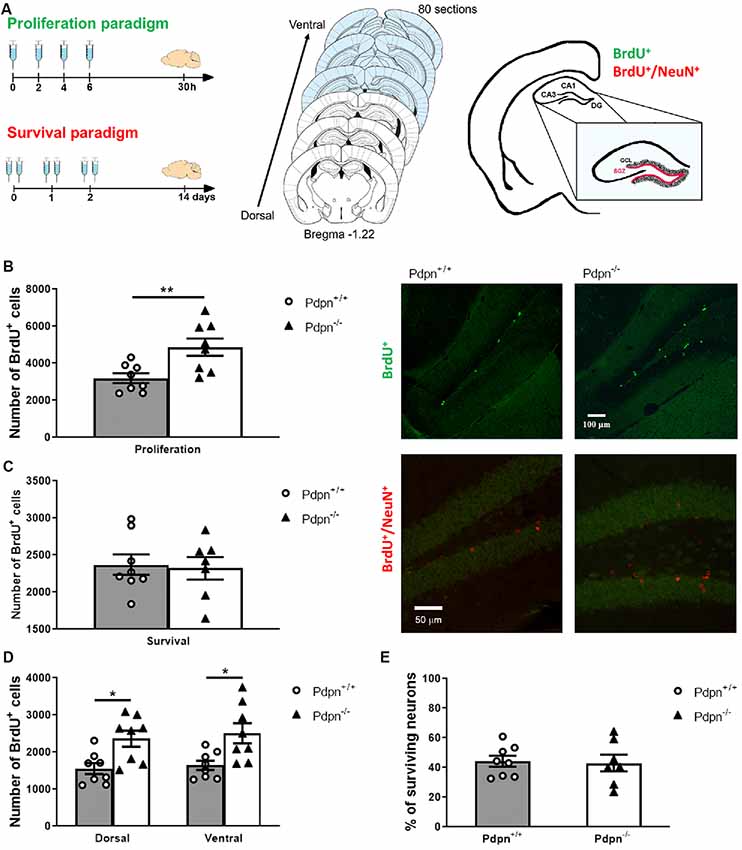
Figure 1. Lack of Podoplanin increases the proliferation of cells in the subgranular zone (SZG) of the dentate gyrus (DG). (A) Schematic representation of 5-bromodeoxyuridine (BrdU) injection protocols for proliferation and survival paradigms (left panel). Schematic representation span of the rostrocaudal axes from which coronal sections were collected (middle). The depicted coronal sections have been modified from Franklin and Paxinos (2013). The SGZ spans at the border of the granule cell layer (GCL) and hilus of the hippocampal DG (right panel). (B) Analysis of the approximated number of BrdU+ cells per hippocampus in “proliferation” paradigm showed a significant difference between two genotypes (**P = 0.008, t(14) = 3.122, n = 8 per group). Representative photomicrographs of Pdpn+/+ and Pdpn−/− coronal sections immunostained against BrdU in the “proliferation” paradigm (right panel 10× magnification). (C) In the “survival” paradigm quantification of BrdU+ cells showed no differences between hippocampi of Pdpn+/+ and Pdpn−/− mice (P = 0.819, t(13) = 0.233, n = 7–8 per group). Representative photomicrographs of Pdpn+/+ and Pdpn−/− coronal sections immunostained against BrdU in red and against NeuN in green in the “survival” paradigm (right panel 20× magnification). (D) Two-way ANOVA of the number of BrdU+ cells in “proliferation” paradigm between dorsal and ventral hippocampus showed a main significant effect of genotype (***P < 0.0002, F(1,28) = 17.61, n = 8 per group), no significant effect of the area (P = 0.5601, F(1,28) = 0.3477, n = 8 per group) and no significant interaction between paradigm and genotype (P = 0.8912, F(1,28) = 0.0190, n = 8 per group). Post hoc analysis showed significant difference between Dorsal:Pdpn+/+ and Dorsal:Pdpn−/− (*P = 0.0366), Dorsal:Pdpn+/+ and Ventral:Pdpn−/− (*P = 0.0108), as well as Ventral:Pdpn+/+ and Ventral:Pdpn−/− (*P = 0.0233), as represented with asterisks on the graph. (E) Quantification of surviving cells double-labeled with BrdU+ and NeuN+ showed no significant difference in a number of surviving neurons in Pdpn−/− mice compared to their Pdpn+/+ littermates (t(13) = 0.1857, P = 0.9, n = 7–8). Data are displayed as mean ± SEM.
Tissue Preparation
Upon induction of deep anesthesia via i.p. administration of a ketamine/xylazine cocktail (100 mg/kg ketamine, Ketanest®S, Pfizer Corporation Austria Gesellschaft m.b.H., Vienna, Austria and 40 mg/kg xylazine, Rompun®, Bayer, Germany), transcardial in situ perfusions were performed using 4% paraformaldehyde in phosphate-buffered saline (PBS, Cat. No. 70011-036, Thermo Fisher Scientific, Vienna, Austria). Brains were carefully extracted and stored in the fixative solution for 24 h and then transferred to 30% w/w sucrose in PBS solution for an additional 24 h. Serial sections of 30 μm thickness were cut using a cryostat (CM1950, Leica, Vienna, Austria) and stored in cryoprotective solution (30% ethylene glycol, 30% glycerol in PBS) at −20°C until used for immunolabeling. Eighty sections per animal were collected, starting at Bregma −1.22 where the hippocampal structure becomes visible (see Figure 1A, middle panel; Franklin and Paxinos, 2013).
Pretreatment for BrdU Immunohistochemistry
In order to visualize the BrdU incorporation into the nuclear DNA, it is necessary to perform the heat/acid DNA denaturation step (Boulanger et al., 2016). Additionally, and in order to protect the integrity of the tissue from the harsh heat/acid treatment, sections were first incubated in 50% formamide in 2× SSC buffer solution (Cat. No. 15557-044, 0.3 M NaCl, 0.03 M sodium citrate, Thermo Fisher Scientific, Vienna, Austria) at 65°C for 90 min, then rinsed in 2× SSC buffer solution, that was followed by incubation in 2 M HCl for 30 min at 37°C, and finally quick 10 min incubation in 0.1 M borate buffer (pH = 9).
Immunohistochemistry
Every 10th free-foating section of the entire rostrocaudal extent of the hippocampus was used for immunohistochemical analysis (n = 7–8 animals per group). Multicolor immunofluorescence staining protocols for mature granular neurons were applied basically as described elsewhere (Pollak et al., 2008; Khan et al., 2014). In essence, after multiple washing steps, sections were blocked with 10% normal donkey serum (NDS, Cat. No. 017-000-121, Jackson ImmunoResearch) in Trizma base solution (TBS, Trizma® base, Sigma–Aldrich, Vienna, Austria) for 1 h, and subsequently incubated for 72 h at 4°C in 5% NDS blocking solution with following primary antibodies: anti-BrdU (marker of newly generated cells, 1:300, Cat. No. OBT0030G, Abd Serotec, Biorad, Vienna, Austria), anti-NeuN (mature neuron marker, 1:1,000, Cat. No. MAB377, Merck Millipore, Vienna, Austria). Next, sections were washed and incubated with appropriate secondary antibodies (1:500, Alexa Fluor®, Abcam, Cambridge, UK). Negative controls were performed by omitting the primary antibody. For the proliferation paradigm, cells were labeled with the anti-BrdU primary antibody and an Alexa Fluor secondary antibody conjugated with a 488 nm fluorophore. For the survival paradigm, sections were double-labeled with the anti-BrdU primary antibody and the Alexa Fluor secondary antibody conjugated with a 594 nm fluorophore and anti-NeuN primary antibody followed by an Alexa Fluor secondary antibody with a conjugated 488 nm fluorophore.
Stereology
Hippocampal immunofluorescence labeling was quantified conferring to a modified unbiased stereology protocol by an experimenter blinded to the genotype of the animals (Gould et al., 1999; Pollak et al., 2008). In the proliferation paradigm, the total number of cells in the well-defined region of the subgranular zone (SGZ) of the DG of both hippocampi in a 30 μm section was quantified throughout parallel series of sections (see Figure 1A, right). For the quantification of the number of cells, eight sections were quantified per animal. The resulting average number of cells per section per hippocampus was multiplied by the total number of sections to estimate the total number of newly generated cells. In the survival paradigm, all BrdU+ cells within the SGZ and approximately width of one cell above the SGZ, in order to account for cell migration were quantified in the same way as in the proliferation paradigm. The total number of BrdU+ cells in mice sacrificed 24 h after the last BrdU injection was used to evaluate the rate of proliferation. To assess survival rate, average numbers of BrdU+ cells per granule cell layer (GCL) per section were quantified in mice that were sacrificed 14 days following the BrdU injection. Quantification of the total number of cells that showed colocalization of BrdU labeling and immunoreactivity for mature granular neuronal marker- neuron-specific nuclear protein (NeuN+) was used to calculate the number of surviving neurons (Kempermann et al., 1997a,b; Khan et al., 2014). For double-labeled cells, only when it was confirmed that both Alexa Fluor 488 and Alexa Fluor 594 signals are in the same optical plane of focus, cells were scored as double-labeled. Cells that we in outer most, optical plane, were not scored in order to avoid counting cell caps (Huang et al., 1998). Fluorescent signals were detected using a Carl-Zeiss Axiovert-Apotome System (Oberkochen, Germany) coupled to Axiovision software version 4.8. at 20× magnification as previously described (Gould et al., 1999; Yu et al., 2009; Khan et al., 2014).
Electrophysiology
Hippocampal Slices Preparation
Acute hippocampal slices were prepared from behaviorally naïve Pdpn+/+ and Pdpn−/− male mice (n = 8–11 slices per group) following previously published protocols (Cicvaric et al., 2016, 2018a,b). In a nutshell, following a quick decapitation, animal’s brain was extracted into a cold artificial cerebrospinal fluid (aCSF) solution that had following composition (in mM): 125 NaCl, 2.5 KCl, 25 NaHCO3, 2 CaCl2, 1 MgCl2, 25 D-glucose, 1.25 NaH2PO4 with pH adjusted to 7.4. Next, transverse hippocampal slices (400 μm thick) were cut using a McIlwain Tissue Chopper (Mickle Laboratory Engineering, Guildford, Surrey, UK) and moved to recover for at least 1 h in the homemade submerged holding chamber that was maintained at (28 ± 2)°C. All solutions were perfused with carbogen gas mixture (95% O2, 5% CO2) during the course of the experiment.
Extracellular Recordings
For recording, individual slices were transferred to a submerged recording chamber of low volume that was perfused with a constant flow (2–3 ml/min) of carbonated aCSF solution. Evoked field excitatory postsynaptic potentials (fEPSPs) were recorded using a borosilicate glass pipettes that were pulled in a horizontal puller (Sutter Instrument, Novato, CA, USA) thus yielding a tip with resistance of 2–5 MΩ when filled with aCSF solution. Stimulation was done via custom-made Teflon-coated tungsten wire bipolar stimulating electrode (~50 μm diameter tip). The stimulating electrode and recording microelectrode tips were positioned to target two major functional regions of the hippocampal trisynaptic circuit, CA3-Schaffer Collateral-CA1 and medial perforant pathway (MPP) of DG following previously published protocols (Massa et al., 2011; Monje et al., 2012; Cicvaric et al., 2016, 2018a,b). Stimuli needed to produce 40–50% of maximal amplitude that was established by repeated stimulation with pulses of voltage (0–6 V, 1 V increments, 100 μs duration) were used for baseline recordings (pulse duration = 100 μs, 0.03 Hz). To induce LTD, low-frequency stimulation (LFS, 900 pulses at 1 Hz) protocol was delivered at baseline stimulation intensity using an ISO-STIM 01D isolator stimulator (NPI Electronics, Tamm, Germany). Synaptic depression was determined by analyzing the changes in the decaying phase of fEPSP slopes normalized to baseline, averaged within a group and compared between Pdpn+/+ and Pdpn−/− mice. AxoClamp-2B amplifier (Bridge mode) and a Digidata-1440 interface (Axon Instruments, Molecular Devices, Berkshire, UK) were used for recordings and pClamp-10 software (Molecular Devices, Berkshire, UK) was used for offline analysis.
Behavioral Testing
All experiments shown in this study were performed during the light phase of the light/dark cycle by an experimenter blinded to the genotype of the animals. Animals were singly housed for at least 1 week before the start of the experiment and handled daily by the experimenter for 5 min in order to reduce stress that is induced by manipulation of animals throughout the experiments. Additionally, on the day of each test, mice were transferred to their home cages for habituation to the testing room, 1 h prior to experiments. During habituation and throughout the experiments white noise generator was used so as to mask possible intermittent loud noises from the surroundings (Can et al., 2012).
Open Field Test
In addition to the evaluation of the alternations in general locomotor activity, the Open Field test (OFT) is used as a screening test for anxiety-related behavior (Prut and Belzung, 2003). Following previously published protocols (Cicvaric et al., 2018a,b), at the beginning of each session, Pdpn+/+ or Pdpn−/− mouse was placed in the center of an Open Field arena (Cat No. ENV-510, Med Associates Inc., St. Albans, VT, USA) that consisted of four transparent acrylic glass walls (height 20.3 cm) on a white polyvinyl chloride plastic square board (side 27.3 cm), and allowed to explore the arena for 5 min. At the end of each session, the subject was placed back to the home cage and the apparatus was thoroughly cleaned with 70% ethanol and used again only once the odor volatilized. To test for any possible changes in locomotor activity and exploratory behavior distance that each animal traveled in the arena was recorded and analyzed using associated software, Activity Monitor (Cat No. SOF-812, Med Associates Inc., St. Albans, VT, USA). Likewise, to test for alterations in anxiety-like behavior, the time spent in the virtual center of the arena (~25% of the total area) and the number of entries to the center were analyzed (Bailey and Crawley, 2009).
