- 1Department of Psychology, University of Washington, Seattle, WA, United States
- 2Program in Neuroscience, University of Washington, Seattle, WA, United States
Adverse stress effects on the hippocampal memory system are generally thought to be due to the high level of circulating glucocorticoids directly modifying the properties of hippocampal neurons and, accordingly, the results should be reproducible with exogenous administration of cortisol in humans and corticosterone in rodents. However, glucocorticoid levels increased to other events, such as exercise and environment enrichment, do not impair but instead enhance hippocampal memory, indicating that cortisol/corticosterone are not invariant causal factors of stress. To better model the complex psychophysiological attributes of stress (i.e., aversiveness, lack of controllability, and glucose metabolism), we examined the functions of the amygdala, medial prefrontal cortex (mPFC), and corticosterone on a hippocampal-based one-trial novel object recognition (OR) memory task in rats. Specifically, animals were subjected to amygdala stimulation, mPFC inactivation, and corticosterone treatments separately or in combination during behavioral testing. Collective amygdala, mPFC, and corticosterone manipulations significantly impaired OR memory comparable to behavioral stress. By contrast, single and dual treatments failed to reliably decrease memory functioning. These results suggest that negative mnemonic impacts of uncontrollable stress involve the amalgamation of heightened amygdala and diminished mPFC activities, and elevated circulating corticosterone level.
Introduction
The hypothalamic-pituitary-adrenal (HPA) axis hormones are widely thought to play necessary and sufficient roles in producing various detrimental outcomes of uncontrollable stress (Sapolsky, 2000; McEwen, 2013). Among the brain structures, the hippocampus and its mnemonic functions are deemed particularly sensitive to stress because hippocampal cells pack high concentration of receptors for corticosteroids (glucocorticoids and mineralocorticoids), whose synthesis and secretion by the adrenal cortex are augmented by stress (McEwen and Sapolsky, 1995; Kim et al., 2015). In support of this view, human and animal studies have revealed an inverted-U functional relationship between the level of circulating glucocorticoids and the performance of declarative-explicit memory tasks (Lupien and Lepage, 2001; Kim and Diamond, 2002; Het et al., 2005; Kim et al., 2015). For example, patients with Cushing’s syndrome, a hypercortisolemia condition in which tumors affect the HPA axis, and healthy individuals who are administered high doses of cortisol have subpar performance in verbal recall tasks (Starkman et al., 1992; Newcomer et al., 1994). Similarly, rodents injected with corticosterone underperform in spatial memory tasks (de Quervain et al., 1998; Coburn-Litvak et al., 2003). Furthermore, animal studies have implicated corticosterone in altering long-term synaptic plasticity (Pavlides et al., 1996), decreasing dendritic arborization (Woolley et al., 1990; Morales-Medina et al., 2009), suppressing adult neurogenesis (Oomen et al., 2007; Brummelte and Galea, 2010), and even causing necrosis (Masters et al., 1989) in the hippocampus. These neurophysiological changes have been proposed to occur through elevated levels of corticosteroids saturating the lower-affinity Type-II glucocorticoid, as opposed to the high-affinity Type-I mineralocorticoid receptors (Pavlides et al., 1995; Yang et al., 2004; Oomen et al., 2007). Clinically, there are reports of both increased (Pitman and Orr, 1990; Lemieux and Coe, 1995; Maes et al., 1998) and decreased (King et al., 2001; Oquendo et al., 2003; Yehuda et al., 2005) levels of glucocorticoids in stress-induced psychopathologies, namely posttraumatic stress disorder (PTSD).
Although numerous studies ascribe memory-impairing effects of stress solely in relation to cortisol (in humans) and corticosterone (in rodents) levels (Starkman et al., 1992; Newcomer et al., 1994; McEwen and Sapolsky, 1995; Heinrichs et al., 1996; de Quervain et al., 1998), there is conflicting evidence that glucocorticoids alone cannot reproduce behavioral stress effects on the hippocampus. For example, exercise and environmental enrichment substantially increase corticosterone levels, but they enhance, rather than impair, hippocampal memory and neurogenesis (Kempermann et al., 2002; Hötting et al., 2016; Kim et al., 2018). Likewise, male rats exposed to receptive females show intact spatial working memory despite having significantly elevated plasma corticosterone levels equivalent to stress-induced levels (Woodson et al., 2003). Animal studies have also demonstrated a double dissociation between corticosterone and hippocampal functions. Specifically, stress continues to impair hippocampal long-term potentiation (LTP) in adrenalectomized rats depleted of corticosterone (Diamond et al., 1992), and amygdala lesion/inactivation block stress impairment of LTP and spatial memory without impeding stress enhancement of corticosterone levels (Kim et al., 2001, 2005). The medial prefrontal cortex (mPFC) has also been found to mitigate stress-induced learned helplessness via inhibiting the dorsal raphe nucleus (DRN; Amat et al., 2005) and regulate the HPA axis hormone responses to stress (Diorio et al., 1993). Correspondingly, the mPFC has been reported to be volumetrically smaller and hyporesponsive in PTSD patients (Shin et al., 2001). It appears then the cognitive-affective-arousal reactivity aspects of uncontrollable stress require a systems-level, rather than glucocorticoids-centered, analysis. Hence, the present study examined, for the first time, the ensemble functions of the amygdala (AMYG; concerned with affective responses), mPFC (implicated in top-down cognitive control), and corticosterone (CORT; indicative of heightened arousal and glucose metabolism) in generating stress effects on hippocampal-based one-trial novel object recognition (OR) memory in rats (Ennaceur and Delacour, 1988; Clark et al., 2000; Baker and Kim, 2002).
