- Institute for Biochemistry, Charité Universitätsmedizin Berlin, Berlin, Germany
Astrocytes are the most prevalent glial cells in the brain. Historically considered as “merely supporting” neurons, recent research has shown that astrocytes actively participate in a large variety of central nervous system (CNS) functions including synaptogenesis, neuronal transmission and synaptic plasticity. During disease and injury, astrocytes efficiently protect neurons by various means, notably by sealing them off from neurotoxic factors and repairing the blood-brain barrier. Their ramified morphology allows them to perform diverse tasks by interacting with synapses, blood vessels and other glial cells. In this review article, we provide an overview of how astrocytes acquire their complex morphology during development. We then move from the developing to the mature brain, and review current research on perisynaptic astrocytic processes, with a particular focus on how astrocytes engage synapses and modulate their formation and activity. Comprehensive changes have been reported in astrocyte cell shape in many CNS pathologies. Factors influencing these morphological changes are summarized in the context of brain pathologies, such as traumatic injury and degenerative conditions. We provide insight into the molecular, cellular and cytoskeletal machinery behind these shape changes which drive the dynamic remodeling in astrocyte morphology during injury and the development of pathologies.
Introduction
Astrocytes have classically been depicted as star-like cells (Ramón y Cajal, 1913), however advanced visualization techniques have instead revealed astrocytes as bush- or sponge-like cells, each of which covers a distinct territory in the central nervous system (CNS; Bushong et al., 2004; Benediktsson et al., 2005). One of the most intriguing features of mature astrocytes is their extraordinary complexity: at high resolution, the ramification of the spongiform astrocytic processes into myriads of nanoscopic protrusions can be observed, frequently of sizes below the diffraction limit of light, and associated with synapses (Witcher et al., 2007; Medvedev et al., 2014). Their morphological complexity correlates with the plethora of functions astrocytes execute in the healthy CNS, including maintaining homeostasis, providing metabolic and neurotrophic support, promoting synaptogenesis, neurotransmitter uptake and recycling, modulating the plasticity and density of synapses. All of these tasks require close contacts between astrocytes and their targets, where the interactions are particularly plastic and can change depending on the individual physiological conditions. Moreover, astrocytes respond to pathologies in a process known as reactive astrogliosis, when they undergo substantial morphological changes to protect the CNS from inflammation, infection and neurodegeneration. In this review article, we summarize current knowledge of how astrocytes acquire, maintain and change their elaborate morphology through their molecular machinery and, in particular, via the cytoskeleton. We begin by describing the developmental process of astrogenesis and then focus on how astrocytes associate with and influence synapses in the mature CNS via their smallest processes, the perisynaptic astrocytic processes (PAPs). Finally, we discuss reactive astrogliosis and concentrate on the pathologies leading to the most profound shifts in astrocyte shape.
Development: How to Become a Star
Like neurons, astrocytes originate from radial glial cells, which, despite their uniform appearance, form different progenitor domains within the ventricles of the developing brain, and generate cell subtypes with distinct morphological, functional and positional identities. Neurogenesis and gliogenesis from radial glial cells follow a step-like arrangement during development (Hirabayashi and Gotoh, 2005). In the mouse brain, neurogenesis starts at day E11 of prenatal development. The first cells exhibiting astrocyte characteristics appear between E16–E18, when neurogenesis decreases in favor of gliogenesis (Costa et al., 2009; Gao et al., 2014). However, the vast majority of astrocytes will only become detectable during the first 3 weeks after birth. Astrogenesis requires the early commitment of glial cell precursors to the astrocyte lineage, and the subsequent colonization of the CNS by differentiating astrocytes.
Early and intermediate astrocyte progenitors are difficult to trace and manipulate. To our knowledge, unique factors which actively instruct precursors to differentiate into astrocytes have not to date been identified. Rather, it appears that astrogenesis relies on the repression of neurogenic genes through numerous signaling pathways (Kanski et al., 2014; Nagao et al., 2016). However, the pro-gliogenic transcription factors involved are not restricted to astrocyte differentiation as they are also required for the generation of oligodendrocytes (Stolt et al., 2003). To acquire their positional identity, differentiating astrocytes “re-use” molecular mechanisms, such as the homeodomain code, which neurons follow earlier in development (Hochstim et al., 2008). Subsequent to the principal commitment of precursor cells to the astrocyte lineage, astrocyte progenitors leave their cradles and populate the entire CNS (Bandeira et al., 2009). Region-specific fate mapping in the spinal cord and cortex revealed that the migration of astrocyte progenitors occurs along radial glial cell processes (Figure 1A; Tsai et al., 2012). However these processes disappear early in postnatal development, raising the question as to how later-appearing astrocytes reach their specific locations throughout the CNS (Rakic, 2003). One possible explanation is that early emerging astrocytic precursors migrate along the radial glial cells and pioneer unhabituated brain regions. After reaching their final positions, these pioneer astrocytes expand by symmetric cell division and colonize defined brain areas. Tracing experiments in the postnatal cortex demonstrated the capability of astrocytes to generate up to 50% of the total astrocyte numbers via symmetric cell division (Ge et al., 2012). Another 10% of cortical astrocytes are generated directly from radial glial cells during their final cell division (Masahira et al., 2006). The remaining 40% of the total astrocyte population are derived from progenitor cells originating from the subventricular zone (SVZ). Despite the absence of the guiding processes of radial glial cells, this astrocyte population nonetheless continues to migrate along the tracks of pioneering cells (Jacobsen and Miller, 2003; Rakic, 2003). It is currently unknown how these “latecomers” move along formerly beaten tracks, nor how they find their precise destination in the developing brain. These “second wave” astrocytes typically cover shorter distances compared to the pioneering astrocytes, and populate the cortical layers adjacent to the SVZs (Figure 1B; Ge et al., 2012).
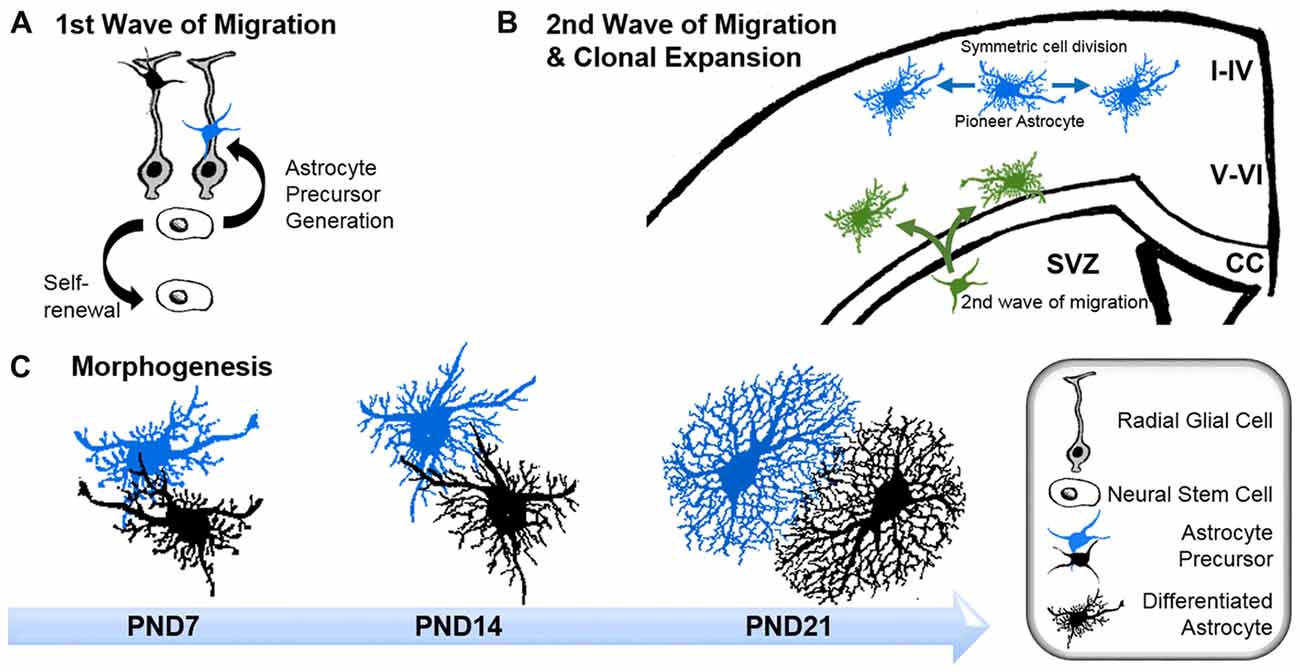
Figure 1. Astrogenesis and morphogenesis of astrocytes during development. (A) First wave of progenitor migration: asymmetric division of neural stem cells within the ventricular zones creates the first wave of astrocyte precursor cells (black and blue), which migrate along the processes of radial glial cells (gray) towards their final location in the central nervous system (CNS). (B) Clonal expansion of pioneering astrocytes and second wave of migrating astrocyte progenitor during late development: pioneering astrocytes of the first migration wave (blue) undergo symmetric cell division in the upper cortical layers, while a second wave of astrocyte progenitors emerges from the subventricular zone (SVZ; green), predominantly colonizing the lower cortical layers. Corpus callosum (CC). (C) Time course of astrocyte morphogenesis during postnatal development, demonstrated by two neighboring astrocytes (blue and black). Differentiating astrocytes possess long main processes at postnatal day (PND) 7, which invade the domains of neighboring astrocytes. At PND14, ramification into smaller processes has increased, whilst the extent of invasion into domains of neighboring astrocytes is reduced. Three weeks after birth, at PND21, astrocytes have acquired their complex morphologies within their distinct domains. At this stage, only very limited intermingling with neighboring astrocytes occurs.
After arriving at their designated position, astrocytes begin to express their canonical markers, such as glial fibrillary acidic protein (GFAP), S100β, Aldh1L1, Sox9, AldoC, Glt1 and glutamine synthase (for a review see Molofsky et al., 2012). During this period, astrocytes also initiate the formation of their stellate morphology (Figure 1C). The time course in forming the uniquely ramified morphology of astrocytes has been documented in rats by Bushong et al. (2004) using immunolabeling and dye filling. In brief, during the first week of postnatal development, protoplasmic astrocytes display considerable diversity in morphology. S100ß and GFAP-positive cells extend between three and six long main processes and develop a ramification of fine, stringy or filamentous processes. At this stage, astrocytes are not yet restricted to the defined domains typically observed for mature astrocytes, and can extend their long main processes beyond any rudimentary boundaries. By postnatal week 2, astrocytes have adopted a more uniform morphology, with complex ramification patterns of their processes. However, the astrocytic processes are still stringy or filiform and have not yet matured into the characteristic spongiform shapes. During this period, the well-documented astrocytic territorial domains are recognizable for the first time, although overlap of processes is still present. By postnatal week 3–4, astrocytes have matured into their terminally differentiated state through the establishment of dense spongiform processes within their individual domains. However, the morphological and functional identity of astrocytes is not entirely hardwired but rather depends on the individual environment, particularly on the input of surrounding neurons throughout their lifetime.
Scientists are only now beginning to comprehend the molecular signals and their downstream mechanisms involved in establishing astrocyte morphology. Genetic studies in Drosophila identified neuron-secreted FGFs as critical for the elaboration of astrocyte morphology (Stork et al., 2014). Recent work in the optical lobe of Drosophila showed a role for the transmembrane leucine-rich repeat protein Lapsyn in regulating the morphogenesis of the astrocyte-like medulla neuropil glia, which also cooperates with FGF to promote astrocyte branch formation and survival (Richier et al., 2017). Moreover, a comprehensive study in the rodent cerebellum indicated that the acquired identity of astrocytes could be overwritten by surrounding neurons (Farmer et al., 2016). In this study, the authors demonstrate that the morphogen sonic hedgehog secreted from the adult Purkinje cells sustains the functional identity of adjacent Bergmann glia. Manipulating sonic hedgehog in the mature cerebellum induces another astrocyte subpopulation, so-called velate astrocytes, to acquire the transcriptome and electrophysiological characteristics of Bergmann glia. Taken together, these studies show that astrocytes represent a population of particularly plastic and heterogeneous cells, which are responsive to their neuronal neighbors.
What Stars Are Made of and What Keeps Them in Shape
The development of the stunning complexity of astrocytes from the thin precursor cylindrical radial glial cells necessarily involves extensive remodeling of the cytoskeleton. However very little is known about the molecular machinery behind these comprehensive shapeshifts. A key reason contributing to this limited insight is that the commonly-used cell culture astrocyte models do not accurately recapitulate astrocytes in situ or during normal morphogenesis. While neurons develop spontaneously from apolar progenitors and form mature cells with elaborate axon and dendrite morphologies in culture, astrocytes in serum-enriched cultures acquire a polygonal morphology analogous to non-neuronal cells (McCarthy and de Velllis, 1980). More importantly, transcriptome analysis revealed that cultured polygonal astrocytes have different genetic profiles compared to astrocytes in situ, but share similar profiles with immature and reactive astrocytes (Foo et al., 2012). Changes in culture conditions and pharmacological treatments, such as artificially increasing intracellular cAMP levels, can convert polygonal astrocytes into stellate astrocytes within a matter of minutes (Shapiro, 1973). However, this process does not resemble in vivo differentiation of progenitor cells into ramified astrocytes. Instead, this so-called process of “stellation” is a model used to identify the cellular architecture necessary to maintain the typical astrocyte morphology. Keeping these limitations in mind, we review here what is known about the cytoskeletal organization of stellate astrocytes in culture and in vivo.
Microtubules
The function of microtubules has rarely been addressed in stellate astrocytes in general, and during astrogenesis in particular. An early electron microscopy study demonstrated dense microtubule networks in mature astrocytes, compared to immature astrocytes that exhibit more loosely packed microtubules (Peters and Vaughn, 1967). Only recently, microtubules were visualized for the first time in radial glia and their astroglia progeny in living brain tissue (Eom et al., 2011). Short-term live imaging revealed the restriction of microtubules to the main processes, where they appear to be relatively stable. In cell culture, the in vivo distribution of microtubules is analogously present in stellating astrocytes, where microtubules co-extend with intermediate filaments in forming the processes. Pharmacological inhibition of microtubule polymerization during stellation prevents the transition of astrocytes into the star-like cells (Goetschy et al., 1986). Nonetheless comprehensive analyses of the changes and functions of microtubules in astrocytes during stellation are not currently available and further studies are needed.