Light Dark Box
To further test for specific influence that genetic-targeting of Pdpn could have on anxiety-like behavior in mice we firstly employed the Light/Dark Box test (LDB) essentially as described before (Leach et al., 2013; Cicvaric et al., 2018a). Briefly, the OFT arena was modified by inserting a specialized, opaque Dark Box Insert (Cat No. ENV-511, Med Associates Inc., St. Albans, VT, USA) to enclose two equal-sized compartments, connected via small passage, with one section illuminated and the other one darkened. At the start of the experiment, the animal was positioned in the bright section facing away from the passage and allowed to explore freely both sections for 10 min. Once the session was completed, the animal was returned to the home cage and the test arena was carefully whipped with 70% ethanol and used again only once the scent volatilized to prevent a bias based on olfactory cues. Since mice instinctively avoid the brightly lit compartment, percentage of time spent in the lit area as well as number of crossings between compartments were automatically measured and recorded by a specialized software package (Cat No. SOF-812, Activity Monitor, Med Associates, St. Albans, VT, USA) and used as experimental indices of anxiety.
Elevated Plus-Maze
The elevated plus maze (EPM), currently one of the most popular tests to examine anxiety-like behaviors, is based on the mice’ innate fear of open, elevated areas. When given a choice, mice show an inclination to stay in the safe (closed arms) compared to risky (open arms) surroundings (Pinheiro et al., 2007; Ramos, 2008). Here, EPM was carried out following established, previously published protocols (Shumyatsky et al., 2002; Glangetas et al., 2017; Cicvaric et al., 2018a). Experiments were performed using a plus-shaped Plexiglas elevated (~50 cm above ground) apparatus (Viewpoint, Lyon, France) that consisted of two opposing closed and two opposing open arms. All arms of the EPM were 40 cm long and fitted with white floors, while closed arms had an additional dark insert with 20 cm high walls. Open arms were brightly illuminated (110 lx), while the closed arm had a dim illumination (15 lx). At the beginning of each 5 min session, the subject was placed at the juncture area (central square) so as to face one of the open arms and allowed to explore the maze without restrictions. Animal behavior was recorded and analyzed using an accompanying video tracking software (VideoTrack, Viewpoint, Lyon, France). Several conventional and ethological parameters like amount of time animal spent in the open arms, number of entries to open arms and total distance traveled in the maze during the test were evaluated as described before (Walf and Frye, 2007).
Neurogenesis Analysis in vitro
Embryonic Mouse Neural Stem Cell Culture
Timed-pregnant Pdpn−/− or Pdpn+/+ mice were killed by cervical dislocation and the pups quickly removed and decapitated in sterile Hank’s Balanced Salt Solution (HBSS, Cat. No. 14185052, GIBCO®, Thermo Fisher Scientific, Waltham, MA, USA) on Embryonic Day 15. Hippocampi were dissected, placed in a small volume of ice-cold standard Dulbecco’s Modified Eagle’s Medium (DMEM Thermo Fisher Scientific, Waltham, MA, USA) and used to prepare embryonic mouse neural stem cell (NSC) culture following standardized protocols described before (Brewer and Torricelli, 2007; Azari et al., 2011a,b,c; Siebzehnrubl et al., 2011). Dissected hippocampal tissue was cut and enzymatically dissociated using 1× Trypsin (Cat. No. 15090046, Thermo Fisher Scientific, Waltham, MA, USA) 37°C for 10 min. Trypsin was removed and dissociated tissue was gently washed four times with warm DMEM. Following mechanical dissociation, the cell suspension was centrifuged at 1,800 rpm for 2 min (Universal 320 from Hettich GMB, Tuttlingen, Germany, 10 cm rotor ratio, type 1554). Pellet was then re-suspended in warm NeurocultTM basal medium (Cat. No. 05700, Stem Cell Technologies, Vancouver, BC, Canada) supplemented with NeurocultTM proliferation supplement (Cat. No. 05701, Stem Cell Technologies, Vancouver, BC, Canada) and 20 ng/ml EGF (Cat. No. 02633, Stem Cell Technologies, Vancouver, BC, Canada) and plated at a density of 8 × 104 viable cells/cm2. Neurospheres were harvested at 5 DIV and frozen in 10% DMSO in NeurocultTM basal medium.
Differentiation
Cells were cultured following standard procedures but never sub-cultured (passaged) more than once in order to avoid possible alterations in self-renewal capability due to many passages (Azari et al., 2011c). After quick thawing frozen aliquots of the NSC were centrifuged and the pellet re-suspended in warm NeurocultTM basal medium supplemented with NeurocultTM proliferation supplement and 20 ng/ml EGF. After 24 h, primary spheres were passaged by incubation in 0.1× Trypsin for 1.5 min at 37°C. The activity of Trypsin was stopped with Trypsin inhibitor (Cat. No17075029). Thermo Fisher Scientific and the spheres were then mechanically dissociated into a single-cell suspension. 0.2 × 106 viable cells per coverslip for both genotypes were plated in DMEM medium (Cat. No. 21103049, Thermo Fisher Scientific, Waltham, MA, USA) supplemented with B27 supplement (Cat. No. 17504044, Thermo Fisher Scientific, Waltham, MA, USA). Coverslips were double-coated, first with Poly-L-Lysine (Cat. No. P6282, Sigma–Aldrich, Vienna, Austria), followed by a coat of laminin (Cat. No. L2020, Sigma–Aldrich, Vienna, Austria) to allow cells to adhere and differentiate. Cells were treated continuously every 24 h with NGF (50 ng/ml in DMEM with B27) for 4 or 8 days.
Immunocytochemistry
Cells were fixed with 4% PFA in PBS solution at appropriate time points. First, cells were blocked with 5% normal goat serum (NGS, Cat. No. X0907, Dako) and 5% normal donkey serum (NDS, Cat. No. 017-000-121, Jackson ImmunoResearch, West Grove, PA, USA) in PBS and then further incubated with anti-glial fibrillary acidic protein (GFAP; Cat. No. SAB5500113, rabbit polyclonal, 1:500, Sigma–Aldrich, St. Louis, MO, USA), anti-microtubule associated protein 2 (MAP2; Cat. No. ab5392, chicken polyclonal, 1:25,000, Abcam, Cambridge, UK) primary antibodies that were diluted in PBS containing 0.1% BSA (A8022, Sigma–Aldrich, St. Louis, MO, USA), 1% NGS, 1% NDS and 0.1% Triton X-100 (Cat. No. T8787, Sigma–Aldrich, St. Louis, MO, USA) for 48 h at 4°C. Next, cells were washed with PBS and subsequently incubated with Alexa Fluor 488 conjugated goat-anti-chicken IgGs (Cat. No. A11039, 1:1,000, Thermo Fisher Scientific, Waltham, MA, USA) and Alexa Fluor 594 conjugated donkey-anti-rabbit IgGs (Cat. No. A21207, 1:1,000, Thermo Fisher Scientific, Waltham, MA, USA) secondary antibodies diluted in 2% BSA in PBS solution for 1 h at room temperature. Negative controls were produced by omitting primary antibodies. Cell nuclei were stained with 4′,6-diamidino-2-phenylindole (DAPI, Cat. No. D1306, 1:1,000, Thermo Fisher Scientific, Waltham, MA, USA) diluted in PBS for 5 min at room temperature. Cells were washed with PBS, mounted with Fluoro-Gel mounting medium (Cat. No. 17985–10, Electron Microscopy Sciences, Hatfield, PA, USA) and viewed with a conventional fluorescence microscope. Six non-overlapping images per coverslip were captured at 40× magnification with a Zeiss Axiovert Colibri7 microscope and exported using the ZEN 2.3 (blue edition) software (Zeiss, Jena, Germany). Only cells overlapping with DAPI were further manually counted with ImageJ (NIH) using a cell counter plugin (Kurt De Vos, ImageJ, NIH).
Statistical Analysis
Statistical analyses were done using GraphPad Prism Software, version 7.0 (San Diego, CA, USA). To test for the normality of data, the D’Agostino’s K2 test was used prior to further statistical analyses. Two-sided unpaired student’s t-test was used for two-group comparisons and two-way ANOVA followed by Tukey’s test to account for multiple comparisons unless otherwise indicated. P, t, and F values together with the number of samples used (n) are presented in the figure legends. All data are expressed as means ± standard error. P < 0.05 was considered significant. *P < 0.05, **P < 0.01, ***P < 0.001, ****P < 0.0001. All source data used for the preparation of graphs and statistical analysis and other relevant data that support the conclusions presented in the study are available from the authors upon request.
Results
Lack of Pdpn Increases Proliferation of Cells in the Subgranular Zone of the Dentate Gyrus
To study the involvement of Pdpn in adult neurogenesis, we used a previously characterized Pdpn knockout mice (Uhrin et al., 2010; Cicvaric et al., 2016) and sought out to analyze in these mice the properties of cell proliferation and survival in the SGZ of the hippocampal DG by using BrdU-labeling as a strategy to determine newly formed cells (Figure 1A). Quantitative analysis 24 h post-injection indicated a significant increase in the number of BrdU+ cells in the SGZ of the Pdpn−/− compared to Pdpn+/+ mice (Figure 1B). No statistically significant differences in the number of BrdU+ cells were observed between SGZs of Pdpn−/− and Pdpn+/+ mice 14 days post-injection, indicating that while lack of Pdpn augmented the proliferation of neural progenitor cells in the SGZ, there was no difference in the number of surviving cells 2 weeks after BrdU injection (Figures 1B,C). Considering previously shown the differential contribution of the dorsal and ventral hippocampus to different sets of behaviors, we sought out to determine whether the changes we see in the proliferation rates will be confined to one and not the other part of the hippocampus. We found that while differences in rates of the proliferation between genotypes were conserved there was no significant difference in the proliferation rates between the dorsal and ventral hippocampus (Figure 1D). To determine whether the perturbations in proliferation could have had influence specifically on the survival of mature neurons, we performed multiplex labeling experiments with the neuron-specific nuclear protein NeuN and BrdU (BrdU+/NeuN+). No significant difference in the percentage of surviving neurons between Pdpn−/− and Pdpn+/+ mice was observed (Figure 1E).
Pdpn Deletion Alters Long-Term Depression in the Hippocampal Dentate Gyrus
In order to examine if Pdpn could be involved in bidirectional synaptic plasticity, we applied protocols of low-frequency electrical stimulation (LFS) to both the CA3-to-CA1 and MPP synaptic pathways in acute hippocampal slices from Pdpn+/+ and Pdpn−/− mice. Data showed that the induction and maintenance of LTD in CA3-Schaffer Collateral-CA1 pathway synapses of Pdpn−/− mice were indistinguishable from those from wild-type animals (Figure 2A). However, when LFS protocol was applied to the MPP pathway, it resulted in stronger maintenance of the induced LTD in slices from Pdpn−/− mice as compared to those from their control wild type counterparts (Figure 2B).
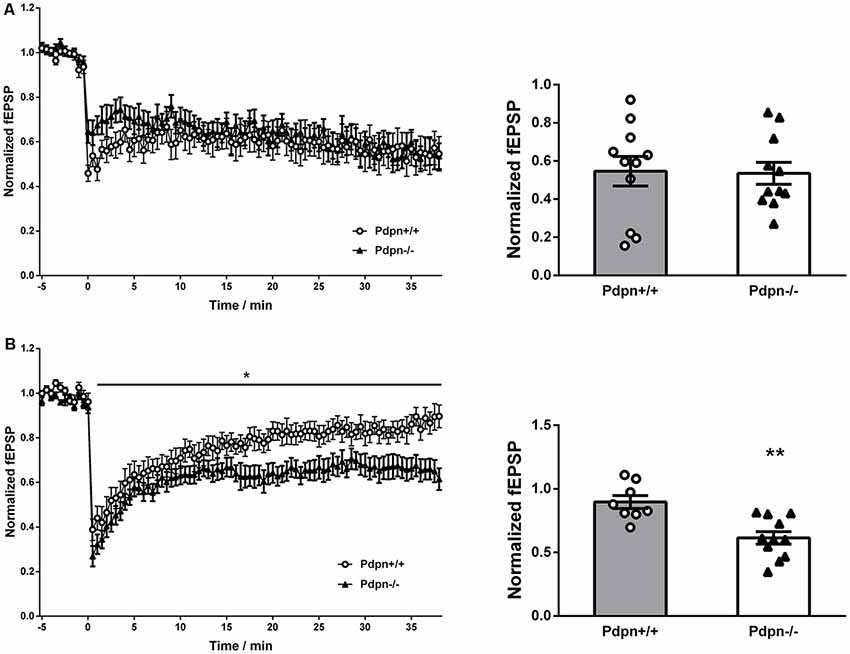
Figure 2. Podoplanin deletion alters long-term depression (LTD) selectively in the hippocampal DG. (A) Temporal courses of averaged slopes of fEPSP in CA3-Schaffer collateral-CA1 synapses (left panel) showed a strong main significant effect of time (****P < 0.0001, F(76,1520) = 3.72, n = 11), no significant main effect of genotype (P = 0.6, F(1,20) = 0.36, n = 11) and a significant interaction between time and genotype (***P = 0.0004, F(76,1520) = 1.7). Corresponding bar graphs of the end-time points (right panel) showed no significant differences between Pdpn+/+ and Pdpn−/− (t(20) = 0.1124, P = 0.9, n = 11). (B) Temporal courses of averaged slopes of fEPSP in medial perforant pathway (MPP) of DG (left panel) showed a strong main significant effect of time (****P < 0.0001, F(75,1275) = 27.87, n = 8–11), a moderate significant main effect of genotype (*P = 0.03, F(1,17) = 5.99, n = 8–11) and a significant interaction between time and genotype (****P < 0.0001, F(75,1275) = 1.88). Corresponding bar graphs of the end-time points (right panel) showed significant differences between Pdpn+/+ and Pdpn−/− (t(17) = 3.93, **P = 0.002, n = 8–11). Data are displayed as mean ± SEM.
