- 1International Institute for Integrative Sleep Medicine (WPI-IIIS), University of Tsukuba, Tsukuba, Japan
- 2Ph.D. Program in Human Biology, School of Integrative and Global Majors, University of Tsukuba, Tsukuba, Japan
- 3The University of Tokyo Hospital, The University of Tokyo, Tokyo, Japan
Injection of nanomolar amounts of prostaglandin D2 (PGD2) into the rat brain has dose and time-dependent somnogenic effects, and the PGD2-induced sleep is indistinguishable from physiologic sleep. Sleep-inducing PGD2 is produced in the brain by lipocalin-type PGD2 synthase (LPGDS). Three potential intracranial sources of LPGDS have been identified: oligodendrocytes, choroid plexus, and leptomeninges. We aimed at the identification of the site of synthesis of somnogenic PGD2 and therefore, generated a transgenic mouse line with the LPGDS gene amenable to conditional deletion using Cre recombinase (flox-LPGDS mouse). To identify the cell type responsible for producing somnogenic PGD2, we engineered animals lacking LPGDS expression specifically in oligodendrocytes (OD-LPGDS KO), choroid plexus (CP-LPGDS KO), or leptomeninges (LM-LPGDS KO). We measured prostaglandins and LPGDS concentrations together with PGD synthase activity in the brain of these mice. While the LPGDS amount and PGD synthase activity were drastically reduced in the OD- and LM-LPGDS KO mice, they were unchanged in the CP-LPGDS KO mice compared with control animals. We then recorded electroencephalograms, electromyograms, and locomotor activity to measure sleep in 10-week-old mice with specific knockdown of LPGDS in each of the three targets. Using selenium tetrachloride, a specific PGDS inhibitor, we demonstrated that sleep is inhibited in OD-LPGDS and CP-LPGDS KO mice, but not in the LM-LPGDS KO mice. We concluded that somnogenic PGD2 is produced primarily by the leptomeninges, and not by oligodendrocytes or choroid plexus.
Introduction
In 1982, it was discovered that microinjecting prostaglandin (PG) D2 into the preoptic area of conscious rats induces sleep (Ueno et al., 1982). It is now widely accepted that PGD2 (Urade and Hayaishi, 2010; Huang et al., 2011) is one of the endogenous chemicals proposed by Ishimori (Ishimori, 1909; Kubota, 1989) and Pieron (Legendre and Pieron, 1913) more than 100 years ago to induce sleep. Other substances that induce sleep are cytokines (Krueger et al., 2011), adenosine (Porkka-Heiskanen et al., 1997; McCarley, 2007; Huang et al., 2011; Porkka-Heiskanen and Kalinchuk, 2011), anandamide (Garcia-Garcia et al., 2009), and peptides such as urotensin II (Huitron-Resendiz et al., 2005). These studies independently postulated that prolonged periods of wakefulness can lead to an accumulation of hypothetical endogenous substances that induce sleep. Indeed, elevated PGD2 levels are found in diseases with sleep alterations such as mastocytosis and African trypanosomiasis (Roberts et al., 1980; Pentreath et al., 1990).
Prostaglandin D2 is a derivative of arachidonic acid produced by two different PGDS, hematopoietic PGDS, and LPGDS. Hematopoietic PGDS is a member of the sigma class glutathione-S-transferase family (Urade et al., 1987) and synthesizes PGD2, for example, in mast cells during allergic reactions (Tanaka et al., 2000), whereas glutathione-independent LPGDS is primarily expressed in the brain (Urade and Hayaishi, 2000). Natural sleep is inhibited in wild-type and hematopoietic PGDS knockout (KO) mice, but not LPGDS KO mice, after administering the inorganic tetravalent selenium compound selenium tetrachloride (SeCl4), a specific inhibitor of PGDS activity (Islam et al., 1991; Matsumura et al., 1991; Takahata et al., 1993), demonstrating that PGD2 is involved in regulating physiologic sleep (Qu et al., 2006). A recent study from our lab showed that postictal (pathological) sleep is also regulated via LPGDS-derived PGD2 (Kaushik et al., 2014).
Experiments based on in situ hybridization and immunohistochemistry in rat brains demonstrated that LPGDS is expressed in three intracranial cell populations: cells of the CP, cells of the LM, and OD (Urade et al., 1993). Which of these cell population produces the PGD2 involved in sleep-wake regulation is, however, unclear.
In the present study, we generated a mouse line with a loxP-site-inserted LPGDS gene that is amenable to conditional disruption by cell type-specific expression of Cre recombinase to obtain CP-LPGDS KO mice, LM-LPGDS KO mice, and OD-LPGDS KO mice. When the mice were administered the PGDS inhibitor SeCl4, only the CP- and OD-LPGDS KO mice exhibited disrupted sleep, and not the LM-LPGDS KO mice. Our findings reveal that the LM, but not the CP and OD, produce the PGD2 that induces physiologic sleep.
Materials and Methods
Genetic Mouse Models
Animals were handled according to the NIH Guide for the Care and Use of Laboratory Animals and in accordance with protocols approved by animal research committees at the Osaka Bioscience Institute and the International Institute for Integrative Sleep Medicine (animal protocol #16-086). All male mice (weighing 24–28 g, 10–14 weeks old) used in the present study were housed at a constant temperature (22 ± 1°C) with a relative humidity of 50 ± 2% on an automatically controlled 12:12 light/dark cycle (light on at 8:00 am). A mouse line called flox-LPGDS on a C57BL/6 background with a loxP-site-inserted LPGDS gene that is conditionally disrupted by expressing Cre recombinase was generated as previously described (Kaneko et al., 2012) and used in this study. This mouse line has not been deposited to any animal repository.
Generation of Cell Type-Specific LPGDS Knockout Animals
Several serotypes of AAV have been identified and are commonly used in neuroscience. These serotypes differ in their tropism (the types of cells they infect), making AAV a very useful system for preferentially targeting the gene of interest in specific cell types. We tested the ability for AAV to specifically infect the LM and the CP. We tested 5 different serotypes of AAVs (serotypes 2, 5, 8, 10, and 11) expressing the reporter protein mCHERRY in wild-type mice and discovered that the AAV of serotype 5 was the most efficient to target the CP, while only the serotype 8, when injected in postnatal mice (2-day-old), could infect the LM. Therefore LM-LPGDS KO mice were obtained by micro-injecting 6 μL of serotype 8 AAV-Cre into the lateral ventricle of 2-day-old flox-LPGDS mice. Briefly, neonatal mice were anesthetized by hypothermia on ice for 5 min before fixing to the pad of a stereotaxic arm. A glass micropipette with a 10- to 20-μm-diameter tip was introduced manually into the external corner of the right eye of the animal. Light pressure was applied to pass through the eye socket bone and deliver the AAV vectors into the lateral ventricle (Kalamarides et al., 2002). Using an air pressure injection system, 6 μL of viral vector from serotype 8 was delivered into the CSF over 3 min. After the injection, the pipette was kept in place for a few seconds until the CSF pressure returned to a normal level and then removed. Following the injection, the neonatal mice were kept in a cage warmed to 37°C until they recovered and then returned to their mother.