Materials and Methods
Ethics Statement
All experiments were performed in compliance with the NIH Guide for the Care and Use of Laboratory Animals and under protocols approved by the University of Washington Animal Care and Use Committee.
Subjects
Experimentally naive male Long-Evans rats (250–300 g) were individually housed in a standard polycarbonate cage, equipped with feeder and water bottle, in a climate-controlled vivarium (on a 12-h light:dark cycle, lights off at 7 AM). All test procedures were conducted during the dark phase of the cycle when rats are normally active. Animals were assigned to either SINGLE, DYAD or BEHAVIORAL STRESS treatment conditions (all within-subjects design) as detailed below.
Surgery
Animals in the SINGLE and DYAD conditions were anesthetized with ketamine HC1 (30 mg/kg) and xylazine (2.5 mg/kg), head-fixed in a stereotaxic apparatus, and implanted chronically with bipolar stainless steel wire electrodes (bare tip diameter, 0.125 mm; Plastics One) bilaterally in the basolateral nucleus of the amygdala (BLA; from Bregma: −2.8 mm posterior, 5.2 mm lateral, 8.4 mm ventral) and a dual guide cannula (1.5 mm center-to-center distance, Plastics One) in the (mPFC; from Bregma: 2.7 mm anterior, 0.5 mm lateral, 4.1 mm ventral). Animals were adapted to daily handling during the 5–7 day postoperative recovery period.
Object Recognition (OR) Apparatus
Behavioral testing took place inside a square arena (57 × 57 × 59 cm high; constructed of white fiberboard) illuminated indirectly by an incandescent lamp and with a constant white noise (60 dB) background. An ultra-digital wireless camera (LW2101; Lorex Technology Inc.,) affixed over the apparatus was connected to a Sony HD DVD recorder (RDR-HX900) and a PC (in the adjacent room) to record the animal’s behavior. The ANY-maze video tracking system (Stoelting Company) was used to capture video images and track the animal’s movement (30 frames/s). Three identical sets of different objects, made of plastic, glass, metal or wood, and varied in shape and texture were used. All animals were exposed to two different types of (familiar and novel) objects simultaneously with the order of object presentations counterbalanced. To minimize the possible spatial-location influence, the familiar and novel objects were always placed in the same two corners of the arena in a counterbalanced manner.
Procedure
The SINGLE rats underwent AMYG, mPFC, CORT (individual) and AMYG + mPFC + CORT (combined, COMB) manipulations (counterbalanced), whereas the DYAD rats underwent AMYG + mPFC, mPFC + CORT and AMYG + CORT (paired) and AMYG + mPFC + CORT (COMB) manipulations (counterbalanced). All animals were habituated to an open field chamber without any objects for 10 min per day for four consecutive days (habituation phase). Twenty-four hours after the last habituation session, animals were given 10 min to explore two identical objects placed in a familiar chamber (familiarization phase). Afterward, they received: (i) the GABA-A receptor agonist muscimol infusions into the mPFC (10 mM, 0.3 μl per side, 0.1 μl/min; see Yoon et al., 2008); (ii) an injection of CORT (3 mg/kg subcutaneous); which has been shown to increase the plasma corticosterone by four-fold (see Kim et al., 2012); (iii) electrical stimulation of the AMYG (0.5-ms pulses at 100 Hz, 60 5-s trains, 35–75-s ITIs, 100–400 μA), which produces freezing and 22-kHz ultrasonic vocalization (see Kim et al., 2012); (iv) dual combinations of i + ii, i + iii and ii + iii manipulations (DYAD condition); (v) a COMB i + ii + iii manipulation; (vi) behavioral stress (60 min restraint and tailshocks: 1 mA, 1-s, 5–115 s apart; Baker and Kim, 2002); or (vii) a homecage control (CTRL) condition. Considering the time it takes for exogenously administered CORT and muscimol to reach relatively stable levels (~30 min; Baraldi et al., 1979; Wiegert et al., 2006), mPFC muscimol and CORT drug injections were given promptly after the familiarization phase. The AMYG stimulation and STRESS treatments commenced ~30 min after the familiarization phase to match the CORT/muscimol time frame. On the next day (test phase), one object identical to the familiarization phase and the other a novel object was placed in the chamber and animals were given 5 min of exploration. After each phase, animals underwent at least two 5-min habituation phases before the next familiarization phase resumed.