Effects of Pdpn Deletion on Anxiety-Like Behavior
Since the process of neurogenesis has been previously linked to the expression of mood-related behaviors (Revest et al., 2009; Parihar et al., 2011; Anacker et al., 2018), we next implemented widely standardized behavioral tests aiming to examine whether absence of Pdpn would result in possible changes in anxiety-like behaviors. We first examined Pdpn+/+ and Pdpn−/− mice in the OFT and observed that Pdpn−/− mice showed significantly higher anxiety-like levels compared to their wild type littermates as indicated by a reduction in percentage of the time that Pdpn−/− mice spent in the center of the Open field arena (Figure 3A, left panel). No differences were found, however, in total distance traveled or the number of entries to the brightly lit center, indicating an absence of locomotor defects resulting from Pdpn deficiency (Figure 3A, middle and right panel). When both groups of animals were examined in the LDB, data indicated no significant differences in the percentage of time mice spent in the light area of the LDB arena, total distance traveled or number of entries to the light area of the test arena (Figure 3B). In the Elevated Plus Maze test (EPM), a very significant reduction in the time Pdpn−/− mice spent in the open arms of the maze was apparent, indicating increased levels of anxiety in Pdpn−/− mice compared to their wild type littermates (Figure 3C, left panel). A reduction in the distance traveled during the test in the open arms was also observed (Figure 3C, middle panel). No significant differences between groups were observed in the number of entries to the open arms (Figure 3C, right panel).
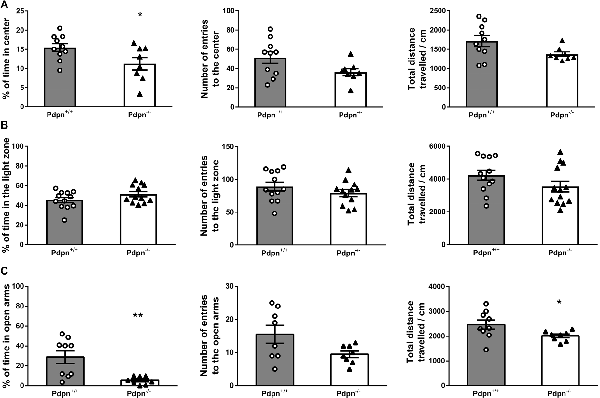
Figure 3. Effects of podoplanin deletion on anxiety-like behavior. Anxiety-like behavior was evaluated using several tests. (A) In Open Field test (OFT) significant difference in percentage of time spent in the brightly lit center between Pdpn−/− and their wild-type littermate was found (t(16) = 2.29, *P = 0.04, n = 8–10). No significant differences were observed in total distance traveled and the number of entries to the brightly lit center (t(16) = 2.05, P = 0.06, n = 8–10 and t(16) = 1.99, P = 0.07, n = 8–10). (B) No differences were observed in the percentage of time spent in the light area of the Light/Dark Box test (LDB) arena (t(22) = 1.61, P = 0.2, n = 12). Mice from both groups also showed comparable values for the total distances traveled and the number of entries to the LDB arena (t(23) = 1.54, P = 0.2, n = 12–13 and t(22) = 1.16, P = 0.3, n = 12). (C) Pdpn−/− animals presented with significantly less time spent in the open arms of the elevated plus-maze (t(15) = 3.37, **P = 0.005, n = 8–9) and significantly less traveled distance in the open arms (t(15) = 2.16, *P = 0.05, n = 8–9), as compared to their wild type littermates. No significant differences between groups were observed in the number of entries to the open arms (t(14) = 2.08, P = 0.06, n = 8). Data are displayed as mean ± SEM.
Lack of Pdpn Selectively Decreases the Survival of Neuronal Cells That Is Rescued by NGF Treatment
On the basis of our observations from in vivo neurogenesis experiments in Pdpn−/− mice, indicating significantly increased proliferation in the SGZ without alterations in the number of surviving neurons even after 2 weeks of cell-labeling, we asked ourselves whether this transitory enhanced proliferation phenotype was followed by cell death of newly-generated neuronal cells. In order to explore this possibility using an entirely different experimental approach, we prepared neurosphere cultures from wild type and Pdpn−/− mice, as other groups have successfully proven the advantages of conducting in vitro assays using NSCs when studying the structural morphological as well as the functional signaling mechanisms underlying cell differentiation and neurogenesis (Schramm and Schulte, 2014). Following cell differentiation, we tracked the survival of neuronal and glial cells throughout 8 days at 2 days intervals. The phenotype of the cells was determined using the specific markers microtubule-associated protein 2 (MAP2) for neurons and GFAP for glial cells (Figure 4C). While there were no significant differences in the survival of glial cells from Pdpn+/+ and Pdpn−/− mice, there was a significant sharp decrease in the number of surviving neuronal cells in cultures derived from Pdpn−/− mice, starting at day 4 (Figures 4A,B, upper panels). A particular strength of this assay its ability to easily study the activity of soluble factors on NSCs. We have previously shown that Pdpn can physically interact with NGF (Cicvaric et al., 2016), a very functionally relevant neurotrophin known to hold a significant role in the differentiation and survival of new neurons (Levi-Montalcini, 1987a,b). We therefore next quantified the number of neuronal and glial cells on day 4 following differentiation in the presence or absence of NGF. In neuronal cells, NGF treatment significantly increased the percentage of Pdpn−/− surviving cells, thus bringing it to the levels comparable to the Pdpn+/+ cells with or without NGF treatment, suggesting that NGF rescued the effect of Pdpn deletion. In glial cells, NGF treatment increased the number of Pdpn−/− surviving cells.
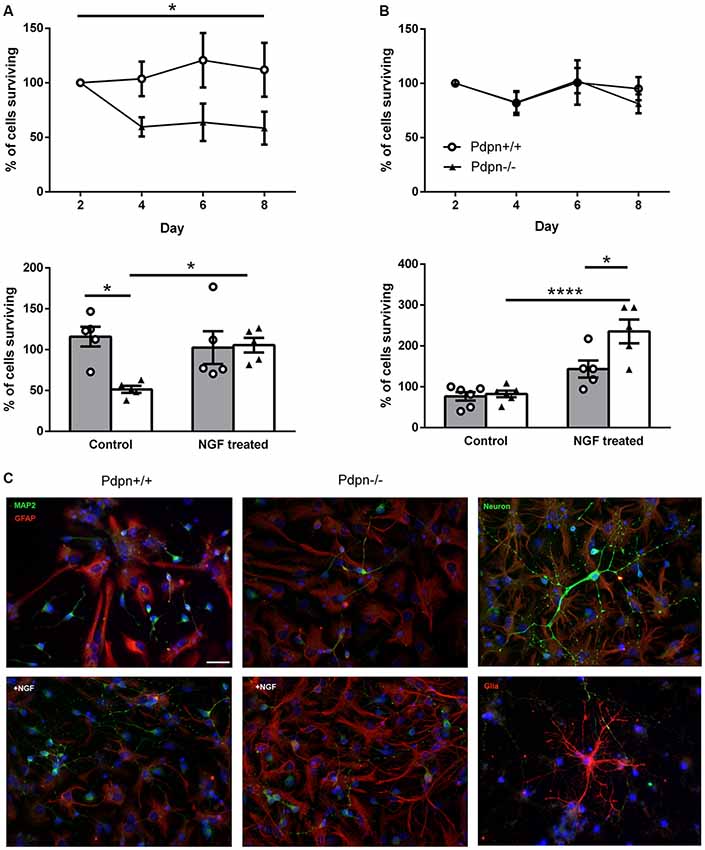
Figure 4. Lack of Podoplanin selectively decreases the survival of neuronal cells that are rescued by NGF treatment. Percentage of (A) neuronal and (B) glial cells derived from Pdpn+/+ and Pdpn−/− neurospheres surviving during 8 days (upper panels), showed a significant main effect of the genotype on number of surviving neurons (*P = 0.02, F(1,10) = 8.69, n = 6) but not on the number of glial cells (P = 0.8, F(1,8) = 0.07, n = 5). No significant effects of time were observed on a number of neuronal or glial cells. Percentage of (A) neuronal and (B) glial cells derived from Pdpn+/+ and Pdpn−/− neurospheres surviving after 4 days of NGF treatment, lower panels. In neuronal cells two-way ANOVA showed no significant effect of treatment (P = 0.2, F(1,16) = 2.556, n = 6) but a significant effect of genotype (*P = 0.03, F(1,16) = 5.84, n = 6) and a significant interaction between treatment and genotype (*P = 0.02, F(1,16) = 7.07). Post hoc analysis showed a significant difference between Pdpn+/+ and Pdpn−/− without treatment (*P = 0.02) and between Pdpn−/− with and without NGF treatment (*P = 0.059), as represented with asterisk on the graph. Analysis of glial cells showed a strong main significant effect of treatment (****P < 0.0001, F(1,18) = 38.73, n = 5), significant main effect of the genotype (*P = 0.02, F(1,18) = 7.695, n = 5) and a significant interaction between two factors (*P = 0.03, F(1,18) = 5.942, n = 5). Post hoc analysis showed a significant difference between Pdpn−/− with and without NGF treatment (****P < 0.0001) and between Pdpn+/+ and Pdpn−/− when treated with NGF (*P = 0.02), as represented with an asterisk on the graph. (C) Representative images of differentiated neurospheres immunolabeled with neuronal (MAP2) and glial (GFAP) markers (left and central pictures). In the right, upper and lower pictures, comprise representative images of immuno-based identified neurons (upper) and glial (lower) cells. Data are displayed as mean ± SEM.
Discussion
Pdpn and Neurogenesis in the Adult Brain
The role of Pdpn has been widely studied in last decades in several different disciplines including vascular physiology, cardiology, urology, and pulmonology). In the brain, Pdpn has been also shown to be expressed in hippocampal neurons and in nestin-positive neural progenitor stem cells in the SGZ of the hippocampal DG (Kotani et al., 2002, 2003; Astarita et al., 2012; Tomooka et al., 2013; Song et al., 2014), a region central for the acquisition of memory functions (Goncalves et al., 2016; Milner and Klein, 2016). Yet, most of the available studies about Pdpn in the brain have focused on its involvement in the promotion of brain tumors (Astarita et al., 2012; Peterziel et al., 2012; Grau et al., 2015; Kolar et al., 2015) and the intrinsic role of Pdpn in neurogenesis and memory-related synaptic plasticity in the adult brain remained undetermined. Here, we propose Pdpn as a molecular player involved in the functional fine-tuning of hippocampal neurogenesis. In agreement with our results here, previous reports had linked Pdpn to the regulation of cellular proliferation (Ramirez et al., 2003; Williams, 2003; Spinella et al., 2009; Cortez et al., 2010; Yan et al., 2011; Peterziel et al., 2012; Acton et al., 2014; Grau et al., 2015); and novel in vitro models studying stem cell and glioma invasion have also revealed elevated levels of Pdpn in migrating and invasive NSCs (Sailer et al., 2013). Additionally, studies in P19 embryonic carcinoma cells that can be differentiated into neuronal cells, and in neuronal stem cells that were sorted out based on their Pdpn expression levels, had shown that precursor cells with higher levels of Pdpn expression exhibit higher self-renewal capabilities and greater variability in their differentiation profiles (multi-lineage differentiation into neural cells), whereas precursor cells with lower levels of Pdpn expression displayed low self-renewability and differentiated predominantly into neurons (Kotani et al., 2002, 2003, 2007). Studies in vitro from other groups had moreover shown the presence of Pdpn in hippocampal nestin-positive neural progenitor cells (Kotani et al., 2002, 2003, 2007). All these observations are in agreement with our findings and support our hypothesis for an involvement of Pdpn in the regulation of the in vivo proliferative properties of hippocampal neural progenitor stem cells; our in vivo neurogenesis experiments show that deletion of Pdpn significantly increases the proliferation of NSCs in the SZG of the DG, hence hinting that Pdpn might be part of a proliferation suppression pathway (Figure 1B).