CP-LPGDS KO mice were obtained by micro-injecting serotype 5 AAV-Cre into the lateral ventricle of adult male flox-LPGDS mice. Briefly, mice weighing 24–28 g were anesthetized with pentobarbital (50 mg/kg, i.p.), and 50 μl AAV5-Cre was stereotaxically microinjected into the left lateral ventricle (0.45 mm caudal to bregma, 1.6 mm lateral from bregma, and 1.6 mm below the dural surface) at a flow rate of 0.6 μL/min using a 100-μL Hamilton syringe and a syringe pump. Our observations indicate that the virus can disperse across ventricles and infect the remaining CP.
OD-LPGDS KO mice were obtained by cross-breeding flox-LPGDS females with transgenic mice expressing Cre recombinase under control of the nestin promoter. The nestin promoter drives Cre expression only in neural precursor cells, leading to total KO of LPGDS in the derived cells, including the OD, but not in the LM or CP.
As controls, in the case of LM- and CP-LPGDS KO mice we used littermate Flox-LPGDS mice injected with AAVs of the same serotypes and administered following the same protocol, however, these AAVs express the fluorescent protein mCHERRY instead of Cre recombinase (hence called littermate control mice). In the case of OD-LPGDS KO mice, littermate control mice are born from the same parents but did not express Cre under the control of Nestin promoter (n = 6 for each group).
Vigilance State Assessment Using Electroencephalogram (EEG), Electromyogram (EMG) and Locomotor Activity Recordings
Vigilance states were assessed in adult male conditional LPGDS KO mice as described earlier (Huang et al., 2001). All mice subjected to EEG recordings were injected with vehicle or drug on two consecutive days. On day 1, the mice were injected with vehicle (saline, i.p.) at 10 am, and the 24-h recordings performed on day 1 were used as baseline data. On day 2, the mice were injected with SeCl4 (i.p., 5 mg/kg, 10 mL/kg body weight) and EEG/EMG signals were recorded for 24 h. The EEG/EMG signals were amplified and filtered (EEG: 0.5–30 Hz, EMG: 20–200 Hz), then digitized at a sampling rate of 128 Hz, and recorded using SLEEPSIGN software (Kohtoh et al., 2008). In addition, locomotor activity was recorded with an infrared photocell sensor (Biotex, Kyoto, Japan). The vigilance state of each 10-s epoch was automatically scored offline into three stages: waking, REM sleep, and non-REM sleep, according to standard criteria (Mizoguchi et al., 2001). As a final step, defined vigilance stages were examined visually, and corrected manually when necessary. A total of 6 to 8 animals per group were used in this experiment.
Generation of AAV Vectors
AAV-CMV-Cre and AAV-CMV-mCherry (mCHE) vectors from serotypes 5 and 8 were obtained as described earlier (Lazarus et al., 2011). Briefly, the AAV-mCHE vector plasmid was generated by replacing the humanized Renilla reniformis green fluorescent protein (hrGFP) sequence from the AAV-hrGFP vector plasmid with the mCHE sequence. Subsequently, the gene coding for mCHE was replaced with the Cre recombinase coding sequence derived by polymerase chain reaction from the pBS185 plasmid (Sauer and Henderson, 1990). The serotype 5 AAV was generated by tripartite transfection (AAV-pXR5 capsid plasmid, adenovirus helper plasmid, and AAV-vector plasmid), as well as the serotype 8 AAV (AAV-rep2/cap8 capsid plasmid, adenovirus helper plasmid, and AAV-vector plasmid) into HEK293-derived AAV-293 cells (Stratagene, catalog #240073). Three days after transfection, the virus was extracted and then quantified by quantitative polymerase chain reaction.
Immunohistochemistry
Following all procedures, the animals were deeply anesthetized with chloral hydrate (500 mg/kg, i.p.) and perfused through the left ventricle of the heart with saline followed by neutral buffered 10% formalin. The brains were removed and placed in 20% sucrose in phosphate-buffered saline (PBS) overnight at 4°C for cryoprotection. The brains were then frozen on dry ice and sectioned at 30 μm on a freezing microtome. Immunohistochemistry was performed on free-floating sections with a primary antibody directed at LPGDS as described previously (Beuckmann et al., 2000). Briefly, sections were rinsed in PBS, incubated in 3% hydrogen peroxide in PBS for 30 min at room temperature, and then sequentially at room temperature in 3% normal donkey serum and 0.25% Triton X-100 in PBS (PBT) for 1 h and primary antibody diluted in PBT with 0.02% sodium azide overnight. After several washes in PBS, the sections were incubated in goat anti-rabbit horseradish peroxidase-conjugated secondary antibody for 1 h, and the presence of LPGDS was revealed by a 0.05% 3,3′-diaminobenzidine/0.015% hydrogen peroxidase reaction for 10 min. Photomicrographs were obtained using a Nikon Eclipse E600 microscope coupled to a Digital Sight DS-2Mv camera. LM, CP, and OD were characterized by their localization in the tissue sections, as well as their morphology and their potential expression for LPGDS.
Enzymatic PGDS Assay
The effect of the conditional KO of LPGDS (n = 6 for each group) in LM, CP, and OD on the brain PGDS enzymatic activity was measured by incubating 40 μg of brain extracts at 25°C for 1 min with [1-14C]PG H2 (final concentration of 40 μM) in 50 μL of 0.1 M Tris–HCl (pH 8.0) containing 1 mM dithiothreitol (Urade et al., 1995). [1-14C]PG H2 was prepared from [1-14C]arachidonic acid (2.20 GBq/mmol; PerkinElmer, Wellesley, MA, United States) as described previously (Urade et al., 1985).