Behavioral Data Collection and Analysis
A custom-written program in QBASIC was used to quantify exploratory behavior (see Baker and Kim, 2002) from the ANY-maze video playback. In brief, manual keystrokes on the computer keyboard, by a trained “blind” observer, recorded the duration and frequency of object exploration. Exploration was scored only when the rat’s head both traversed a predefined object boundary outlined on the monitor screen and was directed toward the object. Exploration was not scored when the animal climbed on top of the object or if another part of the rat’s body touched the object (see Clark et al., 2000), which the ANY-maze video tracking system cannot reliably differentiate from exploratory behavior.
Histology
At the completion of behavioral testing, marking lesions were made at the tips of stimulating electrodes (100 μA, 10 s) to verify the electrode placement. All rats were overdosed with Beuthanasia and perfused intracardially with 0.9% saline, followed by 10% buffered formalin. The brains were removed and stored in a 30% sucrose solution until they sank before slicing. Coronal sections (60 μm) were taken through the extent of the cannulae and electrode tracks, mounted on gelatinized slides, and stained with Prussian blue and cresyl violet dyes.
Statistical Analyses
Results are presented as means ± SEM. All statistical analyses were performed with SPSS (version 11.0; SPSS Inc., Chicago, IL, USA). Object exploration time data were analyzed using a paired t-test (p < 0.05, two-tailed) and discrimination index (novel object exploration time-familiar object exploration time)/(novel object exploration time + familiar object exploration time), data were analyzed using one sample t-test (p < 0.05, two-tailed). A non-parametric Wilcoxon signed ranks test was used for those exploration time data that were not normally distributed. Of 33 rats used, two animals were excluded from analyses due to tailshock delivery and video recording errors.
Results
Figure 1A shows the placements of guide cannulae and stimulating electrodes aimed at the mPFC and BLA regions, respectively. Representative visit maps recorded during test sessions are presented in Figure 1B, showing biased (CTRL; left) and unbiased (COMB; right) exploration towards the novel object location.
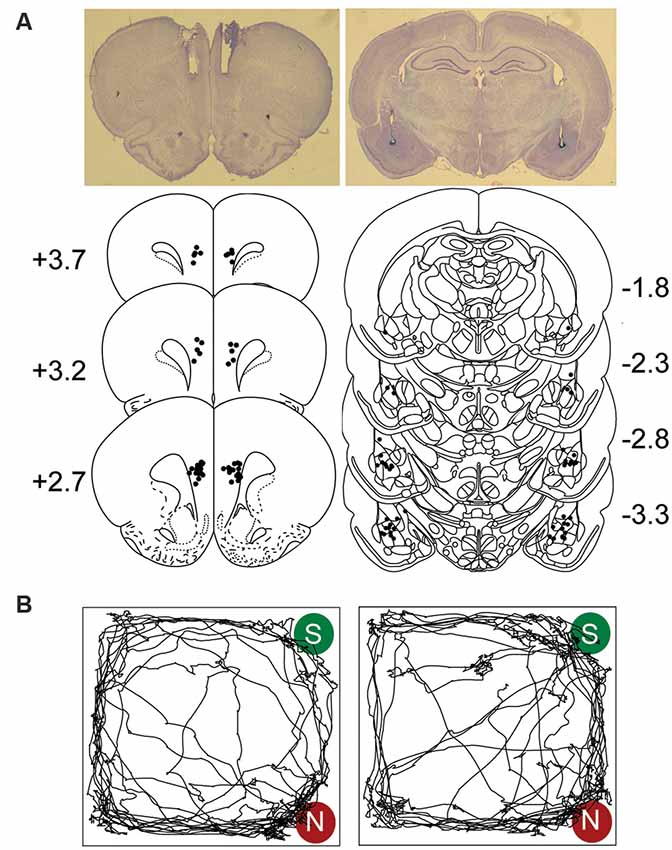
Figure 1. Electrodes and cannulae placements and track plots. (A) Photomicrograph and histological reconstruction of cannulae medial prefrontal cortex (mPFC, each dot represents the tips of the guide cannulae) and stimulating electrodes amygdala (BLA) implantations. (B) Representative visit maps from CTRL (left) and COMB treatments (right) during the sample (S) vs. novel (N) object test session. See text for object exploration criteria.