The findings linking Pdpn to hippocampal neurogenesis are timely and relevant. Neurogenesis has been described in several species and is associated to the genesis of a number of severe brain neuropathologies (Jin et al., 2004; He and Shen, 2009; Chuang, 2010; Demars et al., 2010; Spalding et al., 2013; Regensburger et al., 2014; Bergmann et al., 2015; Ernst and Frisén, 2015; Verdaguer et al., 2015; Frisén, 2016; Kang et al., 2016; Boldrini et al., 2018; Kempermann et al., 2018; Lucassen et al., 2019; Moreno-Jiménez et al., 2019). Neurogenesis is also implicated in the regulation of synaptic plasticity and learning and memory processes (Snyder et al., 2001; Dupret et al., 2008; Akers et al., 2014; Lazarov and Hollands, 2016). However, the mechanism regulating neurogenesis, synaptic plasticity and mood- and memory-related functions continue to be poorly understood and the specific signaling pathways linking Pdpn to these processes remain to be elucidated. There are several molecular and functional interacting partners of Pdpn that are expressed in brain neurons; that have the potential to crosstalk with Pdpn to influence neurogenesis; and which had been implicated in synaptic transmission, synaptic plasticity, neuronal morphology, neurogenesis and/or memory-related functions. Some of these proteins include RhoA (O’Kane et al., 2003, 2004; Dash et al., 2004; Wang et al., 2005; Martín-Villar et al., 2006; Diana et al., 2007), CDC42 (Okabe et al., 2003; Kim et al., 2014), Ezrin (Martín-Villar et al., 2006; Kim et al., 2010; Marsick et al., 2012), CD44 (Roszkowska et al., 2016), and NGF (Conner et al., 2009; Wang et al., 2012; Uzakov et al., 2015; Cicvaric et al., 2016). In particular, Ezrin is an interesting prospect to jointly mediate with Pdpn in the regulation of in vivo neurogenesis. In non-neuronal cells, it has been shown that Pdpn can exert its function via interactions with Ezrin, which is known to regulate neurotrophin-induced remodeling of F-actin and cell growth (Martín-Villar et al., 2006; Fernández-Muñoz et al., 2011; Smith and Melrose, 2011), and neuronal growth and morphology via redistribution of adhesion receptors (Sizemore et al., 2007). Recent studies have moreover unveiled Ezrin as a mediator of neurogenesis (Matsumoto et al., 2014) via down-regulation of the activity of RhoA, which is on itself a known downstream mediator of the Pdpn activity (Martín-Villar et al., 2006; Mahtab et al., 2009). Previous studies from our group have also demonstrated that brain neuronal Pdpn deletion results in altered levels of phospho-Ezrin upon stimulation with NGF (Cicvaric et al., 2016). Additionally, not only was NGF previously related to the regulation of neurogenesis and modulation of proliferation and migration of neural precursor cells (Maisonpierre et al., 1990; Chen et al., 2010; Birch et al., 2013; Tzeng et al., 2013; Zhang et al., 2013; Campos et al., 2014; Montalban et al., 2014; Oliveira et al., 2015; Sarma et al., 2015; Martorana et al., 2018; Mondal and Fatima, 2019), but our group has recently demonstrated (using Surface Plasmon Resonance) that Pdpn can directly interact with NGF and that Pdpn deletion alters the response of brain neurons to NGF stimulation (Cicvaric et al., 2016). Furthermore, both Pdpn and Ezrin protein expression have been identified in the neurogenic plexus choroids (Ming and Song, 2011) lateral ventricles region (Cleary et al., 2006; Kaji et al., 2010; Persson et al., 2010; Sathyanesan et al., 2012; Moon et al., 2013; Tomooka et al., 2013). Additionally, both Pdpn and the p75 NGF Receptor have been also previously associated with the regulation of cell migratory activity of NSCs and their invasive motility properties (Sailer et al., 2013). All these observations, together with our data here, provide several hints for future research aiming to elucidate the specific molecular pathways mechanistically linking Pdpn to in vivo neurogenesis in the adult mammalian brain.
Pdpn Mediates in the Neuroprotective Effects of NGF
The experimental use of neurospheres cultures is widespread, as under specific conditions one can differentiate neurospheres into a variety of cells, including neurons, which provide thus a useful tool to examine molecular events critical for both the proliferation and differentiation stages of neurogenesis (Siebzehnrubl et al., 2011; Morte et al., 2013). In the in vitro experiments using neurospheres presented here, knockdown of Pdpn induced highly significant extensive cell death starting 4 days after cell differentiation (Figure 4A), indicating that Pdpn might be implicated in cell survival. Interestingly, this phenomenon was only observed in non-mitotic neuronal cells, as lack of Pdpn had no effect on the glial cell population (Figure 4B). NGF is a critical modulator of brain neuronal survival, neurotransmission, synaptic plasticity, and learning memory functions (Zhou et al., 1995; Conner et al., 2009; Zhang et al., 2013). NGF has been additionally implicated in the molecular signaling pathways regulating hippocampal neurogenesis (Birch et al., 2013; Zhang et al., 2013; Toyoda et al., 2014; Martorana et al., 2018). Our group has previously examined the involvement of Pdpn in neuritic outgrowth under basal conditions and in response to NGF using hippocampus-derived cultured neurons from Pdpn+/+ and Pdpn−/− mice, and found that Pdpn mediates in the effects of NGF on hippocampal neuron neuritic outgrowth (Cicvaric et al., 2016). These observations support our hypothesis for the role of Pdpn in neuronal survival and for the Pdpn-dependent incorporation of newly generated neurons into existing circuits through cooperative signaling with NGF. To challenge this hypothesis, we cultured NSCs from wild type and Pdpn knockout mice for 4 days following differentiation and observed significantly higher amounts of cell death in cultures lacking Pdpn; an effect that was completely rescued by NGF treatment (Figure 4A). Given the importance of cell survival for the process of neurogenesis (Baptista and Andrade, 2018), our data thus for the first time propose Pdpn not only as a potential molecular candidate to influence neurogenesis, but also as a possible molecular element mediating in the NGF-dependent cellular mechanisms preserving the integrity of recently formed neurons in vivo.
Pdpn: A Bidirectional Regulator of Synaptic Plasticity
During the last 40 years, a large number of studies on hippocampal synaptic plasticity have proven LTP as a powerful experimental tool to study the mechanisms that might lie beneath the hippocampal function, and learning acquisition and memory retention (Nguyen et al., 1994; Toni et al., 1999; Abraham and Williams, 2003; Sweatt, 2003a,b; Zakharenko et al., 2003). Information continues to be comparatively scarce, however, relating to molecular elements influencing hippocampal LTD (Kemp and Manahan-Vaughan, 2004; Etkin et al., 2006; Malleret et al., 2010). Previous reports have demonstrated that alterations in DG neurogenesis can influence both synaptic potentiation and synaptic depression at CA3-Schaffer collateral-CA1 and Medial Perforant Path-DG synapses (Wang et al., 2000; Snyder et al., 2001, 2005; Schmidt-Hieber et al., 2004; Saxe et al., 2006; Ge et al., 2007; Massa et al., 2011). These observations indicate that neurogenesis not only does not exclusively influences synaptic activity at a single hippocampal sub-region but its effects are not limited to synaptic potentiation. Indeed, in this type of experiment, the reestablishment of neurogenesis results in the full restitution of both synaptic potentiation and depression, with the latter requiring a much longer time to reach complete recovery (Massa et al., 2011). The molecular elements relating neurogenesis to both LTP and LTD remain, however, poorly characterized (Holderbach et al., 2007). Additionally, previous studies have established that both LTP and LTD are influenced by adult neurogenesis in the DG (Kitamura et al., 2010; Massa et al., 2011; Alam et al., 2018). It has been also shown that while newborn neurons—following their incorporation in the DG network—can improve the facilitation of the expression of LTP and LTD in DG, this process happens in a sequential time-dependent manner (Massa et al., 2011). In our previously established Pdpn knockout mouse model, we reported that the deletion of Pdpn selectively impairs long-term synaptic plasticity in the hippocampal DG (Cicvaric et al., 2016). However, the understanding of the contribution of Pdpn to synaptic transmission and plasticity in the DG remains far from complete. For example, while an impact of Pdpn deletion on synaptic potentiation was unveiled (Cicvaric et al., 2016), other forms of plasticity, such as synaptic depression, remained to be examined for its possible relation to the Pdpn function. Here, we expand these studies and further unveil that Pdpn gene deletion also influences LTD selectively at the DG (Figure 2). Our data thus for the first time propose Pdpn as one of the potential in vivo bidirectional and sub-region selective regulators of neurogenesis and memory-related synaptic plasticity.
Podoplanin and the Crosstalk Between Neurogenesis, Synaptic Depression, and Anxiety-Like Behavior
Using the mouse as an experimental model organism, we here implemented for the first time a basic behavioral screening addressing the effects of Pdpn gene deletion on anxiety-related behavior. Previous research had established a physiological link between altered neurogenesis and depression- (Jacobs et al., 2000; Sahay and Hen, 2007; Lucassen et al., 2010; Eisch and Petrik, 2012) and anxiety-like behaviors (Revest et al., 2009; Baptista and Andrade, 2018). Our data had additionally shown that the deletion of Pdpn resulted in both altered neural progenitor cell proliferation and impaired LTD specifically at the neurogenic DG. LTD has been associated to memory-related cognitive impairment and to anxiety and mood-related disorders (Rolls, 2013; King et al., 2014; Leal et al., 2017). Changes in adult neurogenesis in the DG are also known to be importantly implicated in the anxiety- and depression-like behaviors in experimental animal models (Santarelli et al., 2003; Warner-Schmidt and Duman, 2006; Lagace et al., 2010; Parihar et al., 2011; Eisch and Petrik, 2012; Petrik et al., 2012; Aiello et al., 2015; Anacker et al., 2018; Cantacorps et al., 2018). Our experimental results indicated, for the first time, that Pdpn deletion associates with an apparent preference for avoiding the brightly lit center of an open space in the OF test (Figure 3A), which is a behavior indicative of increased anxiety (Olivier et al., 2008; Bailey and Crawley, 2009; Hughes et al., 2014; Kulesskaya and Voikar, 2014; Sturman et al., 2018). These observations were consistent with the data obtained during the EPM test (Figure 3C), which is also widely used as an indicator of anxiety-related behavior (Bailey and Crawley, 2009) and which further propose the possibility that Pdpn might serve a physiological role in the prevention of the expression of anxiety-related behaviors that might occur in concomitance with altered neurogenesis. Only the LDB test showed no significant differences between wild type and Pdpn knockout animals (Figure 3B). Differences in results in the LDB could be therefore indicative of subtle variances in the manifestation of what is defined as “mood,” which in certain cases might not be reflected in all of the different tests. For example, other groups have described that differences in the results from behavioral mood-related tests might become apparent not only due to the intrinsic differences of the experimental setting itself (as for example possible changes in the transparency or the degree of illumination) but can become exacerbated in specific strains, pharmacological interventions or genetic modifications (Hogg, 1996; Bourin and Hascoét, 2003; Hagenbuch et al., 2006; Ramos, 2008; Violle et al., 2009; Miller et al., 2011; Steimer, 2011). Further research from other groups and using other behavioral tests (e.g., Social Interaction Tests), is therefore encouraged in order to independently validate our observations. Taken together, data presented here for the first time propose Pdpn as a potential molecular component mediating in the regulation of neurogenesis and in the expression of anxiety-related behaviors. Further experiments using alternative Pdpn-down-regulation approaches like CRISPR-Cas9-based (Cong et al., 2013; Horvath et al., 2017; Ma et al., 2017) targeted Pdpn gene deletion could additionally contribute to the verification of the here proposed in vivo role of Pdpn in neurogenesis, NGF-mediated neuroprotection and anxiety-related behaviors.
Data Availability Statement
The datasets generated for this study are available on request to the corresponding author.
Ethics Statement
The animal study was reviewed and approved by the National Ethical Committee on animal care and use (BMWFW104 66.009/0201-WF/II/3b/2014, Bundesministerium für Wissenschaft, Forschung und Wirtschaft).
Author Contributions
FM conceived the project. AC and FM designed the experiments and performed most of the behavioral, electrophysiological, cell culture experiments and immunohistochemical analyses, supervised the data analysis, interpretation and jointly wrote the full body of the manuscript. HS helped with immunohistological studies and independently replicated parts of the immunohistological results. TSt performed immunocytological experiments and data quantification. TSm advised on molecular experiments and conducted data analysis. DS and TM independently replicated some of the cell culture experiments and helped with parts of the writing of the manuscript. PU provided the animals used in the study and helped with the data interpretation. All authors discussed the findings presented in this study and commented on the manuscript.
Funding
AC, PU and FM were supported by the Austrian Science Fund [FWF: Project Number P_27551].
Conflict of Interest
The authors declare that the research was conducted in the absence of any commercial or financial relationships that could be construed as a potential conflict of interest.