Prostaglandin D2, Enzyme Immunoassays
The brains of LM-LPGDS KO, CP-LPGDS KO, and OD-LPGDS KO mice (n = 6 for each group) were harvested and immediately frozen in liquid nitrogen. They were then homogenized in ethanol containing 0.02% HCl at pH 2.0 and centrifuged at 500 g for 20 min. 3H-Labeled PGD2 (60 Bq/assay; PerkinElmer) was added to the supernatant as tracer to estimate recovery. The recovery value was approximately 60%. PGD2 was extracted with ethyl acetate, which was evaporated under nitrogen. The samples were then separated by HPLC (Gilson, Middleton, WI, United States) (Pinzar et al., 2000). PGD2 was quantified using a PGD2 enzyme immunoassay kit for this prostanoid (Cayman Chemicals, Ann Arbor, MI, United States).
Statistical Analysis
Data are presented as the mean ± standard error of the mean (SEM). For the sleep data analysis, an unpaired Student’s t-test was used to analyze the amount of time spent in the different sleep-wake states. A one- or two-way ANOVA followed by the Fisher protected least significant difference test was used to determine whether differences in LPGDS, PGD2 contents, or PGDS activity in the brain of control or KO mice were statistically significant. In all cases, p < 0.05 was considered statistically significant.
Results
Generation of Cell Type-Specific LPGDS KO Mice
To determine which intracranial cell population produces the PGD2 that regulates sleep, we generated cell type-specific LPGDS KO mice for the CP, OD, and LM based on a mouse line in which the LPGDS gene is flanked by loxP sites in intron 1 and 6 and is amenable to conditional deletion by Cre recombinase (Supplementary Figure S1) (Kaneko et al., 2012). To obtain the LM-LPGDS KO mice, we injected 6 μL of AAV serotype 8 expressing Cre recombinase (AAV8-Cre) into the CSF of 2-day-old mice. Two months later, immunohistochemistry confirmed the absence of LPGDS expression in the LM, but not in the CP or OD (Figures 1E–H). To generate CP-LPGDS KO mice, we infused 50 μL of AAV-Cre, serotype 5 (AAV5-Cre), into the lateral ventricle of adult animals and 3 weeks later immunostaining confirmed the selective absence of LPGDS in the CP (Figures 1I–L). Moreover, we crossed our floxed LPGDS mice with mice expressing Cre under control of the rat Nestin promoter and enhancer (Tronche et al., 1999). The Nestin promoter drives Cre recombinase expression only in cells from the brain parenchyma of neuroectodermal origin (neurons, astrocyte, and OD), and among them only the OD express LPGDS (Urade and Hayaishi, 2011). This resulted to the deletion of LPGDS in the OD (OD-LPGDS KO mice; Figures 1M–P). In contrast, LPGDS immunostaining in the control flox-LPGDS animals revealed intense staining for LPGDS in the LM, CP, and OD (Figures 1A–D).
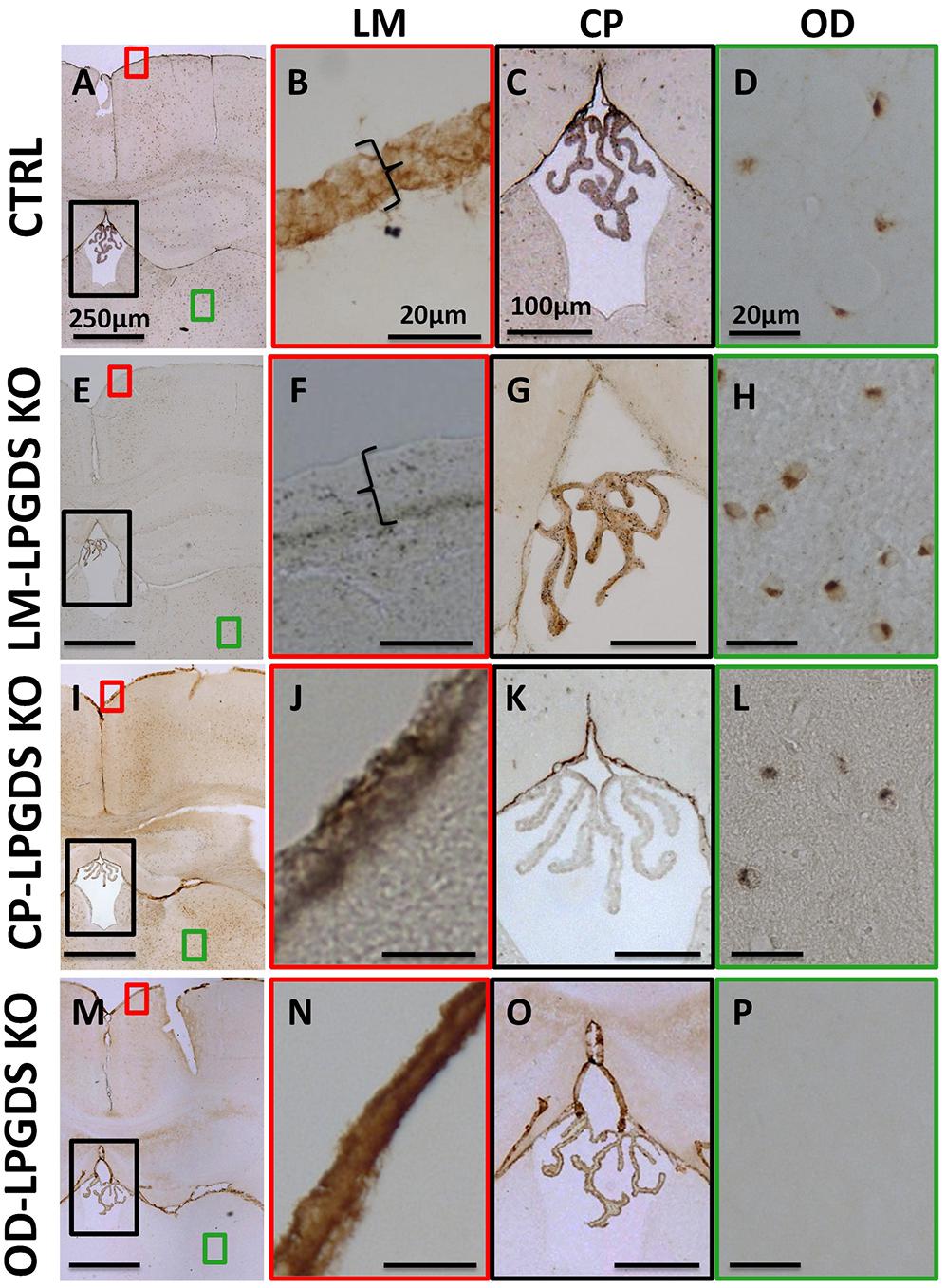
FIGURE 1. Localization of LPGDS expression in the LPGDSflox control (CTRL) mice (A), LM-LPGDS KO mice (E), CP-LPGDS KO mice (I), and OD-LPGDS KO mice (M) by immunohistochemistry. Serial coronal sections were stained with an LPGDS antibody and representative images are presented. LPGDS was not detected in the LM of LM-LPGDS KO mice (F) while it was strongly expressed in the LM of LPGDSflox control mice (B) and other cell type-specific KO mice (J,N). LPGDS staining was absent from CP cells in CP-LPGDS KO mice (K), but was expressed in the other mice (C,G,O). No LPGDS staining was detected in the brain parenchyma of the OD-LPGDS KO mice (P) where many OD, morphologically identified, were positive for LPGDS staining in the other mice (D,H,L).