Intermediate Filaments
Intermediate filaments have been studied extensively in astrocytes thanks to the availability of knockout mouse models. Like microtubules, intermediate filaments are restricted to the main processes of astrocytes in vivo (Figure 2A; Bushong et al., 2002). The interconnected scaffold-like network in astrocytes is a composite of different intermediate filament proteins which change during development and maturation. Astrocyte progenitors express the intermediate filament proteins of vimentin, nestin and synemin, whereas maturing and differentiated astrocytes express only GFAP and vimentin (Sultana et al., 2000). During adulthood, GFAP expression in astrocytes varies largely depending on the brain region. In the cortex, 85% of astrocytes are negative for GFAP but upregulate this intermediate filament protein again during aging (Kimelberg, 2004). GFAP or vimentin are the essential subunits for polymerizing intermediate filaments. In immature astrocytes deficient in both GFAP and vimentin, nestin itself is unable to polymerize into filaments (Pekny et al., 1999). Intermediate filaments also play a scaffolding role in organizing the cytoplasm and organelles, and modulate directed vesicle transport (Potokar et al., 2007). However, deficiencies in GFAP and/or vimentin have no obvious effect on the outgrowth of astrocytic processes in mixed neuronal cultures or on the development and maturation of astrocytes in vivo, despite impaired intermediate filament formation (Pekny et al., 1995, 1998).
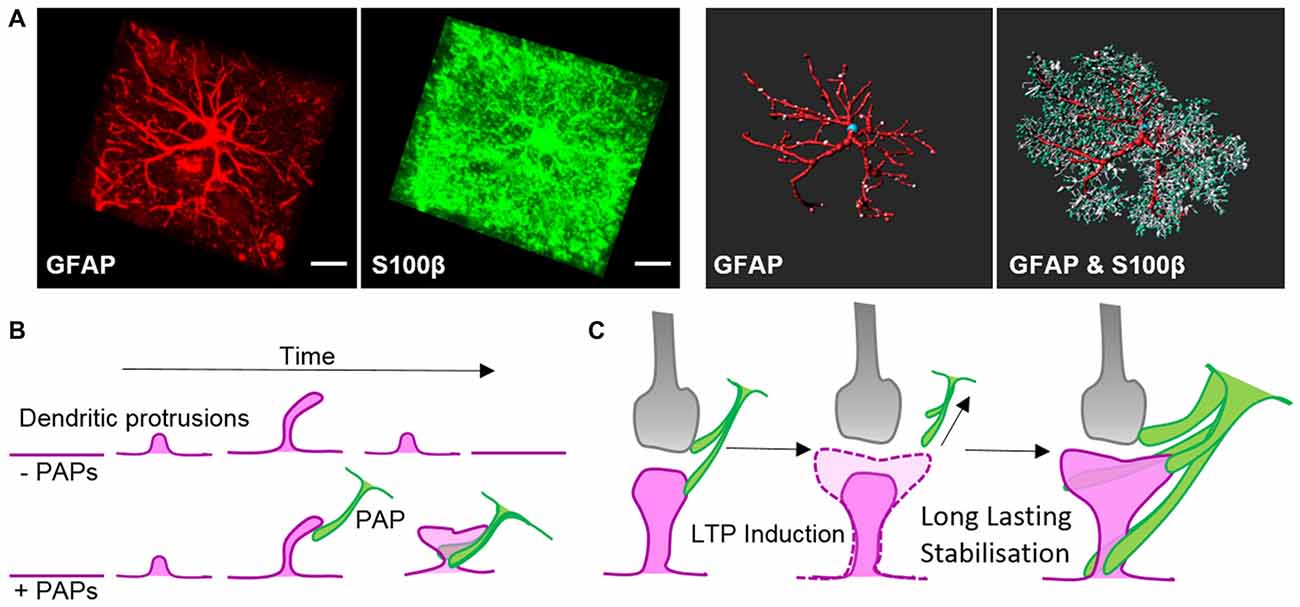
Figure 2. Morphology of astrocytes in vivo and their dynamic association with synapses. (A) Z-projection of a cortical rat astrocyte (P14), stained for glial fibrillary acidic protein (GFAP; red) and S100β (green), by confocal microscopy after tissue clearance (left). Scale bars: 10 μm. 3D rendering and morphometric analyses show the restriction of the intermediate filament protein GFAP to main processes, which are decorated with myriads of fine S100β-positive processes (right; with permission from Murk et al., 2013). (B) Schematic of dendritic filopodia, with the precursors of dendritic spines, emerging from dendrites (magenta) in the absence (top) or presence of perisynaptic astrocytic processes (PAPs; green, bottom). Without the support of astrocytic processes, sprouting dendritic filopodia have a short lifespan and are likely to retract. Astrocytic processes contact filopodia-like protrusions, which then exhibit an increased stability and higher tendency to develop into mature dendritic spines. (C) Schematic of a mature tripartite synapse consisting of the pre-synapse (gray), post-synapse (magenta) and PAPs (green), which respond to long-term potentiation (LTP) with structural changes. Induction of LTP transiently enhances the motility and retraction of PAPs allowing growth of the postsynaptic dendritic spine to occur. Subsequent to dendritic spine remodeling, PAPs intensify their coverage of synapses.
The Actin Cytoskeleton
Studies that induced drastic shapeshifting between polygonal and stellate morphologies of astrocytes in cell culture have increased our understanding of the actin cytoskeleton in this process. In their polygonal shape, astrocytes possess prominent actin fibers that depend on a high activation state of myosin-II (John, 2004). In contrast, when astrocytes adopt stellate shapes in vitro, they exhibit a lack of actin fibers and instead establish Arp2/3-dependent actin networks (Murk et al., 2013). Branched Arp2/3-based actin arrays and the machinery for linear actin filaments act in direct opposition to each other, and rely on distinct signaling pathways (Rotty et al., 2015). Signaling through receptors such as beta-adrenergic receptors activates PKA and/or PKCepsilon and inhibits the ROCK-RhoA-axis. Concurrently, an increase in Rac1 activity drives the remodeling of contractile actin fibers into branched actin arrays (Moonen et al., 1976; Ramakers and Moolenaar, 1998; Burgos et al., 2007; Racchetti et al., 2012; Kobayashi et al., 2013; Murk et al., 2013). In addition to experiments using polygonal astrocytes, improved cell culture models based on defined culture media (Scholze et al., 2014; Wolfes and Dean, 2018), immunopanning (Foo et al., 2012), 3D scaffolds and matrices (Lau et al., 2014; Woo et al., 2017), and organoids (Renner et al., 2017) will facilitate functional studies of the underlying molecular machinery controlling astrocyte morphogenesis.
A Starring Role for Astrocytes at Synapses
The main processes of astrocytes form the basis of their star-like shape, while their true spongiform morphology relies on myriads of small protrusions. After birth, the sophisticated ramification into nanoscopic astroglial protrusions occurs in parallel with synaptogenesis, when astrocytes begin to associate with developing neuronal connections via PAPs and establish “tripartite synapses.” The frequency of establishing tripartite synapses depends on the brain area and local neuronal activity (Araque et al., 1999). Studies in different organisms from Drosophila to humans indicate PAPs as conserved structures that are essential for brain function. Consistently, the number of PAP-associated synapses covered by astrocytes is increasing from fly to human (Oberheim et al., 2009; Stork et al., 2014). For instance, a single rodent astrocyte can associate with up to 120,000 synapses (Bushong et al., 2002), whereas a human astrocyte, according to a several-fold larger cell size and a 10-fold increase in the number of main processes, contacts up to two million synapses (Oberheim et al., 2006, 2009). These hominid-specific characteristics in astrocyte morphology are fundamental for sophisticated learning and memory, as shown in chimeric mice harboring human induced pluripotent stem cells (iPSC)-derived astrocytes. The transplanted human astrocytes replace the host’s astroglia and develop the characteristic larger ramification of normal human astrocytes. Moreover, the chimeric mice show substantially enhanced synaptic plasticity and learning, compared to control animals (Han et al., 2013). Although further experimental evidence is needed to directly test for the link of PAPs in moderating synaptic responses in tripartite synapses, the correlation of enhanced learning and memory with increasing ramification and astrocyte-synapse interactions is impressive. It implies PAPs are key structures that astrocytes require to actively partake in synaptic activity.
Astrocytes control the ionic homeostasis of the CNS (Simard and Nedergaard, 2004) where they modulate synaptic plasticity by active buffering of potassium ions (Pannasch et al., 2011). In addition, astrocytes clear neurotransmitters and recycle their inactive derivatives back to the presynaptic terminal (Schousboe et al., 2013). Gliotransmitters such as d-serine, glutamate, ATP, taurine and TNFα are secreted by astrocytes and regulate synaptic activity (Panatier et al., 2006; Stellwagen and Malenka, 2006; Cao et al., 2013; Martin-Fernandez et al., 2017; Tan et al., 2017; Van Horn et al., 2017). During postnatal development, astrocytes sequentially secrete a range of factors, such as thrombospondins and hevin, that are involved in initiating the formation of silent synapses (Christopherson et al., 2005; Kucukdereli et al., 2011; Singh et al., 2016). Subsequently, other astrocyte-secreted factors, such as glypicans 4 and 6, turn the established silent synapses into active connections (Allen et al., 2012). To establish and maintain a neuronal network with individually controllable synapses through diffusible molecules require a tight spatial-temporal control of signaling in vicinity of synapses. The molecular equipment required for these tasks, including metabotropic glutamate receptors (mGluRs), glutamate transporters and ion channels, is enriched in PAPs and is thus in immediate proximity to synapses (Chaudhry et al., 1995; Higashi et al., 2001; Lavialle et al., 2011).
Along with locally secreted factors, PAPs influence synapses through direct contact mediated by cell adhesion molecules. Filopodial precursors of dendritic spines are endowed with a substantially enhanced lifespan and develop into mature dendritic spines with greater frequency in direct association with PAPs (Figure 2B, Nishida and Okabe, 2007). Immunohistochemical and electron microscopy studies showed several cell adhesion molecules that appear to link PAPs to synapses. Several molecules, such as SynCAM1, NCAM and αvβ3-integrins, form connections between PAPs and synapses (Theodosis et al., 2004; Hermosilla et al., 2008; Sandau et al., 2011), but their true nature regarding astrocyte-neuron interactions still needs to be demonstrated in functional approaches. One striking example of a shapeshifter transmembrane protein on astrocytes that affects neuronal functions is neuroligin 2. Neuroligin 2 localizes to astrocyte processes and its specific deletion in astrocytes affects astrocyte morphogenesis and substantially imbalances neuronal circuits by impairing the formation of excitatory synapses (Stogsdill et al., 2017). The γ-protocadherins (γ-Pcdhs) are another candidate for cell adhesion molecules that can functionally connect astrocytes and neurons (Keeler et al., 2015). γ-Pcdhs are present on PAPs and directly drive synaptogenesis in neuron-astrocyte co-culture models (Garrett and Weiner, 2009). However, γ-Pcdhs also directly link neuronal pre- and post-synapses (Rubinstein et al., 2015; Molumby et al., 2016, 2017). Accordingly, the modulation of synapses via γ-Pcdhs likely only relies on PAPs to some extent.
Several studies indicate a supportive role for astrocytes on synapses, whereas others discovered an essential function in reducing the total number and structural plasticity of synapses. One of the first pairs of cell adhesion molecules analyzed in a functional approach is the receptor tyrosine kinase EphA4 and its ligand ephrin-A3 that exhibit a negative regulative effect on excitatory synapses. In the adult hippocampus, EphA4 is restricted to dendritic spines, whereas ephrin-A3 is enriched on adjacent PAPs. The interaction of neuronal EphA4 with astrocytic ephrin-A3 evokes spine retraction, a process which is distorted in EphA4-deficient mice (Murai et al., 2003). Reverse signaling from neuronal EphA4 towards astrocytic ephrin-A3 evokes decreased levels of the glutamate transporters Glt1 and GLAST in PAPs, which correlates with shrinking dendritic spines (Carmona et al., 2009; Filosa et al., 2014). EphA4-ephrin-A3 signaling thus represents a means of how cell-cell contact-based neuron-glial communication can induce negative structural plasticity in neurons. In addition, astrocytic processes contain the phagocytic receptors MERKT and MEGF10 that have both been identified in mediating synapse elimination through active engulfment (Chung et al., 2013).
The Dynamic Cytoskeleton in PAPs
The interplay of PAPs with synapses alters depending on the organism’s physiological condition, such as parturition, lactation, chronic dehydration, starvation, voluntary exercise or sleep deprivation (Theodosis, 2002; Procko et al., 2011; Tatsumi et al., 2016; Bellesi et al., 2017). Recently, quantitative measurements in the sensory-visual cortex during eye occlusion demonstrated the plasticity of PAPs covering synapses. These experiments demonstrated clearly that PAPs are particularly dynamic during development as well as during activity of synaptic circuits (Stogsdill et al., 2017). Overall morphological changes in PAPs in response to environmental cues seem to occur slowly over hours to days, whereas live imaging experiments ex and in vivo revealed extensive structural plasticity PAPs within much shorter time-frames—of only minutes (Figure 2C; Bernardinelli et al., 2014; Perez-Alvarez et al., 2014). Activation of synapses through stimulating metabotropic glutamate receptors or glutamate uncaging triggered transiently increased PAP motility which ultimately evoked a more stable interaction of PAPs with dendritic spines (Figure 2C; Bernardinelli et al., 2014).
Rapid changes in PAPs highlight a prominent role for a dynamic cytoskeleton during structural remodeling of astrocytes (Bernardinelli et al., 2014). In view of the fact that PAPs are devoid of microtubules and intermediate filaments, all structural changes in PAPs are likely to involve the reorganization of the actin cytoskeleton. Dynamic PAPs present as miniature versions of lamellipodia and filopodia, the F-actin-rich subcellular compartments of migrating non-neuronal cells (Hirrlinger et al., 2004). Analogous to typical lamellipodia, extensions of PAPs are severely impaired upon inactivating the small GTPase Rac1 (Nishida and Okabe, 2007), and likely depend on inhibition of Rac1 downstream targets, such as actin regulators forming Arp2/3-dependent branched actin arrays (Rottner et al., 2017). Accordingly, direct inhibition of the Arp2/3 complex in brain tissue or knockdown of its upstream regulators N-WASP, WAVE2 and PICK1 in cell culture induces profound alterations in the morphological complexity of astrocytes. On the one hand, Arp2/3 inactivation in situ has been associated with the loss of fine astrocytic processes (Murk et al., 2013). On the other hand, small G-actin binding proteins called profilins, which charge actin monomers with ATP and mainly enhance actin polymerization (Jockusch et al., 2007), modulate the overall complexity of astrocytes and actin turnover in PAPs. Isoform-specific knockdowns of either ubiquitous profilin 1 or CNS-specific profilin 2a which is involved in neurons in presynaptic membrane trafficking and dendritic spine remodeling (Pilo Boyl et al., 2007; Michaelsen et al., 2010), reduce the total volume of astrocytes in organotypic slices. However, selective inhibition of profilin 1 affects the number and movement of filopodia processes by slowing the reorganization of filamentous actin (Molotkov et al., 2013; Schweinhuber et al., 2015).