References
Abraham, W. C., and Williams, J. M. (2003). Properties and mechanisms of LTP maintenance. Neuroscientist 9, 463–474. doi: 10.1177/1073858403259119
Abrous, D. N., Koehl, M., and Le Moal, M. (2005). Adult neurogenesis: from precursors to network and physiology. Physiol. Rev. 85, 523–569. doi: 10.1152/physrev.00055.2003
Acton, S. E., Farrugia, A. J., Astarita, J. L., Mourão-Sá, D., Jenkins, R. P., Nye, E., et al. (2014). Dendritic cells control fibroblastic reticular network tension and lymph node expansion. Nature 514, 498–502. doi: 10.1038/nature13814
Aiello, R., Crupi, R., Leo, A., Chimirri, S., Rispoli, V., Marra, R., et al. (2015). Long-term β-methasone 21-phosphate disodium treatment has distinct effects in CD1 and DBA/2 mice on animal behavior accompanied by opposite effects on neurogenesis. Behav. Brain Res. 278, 155–166. doi: 10.1016/j.bbr.2014.09.042
Akers, K. G., Martinez-Canabal, A., Restivo, L., Yiu, A. P., De Cristofaro, A., Hsiang, H. L., et al. (2014). Hippocampal neurogenesis regulates forgetting during adulthood and infancy. Science 344, 598–602. doi: 10.1126/science.1248903
Alam, M. J., Kitamura, T., Saitoh, Y., Ohkawa, N., Kondo, T., and Inokuchi, K. (2018). Adult neurogenesis conserves hippocampal memory capacity. J. Neurosci. 38, 6854–6863. doi: 10.1523/JNEUROSCI.2976-17.2018
Anacker, C., Denny, C. A., and Hen, R. (2015). Regulation of hippocampal memory traces by neurogenesis. Neurogenesis 2:e1025180. doi: 10.1080/23262133.2015.1025180
Anacker, C., Luna, V. M., Stevens, G. S., Millette, A., Shores, R., Jimenez, J. C., et al. (2018). Hippocampal neurogenesis confers stress resilience by inhibiting the ventral dentate gyrus. Nature 559, 98–102. doi: 10.1038/s41586-018-0262-4
Astarita, J. L., Acton, S. E., and Turley, S. J. (2012). Podoplanin: emerging functions in development, the immune system, and cancer. Front. Immunol. 3:283. doi: 10.3389/fimmu.2012.00283
Azari, H., Louis, S. A., Sharififar, S., Vedam-Mai, V., and Reynolds, B. A. (2011a). Neural-colony forming cell assay: an assay to discriminate bona fide neural stem cells from neural progenitor cells. J. Vis. Exp. 49:2639. doi: 10.3791/2639
Azari, H., Millette, S., Ansari, S., Rahman, M., Deleyrolle, L. P., and Reynolds, B. A. (2011b). Isolation and expansion of human glioblastoma multiforme tumor cells using the neurosphere assay. J. Vis. Exp. 56:e3633. doi: 10.3791/3633
Azari, H., Sharififar, S., Rahman, M., Ansari, S., and Reynolds, B. A. (2011c). Establishing embryonic mouse neural stem cell culture using the neurosphere assay. J. Vis. Exp. 47:2457. doi: 10.3791/2457
Bailey, K. R., and Crawley, J. N. (2009). “Chapter 5-Anxiety-related behaviors in mice,” in Methods of Behavior Analysis in Neuroscience, 2nd Edn., ed. J. J. Buccafusco (Boca Raton, FL: CRC Press/Taylor & Francis).
Baptista, P., and Andrade, J. P. (2018). Adult hippocampal neurogenesis: regulation and possible functional and clinical correlates. Front. Neuroanat. 12:44. doi: 10.3389/fnana.2018.00044
Bergmann, O., Spalding, K. L., and Frisen, J. (2015). Adult neurogenesis in humans. Cold Spring Harb. Perspect. Biol. 7:a018994. doi: 10.1101/cshperspect.a018994
Birch, A. M., McGarry, N. B., and Kelly, A. M. (2013). Short-term environmental enrichment, in the absence of exercise, improves memory and increases NGF concentration, early neuronal survival, and synaptogenesis in the dentate gyrus in a time-dependent manner. Hippocampus 23, 437–450. doi: 10.1002/hipo.22103
Boldrini, M., Fulmore, C. A., Tartt, A. N., Simeon, L. R., Pavlova, I., Poposka, V., et al. (2018). Human hippocampal neurogenesis persists throughout aging. Cell Stem Cell 22, 589.e5–599.e5. doi: 10.1016/j.stem.2018.03.015
Boulanger, J. J., Staines, W. A., LeBlanc, V., Khoo, E. L., Liang, J., and Messier, C. (2016). A simple histological technique to improve immunostaining when using DNA denaturation for BrdU labelling. J. Neurosci. Methods 259, 40–46. doi: 10.1016/j.jneumeth.2015.11.006
Bourin, M., and Hascoét, M. (2003). The mouse light/dark box test. Eur. J. Pharmacol. 463, 55–65. doi: 10.1016/s0014-2999(03)01274-3
Breiteneder-Geleff, S., Matsui, K., Soleiman, A., Meraner, P., Poczewski, H., Kalt, R., et al. (1997). Podoplanin, novel 43-kd membrane protein of glomerular epithelial cells, is down-regulated in puromycin nephrosis. Am. J. Pathol. 151, 1141–1152.
Brewer, G. J., and Torricelli, J. R. (2007). Isolation and culture of adult neurons and neurospheres. Nat. Protoc. 2, 1490–1498. doi: 10.1038/nprot.2007.207
Campos, A. C., Vaz, G. N., Saito, V. M., and Teixeira, A. L. (2014). Further evidence for the role of interferon-γ on anxiety- and depressive-like behaviors: involvement of hippocampal neurogenesis and NGF production. Neurosci. Lett. 578, 100–105. doi: 10.1016/j.neulet.2014.06.039
Can, A., Dao, D. T., Arad, M., Terrillion, C. E., Piantadosi, S. C., and Gould, T. D. (2012). The mouse forced swim test. J. Vis. Exp. 59:e3638. doi: 10.3791/3638
Cantacorps, L., González-Pardo, H., Arias, J. L., Valverde, O., and Conejo, N. M. (2018). Altered brain functional connectivity and behaviour in a mouse model of maternal alcohol binge-drinking. Prog. Neuropsychopharmacol. Biol. Psychiatry 84, 237–249. doi: 10.1016/j.pnpbp.2018.03.006
Chen, Y. L., Monteith, N., Law, P. Y., and Loh, H. H. (2010). Dynamic association of p300 with the promoter of the G protein-coupled rat delta opioid receptor gene during NGF-induced neuronal differentiation. Biochem. Biophys. Res. Commun. 396, 294–298. doi: 10.1016/j.bbrc.2010.04.083
Chuang, T. T. (2010). Neurogenesis in mouse models of Alzheimer’s disease. Biochim. Biophys. Acta 1802, 872–880. doi: 10.1016/j.bbadis.2009.12.008
Cicvaric, A., Bulat, T., Bormann, D., Yang, J., Auer, B., Milenkovic, I., et al. (2018a). Sustained consumption of cocoa-based dark chocolate enhances seizure-like events in the mouse hippocampus. Food Funct. 9, 1532–1544. doi: 10.1039/c7fo01668a
Cicvaric, A., Yang, J., Bulat, T., Zambon, A., Dominguez-Rodriguez, M., Kühn, R., et al. (2018b). Enhanced synaptic plasticity and spatial memory in female but not male FLRT2-haplodeficient mice. Sci. Rep. 8:3703. doi: 10.1038/s41598-018-22030-4
Cicvaric, A., Yang, J., Krieger, S., Khan, D., Kim, E. J., Dominguez-Rodriguez, M., et al. (2016). The brain-tumor related protein podoplanin regulates synaptic plasticity and hippocampus-dependent learning and memory. Ann. Med. 48, 625–668. doi: 10.1080/07853890.2016.1219455
Cleary, M. A., Uboha, N., Picciotto, M. R., and Beech, R. D. (2006). Expression of ezrin in glial tubes in the adult subventricular zone and rostral migratory stream. Neuroscience 143, 851–861. doi: 10.1016/j.neuroscience.2006.08.028
Colucci-D’Amato, L., Bonavita, V., and di Porzio, U. (2006). The end of the central dogma of neurobiology: stem cells and neurogenesis in adult CNS. Neurol. Sci. 27, 266–270. doi: 10.1007/s10072-006-0682-z
Cong, L., Ran, F. A., Cox, D., Lin, S., Barretto, R., Habib, N., et al. (2013). Multiplex genome engineering using CRISPR/Cas systems. Science 339, 819–823. doi: 10.1126/science.1231143
Conner, J. M., Franks, K. M., Titterness, A. K., Russell, K., Merrill, D. A., Christie, B. R., et al. (2009). NGF is essential for hippocampal plasticity and learning. J. Neurosci. 29, 10883–10889. doi: 10.1523/JNEUROSCI.2594-09.2009
Cortez, M. A., Nicoloso, M. S., Shimizu, M., Rossi, S., Gopisetty, G., Molina, J. R., et al. (2010). miR-29b and miR-125a regulate podoplanin and suppress invasion in glioblastoma. Genes Chromosomes Cancer 49, 981–990. doi: 10.1002/gcc.20808
Costa, V., Lugert, S., and Jagasia, R. (2015). Role of adult hippocampal neurogenesis in cognition in physiology and disease: pharmacological targets and biomarkers. Handb. Exp. Pharmacol. 228, 99–155. doi: 10.1007/978-3-319-16522-6_4
Dash, P. K., Orsi, S. A., Moody, M., and Moore, A. N. (2004). A role for hippocampal Rho-ROCK pathway in long-term spatial memory. Biochem. Biophys. Res. Commun. 322, 893–898. doi: 10.1016/j.bbrc.2004.08.004
Demars, M., Hu, Y. S., Gadadhar, A., and Lazarov, O. (2010). Impaired neurogenesis is an early event in the etiology of familial Alzheimer’s disease in transgenic mice. J. Neurosci. Res. 88, 2103–2117. doi: 10.1002/jnr.22387
Dennis, C. V., Suh, L. S., Rodriguez, M. L., Kril, J. J., and Sutherland, G. T. (2016). Human adult neurogenesis across the ages: an immunohistochemical study. Neuropathol. Appl. Neurobiol. 42, 621–638. doi: 10.1111/nan.12337
Diana, G., Valentini, G., Travaglione, S., Falzano, L., Pieri, M., Zona, C., et al. (2007). Enhancement of learning and memory after activation of cerebral Rho GTPases. Proc. Natl. Acad. Sci. U S A 104, 636–641. doi: 10.1073/pnas.0610059104
Dupret, D., Revest, J. M., Koehl, M., Ichas, F., De Giorgi, F., Costet, P., et al. (2008). Spatial relational memory requires hippocampal adult neurogenesis. PLoS One 3:e1959. doi: 10.1371/journal.pone.0001959
Eisch, A. J., and Petrik, D. (2012). Depression and hippocampal neurogenesis: a road to remission? Science 338, 72–75. doi: 10.1126/science.1222941
Eriksson, P. S., Perfilieva, E., Bjork-Eriksson, T., Alborn, A. M., Nordborg, C., Peterson, D. A., et al. (1998). Neurogenesis in the adult human hippocampus. Nat. Med. 4, 1313–1317. doi: 10.1038/3305
Ernst, A., and Frisén, J. (2015). Adult neurogenesis in humans- common and unique traits in mammals. PLoS Biol. 13:e1002045. doi: 10.1371/journal.pbio.1002045
Etkin, A., Alarcon, J. M., Weisberg, S. P., Touzani, K., Huang, Y. Y., Nordheim, A., et al. (2006). A role in learning for SRF: deletion in the adult forebrain disrupts LTD and the formation of an immediate memory of a novel context. Neuron 50, 127–143. doi: 10.1016/j.neuron.2006.03.013
Fernández-Muñoz, B., Yurrita, M. M., Martín-Villar, E., Carrasco-Ramírez, P., Megías, D., Renart, J., et al. (2011). The transmembrane domain of podoplanin is required for its association with lipid rafts and the induction of epithelial-mesenchymal transition. Int. J. Biochem. Cell Biol. 43, 886–896. doi: 10.1016/j.biocel.2011.02.010
Franklin, K. B. J., and Paxinos, G. (2013). Paxinos and Franklin’s the mouse brain in stereotaxic coordinates. 4th Edn. Amsterdam: Academic Press, an imprint of Elsevier.
Frisén, J. (2016). Neurogenesis and gliogenesis in nervous system plasticity and repair. Annu. Rev. Cell Dev. Biol. 32, 127–141. doi: 10.1146/annurev-cellbio-111315-124953
Ge, S., Yang, C. H., Hsu, K. S., Ming, G. L., and Song, H. (2007). A critical period for enhanced synaptic plasticity in newly generated neurons of the adult brain. Neuron 54, 559–566. doi: 10.1016/j.neuron.2007.05.002
Gittenberger-de Groot, A. C., Mahtab, E. A., Hahurij, N. D., Wisse, L. J., Deruiter, M. C., Wijffels, M. C., et al. (2007). Nkx2.5-negative myocardium of the posterior heart field and its correlation with podoplanin expression in cells from the developing cardiac pacemaking and conduction system. Anat. Rec. 290, 115–122. doi: 10.1002/ar.20406
Glangetas, C., Massi, L., Fois, G. R., Jalabert, M., Girard, D., Diana, M., et al. (2017). NMDA-receptor-dependent plasticity in the bed nucleus of the stria terminalis triggers long-term anxiolysis. Nat. Commun. 8:14456. doi: 10.1038/ncomms14456
Goncalves, J. T., Schafer, S. T., and Gage, F. H. (2016). Adult neurogenesis in the hippocampus: from stem cells to behavior. Cell 167, 897–914. doi: 10.1016/j.cell.2016.10.021
Gould, E., Beylin, A., Tanapat, P., Reeves, A., and Shors, T. J. (1999). Learning enhances adult neurogenesis in the hippocampal formation. Nat. Neurosci. 2, 260–265. doi: 10.1038/6365
Grau, S. J., Trillsch, F., Tonn, J. C., Goldbrunner, R. H., Noessner, E., Nelson, P. J., et al. (2015). Podoplanin increases migration and angiogenesis in malignant glioma. Int. J. Clin. Exp. Pathol. 8, 8663–8670.