LPGDS and PGD2 Content in the Brain of LM-, CP-, and OD-LPGDS KO Mice
First, we measured LPGDS concentration in the brain of the LM-, CP-, and OD-LPGDS KO mice. We then determined the amount of PGD2 in flox-LPGDS mice compared with that in control mice. Whereas the total amount of LPGDS in the brain of the CP-LPGDS KO mice remained unchanged compared with the control mice (Figure 2A), the amount of LPGDS was reduced by 58.3 ± 11.1% (p < 0.001) and 75.7 ± 2.7% (p < 0.001), respectively, in the OD- and LM-LPGDS KO mice compared with the control mice. These results indicate that the contribution of the CP to the production of LPGDS in the mouse brain was negligible. In contrast, one-third to one-half of the brain LPGDS was produced by the OD.
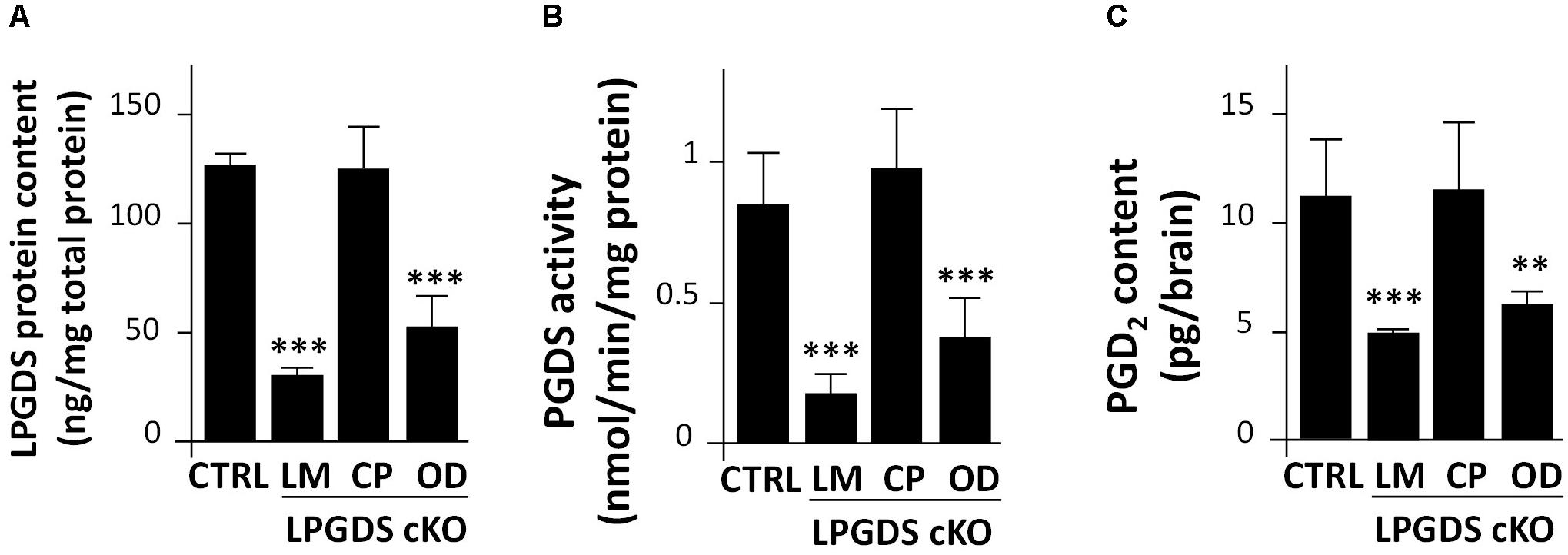
FIGURE 2. Functional analysis of LPGDS disruption in the three conditional LPGDS KO mice. (A) Relative LPGDS protein content in the brain of LPGDSflox (CTRL), LM-LPGDS KO, CP-LPGDS KO, and OD-LPGDS KO mice. (B) PGDS activity in the brain of CTRL and cell type-specific KO mice. (C) PGD2 content in the brain of CTRL and cell type-specific KO mice. Values are means ± SEM (n = 6). ∗∗P < 0.01; ∗∗∗P < 0.001 compared with LPGDSflox (CTRL), one-way ANOVA followed by the Fisher protected least significant difference test.
We next measured PGDS enzymatic activity of the LM-, CP-, OD-LPGDS KO mice and their respective control littermates. PGDS activity in the brain of the CP-LPGDS KO mice remained unchanged compared with the control animals (p = 0.6082), whereas it was significantly reduced by 39.7 ± 6.5% (p = 0.0028) and 78.9 ± 6.9% (p = 0.0004), respectively, in the OD- and LM-LPGDS KO mice (Figure 2B). These findings are in good agreement with the distribution of LPGDS in the brain of the LM-, CP-, and OD-LPGDS KO mice.
Finally, we measured the total amount of PGD2 contained in the brain of the cell type-specific KO animals. In the OD- and LM-LPGDS KO mice, PGD2 concentrations were significantly reduced by 44.2 ± 4.9% (p = 0.004) and 55.5 ± 1.8% (p = 0.001), respectively, compared with the control flox-LPGDS animals (Figure 2C). On the other hand, no change in PGD2 concentration was observed in the brain of CP-LPGDS KO mice compared with the control mice (p = 0.9078).