Table 1 shows the mean ± SEM object exploration time (in seconds) in the arena during the familiarization (two identical objects; 10 min) and test (familiar vs. novel objects; 5 min) phases for all animals. None of the treatments (Figures 2A, 3A, 4A) reliably altered the amounts of time exploring the two identical objects (SINGLE: t’s < 1.3, p’s > 0.2, paired t-test, Figure 2B; DYAD: t’s < 1.313, p’s > 0.225, Figure 3B; STRESS: t’s < 1.5, p’s > 0.2, paired t-test; Figure 4B). This suggests that there were no residual effects of the surgery and repeated testing (following treatments) on the animals’ sensory, motor, and motivational systems for exploring objects.
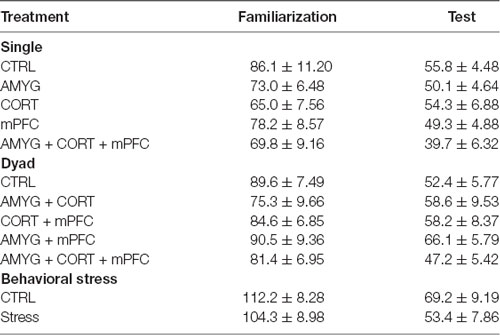
Table 1. Mean total exploration time in seconds (±SEM) animals spent exploring two objects during the familiarization and test phases.
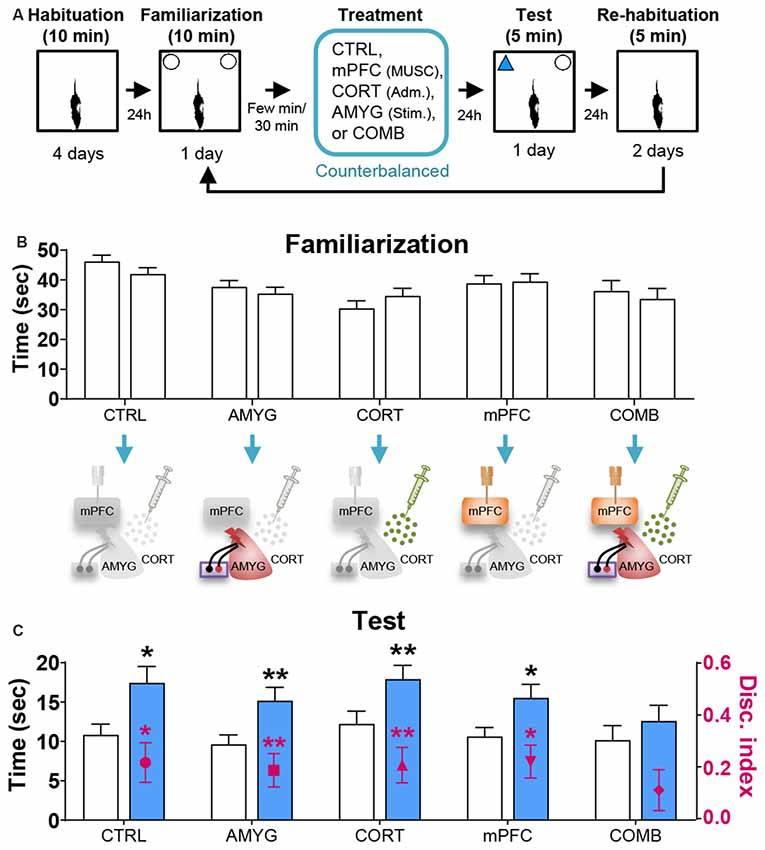
Figure 2. Effects of mPFC inactivation, CORT administration, and amygdalar stimulation on object recognition (OR) memory. (A) Behavior and treatment procedures. (B) Mean time in seconds (± SEM) that animals subjected to CTRL, AMYG, CORT, mPFC, and COMB treatments spent exploring two identical objects during the familiarization phase. (C) Mean time in seconds that different treatment animals spent exploring novel vs. familiar objects and the mean value of discrimination index during the first 2 min of the test phase. *p < 0.05 and **p < 0.01, respectively.