Another actin binding protein prominently localized to PAPs is ezrin, a linker protein connecting the actin cytoskeleton directly to the plasma membrane (Derouiche and Frotscher, 2001; Haseleu et al., 2013). Active ezrin is exclusively located in PAPs and is required for motility of astrocytic filopodia induced by glutamate-activating mGluR3 and 5 (Lavialle et al., 2011). In addition to the typical actin regulators, connexin30 has also been shown to regulate synaptic strength by controlling the synaptic location of astroglial processes (Pannasch et al., 2014). Deletion of connexin30 evokes increased ramification and process length, with PAPs invading the synaptic cleft and causing elevated uptake of glutamate at excitatory synapses. The molecular details that underlie connexin30 regulation of PAPs are currently unclear, but appear to involve its intracellular C-terminus, which is likely to be a hub for interactions with as-yet unidentified actin regulators. A potential candidate might be drebrin, an actin regulator binding sidewise to filaments, which has been shown to interact with connexin43 in cultured astrocytes (Butkevich et al., 2004). However, whether drebrin does indeed bind and control connexin30 function in astrocytes is not known.
Stars With Sensitive Feet
While astrocytes use PAPs to register, support and modulate neuronal activity at synapses, they also almost entirely encompass the vasculature of the CNS with processes known as endfeet. In conjunction with specialized endothelial cells, pericytes and an elaborate basal lamina, astrocytic endfeet create the blood brain barrier to facilitate the brain’s selective uptake of required nutrients and metabolites, the exclusion of toxic substances and immune cells as well as the efflux of waste products (Daneman and Prat, 2015). Perivascular endfeet possess prominent orthogonal arrays of intramembranous particles at their plasma membrane, where aquaporins and potassium channels accumulate (Rash et al., 1998; Warth et al., 2005). Accordingly, astrocytic endfeet act as major hubs to regulate the ion and water homeostasis in the CNS (Min and van der Knaap, 2018). Endfeet and PAPs emplace astrocytes as cellular interface between synapses and vasculature, where they enable the appropriate supply of oxygen and energy to neurons according to their activity-dependent demands. Intensive research showed the ability of astrocytes to regulate the local cerebral blood flow by sensing and relaying neuronal signals to the vasculature. The currently discussed mechanisms of astrocyte-mediated blood flow control comprise potassium siphoning, metabolic neurovascular coupling and intracellular calcium waves, which evoke the synthesis of vasoactive metabolites (see for review MacVicar and Newman, 2015). Despite the relevance of endfeet for astrocyte functions, very little information is available on their intracellular structures. Microtubules have been visualized in endfeet of perivascular astrocytes in a single in vivo study but their role in this subcellular compartment is unknown (Eom et al., 2011). Immunohistochemical analyses revealed prominent GFAP-positive intermediate filaments in endfeet. Endfeet differ distinctively in their fine structures between rodents and humans. Perivascular GFAP appears in rats as rosettes around blood vessels and creates the impression of an incomplete coverage of the vasculature by the astrocyte endfeet (Rungger-Brändle et al., 1993). In contrast, in humans GFAP in endfeet exhibits a densely packed, tile-like pattern entirely encompassing the blood vessels (Oberheim et al., 2009). More recent electron microscopy 3D reconstructions demonstrated the complete coverage of blood vessels in the rat brain by astrocyte endfeet despite the rosette-like GFAP localization (Mathiisen et al., 2010). Under normal conditions, blood vessels in the brain and spinal cord from GFAP- and vimentin-deficient mice frequently show increased dilatation (Pekny et al., 1999). To our knowledge, the microanatomy of actin filaments in astrocyte endfeet is unknown. Indications for a putative role of actin in astrocytes are indirect, reported from loss-of-function studies and cell culture experiments. Astrocyte endfeet have enriched protein complexes composed of the transmembrane protein dystroglycan and its associated ligands syntrophin and dystrophin, and in other cell types this complex bridges the actin cytoskeleton with laminins in the extracellular matrix (Higginson and Winder, 2005). Moreover, the defined localization of potassium channels and aquaporin-4 in the plasma membrane of endfeet partially depends on tethered dystroglycan protein complexes. In cell culture, the actin cytoskeleton directly governs the localization of aquaporin-4 (Nicchia et al., 2008). Deleting the focal adhesion adapter protein vinculin in Bergmann glia disturbs the GFAP distribution in endfeet but has no obvious effect on neurovascular functions (Winkler et al., 2013).
From Stars to Scars
Dealing With Insults
Under normal conditions, the majority of astroglia occupy a distinct territory in the brain parenchyma, with the most distant processes of neighboring astrocytes exhibiting very limited intermingling (Bushong et al., 2002). In terms of morphological plasticity, microscopic observations at low magnification give the impression that astrocytes are rather quiescent under normal conditions. Only the smallest astrocyte protrusions—such as PAPs—are motile and alter their shape upon neuronal activity. However, in response to injury and other pathological conditions, astrocytes undergo astrogliosis and become “reactive.” This process is accompanied by dramatic changes in morphology, including prominent hypertrophy, altered ramification and outgrowth of particularly long processes (Figure 3). Astrogliosis is recognized as a defense mechanism that controls inflammation and the blood-brain barrier integrity. An important contribution of astrogliosis is the isolation of non-injured tissue from damaged areas, and the support of neuronal circuit and tissue regeneration (Burda et al., 2016). The molecular triggers and specific signaling mechanisms of reactive astrogliosis have been reviewed in detail (Sofroniew, 2009; Ben Haim et al., 2015); here we discuss known morphological changes that occur during reactive astrogliosis and present the scope of their physiological function.
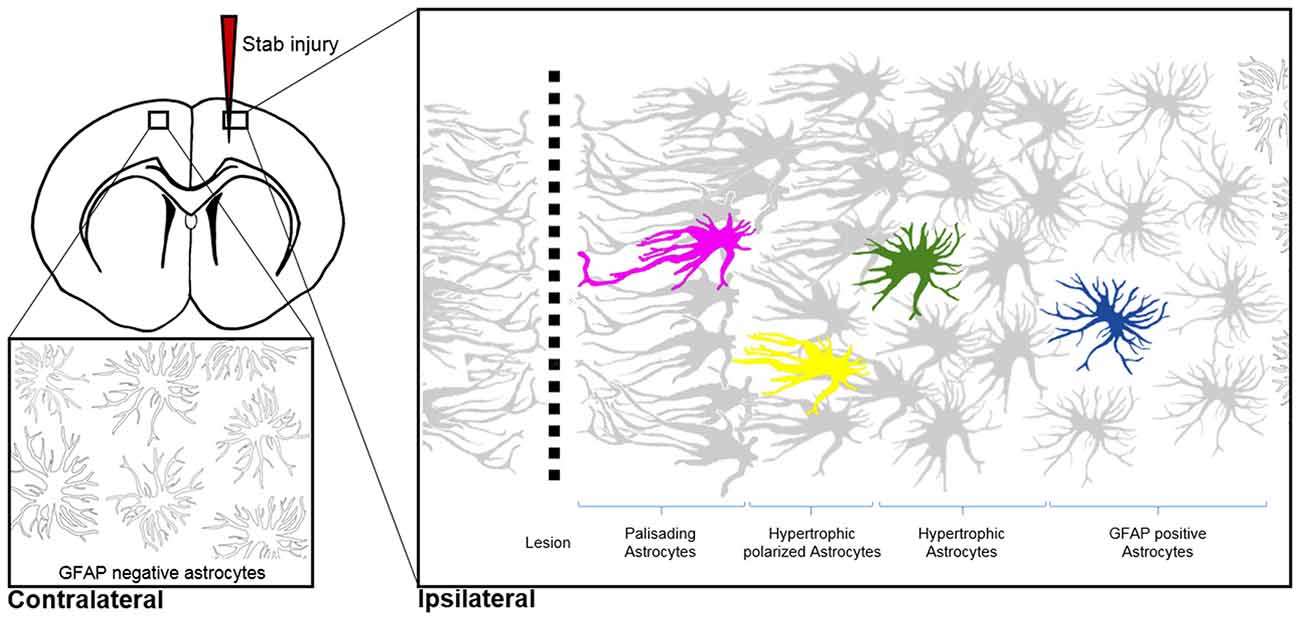
Figure 3. Morphological hallmarks of reactive astrocytes in traumatic brain injury. Typical distribution of reactive astrocytes after a cortical stab wound in the mouse cortex 7 days after injury. Schematic drawings show the main processes of astrocytes, which contain high levels of GFAP (gray) upon injury. GFAP-positive astrocytes exhibit different morphologies depending on their distance from the lesion site: in the immediate vicinity (magenta), astrocytes polarize and extend long “palisading” processes. In the second row, multiple processes of the hypertrophic astrocytes (yellow) are orientated towards the injury site; with increased distance, astrocytes possess hypertrophic cell bodies and primarily main processes (green). Finally, reactive astrocytes at the border of the unaffected parenchyma upregulate GFAP but show no signs of hypertrophy (blue). Note that cortical astrocytes in the uninjured contralateral hemisphere sparsely express GFAP, as indicated with silhouettes (bottom left image).
Shapeshifting of reactive astrocytes depends on the nature and severity of the CNS insult. Acute and diffuse trauma without tissue damage evoke a transient upregulation of GFAP and other intermediate filament proteins in conjunction with minor and reversible hypertrophy of both astrocyte cell bodies and main processes (Wilhelmsson et al., 2006). Reactive astrocytes thereby uphold their local domains and do not proliferate. Longer lasting and more severe injuries provoke higher GFAP levels in astrocytes and lead to more prominent cell body hypertrophy associated with the interpenetrative extension of distant processes into adjacent astrocyte domains and occasional hot spots of cell proliferation (Myer et al., 2006). Reverting hypertrophy and downregulating GFAP levels in highly reactive astrocytes is possible, but correlates negatively with the duration and severity of the diffuse brain injury (Petito et al., 1990). Traumatic brain injuries with severe tissue penetration and profound lesions create the most prominent response of reactive astrocytes by collectively forming a glial scar to protect the surrounding parenchyma from spreading inflammation, infection and neurodegeneration (Figure 3). Astrocytes that are in the vicinity of focal lesions resemble “palisades,” bordering, orienting and extending long processes towards the injury site. Live imaging experiments in mice with cortical stab wounds revealed that 45% of reactive astrocytes are polarized. Polarized and elongated cells originate from mature astrocytes through their transient de-differentiation and proliferation (Bardehle et al., 2013; Wanner et al., 2013). Astrocytes behind the palisades manifest prominent hypertrophy and a tendency to orientate most processes towards the lesion (Kanemaru et al., 2013). With increasing distance from the injury, the hallmarks of astrogliosis—such as hypertrophy and elevated GFAP levels—tend to decrease, and appear to be analogous to mild diffuse CNS injuries (Figure 3). Live imaging and ablation experiments demonstrated the local occurrence of reactive astrogliosis at the insult site without any active migration of additional astrocytes from neighboring brain areas (Bardehle et al., 2013; Tsai et al., 2012).
In comparison to microglia that respond to CNS insults within minutes, the time course of morphological changes for astrocytes is relatively slow (Nimmerjahn et al., 2005). Hypertrophy and GFAP upregulation appear after 2–3 days post-injury, with palisading astrocytes observed approximately 1 week after injury (Robel et al., 2011). Nevertheless, astrogliosis and glial scarring are essential for the early phase of tissue protection after traumatic injuries, as reactive astrocytes protect against spreading cell death and inflammation after spinal cord injuries (Faulkner et al., 2004). In close proximity to lesion sites, palisading and hypertrophic astrocytes sustain their reactivity permanently, whereas astrocytes at greater distances from the injury return to their normal state (Bardehle et al., 2013). Persistent glial scars have been considered a crucial part of the failure of regenerative treatments of traumatic brain and spinal cord injuries (Cregg et al., 2014). Whether reactive astrocytes represent a major hurdle or a benefit for regenerative treatments remains a topic of intensive debate (Anderson et al., 2016).
Stars in Disease
Scar-forming astrocytes have been reported in a number of pathological conditions such as Alzheimer’s disease (AD) and brain tumors, but follow a more complex response pattern during the progression of these diseases. During the later phases of AD, reactive astrocytes form prominent glial scar-like barriers around amyloid plaques and disrupt the anthropoid-specific architecture of non-reactive astrocytes in the neocortex (Colombo et al., 2002). Whether astrocyte reactivity also occurs in earlier phases of AD is currently under debate. PET scans in patients and some mouse models indicate astrogliosis as an early component of AD development (Heneka et al., 2005; Carter et al., 2012). In contrast, the triple transgenic mouse model of AD exhibits comprehensive cytoskeletal atrophy of astrocytes prior to amyloid plaque-associated astrogliosis (Kulijewicz-Nawrot et al., 2012). The precise role of astrocytes in AD needs to be further investigated as both astrocyte atrophy and astrogliosis may indicate the participation of astrocytes in the neuropathology of this disease.
Gliomas are also surrounded by activated astrocytes initially with prominent palisades (Figure 4; Le et al., 2003). However in this disease, the astrogliosis response is unable to isolate the tumor from the intact tissue. Gliomas exploit reactive astrocytes together with other cell types to create a favorable microenvironment. Neurotrophic factors and pro-matrix metalloproteinase-2 (MMP2) secreted from astrocytes promote tumor growth (Hoelzinger et al., 2007; Lee et al., 2009). Interestingly, gliomas enhance cell proliferation and invasive migration via an astrocyte-induced knockdown of the key tumor suppressor PTEN. The epigenetic downregulation of PTEN in the cancer cells relies on the uptake of microRNAs, which are packaged in exosomes and secreted from neighboring astrocytes upon tumor-derived signals (Zhang et al., 2015). Brain tumors then consume the bordering reactive astrocytes over time, before they invade and disrupt the surrounding tissue as well as the blood-brain barrier (Watkins et al., 2014).