SeCl4-Induced Insomnia Was Abolished in LM-, but Not in OD- or CP-LPGDS KO Mice
We analyzed the sleep-wake pattern of the LM-, CP-, and OD-LPGDS KO mice by measuring their EEG and EMG activity before and after an i.p. injection of the PGDS inhibitor SeCl4 at a dose of 5 mg/kg at 10:00 am when mice are mostly asleep, as previously described. SeCl4 is a specific inhibitor of PGDS activity that can inhibit natural sleep in wild-type and hematopoietic PGDS KO mice, but not LPGDS KO mice (Qu et al., 2006). The basal sleep behavior of the cell type-specific LM-, CP-, and OD-LPGDS KO mice was identical to that of their control littermates, except for the LM-LPGDS KO mice, which exhibited moderately reduced REM sleep activity during the daytime (-20.4 ± 5.8%, p = 0.0487; Supplementary Figure S2). Furthermore, we analyzed EEG/EMG recordings obtained during a 4-h period after i.p. injections of SeCl4 (5 mg/kg) and vehicle. The total amount of time spent in sleep was reduced in the CP- and OD-LPGDS KO mice as well as in the control mice during the 3-h period following the injection of SeCl4 compared with vehicle, but in the LM-LPGDS KO mice, the time spent in sleep after the injection of SeCl4 was indistinguishable from that following the vehicle injection (Figure 3A and Supplementary Figure S3).
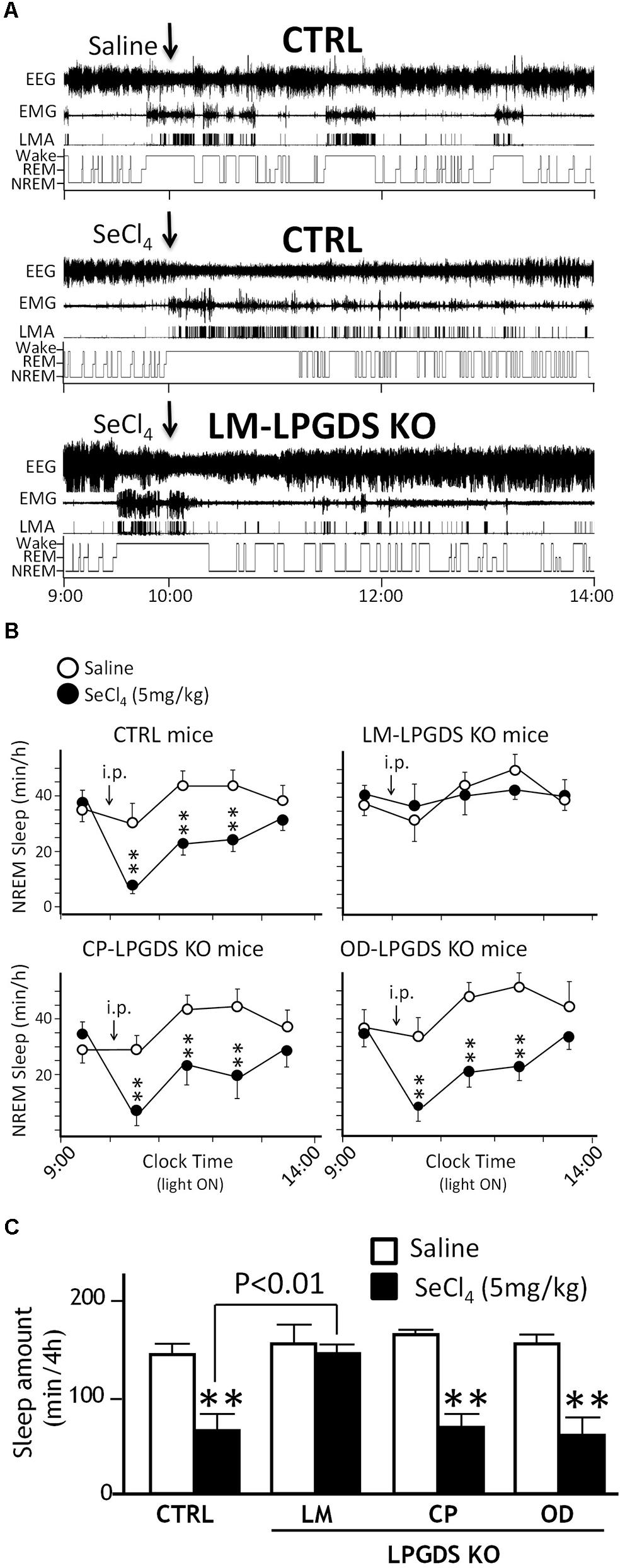
FIGURE 3. SeCl4 inhibited sleep in LPGDSflox control mice (CTRL), CP-LPGDS KO mice, and OD-LPGDS KO mice, but not in LM-LPGDS KO mice. (A) Typical examples of EEG, EMG, and locomotor activity (LMA), and hypnograms after administration of saline (vehicle) or SeCl4 in LPGDSflox control and LM-LPGDS KO mice. (B) Injection of SeCl4 significantly (decreased the hourly amount of sleep during the subsequent 3 h compared with saline in LPGDSflox control mice, CP-LPGDS KO mice, and OD-LPGDS KO mice, but not in LM-LPGDS KO mice. (C) Injection of SeCl4 dramatically decreased the total amount of sleep during the subsequent 4 h compared with saline in LPGDSflox control mice, CP-LPGDS KO mice, and OD-LPGDS KO mice, but not in LM-LPGDS KO mice. Values are means ± SEM (n = 6–8). ∗∗P < 0.01 compared with its own control (saline).)
The changes in the time-course of sleep in the cell type-specific CP-, OD-, and LM-LPGDS KO mice during the 4 h following the i.p. injection of 5 mg/kg SeCl4 is shown in Figure 3B. The total amount of sleep was drastically reduced in the CP- and OD-LPGDS KO mice during the 4 h following the SeCl4 injection (-50.1 ± 11.6%, p = 0.004 and -52.7 ± 8.9%, p = 0.0022, respectively) compared with the vehicle control (Figure 3C). The sleep amount was similarly reduced by the injection of SeCl4 (-59.3 ± 3.1%, p < 0.0001) in the control animals (flox-LPGDS). By contrast, no sleep reduction was observed in the LM-LPGDS KO mice, indicating their complete insensitivity to the SeCl4 injection (-2.5 ± 9.4%, p = 0.7341). These results suggest that LPGDS expressed in the LM, but not the CP or OD, is involved in the regulation of physiologic sleep.