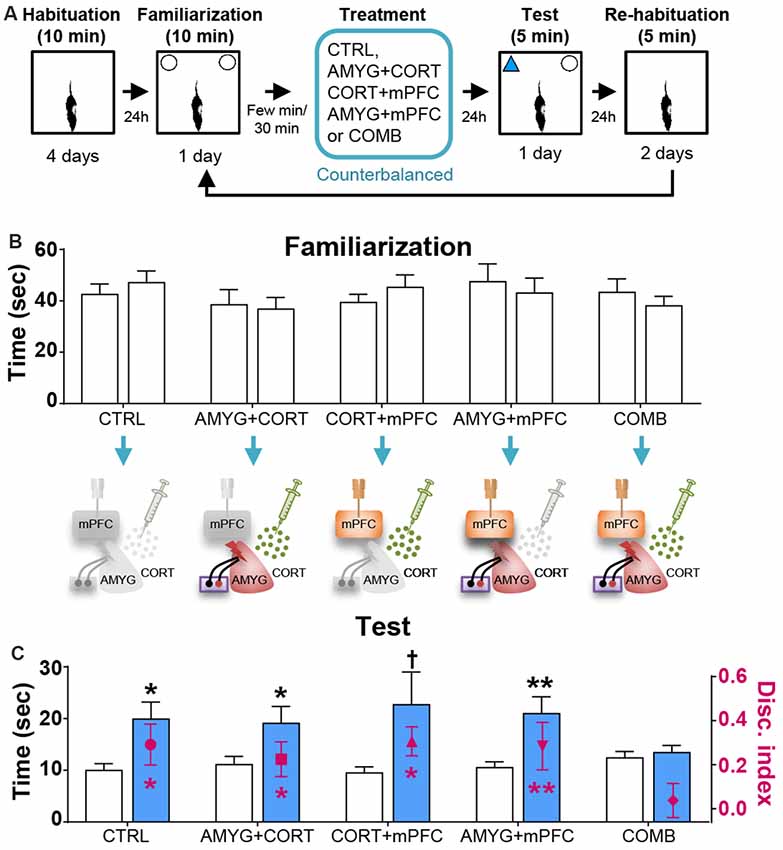
Figure 3. Effects of dyad treatments on OR memory. (A) Behavior and treatment procedures. (B) Mean time in seconds (± SEM) that CTRL, AMYG + CORT, CORT + mPFC, AMYG + mPFC, and COMB treatment animals spent exploring two identical objects during the familiarization phase. (C) Mean time in seconds that different treatment animals spent exploring novel vs. familiar objects and the mean value of discrimination index during the first 2 min of the test phase. *p < 0.05 (t-test), **p < 0.01 (t-test), and †p < 0.05 (Wilcoxon signed ranks test), respectively.
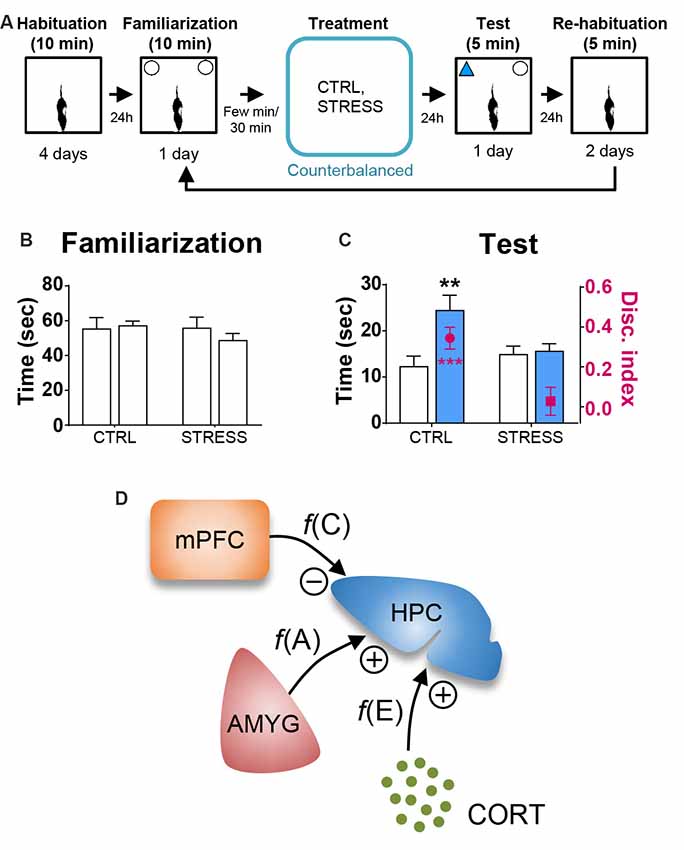
Figure 4. Behavioral stress effects on OR memory. (A) Behavioral procedure. (B) Mean time in seconds (± SEM) that CTRL and STRESS treatment animals spent exploring two identical objects during the familiarization phase. (C) Mean time in seconds that CTRL and STRESS treatment animals spent exploring novel vs. familiar objects and the mean value of discrimination index during the first 2 min of the test phase. **p < 0.01 and ***p < 0.001. (D) A systems-level model of stress comprising of CORT, AMYG and mPFC interaction. The model posits that the CORT, AMYG and mPFC mediate the functions of excitability f(E), aversiveness f(A), and controllability f(C), respectively, and that CORT and AMYG exert excitatory stress influences while mPFC exerts inhibitory stress influence on the hippocampus (HPC). Adapted from references Kim and Diamond (2002); Kim and Haller (2007) and Kim et al. (2015).