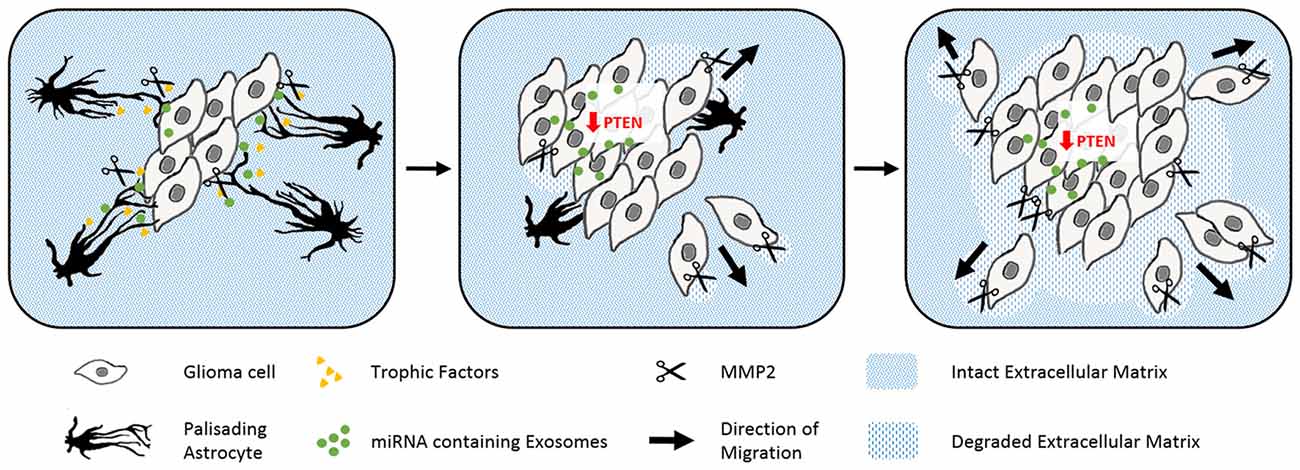
Figure 4. The abuse of scarring astrocytes by glioma. Schematic drawings of how reactive astrocytes turn from initially tumor containing to cancer promoting cells. Astrocytes (black) form prominent palisading processes in response to growing glioma masses (gray rhomboid cells). Glioma derived signals force the reactive astrocytes to secret trophic factors (yellow), pro matrix metalloproteinase-2 (scissors) and microRNA containing exosomes (green, left). The uptake of microRNA containing exosomes evokes the epigenetical downregulation of tumor suppressing PTEN in glioma. PTEN depletion and astrocyte-derived trophic signals enhance the cell proliferation of the glioma cells. Expanding tumor cells use matrix metalloproteinases to degrade the surrounding extracellular matrix (blue stripped pattern) and begin to penetrate the glial scar (center). In the late phase, glioma have comprehensively degraded their adjacent extracellular matrix and seemingly consumed the originally tumor encompassing astrocytes (right).
Profound changes in astrocyte cell morphology and function are induced by external signals and computed from pathological and physiological cues. Non-reactive astrocytes persistently survey their environment for abnormalities such as pathogenic, aggregated and serum proteins, as well as cytokines and chemokines. Contact with these cues activates multiple receptors and signaling pathways, which then trigger astrocyte reactivity programs (Burda et al., 2016). Besides pathological signals, astrocytes constantly receive instructions from healthy neurons, which actively suppress astrocyte reactivity. Key molecules within these signaling pathways include neuron-derived FGF-2 and astrocytic β1-integrin. Interestingly, loss-of-function of both factors causes astrocyte reactivity in the absence of any pathological condition (Robel et al., 2009; Kang et al., 2014). One important signal mediator involved in various signaling pathways, is the aforementioned lipid and protein phosphatase PTEN, a key molecule in antagonizing the PI3K signaling pathways (Kreis et al., 2014). PTEN is involved in the regulation of cell size and proliferation of astrocytes as well as the formation of glial scars (Fraser et al., 2004; Dey et al., 2008; Renault-Mihara et al., 2017). Mechanistic details from the nestin-STAT3+/– mouse model which has impaired astrogliosis show that the affected astrocytes upregulate PTEN. The intrinsic defects in glial scarring comprising process orientation and elongation as well as leukocyte seclusion are rescued through PTEN inhibition, which evokes substantial cytoskeletal remodeling by changing the activation state of the small GTPases RhoA and its downstream effectors (Table 1; Renault-Mihara et al., 2017).
In contrast to the wealth of knowledge on stimuli, receptors and signaling pathways evoking astrogliosis, we know very little about the downstream machinery exerting the functional and morphological changes in reactive astrocytes. One factor partially responsible for the swelling of astrocytes is simply water, which is increasingly taken up through aquaporin-4, and which reactive astrocytes upregulate and redistribute from their perivascular endfeet over the entire plasma membrane (Saadoun et al., 2005; Ren et al., 2013). Aquaporin-4-dependent water uptake contributes to the defense response of astrocytes, as shown in loss of function experiments, where deleting this water channel perturbs the ability of astrocytes to migrate in cell culture and to form glial scars in vivo (Saadoun et al., 2005). Furthermore, loss of aquaporin-4 impairs astrocyte secretion of proinflammatory cytokines in autoimmune encephalitis (Liu et al., 2014). However, the effective impact of aquaporin-4-dependent water uptake in reactive astrocytes in pathological conditions is currently under debate. Depending on the individual nature of the pathological condition, changes in astrocytic aquaporin-4 can either facilitate or counteract the formation of cerebral edema (for a review see Stokum et al., 2015).
Another major driving force in hypertrophy and process outgrowth in scar-forming astrocytes is the cytoskeleton. Below we discuss in depth the individual filament systems of the cytoskeleton in reactive astrocytes and their shape changes under pathological changes, and review in vivo analyses and studies using cultured astrocytes.
Microtubule Activity in Scar-Forming Astrocytes
Cell biology experiments provide substantial insights into the function of the microtubule network during palisading of scar-forming astrocytes. One of the most frequently used cell culture models is the scratch injury model of polygonal astrocytes grown in a 2D monolayer (Etienne-Manneville, 2006). This assay provokes the coordinated orientation, polarization and extension of astrocytes into the wound area, analogous to palisading astroglia during traumatic brain injury (Figures 5, 6), although it should be noted that cultured cells migrate unlike their counterparts in vivo (Bardehle et al., 2013). Astrocyte polarization begins with the microtubule-dependent reorientation of both the microtubule-organizing center and the Golgi apparatus (Etienne-Manneville and Hall, 2001). The reorientation of the Golgi in astrocytes by microtubules is distinct from that in other cells, as Golgi alignment relies solely on the actin cytoskeleton during migration of most other cell types (Magdalena et al., 2003). Microtubules are particularly enriched in long astrocytic processes (up to 150 μm; Figure 6). Moreover, microtubules reach the extreme tip of these astrocyte protrusions in contrast to other cell types, where only a minority of microtubules selectively enter the actin-enriched leading edge (Schober et al., 2007; Dent et al., 2011; Sakamoto et al., 2013). Treatment with the tubulin depolymerizing agent nocodazole demonstrated the integral function of microtubules for the directed outgrowth of palisade-like processes.
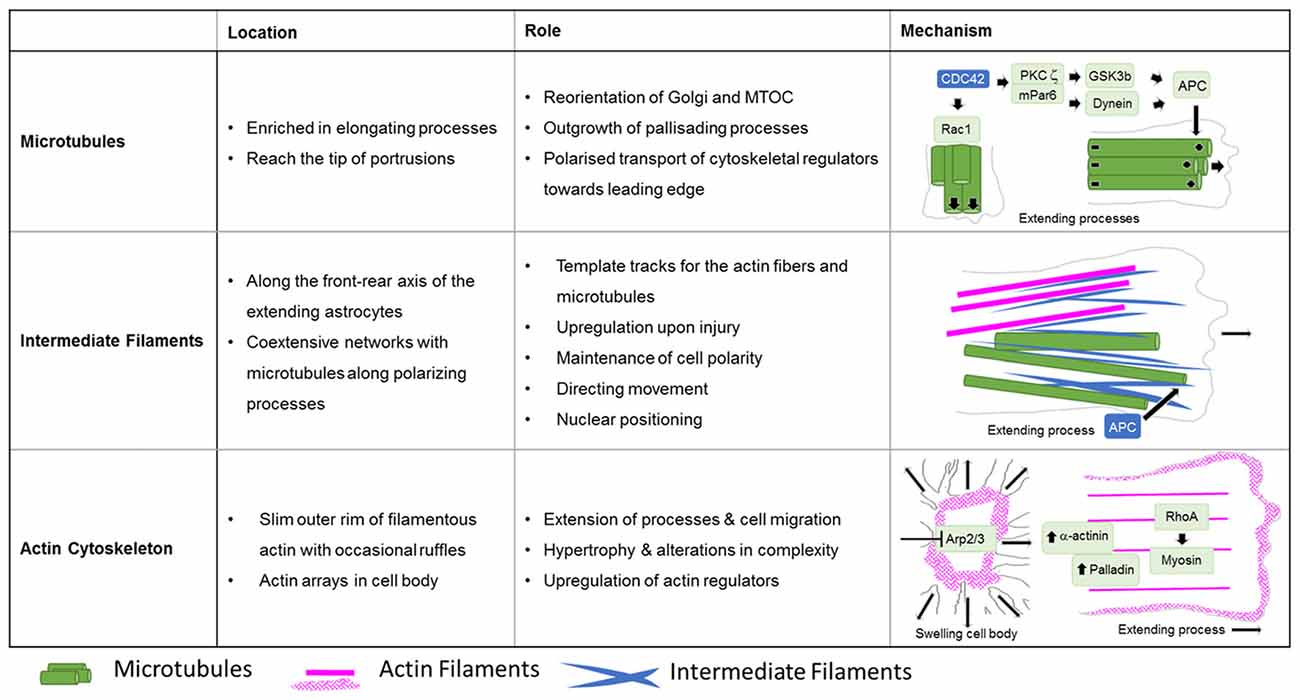
Figure 5. Roles of cytoskeletal systems during the Injury response of astrocytes in vitro. Summary in view of the localization and roles of microtubules, intermediate and actin filaments in cultured astrocytes upon injury. The schematic drawings depict the cytoskeletal systems and their major regulators involved in extension of astrocyte processes and cell body hypertrophy.
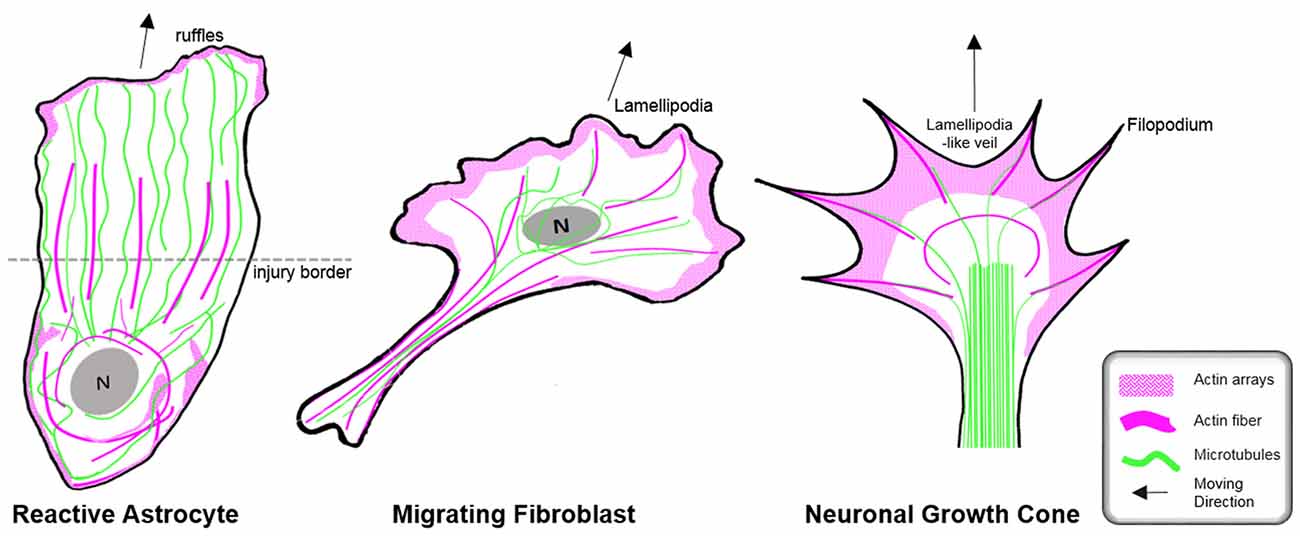
Figure 6. Organization of the cytoskeleton in reactive astrocytes in comparison to fibroblasts and neurons in vitro. Schematic drawings of microtubules (green) and F-actin arrays and fibers (magenta) in cultured mouse astrocytes after injury (left), migrating fibroblasts (center) and in extending axonal growth cones of developing neurons (right). The dashed line indicates the injury border and the nucleus is shown in gray (N). Elongated polygonal astrocytes at the wound edge extend into the cell-free space and have actin filaments (magenta) that are organized around the cell body and in bundles reaching into the center of the processes, while the leading edge possesses only a narrow outer rim of filamentous actin within sparse ruffles (left). Microtubules are enriched throughout the processes and reach into the extreme tip. In contrast, both fibroblasts and neuronal growth cones exhibit prominent actin arrays and fibers in the periphery of their leading edge forming filopodia, lamellipodia or lamellipodia-like veils. Most of their microtubules are present behind the actin-rich periphery, where microtubules form dense parallel bundles in growth cones. Microtubules extending into the actin-rich periphery frequently associate with actin fibers of filopodia.