Hagenbuch, N., Feldon, J., and Yee, B. K. (2006). Use of the elevated plus-maze test with opaque or transparent walls in the detection of mouse strain differences and the anxiolytic effects of diazepam. Behav. Pharmacol. 17, 31–41. doi: 10.1097/01.fbp.0000189811.77049.3e
Hagg, T. (2009). From neurotransmitters to neurotrophic factors to neurogenesis. Neuroscientist 15, 20–27. doi: 10.1177/1073858408324789
He, P., and Shen, Y. (2009). Interruption of β-catenin signaling reduces neurogenesis in Alzheimer’s disease. J. Neurosci. 29, 6545–6557. doi: 10.1523/JNEUROSCI.0421-09.2009
Hogg, S. (1996). A review of the validity and variability of the elevated plus-maze as an animal model of anxiety. Pharmacol. Biochem. Behav. 54, 21–30. doi: 10.1016/0091-3057(95)02126-4
Holderbach, R., Clark, K., Moreau, J. L., Bischofberger, J., and Normann, C. (2007). Enhanced long-term synaptic depression in an animal model of depression. Biol. Psychiatry 62, 92–100. doi: 10.1016/j.biopsych.2006.07.007
Hollands, C., Bartolotti, N., and Lazarov, O. (2016). Alzheimer’s disease and hippocampal adult neurogenesis; exploring shared mechanisms. Front. Neurosci. 10:178. doi: 10.3389/fnins.2016.00178
Hollands, C., Tobin, M. K., Hsu, M., Musaraca, K., Yu, T. S., Mishra, R., et al. (2017). Depletion of adult neurogenesis exacerbates cognitive deficits in Alzheimer’s disease by compromising hippocampal inhibition. Mol. Neurodegener. 12:64. doi: 10.1186/s13024-017-0207-7
Hong, X. P., Peng, C. X., Wei, W., Tian, Q., Liu, Y. H., Cao, F. Y., et al. (2011). Relationship of adult neurogenesis with tau phosphorylation and GSK-3β activity in subventricular zone. Neurochem. Res. 36, 288–296. doi: 10.1007/s11064-010-0316-y
Hong, X. P., Peng, C. X., Wei, W., Tian, Q., Liu, Y. H., Yao, X. Q., et al. (2010). Essential role of tau phosphorylation in adult hippocampal neurogenesis. Hippocampus 20, 1339–1349. doi: 10.1002/hipo.20712
Horvath, P. M., Kavalali, E. T., and Monteggia, L. M. (2017). CRISPR/Cas9 system-mediated impairment of synaptobrevin/VAMP function in postmitotic hippocampal neurons. J. Neurosci. Methods 278, 57–64. doi: 10.1016/j.jneumeth.2016.12.015
Huang, L., DeVries, G. J., and Bittman, E. L. (1998). Photoperiod regulates neuronal bromodeoxyuridine labeling in the brain of a seasonally breeding mammal. J. Neurobiol. 36, 410–420. doi: 10.1002/(sici)1097-4695(19980905)36:3<410::aid-neu8>3.0.co;2-z
Hughes, R. N., Hancock, N. J., Henwood, G. A., and Rapley, S. A. (2014). Evidence for anxiolytic effects of acute caffeine on anxiety-related behavior in male and female rats tested with and without bright light. Behav. Brain Res. 271, 7–15. doi: 10.1016/j.bbr.2014.05.038
Jacobs, B. L., van Praag, H., and Gage, F. H. (2000). Adult brain neurogenesis and psychiatry: a novel theory of depression. Mol. Psychiatry 5, 262–269. doi: 10.1038/sj.mp.4000712
Jin, K., Peel, A. L., Mao, X. O., Xie, L., Cottrell, B. A., Henshall, D. C., et al. (2004). Increased hippocampal neurogenesis in Alzheimer’s disease. Proc. Natl. Acad. Sci. U S A 101, 343–347. doi: 10.1073/pnas.2634794100
Jouvenceau, A., Hedou, G., Potier, B., Kollen, M., Dutar, P., and Mansuy, I. M. (2006). Partial inhibition of PP1 alters bidirectional synaptic plasticity in the hippocampus. Eur. J. Neurosci. 24, 564–572. doi: 10.1111/j.1460-9568.2006.04938.x
Kaji, C., Tomooka, M., Kato, Y., Kojima, H., and Sawa, Y. (2010). The expression of podoplanin and classic cadherins in the mouse brain. J. Anat. 220, 435–446. doi: 10.1111/j.1469-7580.2012.01484.x
Kang, E., Wen, Z., Song, H., Christian, K. M., and Ming, G. L. (2016). Adult neurogenesis and psychiatric disorders. Cold Spring Harb. Perspect. Biol. 8:a019026. doi: 10.1101/cshperspect.a019026
Kemp, A., and Manahan-Vaughan, D. (2004). Hippocampal long-term depression and long-term potentiation encode different aspects of novelty acquisition. Proc. Natl. Acad. Sci. U S A 101, 8192–8197. doi: 10.1073/pnas.0402650101
Kemp, A., and Manahan-Vaughan, D. (2007). Hippocampal long-term depression: master or minion in declarative memory processes? Trends Neurosci. 30, 111–118. doi: 10.1016/j.tins.2007.01.002
Kempermann, G., Gage, F. H., Aigner, L., Song, H., Curtis, M. A., Thuret, S., et al. (2018). Human adult neurogenesis: evidence and remaining questions. Cell Stem Cell 23, 25–30. doi: 10.1016/j.stem.2018.04.004
Kempermann, G., Kuhn, H. G., and Gage, F. H. (1997a). Genetic influence on neurogenesis in the dentate gyrus of adult mice. Proc. Natl. Acad. Sci. U S A 94, 10409–10414. doi: 10.1073/pnas.94.19.10409
Kempermann, G., Kuhn, H. G., and Gage, F. H. (1997b). More hippocampal neurons in adult mice living in an enriched environment. Nature 386, 493–495. doi: 10.1038/386493a0
Khan, D., Fernando, P., Cicvaric, A., Berger, A., Pollak, A., Monje, F. J., et al. (2014). Long-term effects of maternal immune activation on depression-like behavior in the mouse. Transl. Psychiatry 4:e363. doi: 10.1038/tp.2013.132
Kim, H. S., Bae, C. D., and Park, J. (2010). Glutamate receptor-mediated phosphorylation of ezrin/radixin/moesin proteins is implicated in filopodial protrusion of primary cultured hippocampal neuronal cells. J. Neurochem. 113, 1565–1576. doi: 10.1111/j.1471-4159.2010.06713.x
Kim, I. H., Wang, H., Soderling, S. H., and Yasuda, R. (2014). Loss of Cdc42 leads to defects in synaptic plasticity and remote memory recall. Elife 3:e02839. doi: 10.7554/elife.02839
King, M. K., Pardo, M., Cheng, Y., Downey, K., Jope, R. S., and Beurel, E. (2014). Glycogen synthase kinase-3 inhibitors: rescuers of cognitive impairments. Pharmacol. Ther. 141, 1–12. doi: 10.1016/j.pharmthera.2013.07.010
Kitamura, T., Saitoh, Y., Murayama, A., Sugiyama, H., and Inokuchi, K. (2010). LTP induction within a narrow critical period of immature stages enhances the survival of newly generated neurons in the adult rat dentate gyrus. Mol. Brain 3:13. doi: 10.1186/1756-6606-3-13
Kolar, K., Freitas-Andrade, M., Bechberger, J. F., Krishnan, H., Goldberg, G. S., Naus, C. C., et al. (2015). Podoplanin: a marker for reactive gliosis in gliomas and brain injury. J. Neuropathol. Exp. Neurol. 74, 64–74. doi: 10.1097/nen.0000000000000150
Kotani, M., Okamoto, S., Imada, M., Itoh, K., Irie, A., Sakuraba, H., et al. (2007). Flow cytometric analysis of mouse neurospheres based on the expression level of RANDAM-2. Neurosci. Lett. 413, 25–30. doi: 10.1016/j.neulet.2006.10.066
Kotani, M., Osanai, T., Tajima, Y., Kato, H., Imada, M., Kaneda, H., et al. (2002). Identification of neuronal cell lineage-specific molecules in the neuronal differentiation of P19 EC cells and mouse central nervous system. J. Neurosci. Res. 67, 595–606. doi: 10.1002/jnr.10150
Kotani, M., Tajima, Y., Osanai, T., Irie, A., Iwatsuki, K., Kanai-Azuma, M., et al. (2003). Complementary DNA cloning and characterization of RANDAM-2, a type I membrane molecule specifically expressed on glutamatergic neuronal cells in the mouse cerebrum. J. Neurosci. Res. 73, 603–613. doi: 10.1002/jnr.10696
Kulesskaya, N., and Voikar, V. (2014). Assessment of mouse anxiety-like behavior in the light-dark box and open-field arena: role of equipment and procedure. Physiol. Behav. 133, 30–38. doi: 10.1016/j.physbeh.2014.05.006
Lagace, D. C., Donovan, M. H., Decarolis, N. A., Farnbauch, L. A., Malhotra, S., Berton, O., et al. (2010). Adult hippocampal neurogenesis is functionally important for stress-induced social avoidance. Proc. Natl. Acad. Sci. U S A 107, 4436–4441. doi: 10.1073/pnas.0910072107
Lazarov, O., and Hollands, C. (2016). Hippocampal neurogenesis: learning to remember. Prog. Neurobiol. 138–140, 1–18. doi: 10.1016/j.pneurobio.2015.12.006
Leach, G., Adidharma, W., and Yan, L. (2013). Depression-like responses induced by daytime light deficiency in the diurnal grass rat (Arvicanthis niloticus). PLoS One 8:e57115. doi: 10.1371/journal.pone.0057115
Leal, G., Bramham, C. R., and Duarte, C. B. (2017). BDNF and hippocampal synaptic plasticity. Vitam. Horm. 104, 153–195. doi: 10.1016/bs.vh.2016.10.004
Levi-Montalcini, R. (1987a). The nerve growth factor 35 years later. Science 237, 1154–1162. doi: 10.1126/science.3306916
Levi-Montalcini, R. (1987b). The nerve growth factor: thirty-five years later. EMBO J. 6, 1145–1154. doi: 10.1002/j.1460-2075.1987.tb02347.x
Liu, Z., and Martin, L. J. (2006). The adult neural stem and progenitor cell niche is altered in amyotrophic lateral sclerosis mouse brain. J. Comp. Neurol. 497, 468–488. doi: 10.1002/cne.21012
Llorens-Martin, M., Torres-Aleman, I., and Trejo, J. L. (2010). Exercise modulates insulin-like growth factor 1-dependent and -independent effects on adult hippocampal neurogenesis and behaviour. Mol. Cell. Neurosci. 44, 109–117. doi: 10.1016/j.mcn.2010.02.006
Lucassen, P. J., Meerlo, P., Naylor, A. S., van Dam, A. M., Dayer, A. G., Fuchs, E., et al. (2010). Regulation of adult neurogenesis by stress, sleep disruption, exercise and inflammation: implications for depression and antidepressant action. Eur. Neuropsychopharmacol. 20, 1–17. doi: 10.1016/j.euroneuro.2009.08.003
Lucassen, P. J., Toni, N., Kempermann, G., Frisen, J., Gage, F. H., and Swaab, D. F. (2019). Limits to human neurogenesis—really? Mol. Psychiatry doi: 10.1038/s41380-018-0337-5 [Epub ahead of print].