Based on the literature, the first 2 min of exploration time during the test phase, before habituation to the novel object transpired, gives a reliable measure of OR memory (Dix and Aggleton, 1999; Barker et al., 2007). We analyzed this testing period and found that the control and all individual treatment rats (in SINGLE condition) spent significantly more time exploring the novel than familiar object (CTRL, t(14) = 2.614, p < 0.05; AMYG, t(14) = 3.059, p < 0.01; CORT, t(14) = 3.004, p < 0.01; mPFC, t(14) = 2.472, p < 0.05; Figure 2C). The analysis of discrimination index yielded the same results (all t’s > 2.839, p’s < 0.05; Figure 2C). DYAD treatments also did not impair memory performance during the test phase as shown by time (CTRL, t(8) = 3.111, p < 0.05; AMYG + CORT, t(8) = 2.403, p < 0.05; CORT + mPFC, Z = 2.192, p < 0.05; AMYG + mPFC, t(8) = 3.693, p < 0.01; Figure 3C) and discrimination index (all t’s > 2.631, p’s < 0.05; Figure 3C). In both SINGLE and DYAD conditions, however, the COMB (AMYG + mPFC + CORT) treatment rats did not demonstrate a preference for the novel object over the familiar object (t’s < 1.397, p’s > 0.18, Figure 2C; t’s < 0.519, p’s > 0.61, Figure 3C). The effects of AMYG + mPFC + CORT (COMB) treatments on OR memory performance were comparable to that of uncontrollable behavioral stress. While the CTRL rats spent more time exploring the novel object than the previously explored object (time: t(6) = 5.485, p < 0.01; discrimination index: t(6) = 6.300, p < 0.001; Figure 4C), the STRESS animals did not exhibit preference for the novel object over the familiar object (time: t(6) = 0.340, p = 0.745; discrimination index: t(6) = 0.389, p = 0.711), as previously reported (Baker and Kim, 2002). These results indicate that combined, but not individual or dual, treatments of AMYG stimulation, CORT injection, and mPFC inhibition are sufficient to mimic impairing effects of stress on OR memory in naïve rats.
Discussion
In recent decades, the mainstream approaches to investigating stress effects on the brain functions have been to relate the levels and activities of particular hormones, such as glucocorticoids, from the adrenal gland (McEwen and Sapolsky, 1995), peptides, such as corticotropin-releasing factor (CRF), from the hypothalamic paraventricular nucleus (Heinrichs et al., 1995), or neurotransmitters, such as serotonin, from the DRN (Maier and Watkins, 2005), directly to stress. As these strategies are experimentally tractable to both in vitro and in vivo analyses, they have generated a wealth of information putatively in relation to stress (Schaaf et al., 2000; Groc et al., 2008). However, whether a single biochemical system can accurately reflect the multifaceted neural-cognitive-behavioral characteristics of stress needs to be logically questioned (e.g., Kim et al., 2015). Consistent with the view that no single biochemical substance responds uniquely to stress and, thus, none is likely to be a sufficient causal factor of stress, the present findings show that the systemic administration of corticosterone, which yields four-fold increases in the circulating corticosterone level (Kim et al., 2012), failed to influence 24-h delay OR memory, a putative hippocampal-dependent memory task (e.g., Clark et al., 2000; Baker and Kim, 2002; Broadbent et al., 2010; Zhao et al., 2012; Mello-Carpes and Izquierdo, 2013; but see Mumby, 2001). As alluded previously, sex, environment enrichment and exercise all significantly elevate corticosterone levels, but none have been found to impair hippocampal memory functions (for a recent review, see Kim et al., 2015). Environment enrichment and exercise, if anything, enhance dendritic arborization, synaptogenesis, and neurogenesis in the hippocampus, which are opposite effects of stress (e.g., Schoenfeld and Gould, 2012). Furthermore, if glucocorticoids are the main contributing factors in the mediation of stress effects, where low/high levels facilitate/impede hippocampal functions, then removing glucocorticoids during stress and directly applying glucocorticoids in the absence of behavioral stress should preclude and produce stress effects, respectively. However, there are several behavioral, synaptic plasticity, and neural activity data from animal studies inconsistent with this simple curvilinear chemical level-stress effect notion (Kim et al., 2015).