Discussion
The administration of an LPGDS inhibitor (SeCl4) or PGD2 receptor, subtype DP1, antagonist (ONO-4127Na) inhibits sleep in rats and mice, indicating that the PGD2 system is crucial for maintaining physiologic sleep (Qu et al., 2006). The specific intracranial cell population expressing LPGDS and producing the PGD2 responsible for the induction of sleep, however, has been debated over the last 30 years (Narumiya et al., 1982; Hayaishi, 1991; Urade et al., 1993; Vesin et al., 1995; Yamashima et al., 1997; Qu et al., 2006; Urade and Hayaishi, 2011; Zhang et al., 2017a,b). Using cell type-specific LPGDS KO mice for the LM, OD, or CP, we here identified the LM as the primary source of LPGDS involved in the regulation of sleep. Therefore, LPGDS in the LM produces PGD2, which is secreted into the CSF, where it stimulates DP1 receptors as a sleep hormone. In the same pathway, adenosine is then released as a secondary sleep-promoting messenger and activates adenosine A2A receptor-expressing neurons (Lazarus et al., 2012; Zhang et al., 2017a). Through this pathway, the sleep center in the ventrolateral preoptic nucleus is subsequently activated and the histaminergic arousal center in the tuberomammillary nucleus is reciprocally regulated by the primary sleep-promoting neurons in the ventrolateral preoptic nucleus via GABAergic inhibitory projections (Sherin et al., 1998; Chung et al., 2017). Where DP1 and A2A receptors are expressed, however, remains to be clarified.
The basal sleep behavior of the cell type-specific KO mice for the CP and OD was identical to that of their control littermates, while the LM-LPGDS KO mice exhibited slightly reduced REM sleep activity during the daytime. The absence of a major sleep phenotype in the conditional LPGDS KO mice is in good agreement with previous reports of global LPGDS KO mice (Hayaishi et al., 2004). The most widely accepted explanation so far involves a compensatory mechanism during embryonic development that helps the animal to recover a normal sleep pattern, as sleep is essential for the survival of animals. In the LM-PGDS KO mice, disruption of LPGDS occurs in a late-stage of development, which may explain the incomplete compensation and the moderate REM sleep abnormality.
While using AAVs expressing Cre recombinase permitted to delete the expression of LPGDS in the CP and the LM, we cannot exclude the possibility that other cell types might also have been infected and their copy of lpgds gene deleted. Indeed, other cells such as tanycytes -a special ependymal cells found in the third ventricle of the brain and on the floor of the fourth ventricle- or ependymocytes -a type of glial cell forming the ependyma, a thin neuroepithelial lining the ventricular system- were most likely in contact with the AAVs and might have been infected. However, it is unclear if the serotypes of AAVs used in this study can effectively infect such ependymal cells. Furthermore, even if these and other cell types have also been infected, then started to express Cre recombinase, and were deleted of their lpgds gene, we believe that the effect would have remained unnoticed. To our knowledge, these cells have never been reported to express LPGDS and therefore animals should not be impacted by the potential ectopic expression of Cre recombinase.
Conclusion
Lipocalin-type PGDS is implicated in the production of PGD2 that is involved in the regulation of physiologic sleep. Our findings in cell type-specific LPGDS KO mice demonstrate that the somnogenic PGD2 is primarily produced by LPGDS in the LM.
Data Availability Statement
The raw data supporting the conclusions of this manuscript will be made available by the authors, without undue reservation, to any qualified researcher.
Author Contributions
YC and YU designed the research and wrote the paper. YC and KA performed the research. YO contributed the transgenic mice. YC, KA, MKK, and MK analyzed the data.
Funding
This work was supported by grants from JSPS KAKENHI Grant Nos. JP16K18698 (YC), JP18H02534 (YO), and JP16H01881 (YU), a grant from the Japan Foundation for Applied Enzymology (YO), and the World Premier International Research Center Initiative (WPI) from MEXT.
Conflict of Interest Statement
The authors declare that the research was conducted in the absence of any commercial or financial relationships that could be construed as a potential conflict of interest.
Supplementary Material
The Supplementary Material for this article can be found online at: https://www.frontiersin.org/articles/10.3389/fncel.2018.00357/full#supplementary-material
Abbreviations
AAV, adeno-associated virus; CP, choroid plexus; CSF, cerebrospinal fluid; LM, leptomeninges; LPGDS, lipocalin-type PGDS; OD, oligodendrocytes; PGD2, prostaglandin D2; PGDS, PGD synthase; REM, rapid-eye movement.
References
Beuckmann, C. T., Lazarus, M., Gerashchenko, D., Mizoguchi, A., Nomura, S., Mohri, I., et al. (2000). Cellular localization of lipocalin-type prostaglandin D synthase (beta-trace) in the central nervous system of the adult rat. J. Comp. Neurol. 428, 62–78. doi: 10.1002/1096-9861(20001204)428:1<62::AID-CNE6>3.0.CO;2-E
Chung, S., Weber, F., Zhong, P., Tan, C. L., Nguyen, T. N., Beier, K. T., et al. (2017). Identification of preoptic sleep neurons using retrograde labelling and gene profiling. Nature 545, 477–481. doi: 10.1038/nature22350
Garcia-Garcia, F., Acosta-Pena, E., Venebra-Munoz, A., and Murillo-Rodriguez, E. (2009). Sleep-inducing factors. CNS Neurol. Disord. Drug Targets 8, 235–244. doi: 10.2174/187152709788921672
Hayaishi, O. (1991). Molecular mechanisms of sleep-wake regulation: roles of prostaglandins D2 and E2. FASEB J. 5, 2575–2581. doi: 10.1096/fasebj.5.11.1907936
Hayaishi, O., Urade, Y., Eguchi, N., and Huang, Z. L. (2004). Genes for prostaglandin d synthase and receptor as well as adenosine A2A receptor are involved in the homeostatic regulation of nrem sleep. Arch. Ital. Biol. 142, 533–539.