A key molecule in the directed elongation of astrocyte processes is the small GTPase Cdc42, which regulates the alignment of the microtubule-organizing center and Golgi through an mPar6-PKCzeta signaling complex (Etienne-Manneville and Hall, 2001). Downstream targets of Cdc42-mPar6-PKCzeta are dynein motor proteins and inactive GSK3-beta, which induces the steering interaction of the tumor suppressor protein, adenomatous polyposis coli (APC) with the microtubule plus-ends (Figure 5; Etienne-Manneville and Hall, 2003). Cdc42 is actively delivered towards the leading edge through anterograde transport by Arf6-positive vesicles (Osmani et al., 2010). Moreover, Cdc42 controls microtubule-dependent outgrowth of astrocyte processes through Rac1 (Figure 5; Etienne-Manneville and Hall, 2001). However, inducible genetic deletion of Cdc42 in vivo does not affect the orientation of palisading astrocytes but impairs their pathology-induced proliferation in traumatic brain injuries (Table 1; Robel et al., 2011; Bardehle et al., 2013). Whether this in vivo phenotype relies on differences in microtubule regulation between genuine and cultured astrocytes or the mosaic pattern of scattered Cdc42 deficient astrocytes influenced by the majority of surrounding wildtype cells, is currently unknown. Future work will need to further study the significance of microtubules in reactive astrocytes through comprehensive studies in vivo and additional cell culture models.
Intermediate Filaments in Glial Scarring
Because of the prominent upregulation of intermediate proteins in reactive astrocytes, this filament network is traditionally seen as the most relevant cytoskeletal system in astrogliosis (Sultana et al., 2000). In contrast to during development, intermediate filaments substantially participate in astrogliosis and glial scarring, with their impact dependent on the CNS region and the type of pathology. Of note, GFAP and vimentin deficiency impairs the hypertrophy of cell bodies and main processes in reactive astrocytes. Moreover, glial scars that occur as a consequence of brain or spinal cord injuries are less dense and are frequently accompanied by significant bleeding in the central lesion sites (Table 1; Pekny et al., 1999). Deleting both intermediate proteins affects axon remodeling and motor behavioral recovery in mice after stroke and correlates with increased sensitivity of astrocytes to oxidative stress during hypoxia and subsequent reperfusion (de Pablo et al., 2013; Liu et al., 2014). Furthermore, the loss of GFAP and vimentin in a mouse model for chronic neurodegenerative Batten disease impairs the blood-brain barrier and accelerates the onset and progression of the pathology (Macauley et al., 2011). The absence of intermediate filaments also affects the interaction of astrocytes with amyloid plaques in AD mouse models, albeit without significant effect on the AD plaque load (Table 1; Kamphuis et al., 2014). However, deficiency in GFAP and vimentin may also have beneficial effects, as shown in GFAP- and vimentin-deficient mice exhibiting complete axon regeneration after induction of a sciatic nerve lesion (Table 1; Berg et al., 2013). In addition, a lack of GFAP and vimentin induces remarkable synaptic regeneration after entorhinal cortex lesions (Table 1; Wilhelmsson et al., 2004).
The fine structure of the intermediate filament network was mainly studied in culture after scratch induced-injuries, where intermediate filaments run along the cells’ front-rear polarity axis in elongating astrocytes (Sakamoto et al., 2013). Intermediate filaments play an important role in establishing and maintaining cell polarity, directing movement and control of nuclear positioning, and interacting and coordinating with other filament systems of the cytoskeleton (Dupin et al., 2011; Sakamoto et al., 2013; Leduc and Etienne-Manneville, 2017). Intermediate filaments and microtubules create coextensive networks along the elongated processes of astrocytes (Figure 5) and the arrangement of both filament types is coordinated through APC (Sakamoto et al., 2013). On the one hand, a major role of intermediate filaments may involve the creation of template tracks for microtubules to enhance persistence of cell polarity and directed movement (Figure 5; Gan et al., 2016). Alternatively, microtubules may be required to establish stabilizing intermediate filaments according to increased anterograde and reduced retrograde transport of intermediate filament subunits along microtubules in extending astrocytes (Leduc and Etienne-Manneville, 2017). Along with microtubules, intermediate filaments associate with actin filaments and control the assembly of actin fibers and orientate traction forces for direct cell movement (Figure 5; Costigliola et al., 2017; Jiu et al., 2017).
The Actin Cytoskeleton During Astrogliosis
In contrast to the comprehensive knowledge on the actin cytoskeleton in non-neuronal cells and neurons, little is currently known regarding the nature and function of the actin filament cytoskeleton in astrocytes in general (as described above) and, in particular, during astrogliosis. Available data indicate distinct differences in the actin organization of polarizing astrocytes after injury, compared to other extending or migrating cells (Figure 6; Dent et al., 2011; Steffen et al., 2017). In cell culture, protruding processes of polygonal astrocytes exhibit a slim outer rim of filamentous actin with occasional ruffles, where other growing or migrating cells such as fibroblasts and neurons possess prominent actin meshworks and fibers in their periphery and form lamellipodia and filopodia (Figure 6; Etienne-Manneville and Hall, 2001). In scar-forming astrocytes, most actin filaments are instead concentrated around the cell body. Depolymerizing actin filaments with the toxin cytochalasin D seems to have little effect on the formation of long processes in polygonal astrocytes but blocks their artificial migration in scratch wound assays (Etienne-Manneville and Hall, 2001). In contrast, astrocytes with STAT3-dependent defects in reactivity and process elongation also present defects in actin-dependent focal adhesion disassembly and have substantially enhanced actomyosin tonus, while the organization of microtubules is unaffected (Table 1; Renault-Mihara et al., 2017). Moreover, astrocytes do respond to injury in vitro and in vivo by a drastic upregulation of the actin regulators α-actinin and its ligand palladin, which are known to reorganize actin filaments through crosslinking and bundling (Table 1; Abd-El-Basset and Fedoroff, 1997; Boukhelifa et al., 2003). The particular injury-specific increase and accumulation of actin regulators in scar-forming astrocytes imply a currently unknown contribution of the actin cytoskeleton to glial scarring.
Although the relevance of actin dynamics in palisading astrocytes is not clear, studies in astrocytes in culture and in tissues indicate a role of the actin cytoskeleton in controlling hypertrophy and alterations in astrocyte complexity. Acute inhibition of the Arp2/3 complex in brain slices increases the astrocyte cell body size and abundance of large processes analogous to reactive astrocytes in diffuse trauma. Moreover, inactivating the Arp2/3 complex accelerates the hypertrophy of stellate astrocytes in the oxygen/glucose-deprivation model of stroke, whereas overactivating Arp2/3 by depleting its endogenous inhibitor PICK1 or overexpressing the activator N-WASP suppresses cell body expansion (Figure 5; Murk et al., 2013). This is in line with other studies indicating a switch from Rac1 and Arp2/3-dependent networks towards actin organization in reactive astrocytes relying on RhoA and linear actin filaments in association with myosin (John, 2004; Renault-Mihara et al., 2017). However, the expansion of astrocyte cell bodies and main processes upon Arp2/3 inhibition is another indication of distinct actin organization in astrocytes compared to other cells, where the Arp2/3 inactivation instead leads to shrinkage, collapse or inhibited outgrowth (Figure 6; Korobova and Svitkina, 2008; Wu et al., 2012).
Concluding Remarks
The morphological features of astrocytes are integral to the normal shape and functioning of the CNS from late embryonic development throughout all stages of an organism’s life. Despite their critical relevance in both normal and disease states, the molecular mechanisms behind the plastic morphology of astrocytes are poorly understood. Nonetheless, improvements in cell culture methods and the development of new tools and elaboration of sophisticated microscopy techniques will allow observation and manipulation of more authentic astrocyte settings. In this context, nanoscopic PAP-synapse interactions are becoming increasingly accessible with super-resolution light microscopy, and as such, provide an appealing experimental alternative to sophisticated electron microscopy (Heller et al., 2017). Recently introduced astrocyte-specific and inducible gene targeting models will allow the functional characterization of astrocytes throughout the entire CNS without undesired collateral damage in neurogenic radial glia cells (Srinivasan et al., 2016; Winchenbach et al., 2016). Finally, human-specific and disease-relevant characteristics of astrocytes, which are associated with abnormal morphologies and functions (Windrem et al., 2017) can now be studied in vivo. The substitution of murine astrocytes through the implantation of glial progenitors derived from human-iPSC makes it possible to investigate the specific role and contribution of abnormal astrocytes in diverse pathologies. A better understanding of the plasticity of astrocyte morphology and function will help us to gain insight into the fundamental properties of this profound shapeshifter in both normal cells and diverse human pathologies.
Author Contributions
JS, BJE and KM wrote the manuscript. JS and KM created the figures.
Funding
We acknowledge support from the German Research Foundation (DFG) and the Open Access Publication Fund of Charité—Universitätsmedizin Berlin.
Conflict of Interest Statement
The authors declare that the research was conducted in the absence of any commercial or financial relationships that could be construed as a potential conflict of interest.
Acknowledgments
This article relies on a wealth of data from many different laboratories. We have cited the appropriate original publications wherever possible, and we apologize to all researchers whose work on astrocytes was not included in this article.
References
Abd-El-Basset, E. M., and Fedoroff, S. (1997). Upregulation of F-actin and α-actinin in reactive astrocytes. J. Neurosci. Res. 49, 608–616. doi: 10.1002/(sici)1097-4547(19970901)49:5<608::aid-jnr11>3.0.co;2-r
Allen, N. J., Bennett, M. L., Foo, L. C., Wang, G. X., Smith, S. J., and Barres, B. A. (2012). Astrocyte glypicans 4 and 6 promote formation of excitatory synapses via GluA1 AMPA receptors. Nature 486, 410–414. doi: 10.1038/nature11059
Anderson, M. A., Burda, J. E., Ren, Y., Ao, Y., O’Shea, T. M., Kawaguchi, R., et al. (2016). Astrocyte scar formation aids central nervous system axon regeneration. Nature 532, 195–200. doi: 10.1038/nature17623
Araque, A., Parpura, V., Sanzgir, R. P., and Haydon, P. G. (1999). Tripartite synapses: glia, the unacknowledged partner. Trends Neurosci. 22, 208–215. doi: 10.1016/s0166-2236(98)01349-6
Bandeira, F., Lent, R., and Herculano-Houzel, S. (2009). Changing numbers of neuronal and non-neuronal cells underlie postnatal brain growth in the rat. Proc. Natl. Acad. Sci. U S A 106, 14108–14113. doi: 10.1073/pnas.0804650106
Bardehle, S., Krüger, M., Buggenthin, F., Schwausch, J., Ninkovic, J., Clevers, H., et al. (2013). Live imaging of astrocyte responses to acute injury reveals selective juxtavascular proliferation. Nat. Neurosci. 16, 580–586. doi: 10.1038/nn.3371
Bellesi, M., de Vivo, L., Chini, M., Gilli, F., Tononi, G., and Cirelli, C. (2017). Sleep loss promotes astrocytic phagocytosis and microglial activation in mouse cerebral cortex. J. Neurosci. 37, 5263–5273. doi: 10.1523/JNEUROSCI.3981-16.2017
Ben Haim, L., Carrillo-de Sauvage, M.-A., Ceyzériat riat, K., and Escartin, C. (2015). Elusive roles for reactive astrocytes in neurodegenerative diseases. Front. Cell. Neurosci. 9:278. doi: 10.3389/fncel.2015.00278