In the present study, the OR memory performance was also unaffected by inhibition of the mPFC, a structure implicated in the top-down controllability of stressor (Amat et al., 2005; Dalley et al., 2011) or stimulation of the amygdala, a structure concerned with affective responses. However, unlike corticosterone injections and intra-mPFC muscimol infusions, which did not elicit visible distress behaviors, the 60 min intermittent electrical stimulations of the amygdala evoked robust freezing and 22 kHz ultrasonic vocalization behaviors in rats (Kim et al., 2012). The same amygdalar stimulation was also found to alter the firing properties of the hippocampal CA1 place cells (Kim et al., 2012), akin to behavioral stress (Kim et al., 2007). This suggests that amygdalar stimulation-induced alterations of place cells are not critically connected to the OR memory functioning, at least not when the objects are placed on the constant locations in the open-field arena. In contrast to negative findings with individual/dyad corticosterone, mPFC, and amygdala manipulations, the combination of all three treatments was sufficient to impede OR memory performance, comparable to uncontrollable stress. These effects on the OR memory are unlikely due to extraneous factors, such as alterations in the motor and/or motivational systems, because the combined treatments occurred after the animals have already explored two identical objects during the familiarization phase and because any non-specific effects associated with the treatments would have dissipated by the time of the novelty preference test the next day.
The null effects of corticosterone treatment on the OR memory performance are inconsistent with the prevalent view where stress and glucocorticoids are often considered interchangeable (Sapolsky et al., 1986; de Quervain et al., 1998; McEwen, 1999; Yehuda, 2009), when the main function of glucocorticoids is to regulate glucose homeostasis not exclusive to stress but to various psychological and physical events (Nicolaides et al., 2000; Kuo et al., 2015). The present findings are instead more in line with the notion that stress involves three basic psychological factors of excitability/arousal, aversiveness, and uncontrollability, which correspond to biological substrates of elevated levels of glucocorticoids, increased activity in the amygdala, and decreased activity in the mPFC, respectively (Kim and Diamond, 2002; Kim and Haller, 2007; Kim et al., 2015; Figure 4D). These psychological-biological designations are consistent with the evidence that the HPA-axis activity correlates with excitability/arousal (de Quervain et al., 1998; Gutteling et al., 2005; Yehuda et al., 2005), amygdala inactivation/stimulation reduces/evokes aversive responses (Henke, 1990; Helmstetter, 1993; Adamec et al., 1999), and mPFC activity correlates with behavioral controllability while mPFC damage results in the loss of behavioral control (Herry and Garcia, 2002; Milad and Quirk, 2002; Izquierdo et al., 2006; Maier et al., 2006; Radley et al., 2006). The proposed stress model, based on clearly defined psychological constructs and physiologically anchored, offer predictability and testability, via manipulating corticosterone, amygdala, and mPFC in conjunction with behavioral stress. Future studies will also need to determine whether the combined treatments influence other aspects of hippocampal functions (e.g., LTP, dendritic arborization, neurogenesis), identify the specific region of the mPFC (e.g., prelimbic, infralimbic and cingulate cortices) and cell type that contribute to the stress response (e.g., via optogenetic and chemogenetic tools) and its pathway to the hippocampus (e.g., most likely indirect projections via entorhinal cortex or nucleus reuniens), and how corticosterone, amygdala and mPFC inputs integrate to affect the hippocampus. The systems-level approaches, rather than focusing on singular chemical systems, are likely to lead to a better understanding of how stress affects the brain and cognition.
Data Availability
The datasets generated for this study are available on request to the corresponding author.
Ethics Statement
The animal study was reviewed and approved by The University of Washington Animal Care and Use Committee.
Author Contributions
EK and JK designed the research, analyzed the data, wrote the manuscript and contributed to the preparation of the manuscript. EK performed research. All authors revised and approved the final version of the manuscript.
Funding
This work was supported by the National Institutes of Health (NIH) Grant MH099073 (to JK).
Conflict of Interest Statement
The authors declare that the research was conducted in the absence of any commercial or financial relationships that could be construed as a potential conflict of interest.