Huang, Z. L., Qu, W. M., Li, W. D., Mochizuki, T., Eguchi, N., Watanabe, T., et al. (2001). Arousal effect of orexin A depends on activation of the histaminergic system. Proc. Natl. Acad. Sci. U.S.A. 98, 9965–9970. doi: 10.1073/pnas.181330998
Huang, Z. L., Urade, Y., and Hayaishi, O. (2011). The role of adenosine in the regulation of sleep. Curr. Top. Med. Chem. 11, 1047–1057. doi: 10.2174/156802611795347654
Huitron-Resendiz, S., Kristensen, M. P., Sanchez-Alavez, M., Clark, S. D., Grupke, S. L., Tyler, C., et al. (2005). Urotensin II modulates rapid eye movement sleep through activation of brainstem cholinergic neurons. J. Neurosci. 25, 5465–5474. doi: 10.1523/JNEUROSCI.4501-04.2005
Ishimori, K. (1909). True cause of sleep: a hypnogenic substance as evidence in the brain off sleep-deprived animals. Tokyo Igakkai Zasshi 23, 429–457.
Islam, F., Watanabe, Y., Morii, H., and Hayaishi, O. (1991). Inhibition of rat brain prostaglandin D synthase by inorganic selenocompounds. Arch. Biochem. Biophys. 289, 161–166. doi: 10.1016/0003-9861(91)90456-S
Kalamarides, M., Niwa-Kawakita, M., Leblois, H., Abramowski, V., Perricaudet, M., Janin, A., et al. (2002). Nf2 gene inactivation in arachnoidal cells is rate-limiting for meningioma development in the mouse. Genes Dev. 16, 1060–1065. doi: 10.1101/gad.226302
Kaneko, K., Lazarus, M., Miyamoto, C., Oishi, Y., Nagata, N., Yang, S., et al. (2012). Orally administered rubiscolin-6, a delta opioid peptide derived from Rubisco, stimulates food intake via leptomeningeal lipocallin-type prostaglandin D synthase in mice. Mol. Nutr. Food Res. 56, 1315–1323. doi: 10.1002/mnfr.201200155
Kaushik, M. K., Aritake, K., Kamauchi, S., Hayaishi, O., Huang, Z. L., Lazarus, M., et al. (2014). Prostaglandin D(2) is crucial for seizure suppression and postictal sleep. Exp. Neurol. 253, 82–90. doi: 10.1016/j.expneurol.2013.12.002
Kohtoh, S., Taguchi, Y., Matsumoto, N., Wada, M., Huang, Z.-L., and Urade, Y. (2008). Algorithm for sleep scoring in experimental animals based on fast Fourier transform power spectrum analysis of the electroencephalogram. Sleep Biol. Rhythms 6, 163–171. doi: 10.1111/j.1479-8425.2008.00355.x
Krueger, J. M., Clinton, J. M., Winters, B. D., Zielinski, M. R., Taishi, P., Jewett, K. A., et al. (2011). Involvement of cytokines in slow wave sleep. Prog. Brain Res. 193, 39–47. doi: 10.1016/B978-0-444-53839-0.00003-X
Kubota, K. (1989). Kuniomi Ishimori and the first discovery of sleep-inducing substances in the brain. Neurosci. Res. 6, 497–518. doi: 10.1016/0168-0102(89)90041-2
Lazarus, M., Huang, Z. L., Lu, J., Urade, Y., and Chen, J. F. (2012). How do the basal ganglia regulate sleep-wake behavior? Trends Neurosci. 35, 723–732. doi: 10.1016/j.tins.2012.07.001
Lazarus, M., Shen, H. Y., Cherasse, Y., Qu, W. M., Huang, Z. L., Bass, C. E., et al. (2011). Arousal effect of caffeine depends on adenosine A2A receptors in the shell of the nucleus accumbens. J. Neurosci. 31, 10067–10075. doi: 10.1523/JNEUROSCI.6730-10.2011
Legendre, R., and Pieron, H. (1913). Recherches sur le besoin de sommeil consécutif à une veille prolongée. Z. Allegem. Physiol. 14, 235–262.
Matsumura, H., Takahata, R., and Hayaishi, O. (1991). Inhibition of sleep in rats by inorganic selenium compounds, inhibitors of prostaglandin D synthase. Proc. Natl. Acad. Sci. U.S.A. 88, 9046–9050. doi: 10.1073/pnas.88.20.9046
McCarley, R. W. (2007). Neurobiology of REM and NREM sleep. Sleep Med. 8, 302–330. doi: 10.1016/j.sleep.2007.03.005
Mizoguchi, A., Eguchi, N., Kimura, K., Kiyohara, Y., Qu, W. M., Huang, Z. L., et al. (2001). Dominant localization of prostaglandin D receptors on arachnoid trabecular cells in mouse basal forebrain and their involvement in the regulation of non-rapid eye movement sleep. Proc. Natl. Acad. Sci. U.S.A. 98, 11674–11679. doi: 10.1073/pnas.201398898
Narumiya, S., Ogorochi, T., Nakao, K., and Hayaishi, O. (1982). Prostaglandin D2 in rat brain, spinal cord and pituitary: basal level and regional distribution. Life Sci. 31, 2093–2103. doi: 10.1016/0024-3205(82)90101-1
Pentreath, V. W., Rees, K., Owolabi, O. A., Philip, K. A., and Doua, F. (1990). The somnogenic T lymphocyte suppressor prostaglandin D2 is selectively elevated in cerebrospinal fluid of advanced sleeping sickness patients. Trans. R. Soc. Trop. Med. Hyg. 84, 795–799. doi: 10.1016/0035-9203(90)90085-S
Pinzar, E., Kanaoka, Y., Inui, T., Eguchi, N., Urade, Y., and Hayaishi, O. (2000). Prostaglandin D synthase gene is involved in the regulation of non-rapid eye movement sleep. Proc. Natl. Acad. Sci. U.S.A. 97, 4903–4907. doi: 10.1073/pnas.090093997
Porkka-Heiskanen, T., and Kalinchuk, A. V. (2011). Adenosine, energy metabolism and sleep homeostasis. Sleep Med. Rev. 15, 123–135. doi: 10.1016/j.smrv.2010.06.005
Porkka-Heiskanen, T., Strecker, R. E., Thakkar, M., Bjorkum, A. A., Greene, R. W., and Mccarley, R. W. (1997). Adenosine: a mediator of the sleep-inducing effects of prolonged wakefulness. Science 276, 1265–1268. doi: 10.1126/science.276.5316.1265
Qu, W. M., Huang, Z. L., Xu, X. H., Aritake, K., Eguchi, N., Nambu, F., et al. (2006). Lipocalin-type prostaglandin D synthase produces prostaglandin D2 involved in regulation of physiological sleep. Proc. Natl. Acad. Sci. U.S.A. 103, 17949–17954. doi: 10.1073/pnas.0608581103
Roberts, L. J. II, Sweetman, B. J., Lewis, R. A., Austen, K. F., and Oates, J. A. (1980). Increased production of prostaglandin D2 in patients with systemic mastocytosis. N. Engl. J. Med. 303, 1400–1404. doi: 10.1056/NEJM198012113032405
Sauer, B., and Henderson, N. (1990). Targeted insertion of exogenous DNA into the eukaryotic genome by the Cre recombinase. New Biol. 2, 441–449.