Benediktsson, A. M., Schachtele, S. J., Green, S. H., and Dailey, M. E. (2005). Ballistic labeling and dynamic imaging of astrocytes in organotypic hippocampal slice cultures. J. Neurosci. Methods 141, 41–53. doi: 10.1016/j.jneumeth.2004.05.013
Berg, A., Zelano, J., Pekna, M., Wilhelmsson, U., Pekny, M., and Cullheim, S. (2013). Axonal regeneration after sciatic nerve lesion is delayed but complete in GFAP- and vimentin-deficient mice. PLoS One 8:e79395. doi: 10.1371/journal.pone.0079395
Bernardinelli, Y., Randall, J., Janett, E., Nikonenko, I., König, S., Jones, E. V., et al. (2014). Activity-dependent structural plasticity of perisynaptic astrocytic domains promotes excitatory synapse stability. Curr. Biol. 24, 1679–1688. doi: 10.1016/j.cub.2014.06.025
Boukhelifa, M., Hwang, S. J., Valtschanoff, J. G., Meeker, R. B., Rustioni, A., and Otey, C. A. (2003). A critical role for palladin in astrocyte morphology and response to injury. Mol. Cell. Neurosci. 23, 661–668. doi: 10.1016/s1044-7431(03)00127-1
Burda, J. E., Bernstein, A. M., and Sofroniew, M. V. (2016). Astrocyte roles in traumatic brain injury. Exp. Neurol. 275, 305–315. doi: 10.1016/j.expneurol.2015.03.020
Burgos, M., Calvo, S., Molina, F., Vaquero, C. F., Samarel, A., Llopis, J., et al. (2007). PKCε induces astrocyte stellation by modulating multiple cytoskeletal proteins and interacting with Rho a signalling pathways: implications for neuroinflammation. Eur. J. Neurosci. 25, 1069–1078. doi: 10.1111/j.1460-9568.2007.05364.x
Bushong, E. A., Martone, M. E., and Ellisman, M. H. (2004). Maturation of astrocyte morphology and the establishment of astrocyte domains during postnatal hippocampal development. Int. J. Dev. Neurosci. 22, 73–86. doi: 10.1016/j.ijdevneu.2003.12.008
Bushong, E. A., Martone, M. E., Jones, Y. Z., and Ellisman, M. H. (2002). Protoplasmic astrocytes in CA1 stratum radiatum occupy separate anatomical domains. J. Neurosci. 22, 183–192. doi: 10.1523/JNEUROSCI.22-01-00183.2002
Butkevich, E., Hülsmann, S., Wenzel, D., Shirao, T., Duden, R., and Majoul, I. (2004). Drebrin is a novel connexin-43 binding partner that links gap junctions to the submembrane cytoskeleton. Curr. Biol. 14, 650–658. doi: 10.1016/j.cub.2004.03.063
Cao, X., Li, L. P., Wang, Q., Wu, Q., Hu, H. H., Zhang, M., et al. (2013). Astrocyte-derived ATP modulates depressive-like behaviors. Nat. Med. 19, 773–777. doi: 10.1038/nm.3162
Carmona, M. A., Murai, K. K., Wang, L., Roberts, A. J., and Pasquale, E. B. (2009). Glial ephrin-A3 regulates hippocampal dendritic spine morphology and glutamate transport. Proc. Natl. Acad. Sci. U S A 106, 12524–12529. doi: 10.1073/pnas.0903328106
Carter, S. F., Scholl, M., Almkvist, O., Wall, A., Engler, H., Langstrom, B., et al. (2012). Evidence for astrocytosis in prodromal Alzheimer disease provided by 11c-deuterium-l-deprenyl: a multitracer PET paradigm combining 11C-pittsburgh compound B and 18F-FDG. J. Nucl. Med. 53, 37–46. doi: 10.2967/jnumed.110.087031
Chaudhry, F. A., Lehre, K. P., van Lookeren Campagne, M., Ottersen, O. P., Danbolt, N. C., and Storm-Mathisen, J. (1995). Glutamate transporters in glial plasma membranes: highly differentiated localizations revealed by quantitative ultrastructural immunocytochemistry. Neuron 15, 711–720. doi: 10.1016/0896-6273(95)90158-2
Christopherson, K. S., Ullian, E. M., Stokes, C. C. A., Mullowney, C. E., Hell, J. W., Agah, A., et al. (2005). Thrombospondins are astrocyte-secreted proteins that promote CNS synaptogenesis. Cell 120, 421–433. doi: 10.1016/j.cell.2004.12.020
Chung, W. S., Clarke, L. E., Wang, G. X., Stafford, B. K., Sher, A., Chakraborty, C., et al. (2013). Astrocytes mediate synapse elimination through MEGF10 and MERTK pathways. Nature 504, 394–400. doi: 10.1038/nature12776
Colombo, J. A., Quinn, B., and Puissant, V. (2002). Disruption of astroglial interlaminar processes in Alzheimer’s disease. Brain Res. Bull. 58, 235–242. doi: 10.1016/s0361-9230(02)00785-2
Costa, M. R., Bucholz, O., Schroeder, T., and Götz, M. (2009). Late origin of glia-restricted progenitors in the developing mouse cerebral cortex. Cereb. Cortex 19, i135–i143. doi: 10.1093/cercor/bhp046
Costigliola, N., Ding, L., Burckhardt, C. J., Han, S. J., Gutierrez, E., Mota, A., et al. (2017). Vimentin fibers orient traction stress. Proc. Natl. Acad. Sci. U S A 114, 5195–5200. doi: 10.1073/pnas.1614610114
Cregg, J. M., DePaul, M. A., Filous, A. R., Lang, B. T., Tran, A., and Silver, J. (2014). Functional regeneration beyond the glial scar. Exp. Neurol. 253, 197–207. doi: 10.1016/j.expneurol.2013.12.024
Daneman, R., and Prat, A. (2015). The blood-brain barrier. Cold Spring Harb. Perspect. Biol. 7:a020412. doi: 10.1101/cshperspect.a020412
de Pablo, Y., Nilsson, M., Pekna, M., and Pekny, M. (2013). Intermediate filaments are important for astrocyte response to oxidative stress induced by oxygen-glucose deprivation and reperfusion. Histochem. Cell Biol. 140, 81–91. doi: 10.1007/s00418-013-1110-0
Dent, E. W., Gupton, S. L., and Gertler, F. B. (2011). The growth cone cytoskeleton in axon outgrowth and guidance. Cold Spring Harb. Perspect. Biol. 3:a001800. doi: 10.1101/cshperspect.a001800
Derouiche, A., and Frotscher, M. (2001). Peripheral astrocyte processes: monitoring by selective immunostaining for the actin-binding ERM proteins. Glia 36, 330–341. doi: 10.1002/glia.1120
Dey, N., Crosswell, H. E., Pradip, D., Parsons, R., Peng, Q., Jing, D. S., et al. (2008). The protein phosphatase activity of PTEN regulates Src family kinases and controls glioma migration. Cancer Res. 68, 1862–1871. doi: 10.1158/0008-5472.can-07-1182
Dupin, I., Sakamoto, Y., and Etienne-Manneville, S. (2011). Cytoplasmic intermediate filaments mediate actin-driven positioning of the nucleus. J. Cell Sci. 124, 865–872. doi: 10.1242/jcs.076356
Eom, T. Y., Stanco, A., Weimer, J., Stabingas, K., Sibrack, E., Gukassyan, V., et al. (2011). Direct visualization of microtubules using a genetic tool to analyse radial progenitor-astrocyte continuum in brain. Nat. Commun. 2:446. doi: 10.1038/ncomms1460
Etienne-Manneville, S. (2006). In vitro assay of primary astrocyte migration as a tool to study Rho GTPase function in cell polarization. Methods Enzymol. 406, 565–578. doi: 10.1016/s0076-6879(06)06044-7
Etienne-Manneville, S., and Hall, A. (2001). Integrin-mediated activation of Cdc42 controls cell polarity in migrating astrocytes through PKCζ. Cell 106, 489–498. doi: 10.1016/s0092-8674(01)00471-8
Etienne-Manneville, S., and Hall, A. (2003). Cdc42 regulates GSK-3β and adenomatous polyposis coli to control cell polarity. Nature 421, 753–756. doi: 10.1038/nature01423
Farmer, W. T., Abrahamsson, T., Chierzi, S., Lui, C., Zaelzer, C., Jones, E. V., et al. (2016). Neurons diversify astrocytes in the adult brain through sonic hedgehog signaling. Science 351, 849–854. doi: 10.1126/science.aab3103
Faulkner, J. R., Herrmann, J. E., Woo, M. J., Tansey, K. E., Doan, N. B., and Sofroniew, M. V. (2004). Reactive astrocytes protect tissue and preserve function after spinal cord injury. J. Neurosci. 24, 2143–2155. doi: 10.1523/JNEUROSCI.3547-03.2004
Filosa, A., Paixão, S., Honsek, S. D., Carmona, M. A., Becker, L., Feddersen, B., et al. (2014). Neuron-glia communication via EphA4/ephrinA3 modulates LTP through glial glutamate transport. Nat. Neurosci. 12, 1285–1292. doi: 10.1038/nn.2394
Foo, L. C., Allen, N. J., Bushong, E. A., Ventura, P. B., Chung, W., Zhou, L., et al. (2012). Development of a novel method for the purification and culture of rodent astrocytes. Neuron 71, 799–811. doi: 10.1016/j.neuron.2011.07.022
Fraser, M. M., Zhu, X., Kwon, C. H., Uhlmann, E. J., Gutmann, D. H., and Baker, S. J. (2004). Pten loss causes hypertrophy and increased proliferation of astrocytes in vivo. Cancer Res. 64, 7773–7779. doi: 10.1158/0008-5472.can-04-2487
Gan, Z., Ding, L., Burckhardt, C. J., Lowery, J., Zaritsky, A., Sitterley, K., et al. (2016). Vimentin intermediate filaments template microtubule networks to enhance persistence in cell polarity and directed migration. Cell Syst. 3, 252.e8–263.e8. doi: 10.1016/j.cels.2016.08.007
Gao, P., Postiglione, M. P., Krieger, T. G., Hernandez, L., Wang, C., Han, Z., et al. (2014). Deterministic progenitor behavior and unitary production of neurons in the neocortex. Cell 159, 775–788. doi: 10.1016/j.cell.2014.10.027
Garrett, A. M., and Weiner, J. A. (2009). Control of CNS synapse development by γ-protocadherin-mediated astrocyte-neuron contact. J. Neurosci. 29, 11723–11731. doi: 10.1523/JNEUROSCI.2818-09.2009
Ge, W. P., Miyawaki, A., Gage, F. H., Jan, Y. N., and Jan, L. Y. (2012). Local generation of glia is a major astrocyte source in postnatal cortex. Nature 484, 376–380. doi: 10.1038/nature10959
Goetschy, J. F., Ulrich, G., Aunis, D., and Ciesielski-Treska, J. (1986). The organization and solubility properties of intermediate filaments and microtubules of cortical astrocytes in culture. J. Neurocytol. 15, 375–387. doi: 10.1007/bf01611439
Han, X., Chen, M., Wang, F., Windrem, M., and Wang, S. (2013). Forebrain engraftment by human glial progenitor cells enhances synaptic plasticity and learning in adult mice. Cell Stem Cell 12, 342–353. doi: 10.1016/j.stem.2012.12.015
Haseleu, J., Anlauf, E., Blaess, S., Endl, E., and Derouiche, A. (2013). Studying subcellular detail in fixed astrocytes: dissociation of morphologically intact glial cells (DIMIGs). Front. Cell. Neurosci. 7:54. doi: 10.3389/fncel.2013.00054
Heller, J. P., Michaluk, P., Sugao, K., and Rusakov, D. A. (2017). Probing nano-organization of astroglia with multi-color super-resolution microscopy. J. Neurosci. Res. 95, 2159–2171. doi: 10.1002/jnr.24026
Heneka, M. T., Sastre, M., Dumitrescu-Ozimek, L., Dewachter, I., Walter, J., Klockgether, T., et al. (2005). Focal glial activation coincides with increased BACE1 activation and precedes amyloid plaque deposition in APP[V717I] transgenic mice. J. Neuroinflammation 2:22. doi: 10.1186/1742-2094-2-22
Hermosilla, T., Muñoz, D., Herrera-molina, R., Valdivia, A., Muñoz, N., Nham, S., et al. (2008). Direct Thy-1/αVβ3 integrin interaction mediates neuron to astrocyte communication. Biochim. Biophys. Acta 1783, 1111–1120. doi: 10.1016/j.bbamcr.2008.01.034
Higashi, K., Fujita, A., Inanobe, A., Tanemoto, M., Doi, K., Kubo, T., et al. (2001). An inwardly rectifying K+ channel, Kir4.1, expressed in astrocytes surrounds synapses and blood vessels in brain. Am. J. Physiol. Physiol. 281, C922–C931. doi: 10.1152/ajpcell.2001.281.3.c922
Higginson, J. R., and Winder, S. J. (2005). Dystroglycan: a multifunctional adaptor protein. Biochem. Soc. Trans. 33, 1254–1255. doi: 10.1042/BST20051254
Hirabayashi, Y., and Gotoh, Y. (2005). Stage-dependent fate determination of neural precursor cells in mouse forebrain. Neurosci. Res. 51, 331–336. doi: 10.1016/j.neures.2005.01.004
Hirrlinger, J., Hülsmann, S., and Kirchhoff, F. (2004). Astroglial processes show spontaneous motility at active synaptic terminals in situ. Eur. J. Neurosci. 20, 2235–2239. doi: 10.1111/j.1460-9568.2004.03689.x
Hochstim, C., Deneen, B., Lukaszewicz, A., Zhou, Q., and Anderson, D. J. (2008). Identification of positionally distinct astrocyte subtypes whose identities are specified by a homeodomain code. Cell 133, 510–522. doi: 10.1016/j.cell.2008.02.046
Hoelzinger, D. B., Demuth, T., and Berens, M. E. (2007). Autocrine factors that sustain glioma invasion and paracrine biology in the brain microenvironment. J. Natl. Cancer Inst. 99, 1583–1593. doi: 10.1093/jnci/djm187
Jacobsen, C. T., and Miller, R. H. (2003). Control of astrocyte migration in the developing cerebral cortex. Dev. Neurosci. 25, 207–216. doi: 10.1159/000072269
Jiu, Y., Peränen, J., Schaible, N., Cheng, F., Eriksson, J. E., Krishnan, R., et al. (2017). Vimentin intermediate filaments control actin stress fiber assembly through GEF-H1 and RhoA. J. Cell Sci. 130, 892–902. doi: 10.1242/jcs.196881
Jockusch, B. M., Murk, K., and Rothkegel, M. (2007). The profile of profilins. Rev. Physiol. Biochem. Pharmacol. 159, 131–149. doi: 10.1007/112_2007_704
John, G. R. (2004). Interleukin-1 induces a reactive astroglial phenotype via deactivation of the Rho GTPase-rock axis. J. Neurosci. 24, 2837–2845. doi: 10.1523/JNEUROSCI.4789-03.2004
Kamphuis, W., Middeldorp, J., Kooijman, L., Sluijs, J. A., Kooi, E. J., Moeton, M., et al. (2014). Glial fibrillary acidic protein isoform expression in plaque related astrogliosis in Alzheimer’s disease. Neurobiol. Aging 35, 492–510. doi: 10.1016/j.neurobiolaging.2013.09.035