Ma, Y., Yu, L., Pan, S., Gao, S., Chen, W., Zhang, X., et al. (2017). CRISPR/Cas9-mediated targeting of the Rosa26 locus produces Cre reporter rat strains for monitoring Cre-loxP-mediated lineage tracing. FEBS J. 284, 3262–3277. doi: 10.1111/febs.14188
Mahtab, E. A., Vicente-Steijn, R., Hahurij, N. D., Jongbloed, M. R., Wisse, L. J., DeRuiter, M. C., et al. (2009). Podoplanin deficient mice show a RhoA-related hypoplasia of the sinus venosus myocardium including the sinoatrial node. Dev. Dyn. 238, 183–193. doi: 10.1002/dvdy.21819
Maisonpierre, P. C., Belluscio, L., Friedman, B., Alderson, R. F., Wiegand, S. J., Furth, M. E., et al. (1990). NT-3, BDNF, and NGF in the developing rat nervous system: parallel as well as reciprocal patterns of expression. Neuron 5, 501–509. doi: 10.1016/0896-6273(90)90089-x
Malenka, R. C., and Bear, M. F. (2004). LTP and LTD: an embarrassment of riches. Neuron 44, 5–21. doi: 10.1016/j.neuron.2004.09.012
Malleret, G., Alarcon, J. M., Martel, G., Takizawa, S., Vronskaya, S., Yin, D., et al. (2010). Bidirectional regulation of hippocampal long-term synaptic plasticity and its influence on opposing forms of memory. J. Neurosci. 30, 3813–3825. doi: 10.1523/JNEUROSCI.1330-09.2010
Marsick, B. M., San Miguel-Ruiz, J. E., and Letourneau, P. C. (2012). Activation of ezrin/radixin/moesin mediates attractive growth cone guidance through regulation of growth cone actin and adhesion receptors. J. Neurosci. 32, 282–296. doi: 10.1523/JNEUROSCI.4794-11.2012
Martín-Villar, E., Megías, D., Castel, S., Yurrita, M. M., Vilaró, S., and Quintanilla, M. (2006). Podoplanin binds ERM proteins to activate RhoA and promote epithelial-mesenchymal transition. J. Cell Sci. 119, 4541–4553. doi: 10.1242/jcs.03218
Martorana, F., Gaglio, D., Bianco, M. R., Aprea, F., Virtuoso, A., Bonanomi, M., et al. (2018). Differentiation by nerve growth factor (NGF) involves mechanisms of crosstalk between energy homeostasis and mitochondrial remodeling. Cell Death Dis. 9:391. doi: 10.1038/s41419-018-0429-9
Massa, F., Koehl, M., Wiesner, T., Grosjean, N., Revest, J. M., Piazza, P. V., et al. (2011). Conditional reduction of adult neurogenesis impairs bidirectional hippocampal synaptic plasticity. Proc. Natl. Acad. Sci. U S A 108, 6644–6649. doi: 10.1073/pnas.1016928108
Matsumoto, Y., Inden, M., Tamura, A., Hatano, R., Tsukita, S., and Asano, S. (2014). Ezrin mediates neuritogenesis via down-regulation of RhoA activity in cultured cortical neurons. PLoS One 9:e105435. doi: 10.1371/journal.pone.0105435
Meshi, D., Drew, M. R., Saxe, M., Ansorge, M. S., David, D., Santarelli, L., et al. (2006). Hippocampal neurogenesis is not required for behavioral effects of environmental enrichment. Nat. Neurosci. 9, 729–731. doi: 10.1038/nn1696
Miller, S. M., Piasecki, C. C., and Lonstein, J. S. (2011). Use of the light-dark box to compare the anxiety-related behavior of virgin and postpartum female rats. Pharmacol. Biochem. Behav. 100, 130–137. doi: 10.1016/j.pbb.2011.08.002
Milner, B., and Klein, D. (2016). Loss of recent memory after bilateral hippocampal lesions: memory and memories-looking back and looking forward. J. Neurol. Neurosurg. Psychiatry 87:230. doi: 10.1136/jnnp-2015-311092
Ming, G. L., and Song, H. (2011). Adult neurogenesis in the mammalian brain: significant answers and significant questions. Neuron 70, 687–702. doi: 10.1016/j.neuron.2011.05.001
Mondal, A. C., and Fatima, M. (2019). Direct and indirect evidences of BDNF and NGF as key modulators in depression: role of antidepressants treatment. Int. J. Neurosci. 129, 283–296. doi: 10.1080/00207454.2018.1527328
Monje, F. J., Kim, E. J., Pollak, D. D., Cabatic, M., Li, L., Baston, A., et al. (2012). Focal adhesion kinase regulates neuronal growth, synaptic plasticity and hippocampus-dependent spatial learning and memory. Neurosignals 20, 1–14. doi: 10.1159/000330193
Montalban, E., Mattugini, N., Ciarapica, R., Provenzano, C., Savino, M., Scagnoli, F., et al. (2014). MiR-21 is an Ngf-modulated microRNA that supports Ngf signaling and regulates neuronal degeneration in PC12 cells. Neuromolecular Med. 16, 415–430. doi: 10.1007/s12017-014-8292-z
Moon, Y., Kim, J. Y., Choi, S. Y., Cho, H. M., Kim, H., and Sun, W. (2013). Expression of ezrin in subventricular zone neural stem cells and their progeny in adult and developing mice. Histochem. Cell Biol. 139, 403–413. doi: 10.1007/s00418-012-1048-7
Moreno-Jiménez, E. P., Flor-García, M., Terreros-Roncal, J., Rábano, A., Cafini, F., Pallas-Bazarra, N., et al. (2019). Adult hippocampal neurogenesis is abundant in neurologically healthy subjects and drops sharply in patients with Alzheimer’s disease. Nat. Med. 25, 554–560. doi: 10.1038/s41591-019-0375-9
Morte, M. I., Carreira, B. P., Machado, V., Carmo, A., Nunes-Correia, I., Carvalho, C. M., et al. (2013). Evaluation of proliferation of neural stem cells in vitro and in vivo. Curr. Protoc. Stem Cell Biol. 2:2D.14. doi: 10.1002/9780470151808.sc02d14s24
Nakao, K., Ikegaya, Y., Yamada, M. K., Nishiyama, N., and Matsuki, N. (2002). Hippocampal long-term depression as an index of spatial working memory. Eur. J. Neurosci. 16, 970–974. doi: 10.1046/j.1460-9568.2002.02159.x
Nguyen, P. V., Abel, T., and Kandel, E. R. (1994). Requirement of a critical period of transcription for induction of a late phase of LTP. Science 265, 1104–1107. doi: 10.1126/science.8066450
Nishijima, T., Llorens-Martin, M., Tejeda, G. S., Inoue, K., Yamamura, Y., Soya, H., et al. (2013). Cessation of voluntary wheel running increases anxiety-like behavior and impairs adult hippocampal neurogenesis in mice. Behav. Brain Res. 245, 34–41. doi: 10.1016/j.bbr.2013.02.009
Okabe, T., Nakamura, T., Nishimura, Y. N., Kohu, K., Ohwada, S., Morishita, Y., et al. (2003). RICS, a novel GTPase-activating protein for Cdc42 and Rac1, is involved in the β-catenin-N-cadherin and N-methyl-D-aspartate receptor signaling. J. Biol. Chem. 278, 9920–9927. doi: 10.1074/jbc.m208872200
O’Kane, E. M., Stone, T. W., and Morris, B. J. (2003). Activation of Rho GTPases by synaptic transmission in the hippocampus. J. Neurochem. 87, 1309–1312. doi: 10.1046/j.1471-4159.2003.02102.x
O’Kane, E. M., Stone, T. W., and Morris, B. J. (2004). Increased long-term potentiation in the CA1 region of rat hippocampus via modulation of GTPase signalling or inhibition of Rho kinase. Neuropharmacology 46, 879–887. doi: 10.1016/j.neuropharm.2003.11.020
Oliveira, S. L., Trujillo, C. A., Negraes, P. D., and Ulrich, H. (2015). Effects of ATP and NGF on proliferation and migration of neural precursor cells. Neurochem. Res. 40, 1849–1857. doi: 10.1007/s11064-015-1674-2
Olivier, J. D., Van Der Hart, M. G., Van Swelm, R. P., Dederen, P. J., Homberg, J. R., Cremers, T., et al. (2008). A study in male and female 5-HT transporter knockout rats: an animal model for anxiety and depression disorders. Neuroscience 152, 573–584. doi: 10.1016/j.neuroscience.2007.12.032
Parihar, V. K., Hattiangady, B., Kuruba, R., Shuai, B., and Shetty, A. K. (2011). Predictable chronic mild stress improves mood, hippocampal neurogenesis and memory. Mol. Psychiatry 16, 171–183. doi: 10.1038/mp.2009.130
Persson, A., Lindwall, C., Curtis, M. A., and Kuhn, H. G. (2010). Expression of ezrin radixin moesin proteins in the adult subventricular zone and the rostral migratory stream. Neuroscience 167, 312–322. doi: 10.1016/j.neuroscience.2010.01.035
Peterziel, H., Müller, J., Danner, A., Barbus, S., Liu, H. K., Radlwimmer, B., et al. (2012). Expression of podoplanin in human astrocytic brain tumors is controlled by the PI3K-AKT-AP-1 signaling pathway and promoter methylation. Neuro Oncol. 14, 426–439. doi: 10.1093/neuonc/nos055
Petrik, D., Lagace, D. C., and Eisch, A. J. (2012). The neurogenesis hypothesis of affective and anxiety disorders: are we mistaking the scaffolding for the building? Neuropharmacology 62, 21–34. doi: 10.1016/j.neuropharm.2011.09.003
Pinheiro, S. H., Zangrossi, H. Jr., Del-Ben, C. M., and Graeff, F. G. (2007). Elevated mazes as animal models of anxiety: effects of serotonergic agents. An. Acad. Bras. Cienc. 79, 71–85. doi: 10.1590/s0001-37652007000100010
Pollak, D. D., Monje, F. J., Zuckerman, L., Denny, C. A., Drew, M. R., and Kandel, E. R. (2008). An animal model of a behavioral intervention for depression. Neuron 60, 149–161. doi: 10.1016/j.neuron.2008.07.041
Prut, L., and Belzung, C. (2003). The open field as a paradigm to measure the effects of drugs on anxiety-like behaviors: a review. Eur. J. Pharmacol. 463, 3–33. doi: 10.1016/s0014-2999(03)01272-x
Ramirez, M. I., Millien, G., Hinds, A., Cao, Y., Seldin, D. C., and Williams, M. C. (2003). T1α, a lung type I cell differentiation gene, is required for normal lung cell proliferation and alveolus formation at birth. Dev. Biol. 256, 61–72. doi: 10.1016/s0012-1606(02)00098-2
Ramos, A. (2008). Animal models of anxiety: do I need multiple tests? Trends Pharmacol. Sci. 29, 493–498. doi: 10.1016/j.tips.2008.07.005
Regensburger, M., Prots, I., and Winner, B. (2014). Adult hippocampal neurogenesis in Parkinson’s disease: impact on neuronal survival and plasticity. Neural Plast. 2014:454696. doi: 10.1155/2014/454696
Revest, J. M., Dupret, D., Koehl, M., Funk-Reiter, C., Grosjean, N., Piazza, P. V., et al. (2009). Adult hippocampal neurogenesis is involved in anxiety-related behaviors. Mol. Psychiatry 14, 959–967. doi: 10.1038/mp.2009.15
Rishi, A. K., Joyce-Brady, M., Fisher, J., Dobbs, L. G., Floros, J., Vanderspek, J., et al. (1995). Cloning, characterization, and development expression of a rat lung alveolar type I cell gene in embryonic endodermal and neural derivatives. Dev. Biol. 167, 294–306. doi: 10.1006/dbio.1995.1024
Rolls, E. T. (2013). The mechanisms for pattern completion and pattern separation in the hippocampus. Front. Syst. Neurosci. 7:74. doi: 10.3389/fnsys.2013.00074
Roszkowska, M., Skupien, A., Wójtowicz, T., Konopka, A., Gorlewicz, A., Kisiel, M., et al. (2016). CD44: a novel synaptic cell adhesion molecule regulating structural and functional plasticity of dendritic spines. Mol. Biol. Cell 27, 4055–4066. doi: 10.1091/mbc.e16-06-0423
Sahay, A., and Hen, R. (2007). Adult hippocampal neurogenesis in depression. Nat. Neurosci. 10, 1110–1115. doi: 10.1038/nn1969
Sailer, M. H., Gerber, A., Tostado, C., Hutter, G., Cordier, D., Mariani, L., et al. (2013). Non-invasive neural stem cells become invasive in vitro by combined FGF2 and BMP4 signaling. J. Cell Sci. 126, 3533–3540. doi: 10.1242/jcs.125757
Sajikumar, S., and Frey, J. U. (2004). Late-associativity, synaptic tagging, and the role of dopamine during LTP and LTD. Neurobiol. Learn. Mem. 82, 12–25. doi: 10.1016/j.nlm.2004.03.003
Santarelli, L., Saxe, M., Gross, C., Surget, A., Battaglia, F., Dulawa, S., et al. (2003). Requirement of hippocampal neurogenesis for the behavioral effects of antidepressants. Science 301, 805–809. doi: 10.1126/science.1083328
Sarma, T., Koutsouris, A., Yu, J. Z., Krbanjevic, A., Hope, T. J., and Rasenick, M. M. (2015). Activation of microtubule dynamics increases neuronal growth via the nerve growth factor (NGF)- and Gαs-mediated signaling pathways. J. Biol. Chem. 290, 10045–10056. doi: 10.1074/jbc.m114.630632
Sathyanesan, M., Girgenti, M. J., Banasr, M., Stone, K., Bruce, C., Guilchicek, E., et al. (2012). A molecular characterization of the choroid plexus and stress-induced gene regulation. Transl. Psychiatry 2:e139. doi: 10.1038/tp.2012.64
Saxe, M. D., Battaglia, F., Wang, J. W., Malleret, G., David, D. J., Monckton, J. E., et al. (2006). Ablation of hippocampal neurogenesis impairs contextual fear conditioning and synaptic plasticity in the dentate gyrus. Proc. Natl. Acad. Sci. U S A 103, 17501–17506. doi: 10.1073/pnas.0607207103
Saxe, M. D., Malleret, G., Vronskaya, S., Mendez, I., Garcia, A. D., Sofroniew, M. V., et al. (2007). Paradoxical influence of hippocampal neurogenesis on working memory. Proc. Natl. Acad. Sci. U S A 104, 4642–4646. doi: 10.1073/pnas.0611718104
Schmidt-Hieber, C., Jonas, P., and Bischofberger, J. (2004). Enhanced synaptic plasticity in newly generated granule cells of the adult hippocampus. Nature 429, 184–187. doi: 10.1038/nature02553
Schramm, J., and Schulte, D. (2014). A fast and simple differentiation protocol to study the pro-neurogenic activity of soluble factors in neurospheres. Neurosci. Lett. 562, 69–74. doi: 10.1016/j.neulet.2014.01.003
Shibahara, J., Kashima, T., Kikuchi, Y., Kunita, A., and Fukayama, M. (2006). Podoplanin is expressed in subsets of tumors of the central nervous system. Virchows Arch. 448, 493–499. doi: 10.1007/s00428-005-0133-x
Shumyatsky, G. P., Tsvetkov, E., Malleret, G., Vronskaya, S., Hatton, M., Hampton, L., et al. (2002). Identification of a signaling network in lateral nucleus of amygdala important for inhibiting memory specifically related to learned fear. Cell 111, 905–918. doi: 10.1016/s0092-8674(02)01116-9
Siebzehnrubl, F. A., Vedam-Mai, V., Azari, H., Reynolds, B. A., and Deleyrolle, L. P. (2011). Isolation and characterization of adult neural stem cells. Methods Mol. Biol. 750, 61–77. doi: 10.1007/978-1-61779-145-1_4
Sizemore, S., Cicek, M., Sizemore, N., Ng, K. P., and Casey, G. (2007). Podocalyxin increases the aggressive phenotype of breast and prostate cancer cells in vitro through its interaction with ezrin. Cancer Res. 67, 6183–6191. doi: 10.1158/0008-5472.can-06-3575
Smith, S. M., and Melrose, J. (2011). Podoplanin is expressed by a sub-population of human foetal rib and knee joint rudiment chondrocytes. Tissue Cell 43, 39–44. doi: 10.1016/j.tice.2010.11.003
Snyder, J. S., Hong, N. S., McDonald, R. J., and Wojtowicz, J. M. (2005). A role for adult neurogenesis in spatial long-term memory. Neuroscience 130, 843–852. doi: 10.1016/j.neuroscience.2004.10.009
Snyder, J. S., Kee, N., and Wojtowicz, J. M. (2001). Effects of adult neurogenesis on synaptic plasticity in the rat dentate gyrus. J. Neurophysiol. 85, 2423–2431. doi: 10.1152/jn.2001.85.6.2423
Song, Y., Shen, J., Lin, Y., Shen, J., Wu, X., Yan, Y., et al. (2014). Up-regulation of podoplanin involves in neuronal apoptosis in LPS-induced neuroinflammation. Cell. Mol. Neurobiol. 34, 839–849. doi: 10.1007/s10571-014-0060-y
Spalding, K. L., Bergmann, O., Alkass, K., Bernard, S., Salehpour, M., Huttner, H. B., et al. (2013). Dynamics of hippocampal neurogenesis in adult humans. Cell 153, 1219–1227. doi: 10.1016/j.cell.2013.05.002
Spinella, F., Garrafa, E., Di Castro, V., Rosanò, L., Nicotra, M. R., Caruso, A., et al. (2009). Endothelin-1 stimulates lymphatic endothelial cells and lymphatic vessels to grow and invade. Cancer Res. 69, 2669–2676. doi: 10.1158/0008-5472.can-08-1879
Staubli, U., and Lynch, G. (1990). Stable depression of potentiated synaptic responses in the hippocampus with 1-5 Hz stimulation. Brain Res. 513, 113–118. doi: 10.1016/0006-8993(90)91096-y
Staubli, U., Larson, J., and Lynch, G. (1990). Mossy fiber potentiation and long-term potentiation involve different expression mechanisms. Synapse 5, 333–335. doi: 10.1002/syn.890050410
Steimer, T. (2011). Animal models of anxiety disorders in rats and mice: some conceptual issues. Dialogues Clin. Neurosci. 13, 495–506.