Sherin, J. E., Elmquist, J. K., Torrealba, F., and Saper, C. B. (1998). Innervation of histaminergic tuberomammillary neurons by GABAergic and galaninergic neurons in the ventrolateral preoptic nucleus of the rat. J. Neurosci. 18, 4705–4721. doi: 10.1523/JNEUROSCI.18-12-04705.1998
Takahata, R., Matsumura, H., Kantha, S. S., Kubo, E., Kawase, K., Sakai, T., et al. (1993). Intravenous administration of inorganic selenium compounds, inhibitors of prostaglandin D synthase, inhibits sleep in freely moving rats. Brain Res. 623, 65–71. doi: 10.1016/0006-8993(93)90010-K
Tanaka, K., Ogawa, K., Sugamura, K., Nakamura, M., Takano, S., and Nagata, K. (2000). Cutting edge: differential production of prostaglandin D2 by human helper T cell subsets. J. Immunol. 164, 2277–2280. doi: 10.4049/jimmunol.164.5.2277
Tronche, F., Kellendonk, C., Kretz, O., Gass, P., Anlag, K., Orban, P. C., et al. (1999). Disruption of the glucocorticoid receptor gene in the nervous system results in reduced anxiety. Nat. Genet. 23, 99–103. doi: 10.1038/12703
Ueno, R., Ishikawa, Y., Nakayama, T., and Hayaishi, O. (1982). Prostaglandin D2 induces sleep when microinjected into the preoptic area of conscious rats. Biochem. Biophys. Res. Commun. 109, 576–582. doi: 10.1016/0006-291X(82)91760-0
Urade, Y., Fujimoto, N., and Hayaishi, O. (1985). Purification and characterization of rat brain prostaglandin D synthetase. J. Biol. Chem. 260, 12410–12415.
Urade, Y., Fujimoto, N., Ujihara, M., and Hayaishi, O. (1987). Biochemical and immunological characterization of rat spleen prostaglandin D synthetase. J. Biol. Chem. 262, 3820–3825.
Urade, Y., and Hayaishi, O. (2000). Prostaglandin D synthase: structure and function. Vitam. Horm. 58, 89–120. doi: 10.1016/S0083-6729(00)58022-4
Urade, Y., and Hayaishi, O. (2010). Crucial role of prostaglandin D2 and adenosine in sleep regulation: experimental evidence from pharmacological approaches to gene-knockout mice. Future Neurol. 5, 363–376. doi: 10.2217/fnl.10.18
Urade, Y., and Hayaishi, O. (2011). Prostaglandin D2 and sleep/wake regulation. Sleep Med. Rev. 15, 411–418. doi: 10.1016/j.smrv.2011.08.003
Urade, Y., Kitahama, K., Ohishi, H., Kaneko, T., Mizuno, N., and Hayaishi, O. (1993). Dominant expression of mRNA for prostaglandin D synthase in leptomeninges, choroid plexus, and oligodendrocytes of the adult rat brain. Proc. Natl. Acad. Sci. U.S.A. 90, 9070–9074. doi: 10.1073/pnas.90.19.9070
Urade, Y., Tanaka, T., Eguchi, N., Kikuchi, M., Kimura, H., Toh, H., et al. (1995). Structural and functional significance of cysteine residues of glutathione-independent prostaglandin D synthase. J. Biol. Chem. 270, 1422–1428. doi: 10.1074/jbc.270.3.1422
Vesin, M. F., Urade, Y., Hayaishi, O., and Droz, B. (1995). Neuronal and glial prostaglandin D synthase isozymes in chick dorsal root ganglia: a light and electron microscopic immunocytochemical study. J. Neurosci. 15, 470–476. doi: 10.1523/JNEUROSCI.15-01-00470.1995
Yamashima, T., Sakuda, K., Tohma, Y., Yamashita, J., Oda, H., Irikura, D., et al. (1997). Prostaglandin D synthase (beta-trace) in human arachnoid and meningioma cells: roles as a cell marker or in cerebrospinal fluid absorption, tumorigenesis, and calcification process. J. Neurosci. 17, 2376–2382. doi: 10.1523/JNEUROSCI.17-07-02376.1997
Zhang, B. J., Huang, Z. L., Chen, J. F., Urade, Y., and Qu, W. M. (2017a). Adenosine A2A receptor deficiency attenuates the somnogenic effect of prostaglandin D2 in mice. Acta Pharmacol. Sin. 38, 469–476. doi: 10.1038/aps.2016.140
Keywords: prostaglandin D2, leptomeninges, lipocalin-type prostaglandin D synthase, sleep, adeno-associated virus (AAV)
Citation: Cherasse Y, Aritake K, Oishi Y, Kaushik MK, Korkutata M and Urade Y (2018) The Leptomeninges Produce Prostaglandin D2 Involved in Sleep Regulation in Mice. Front. Cell. Neurosci. 12:357. doi: 10.3389/fncel.2018.00357
Received: 20 July 2018; Accepted: 24 September 2018;
Published: 11 October 2018.
Edited by:
Qinghua Liu, National Institute of Biological Sciences (NIBS), ChinaReviewed by:
Marina Bentivoglio, Università degli Studi di Verona, ItalyWilliam Wisden, Imperial College London, United Kingdom
Copyright © 2018 Cherasse, Aritake, Oishi, Kaushik, Korkutata and Urade. This is an open-access article distributed under the terms of the Creative Commons Attribution License (CC BY). The use, distribution or reproduction in other forums is permitted, provided the original author(s) and the copyright owner(s) are credited and that the original publication in this journal is cited, in accordance with accepted academic practice. No use, distribution or reproduction is permitted which does not comply with these terms.
*Correspondence: Yoan Cherasse, Q2hlcmFzc2UueW9hbi5mbUB1LnRzdWt1YmEuYWMuanA= Yoshihiro Urade, dXJhZGV5QGdtYWlsLmNvbQ==
†Present address: Kosuke Aritake, School of Pharmaceutical Sciences, Daiichi University of Pharmacy, Fukuoka, Japan