Kanemaru, K., Kubota, J., Sekiya, H., Hirose, K., Okubo, Y., and Iino, M. (2013). Calcium-dependent N-cadherin up-regulation mediates reactive astrogliosis and neuroprotection after brain injury. Proc. Natl. Acad. Sci. U S A 110, 11612–11617. doi: 10.1073/pnas.1300378110
Kang, W., Balordi, F., Su, N., Chen, L., Fishell, G., and Hebert, J. M. (2014). Astrocyte activation is suppressed in both normal and injured brain by FGF signaling. Proc. Natl. Acad. Sci. U S A 111, E2987–E2995. doi: 10.1073/pnas.1320401111
Kanski, R., van Strien, M. E., van Tijn, P., and Hol, E. M. (2014). A star is born: new insights into the mechanism of astrogenesis. Cell. Mol. Life Sci. 71, 433–447. doi: 10.1007/s00018-013-1435-9
Keeler, A. B., Molumby, M. J., and Weiner, J. A. (2015). Protocadherins branch out: multiple roles in dendrite development. Cell Adhes. Migr. 9, 214–226. doi: 10.1080/19336918.2014.1000069
Kimelberg, H. K. (2004). The problem of astrocyte identity. Neurochem. Int. 45, 191–202. doi: 10.1016/s0197-0186(03)00286-9
Kobayashi, M., Debold, E. P., Turner, M. A., and Kobayashi, T. (2013). Cardiac muscle activation blunted by a mutation to the regulatory component, troponin T. J. Biol. Chem. 288, 26335–26349. doi: 10.1074/jbc.M113.494096
Korobova, F., and Svitkina, T. (2008). Arp2/3 complex is important for filopodia formation, growth cone motility, and neuritogenesis in neuronal cells. Mol. Biol. Cell 19, 1561–1574. doi: 10.1091/mbc.E07-09-0964
Kreis, P., Leondaritis, G., Lieberam, I., and Eickholt, B. J. (2014). Subcellular targeting and dynamic regulation of PTEN: implications for neuronal cells and neurological disorders. Front. Mol. Neurosci. 7:23. doi: 10.3389/fnmol.2014.00023
Kucukdereli, H., Allen, N. J., Lee, A. T., Feng, A., Ozlu, M. I., Conatser, L. M., et al. (2011). Control of excitatory CNS synaptogenesis by astrocyte-secreted proteins Hevin and SPARC. Proc. Natl. Acad. Sci. U S A 108, E440–E449. doi: 10.1073/pnas.1104977108
Kulijewicz-Nawrot, M., Verkhratsky, A., Chvátal, A., Syková, E., and Rodríguez, J. J. (2012). Astrocytic cytoskeletal atrophy in the medial prefrontal cortex of a triple transgenic mouse model of Alzheimer’s disease. J. Anat. 221, 252–262. doi: 10.1111/j.1469-7580.2012.01536.x
Lau, C. L., Kovacevic, M., Tingleff, T. S., Forsythe, J. S., Cate, H. S., Merlo, D., et al. (2014). 3D Electrospun scaffolds promote a cytotrophic phenotype of cultured primary astrocytes. J. Neurochem. 130, 215–226. doi: 10.1111/jnc.12702
Lavialle, M., Aumann, G., Anlauf, E., Pröls, F., Arpin, M., and Derouiche, A. (2011). Structural plasticity of perisynaptic astrocyte processes involves ezrin and metabotropic glutamate receptors. Proc. Natl. Acad. Sci. U S A 108, 12915–12919. doi: 10.1073/pnas.1100957108
Le, D. M., Besson, A., Fogg, D. K., Choi, K. S., Waisman, D. M., Goodyer, C. G., et al. (2003). Exploitation of astrocytes by glioma cells to facilitate invasiveness: a mechanism involving matrix metalloproteinase-2 and the urokinase-type plasminogen activator-plasmin cascade. J. Neurosci. 23, 4034–4043. doi: 10.1523/JNEUROSCI.23-10-04034.2003
Leduc, C., and Etienne-Manneville, S. (2017). Regulation of microtubule-associated motors drives intermediate filament network polarization. J. Cell Biol. 216, 1689–1703. doi: 10.1083/jcb.201607045
Lee, J., Lund-Smith, C., Borboa, A., Gonzalez, A. M., Baird, A., and Eliceiri, B. P. (2009). Glioma-induced remodeling of the neurovascular unit. Brain Res. 1288, 125–134. doi: 10.1016/j.brainres.2009.06.095
Liu, Z., Li, Y., Cui, Y., Roberts, C., Lu, M., Wilhelmsson, U., et al. (2014). Beneficial effects of gfap/vimentin reactive astrocytes for axonal remodeling and motor behavioral recovery in mice after stroke. Glia 62, 2022–2033. doi: 10.1002/glia.22723
Macauley, S. L., Pekny, M., and Sands, M. S. (2011). The role of attenuated astrocyte activation in infantile neuronal ceroid lipofuscinosis. J. Neurosci. 31, 15575–15585. doi: 10.1523/JNEUROSCI.3579-11.2011
MacVicar, B. A., and Newman, E. A. (2015). Astrocyte regulation of blood flow in the brain. Cold Spring Harb. Perspect. Biol. 7:a020388. doi: 10.1101/cshperspect.a020388
Magdalena, J., Millard, T. H., and Machesky, L. M. (2003). Microtubule involvement in NIH 3T3 Golgi and MTOC polarity establishment. J. Cell Sci. 116, 743–756. doi: 10.1242/jcs.00288
Martin-Fernandez, M., Jamison, S., Robin, L. M., Zhao, Z., Martin, E. D., Aguilar, J., et al. (2017). Synapse-specific astrocyte gating of amygdala-related behavior. Nat. Neurosci. 20, 1540–1548. doi: 10.1038/nn.4649
Masahira, N., Takebayashi, H., Ono, K., Watanabe, K., Ding, L., Furusho, M., et al. (2006). Olig2-positive progenitors in the embryonic spinal cord give rise not only to motoneurons and oligodendrocytes, but also to a subset of astrocytes and ependymal cells. Dev. Biol. 293, 358–369. doi: 10.1016/j.ydbio.2006.02.029
Mathiisen, T. M., Lehre, K. P., Danbolt, N. C., and Ottersen, O. P. (2010). The perivascular astroglial sheath provides a complete covering of the brain microvessels: an electron microscopic 3D reconstruction. Glia 58, 1094–1103. doi: 10.1002/glia.20990
McCarthy, K. D., and de Velllis, J. (1980). Preparation of separate astroglial and oligodendroglial cell cultures from rat cerebral tissue. J. Cell Biol. 85, 890–902. doi: 10.1083/jcb.85.3.890
Medvedev, N., Popov, V., Henneberger, C., Kraev, I., Rusakov, D. A., and Stewart, M. G. (2014). Glia selectively approach synapses on thin dendritic spines. Philos. Trans. R. Soc. Lond. B Biol. Sci. 369:20140047. doi: 10.1098/rstb.2014.0047
Michaelsen, K., Murk, K., Zagrebelsky, M., Dreznjak, A., Jockusch, B. M., Rothkegel, M., et al. (2010). Fine-tuning of neuronal architecture requires two profilin isoforms. Proc. Natl. Acad. Sci. U S A 107, 15780–15785. doi: 10.1073/pnas.1004406107
Min, R., and van der Knaap, M. S. (2018). Genetic defects disrupting glial ion and water homeostasis in the brain. Brain Pathol. 28, 372–387. doi: 10.1111/bpa.12602
Molofsky, A. V., Krenick, R., Ullian, E., Tsai, H. H., Deneen, B., Richardson, W. D., et al. (2012). Astrocytes and disease: a neurodevelopmental perspective. Genes Dev. 26, 891–907. doi: 10.1101/gad.188326.112
Molotkov, D., Zobova, S., Arcas, J. M., and Khiroug, L. (2013). Calcium-induced outgrowth of astrocytic peripheral processes requires actin binding by Profilin-1. Cell Calcium 53, 338–348. doi: 10.1016/j.ceca.2013.03.001
Molumby, M. J., Anderson, R. M., Newbold, D. J., Koblesky, N. K., Garrett, A. M., Schreiner, D., et al. (2017). γ-protocadherins interact with neuroligin-1 and negatively regulate dendritic spine morphogenesis. Cell Rep. 18, 2702–2714. doi: 10.1016/j.celrep.2017.02.060
Molumby, M. J., Keeler, A. B., and Weiner, J. A. (2016). Homophilic protocadherin cell-cell interactions promote dendrite complexity. Cell Rep. 15, 1037–1050. doi: 10.1016/j.celrep.2016.03.093
Moonen, G., Heinen, E., and Goessens, G. (1976). Comparative ultrastructural study of the effects of serum-free medium and dibutyryl-cyclic AMP on newborn rat astroblasts. Cell Tissue Res. 167, 221–227. doi: 10.1007/bf00224329
Murai, K. K., Nguyen, L. N., Irie, F., Yamaguchi, Y., and Pasquale, E. B. (2003). Control of hippocampal dendritic spine morphology through ephrin-A3/EphA4 signaling. Nat. Neurosci. 6, 153–160. doi: 10.1038/nn994
Murk, K., Blanco Suarez, E. M., Cockbill, L. M. R., Banks, P., and Hanley, J. G. (2013). The antagonistic modulation of Arp2/3 activity by N-WASP, WAVE2 and PICK1 defines dynamic changes in astrocyte morphology. J. Cell Sci. 126, 3873–3883. doi: 10.1242/jcs.125146
Myer, D. J., Gurkoff, G. G., Lee, S. M., Hovda, D. A., and Sofroniew, M. V. (2006). Essential protective roles of reactive astrocytes in traumatic brain injury. Brain 129, 2761–2772. doi: 10.1093/brain/awl165
Nagao, M., Ogata, T., Sawada, Y., and Gotoh, Y. (2016). Zbtb20 promotes astrocytogenesis during neocortical development. Nat. Commun. 7:11102. doi: 10.1038/ncomms11102
Nicchia, G. P., Rossi, A., Mola, M. G., Procino, G., Frigeri, A., and Svelto, M. (2008). Actin cytoskeleton remodeling governs aquaporin-4 localization in astrocytes. Glia 56, 1755–1766. doi: 10.1002/glia.20724
Nimmerjahn, A., Kirchhoff, F., and Helmchen, F. (2005). Neuroscience: resting microglial cells are highly dynamic surveillants of brain parenchyma in vivo. Science 308, 1314–1318. doi: 10.1126/science.1110647
Nishida, H., and Okabe, S. (2007). Direct astrocytic contacts regulate local maturation of dendritic spines. J. Neurosci. 27, 331–340. doi: 10.1523/JNEUROSCI.4466-06.2007
Oberheim, N. A., Takano, T., Han, X., He, W., Lin, J. H. C., Wang, F., et al. (2009). Uniquely hominid features of adult human astrocytes. J. Neurosci. 29, 3276–3287. doi: 10.1523/JNEUROSCI.4707-08.2009
Oberheim, N. A., Wang, X., Goldman, S., and Nedergaard, M. (2006). Astrocytic complexity distinguishes the human brain. Trends Neurosci. 29, 547–553. doi: 10.1016/j.tins.2006.08.004
Osmani, N., Peglion, F., Chavrier, P., and Etienne-Manneville, S. (2010). Cdc42 localization and cell polarity depend on membrane traffic. J. Cell Biol. 191, 1261–1269. doi: 10.1083/jcb.201003091
Panatier, A., Theodosis, D. T., Mothet, J. P., Touquet, B., Pollegioni, L., Poulain, D. A., et al. (2006). Glia-derived D-serine controls NMDA receptor activity and synaptic memory. Cell 125, 775–784. doi: 10.1016/j.cell.2006.02.051
Pannasch, U., Freche, D., Dallérac, G., Ghézali, G., Escartin, C., Ezan, P., et al. (2014). Connexin 30 sets synaptic strength by controlling astroglial synapse invasion. Nat. Neurosci. 17, 549–558. doi: 10.1038/nn.3662
Pannasch, U., Vargová, L., Reingruber, J., Ezan, P., Holcman, D., Giaume, C., et al. (2011). Astroglial networks scale synaptic activity and plasticity. Proc. Natl. Acad. Sci. U S A 108, 8467–8472. doi: 10.1073/pnas.1016650108
Pekny, M., Eliasson, C., Chien, C. L., Kindblom, L. G., Liem, R., Hamberger, A., et al. (1998). GFAP-deficient astrocytes are capable of stellation in vitro when cocultured with neurons and exhibit a reduced amount of intermediate filaments and an increased cell saturation density. Exp. Cell Res. 239, 332–343. doi: 10.1006/excr.1997.3922
Pekny, M., Johansson, C. B., Eliasson, C., Stakeberg, J., Wallén, A., Perlmann, T., et al. (1999). Abnormal reaction to central nervous system injury in mice lacking glial fibrillary acidic protein and vimentin. J. Cell Biol. 145, 503–514. doi: 10.1083/jcb.145.3.503
Pekny, M., Leveen, P., Pekna, M., Eliasson, C., Berthold, C., Westermark, B., et al. (1995). Mice lacking glial fibrillary acidic protein display astrocytes devoid of intermediate filaments but develop and reproduce normally. EMBO J. 14, 1590–1598.
Perez-Alvarez, A., Navarrete, M., Covelo, A., Martin, E. D., and Araque, A. (2014). Structural and functional plasticity of astrocyte processes and dendritic spine interactions. J. Neurosci. 34, 12738–12744. doi: 10.1523/JNEUROSCI.2401-14.2014
Peters, A., and Vaughn, J. E. (1967). Microtubules and filaments in the axons and astrocytes of early postnatal rat optic nerves. J. Cell Biol. 32, 113–119. doi: 10.1083/jcb.32.1.113
Petito, C. K., Morgello, S., Felix, J. C., and Lesser, M. L. (1990). The two patterns of reactive astrocytosis in postischemic rat brain. J. Cereb. Blood Flow Metab. 10, 850–859. doi: 10.1038/jcbfm.1990.141
Pilo Boyl, P., Di Nardo, A., Mulle, C., Sassoè-Pognetto, M., Panzanelli, P., Mele, A., et al. (2007). Profilin2 contributes to synaptic vesicle exocytosis, neuronal excitability, and novelty-seeking behavior. EMBO J. 26, 2991–3002. doi: 10.1038/sj.emboj.7601737
Potokar, M., Kreft, M., Li, L., Daniel Andersson, J., Pangršič, T., Chowdhury, H. H., et al. (2007). Cytoskeleton and vesicle mobility in astrocytes. Traffic 8, 12–20. doi: 10.1111/j.1600-0854.2006.00509.x
Procko, C., Lu, Y., and Shaham, S. (2011). Glia delimit shape changes of sensory neuron receptive endings in C. elegans. Development 138, 1371–1381. doi: 10.1242/dev.058305
Racchetti, G., D’Alessandro, R., and Meldolesi, J. (2012). Astrocyte stellation, a process dependent on Rac1 is sustained by the regulated exocytosis of enlargeosomes. Glia 60, 465–475. doi: 10.1002/glia.22280
Rakic, P. (2003). Developmental and evolutionary adaptations of cortical radial glia. Cereb. Cortex 13, 541–549. doi: 10.1093/cercor/13.6.541
Ramakers, G. J. A., and Moolenaar, W. H. (1998). Regulation of astrocyte morphology by RhoA and lysophosphatidic acid. Exp. Cell Res. 245, 252–262. doi: 10.1006/excr.1998.4224
Ramón y Cajal, S. (1913). Un nuevo proceder para la impregnación de la neuroglía. Bol. Soc. Esp. Bio. 2, 104–108.