Sturman, O., Germain, P. L., and Bohacek, J. (2018). Exploratory rearing: a context- and stress-sensitive behavior recorded in the open-field test. Stress 21, 443–452. doi: 10.1080/10253890.2018.1438405
Sweatt, J. D. (2003a). “Chapter 6—the biochemistry of LTP induction,” in Mechanisms of Memory, ed. J. David Sweatt (San Diego, CA: Academic Press), 147–188.
Sweatt, J. D. (2003b). “Chapter 9—LTP does not equal memory,” in Mechanisms of Memory, ed. J. David Sweatt (San Diego, CA: Academic Press), 263–306.
Toda, T., Parylak, S. L., Linker, S. B., and Gage, F. H. (2019). The role of adult hippocampal neurogenesis in brain health and disease. Mol. Psychiatry 24, 67–87. doi: 10.1038/s41380-018-0036-2
Tomooka, M., Kaji, C., Kojima, H., and Sawa, Y. (2013). Distribution of podoplanin-expressing cells in the mouse nervous systems. Acta Histochem. Cytochem. 46, 171–177. doi: 10.1267/ahc.13035
Toni, N., Buchs, P. A., Nikonenko, I., Bron, C. R., and Muller, D. (1999). LTP promotes formation of multiple spine synapses between a single axon terminal and a dendrite. Nature 402, 421–425. doi: 10.1038/46574
Tononi, G., and Cirelli, C. (2006). Sleep function and synaptic homeostasis. Sleep Med. Rev. 10, 49–62. doi: 10.1016/j.smrv.2005.05.002
Toyoda, A., Iio, W., Goto, T., Koike, H., and Tsukahara, T. (2014). Differential expression of genes encoding neurotrophic factors and their receptors along the septal-temporal axis of the rat hippocampus. Anim. Sci. J. 85, 986–993. doi: 10.1111/asj.12268
Trejo, J. L., Llorens-Martin, M. V., and Torres-Aleman, I. (2008). The effects of exercise on spatial learning and anxiety-like behavior are mediated by an IGF-I-dependent mechanism related to hippocampal neurogenesis. Mol. Cell. Neurosci. 37, 402–411. doi: 10.1016/j.mcn.2007.10.016
Tzeng, W. Y., Chuang, J. Y., Lin, L. C., Cherng, C. G., Lin, K. Y., Chen, L. H., et al. (2013). Companions reverse stressor-induced decreases in neurogenesis and cocaine conditioning possibly by restoring BDNF and NGF levels in dentate gyrus. Psychoneuroendocrinology 38, 425–437. doi: 10.1016/j.psyneuen.2012.07.002
Uhrin, P., Zaujec, J., Breuss, J. M., Olcaydu, D., Chrenek, P., Stockinger, H., et al. (2010). Novel function for blood platelets and podoplanin in developmental separation of blood and lymphatic circulation. Blood 115, 3997–4005. doi: 10.1182/blood-2009-04-216069
Uzakov, S. S., Ivanov, A. D., Salozhin, S. V., Markevich, V. A., and Gulyaeva, N. V. (2015). Lentiviral-mediated overexpression of nerve growth factor (NGF) prevents β-amyloid [25–35]-induced long term potentiation (LTP) decline in the rat hippocampus. Brain Res. 1624, 398–404. doi: 10.1016/j.brainres.2015.07.051
Vanderbilt, J. N., Allen, L., Gonzalez, R. F., Tigue, Z., Edmondson, J., Ansaldi, D., et al. (2008). Directed expression of transgenes to alveolar type I cells in the mouse. Am. J. Respir. Cell Mol. Biol. 39, 253–262. doi: 10.1165/rcmb.2008-0049OC
Verdaguer, E., Brox, S., Petrov, D., Olloquequi, J., Romero, R., De Lemos, M. L., et al. (2015). Vulnerability of calbindin, calretinin and parvalbumin in a transgenic/knock-in APPswe/PS1dE9 mouse model of Alzheimer disease together with disruption of hippocampal neurogenesis. Exp. Gerontol. 69, 176–188. doi: 10.1016/j.exger.2015.06.013
Violle, N., Balandras, F., Le Roux, Y., Desor, D., and Schroeder, H. (2009). Variations in illumination, closed wall transparency and/or extramaze space influence both baseline anxiety and response to diazepam in the rat elevated plus-maze. Behav. Brain Res. 203, 35–42. doi: 10.1016/j.bbr.2009.04.015
Walf, A. A., and Frye, C. A. (2007). The use of the elevated plus maze as an assay of anxiety-related behavior in rodents. Nat. Protoc. 2, 322–328. doi: 10.1038/nprot.2007.44
Walton, R. M. (2012). Postnatal neurogenesis: of mice, men, and macaques. Vet. Pathol. 49, 155–165. doi: 10.1177/0300985811414035
Wang, S. H., Liao, X. M., Liu, D., Hu, J., Yin, Y. Y., Wang, J. Z., et al. (2012). NGF promotes long-term memory formation by activating poly(ADP-ribose)polymerase-1. Neuropharmacology 63, 1085–1092. doi: 10.1016/j.neuropharm.2012.06.050
Wang, H. G., Lu, F. M., Jin, I., Udo, H., Kandel, E. R., De Vente, J., et al. (2005). Presynaptic and postsynaptic roles of NO, cGK, and RhoA in long-lasting potentiation and aggregation of synaptic proteins. Neuron 45, 389–403. doi: 10.1016/j.neuron.2005.01.011
Wang, S., Scott, B. W., and Wojtowicz, J. M. (2000). Heterogenous properties of dentate granule neurons in the adult rat. J. Neurobiol. 42, 248–257. doi: 10.1002/(sici)1097-4695(20000205)42:2<248::aid-neu8>3.3.co;2-a
Warner-Schmidt, J. L., and Duman, R. S. (2006). Hippocampal neurogenesis: opposing effects of stress and antidepressant treatment. Hippocampus 16, 239–249. doi: 10.1002/hipo.20156
Williams, M. C. (2003). Alveolar type I cells: molecular phenotype and development. Annu. Rev. Physiol. 65, 669–695. doi: 10.1146/annurev.physiol.65.092101.142446
Williams, M. C., Cao, Y., Hinds, A., Rishi, A. K., and Wetterwald, A. (1996). T1 α protein is developmentally regulated and expressed by alveolar type I cells, choroid plexus and ciliary epithelia of adult rats. Am. J. Respir. Cell Mol. Biol. 14, 577–585. doi: 10.1165/ajrcmb.14.6.8652186
Yan, A., Avraham, T., Zampell, J. C., Haviv, Y. S., Weitman, E., and Mehrara, B. J. (2011). Adipose-derived stem cells promote lymphangiogenesis in response to VEGF-C stimulation or TGF-β1 inhibition. Future Oncol. 7, 1457–1473. doi: 10.2217/fon.11.121
Yu, Y., He, J., Zhang, Y., Luo, H., Zhu, S., Yang, Y., et al. (2009). Increased hippocampal neurogenesis in the progressive stage of Alzheimer’s disease phenotype in an APP/PS1 double transgenic mouse model. Hippocampus 19, 1247–1253. doi: 10.1002/hipo.20587
Zakharenko, S. S., Patterson, S. L., Dragatsis, I., Zeitlin, S. O., Siegelbaum, S. A., Kandel, E. R., et al. (2003). Presynaptic BDNF required for a presynaptic but not postsynaptic component of LTP at hippocampal CA1-CA3 synapses. Neuron 39, 975–990. doi: 10.1016/s0896-6273(03)00543-9
Zeng, H., Chattarji, S., Barbarosie, M., Rondi-Reig, L., Philpot, B. D., Miyakawa, T., et al. (2001). Forebrain-specific calcineurin knockout selectively impairs bidirectional synaptic plasticity and working/episodic-like memory. Cell 107, 617–629. doi: 10.1016/s0092-8674(01)00585-2
Zhang, H., Petit, G. H., Gaughwin, P. M., Hansen, C., Ranganathan, S., Zuo, X., et al. (2013). NGF rescues hippocampal cholinergic neuronal markers, restores neurogenesis and improves the spatial working memory in a mouse model of Huntington’s disease. J. Huntingtons Dis. 2, 69–82. doi: 10.3233/jhd-120026
Keywords: neurogenesis, podoplanin, LTD, anxiety-like behavior, NGF, cell proliferation, the hippocampus
Citation: Cicvaric A, Sachernegg HM, Stojanovic T, Symmank D, Smani T, Moeslinger T, Uhrin P and Monje FJ (2020) Podoplanin Gene Disruption in Mice Promotes in vivo Neural Progenitor Cells Proliferation, Selectively Impairs Dentate Gyrus Synaptic Depression and Induces Anxiety-Like Behaviors. Front. Cell. Neurosci. 13:561. doi: 10.3389/fncel.2019.00561
Received: 09 September 2019; Accepted: 05 December 2019;
Published: 15 January 2020.
Edited by:
Oliver von Bohlen und Halbach, Universitätsmedizin Greifswald, GermanyReviewed by:
Joana Gil-Mohapel, University of Victoria, CanadaGerardo Bernabé Ramirez-Rodriguez, National Institute of Psychiatry Ramon de la Fuente Muñiz (INPRFM), Mexico
Copyright © 2020 Cicvaric, Sachernegg, Stojanovic, Symmank, Smani, Moeslinger, Uhrin and Monje. This is an open-access article distributed under the terms of the Creative Commons Attribution License (CC BY). The use, distribution or reproduction in other forums is permitted, provided the original author(s) and the copyright owner(s) are credited and that the original publication in this journal is cited, in accordance with accepted academic practice. No use, distribution or reproduction is permitted which does not comply with these terms.
*Correspondence: Ana Cicvaric, YW5hLmNpY3ZhcmljQG1lZHVuaXdpZW4uYWMuYXQ=; Francisco J. Monje, ZnJhbmNpc2NvLm1vbmplQG1lZHVuaXdpZW4uYWMuYXQ=