Rash, J. E., Yasumura, T., Hudson, C. S., Agre, P., and Nielsen, S. (1998). Direct immunogold labeling of aquaporin-4 in square arrays of astrocyte and ependymocyte plasma membranes in rat brain and spinal cord. Proc. Natl. Acad. Sci. U S A 95, 11981–11986. doi: 10.1073/pnas.95.20.11981
Ren, Z., Iliff, J. J., Yang, L., Yang, J., Chen, X., Chen, M. J., et al. (2013). ‘Hit & Run’ model of closed-skull traumatic brain injury (TBI) reveals complex patterns of post-traumatic AQP4 dysregulation. J. Cereb. Blood Flow Metab. 33, 834–845. doi: 10.1038/jcbfm.2013.30
Renault-Mihara, F., Mukaino, M., Shinozaki, M., Kumamaru, H., Kawase, S., Baudoux, M., et al. (2017). Regulation of RhoA by STAT3 coordinates glial scar formation. J. Cell Biol. 216, 2533–2550. doi: 10.1083/jcb.201610102
Renner, M., Lancaster, M. A., Bian, S., Choi, H., Ku, T., Peer, A., et al. (2017). Self-organized developmental patterning and differentiation in cerebral organoids. EMBO J. 36, 1316–1329. doi: 10.15252/embj.201694700
Richier, B., Vijandi, C. D. M., Mackensen, S., and Salecker, I. (2017). Lapsyn controls branch extension and positioning of astrocyte-like glia in the Drosophila optic lobe. Nat. Commun. 8:317. doi: 10.1038/s41467-017-00384-z
Robel, S., Bardehle, S., Lepier, A., Brakebusch, C., and Götz, M. (2011). Genetic deletion of cdc42 reveals a crucial role for astrocyte recruitment to the injury site in vitro and in vivo. J. Neurosci. 31, 12471–12482. doi: 10.1523/JNEUROSCI.2696-11.2011
Robel, S., Mori, T., Zoubaa, S., Schlegel, J., Sirko, S., Faissner, A., et al. (2009). Conditional deletion of ß1-integrin in astroglia causes partial reactive gliosis. Glia 57, 1630–1647. doi: 10.1002/glia.20876
Rottner, K., Faix, J., Bogdan, S., Linder, S., and Kerkhoff, E. (2017). Actin assembly mechanisms at a glance. J. Cell Sci. 130, 3427–3435. doi: 10.1242/jcs.206433
Rotty, J. D., Wu, C., Haynes, E. M., Suarez, C., Winkelman, J. D., Johnson, H. E., et al. (2015). Profilin-1 serves as a gatekeeper for actin assembly by Arp2/3-dependent and—independent pathways. Dev. Cell 32, 54–67. doi: 10.1016/j.devcel.2014.10.026
Rubinstein, R., Thu, C. A., Goodman, K. M., Wolcott, H. N., Bahna, F., Mannepalli, S., et al. (2015). Molecular logic of neuronal self-recognition through protocadherin domain interactions. Cell 163, 629–642. doi: 10.1016/j.cell.2015.09.026
Rungger-Brändle, E., Messerli, J. M., Niemeyer, G., and Eppenberger, H. M. (1993). Confocal microscopy and computer-assisted image reconstruction of astrocytes in the mammalian retina. Eur. J. Neurosci. 5, 1093–1106. doi: 10.1111/j.1460-9568.1993.tb00963.x
Saadoun, S., Papadopoulos, M. C., Watanabe, H., Yan, D., Manley, G. T., and Verkman, A. S. (2005). Involvement of aquaporin-4 in astroglial cell migration and glial scar formation. J. Cell Sci. 118, 5691–5698. doi: 10.1242/jcs.02680
Sakamoto, Y., Boëda, B., and Etienne-Manneville, S. (2013). APC binds intermediate filaments and is required for their reorganization during cell migration. J. Cell Biol. 200, 249–258. doi: 10.1083/jcb.201206010
Sandau, U. S., Mungenast, A. E., Alderman, Z., Sardi, S. P., Fogel, A. I., Taylor, B., et al. (2011). SynCAM1, a synaptic adhesion molecule, is expressed in astrocytes and contributes to erbB4 receptor-mediated control of female sexual development. Endocrinology 152, 2364–2376. doi: 10.1210/en.2010-1435
Schober, J. M., Komarova, Y. A., Chaga, O. Y., Akhmanova, A., and Borisy, G. G. (2007). Microtubule-targeting-dependent reorganization of filopodia. J. Cell Sci. 120, 1235–1244. doi: 10.1242/jcs.003913
Scholze, A. R., Foo, L. C., Mulinyawe, S., and Barres, B. A. (2014). BMP signaling in astrocytes downregulates EGFR to modulate survival and maturation. PLoS One 9:e110668. doi: 10.1371/journal.pone.0110668
Schousboe, A., Bak, L. K., and Waagepetersen, H. S. (2013). Astrocytic control of biosynthesis and turnover of the neurotransmitters glutamate and GABA. Front. Endocrinol. 4:102. doi: 10.3389/fendo.2013.00102
Schweinhuber, S. K., Meßerschmidt, T., Hänsch, R., Korte, M., and Rothkegel, M. (2015). Profilin isoforms modulate astrocytic morphology and the motility of astrocytic processes. PLoS One 10:e0117244. doi: 10.1371/journal.pone.0117244
Shapiro, D. L. (1973). Morphological and biochemical alterations in foetal rat brain cells cultured in the presence of monobutyryl cyclic AMP. Nature 241, 203–204. doi: 10.1038/241203a0
Simard, M., and Nedergaard, M. (2004). The neurobiology of glia in the context of water and ion homeostasis. Neuroscience 129, 877–896. doi: 10.1016/j.neuroscience.2004.09.053
Singh, S. K., Fiorelli, R., Kupp, R., Rajan, S., Szeto, E., Lo Cascio, C., et al. (2016). Post-translational modifications of OLIG2 regulate glioma invasion through the TGF-β pathway. Cell Rep. 16, 950–966. doi: 10.1016/j.celrep.2016.06.045
Sofroniew, M. V. (2009). Molecular dissection of reactive astrogliosis and glial scar formation. Trends Neurosci. 32, 638–647. doi: 10.1016/j.tins.2009.08.002
Srinivasan, R., Lu, T. Y., Chai, H., Xu, J., Huang, B. S., Golshani, P., et al. (2016). New transgenic mouse lines for selectively targeting astrocytes and studying calcium signals in astrocyte processes in situ and in vivo. Neuron 92, 1181–1195. doi: 10.1016/j.neuron.2016.11.030
Steffen, A., Stradal, T. E. B., and Rottner, K. (2017). Signalling pathways controlling cellular actin organization. Handb. Exp. Pharmacol. 233, 321–353. doi: 10.1007/164_2016_35
Stellwagen, D., and Malenka, R. C. (2006). Synaptic scaling mediated by glial TNF-α. Nature 440, 1054–1059. doi: 10.1038/nature04671
Stogsdill, J. A., Ramirez, J., Liu, D., Kim, Y. H., Baldwin, K. T., Enustun, E., et al. (2017). Astrocytic neuroligins control astrocyte morphogenesis and synaptogenesis. Nature 551, 192–197. doi: 10.1038/nature24638
Stokum, J. A., Kurland, D. B., Gerzanich, V., and Simard, J. M. (2015). Mechanisms of astrocyte-mediated cerebral edema. Neurochem. Res. 40, 317–328. doi: 10.1007/s11064-014-1374-3
Stolt, C. C., Lommes, P., Sock, E., Chaboissier, M. C., Schedl, A., and Wegner, M. (2003). The Sox9 transcription factor determines glial fate choice in the developing spinal cord. Genes Dev. 17, 1677–1689. doi: 10.1101/gad.259003
Stork, T., Sheehan, A., Tasdemir-Yilmaz, O. E., and Freeman, M. R. (2014). Neuron-Glia interactions through the heartless fgf receptor signaling pathway mediate morphogenesis of Drosophila astrocytes. Neuron 83, 388–403. doi: 10.1016/j.neuron.2014.06.026
Sultana, S., Sernett, S. W., Bellin, R. M., Robson, R. M., and Skalli, O. (2000). Intermediate filament protein synemin is transiently expressed in a subset of astrocytes during development. Glia 30, 143–153. doi: 10.1002/(sici)1098-1136(200004)30:2<143::aid-glia4>3.0.co;2-z
Tan, Z., Liu, Y., Xi, W., Lou, H. F., Zhu, L., Guo, Z., et al. (2017). Glia-derived ATP inversely regulates excitability of pyramidal and CCK-positive neurons. Nat. Commun. 8:13772. doi: 10.1038/ncomms13772
Tatsumi, K., Okuda, H., Morita-Takemura, S., Tanaka, T., Isonishi, A., Shinjo, T., et al. (2016). Voluntary exercise induces astrocytic structural plasticity in the globus pallidus. Front. Cell. Neurosci. 10:165. doi: 10.3389/fncel.2016.00165
Theodosis, D. T. (2002). Oxytocin-secreting neurons: a physiological model of morphological neuronal and glial plasticity in the adult hypothalamus. Front. Neuroendocrinol. 23, 101–135. doi: 10.1006/frne.2001.0226
Theodosis, D. T., Piet, R., Poulain, D. A., and Oliet, S. H. R. (2004). Neuronal, glial and synaptic remodeling in the adult hypothalamus: functional consequences and role of cell surface and extracellular matrix adhesion molecules. Neurochem. Int. 45, 491–501. doi: 10.1016/j.neuint.2003.11.003
Tsai, H., Li, H., Fuentealba, L. C., Molofsky, A. V., Taveira-Marques, R., Zhuang, H., et al. (2012). Regional astrocyte allocation regulates CNS synaptogenesis and repair. Science 337, 358–362. doi: 10.1126/science.1222381
Van Horn, M. R., Strasser, A., Miraucourt, L. S., Pollegioni, L., and Ruthazer, E. S. (2017). The gliotransmitter d-serine promotes synapse maturation and axonal stabilization in vivo. J. Neurosci. 37, 6277–6288. doi: 10.1523/JNEUROSCI.3158-16.2017
Wanner, I. B., Anderson, M. A., Song, B., Levine, J., Fernandez, A., Gray-Thompson, Z., et al. (2013). Glial scar borders are formed by newly proliferated, elongated astrocytes that interact to corral inflammatory and fibrotic cells via STAT3-dependent mechanisms after spinal cord injury. J. Neurosci. 33, 12870–12886. doi: 10.1523/JNEUROSCI.2121-13.2013.
Warth, A., Mittelbronn, M., and Wolburg, H. (2005). Redistribution of the water channel protein aquaporin-4 and the K+ channel protein Kir4.1 differs in low- and high-grade human brain tumors. Acta Neuropathol. 109, 418–426. doi: 10.1007/s00401-005-0984-x
Watkins, S., Robel, S., Kimbrough, I. F., Robert, S. M., Ellis-Davies, G., and Sontheimer, H. (2014). Disruption of astrocyte-vascular coupling and the blood-brain barrier by invading glioma cells. Nat. Commun. 5:4196. doi: 10.1038/ncomms5196
Wilhelmsson, U., Bushong, E. A., Price, D. L., Smarr, B. L., Phung, V., Terada, M., et al. (2006). Redefining the concept of reactive astrocytes as cells that remain within their unique domains upon reaction to injury. Proc. Natl. Acad. Sci. U S A 103, 17513–17518. doi: 10.1073/pnas.0602841103
Wilhelmsson, U., Li, L., Pekna, M., Berthold, C. H., Blom, S., and Eliasson, C. (2004). Absence of glial fibrillary acidic protein and vimentin prevents hypertrophy of astrocytic processes and improves post-traumatic regeneration. J. Neurosci. 24, 5016–5021. doi: 10.1523/JNEUROSCI.0820-04.2004
Winchenbach, J., Düking, T., Berghoff, S. A., Stumpf, S. K., Hülsmann, S., Nave, K.-A., et al. (2016). Inducible targeting of CNS astrocytes in Aldh1l1-CreERT2 BAC transgenic mice. F1000Res. 5:2934. doi: 10.12688/f1000research.10509.1
Windrem, M. S., Osipovitch, M., Liu, Z., Bates, J., Chandler-Militello, D., Zou, L., et al. (2017). Human iPSC glial mouse chimeras reveal glial contributions to schizophrenia. Cell Stem Cell 21, 195.e6–208.e6. doi: 10.1016/j.stem.2017.06.012
Winkler, U., Hirrlinger, P. G., Sestu, M., Wilhelm, F., Besser, S., Zemljic-Harpf, A. E., et al. (2013). Deletion of the cell adhesion adaptor protein vinculin disturbs the localization of GFAP in Bergmann glial cells. Glia 61, 1067–1083. doi: 10.1002/glia.22495
Witcher, M. R., Kirov, S. A., and Harris, K. M. (2007). Plasticity of perisynaptic astroglia during synaptogenesis in the mature rat hippocampus. Glia 55, 13–23. doi: 10.1002/glia.20415
Wolfes, A. C., and Dean, C. (2018). Culturing in vivo-like murine astrocytes using the fast, simple, and inexpensive AWESAM protocol. J. Vis. Exp. 131:e56092. doi: 10.3791/56092
Woo, J., Im, S.-K., Chun, H., Jung, S.-Y., Oh, S.-J., Choi, N., et al. (2017). Functional characterization of resting and adenovirus-induced reactive astrocytes in three-dimensional culture. Exp. Neurobiol. 26, 158–167. doi: 10.5607/en.2017.26.3.158
Wu, C., Asokan, S. B., Berginski, M. E., Haynes, E. M., Sharpless, N. E., Griffith, J. D., et al. (2012). Arp2/3 is critical for lamellipodia and response to extracellular matrix cues but is dispensable for chemotaxis. Cell 148, 973–987. doi: 10.1016/j.cell.2011.12.034
Keywords: astrocytes, morphology, CNS, pathology, cytoskeleton, astrogliosis, brain trauma, synapse
Citation: Schiweck J, Eickholt BJ and Murk K (2018) Important Shapeshifter: Mechanisms Allowing Astrocytes to Respond to the Changing Nervous System During Development, Injury and Disease. Front. Cell. Neurosci. 12:261. doi: 10.3389/fncel.2018.00261
Received: 02 May 2018; Accepted: 31 July 2018;
Published: 21 August 2018.
Edited by:
C. Laura Sayas, Universidad de La Laguna, SpainReviewed by:
Matteo Bergami, Universitätsklinikum Köln, GermanyReno Cervo Reyes, Department of Psychiatry, University of California San Francisco, United States
Copyright © 2018 Schiweck, Eickholt and Murk. This is an open-access article distributed under the terms of the Creative Commons Attribution License (CC BY). The use, distribution or reproduction in other forums is permitted, provided the original author(s) and the copyright owner(s) are credited and that the original publication in this journal is cited, in accordance with accepted academic practice. No use, distribution or reproduction is permitted which does not comply with these terms.
*Correspondence: Kai Murk, a2FpLm11cmtAY2hhcml0ZS5kZQ==
Britta J. Eickholt, YnJpdHRhLmVpY2tob2x0QGNoYXJpdGUuZGU=