Acknowledgments
We thank Shannon S. Yoo for her valuable assistance on experiments and data collection/analysis.
References
Adamec, R. E., Burton, P., Shallow, T., and Budgell, J. (1999). NMDA receptors mediate lasting increases in anxiety-like behavior produced by the stress of predator exposure—implications for anxiety associated with posttraumatic stress disorder. Physiol. Behav. 65, 723–737. doi: 10.1016/s0031-9384(98)00226-1
Amat, J., Baratta, M. V., Paul, E., Bland, S. T., Watkins, L. R., and Maier, S. F. (2005). Medial prefrontal cortex determines how stressor controllability affects behavior and dorsal raphe nucleus. Nat. Neurosci. 8, 365–371. doi: 10.1038/nn1399
Baker, K. B., and Kim, J. J. (2002). Effects of stress and hippocampal NMDA receptor antagonism on recognition memory in rats. Learn. Mem. 9, 58–65. doi: 10.1101/lm.46102
Baraldi, M., Grandison, L., and Guidotti, A. (1979). Distribution and metabolism of muscimol in the brain and other tissues of the rat. Neuropharmacology 18, 57–62. doi: 10.1016/0028-3908(79)90009-1
Barker, G. R., Bird, F., Alexander, V., and Warburton, E. C. (2007). Recognition memory for objects, place, and temporal order: a disconnection analysis of the role of the medial prefrontal cortex and perirhinal cortex. J. Neurosci. 27, 2948–2957. doi: 10.1523/JNEUROSCI.5289-06.2007
Broadbent, N. J., Gaskin, S., Squire, L. R., and Clark, R. E. (2010). Object recognition memory and the rodent hippocampus. Learn. Mem. 17, 5–11. doi: 10.1101/lm.1650110
Brummelte, S., and Galea, L. A. (2010). Chronic high corticosterone reduces neurogenesis in the dentate gyrus of adult male and female rats. Neuroscience 168, 680–690. doi: 10.1016/j.neuroscience.2010.04.023
Clark, R. E., Zola, S. M., and Squire, L. R. (2000). Impaired recognition memory in rats after damage to the hippocampus. J. Neurosci. 20, 8853–8860. doi: 10.1523/JNEUROSCI.20-23-08853.2000
Coburn-Litvak, P. S., Pothakos, K., Tata, D. A., McCloskey, D. P., and Anderson, B. J. (2003). Chronic administration of corticosterone impairs spatial reference memory before spatial working memory in rats. Neurobiol. Learn. Mem. 80, 11–23. doi: 10.1016/s1074-7427(03)00019-4
Dalley, J. W., Everitt, B. J., and Robbins, T. W. (2011). Impulsivity, compulsivity, and top-down cognitive control. Neuron 69, 680–694. doi: 10.1016/j.neuron.2011.01.020
de Quervain, D. J., Roozendaal, B., and McGaugh, J. L. (1998). Stress and glucocorticoids impair retrieval of long-term spatial memory. Nature 394, 787–790. doi: 10.1038/29542
Diamond, D. M., Bennett, M. C., Fleshner, M., and Rose, G. M. (1992). Inverted-U relationship between the level of peripheral corticosterone and the magnitude of hippocampal primed burst potentiation. Hippocampus 2, 421–430. doi: 10.1002/hipo.450020409
Diorio, D., Viau, V., and Meaney, M. J. (1993). The role of the medial prefrontal cortex (cingulate gyrus) in the regulation of hypothalamic-pituitary-adrenal responses to stress. J. Neurosci. 13, 3839–3847.
Dix, S. L., and Aggleton, J. P. (1999). Extending the spontaneous preference test of recognition: evidence of object-location and object-context recognition. Behav. Brain Res. 99, 191–200. doi: 10.1016/s0166-4328(98)00079-5
Ennaceur, A., and Delacour, J. (1988). A new one-trial test for neurobiological studies of memory in rats. 1: behavioral data. Behav. Brain Res. 31, 47–59. doi: 10.1016/0166-4328(88)90157-x
Groc, L., Choquet, D., and Chaouloff, F. (2008). The stress hormone corticosterone conditions AMPAR surface trafficking and synaptic potentiation. Nat. Neurosci. 11, 868–870. doi: 10.1038/nn.2150
Gutteling, B. M., de Weerth, C., Willemsen-Swinkels, S. H., Huizink, A. C., Mulder, E. J., Visser, G. H., et al. (2005). The effects of prenatal stress on temperament and problem behavior of 27-month-old toddlers. Eur. Child Adolesc. Psychiatry 14, 41–51. doi: 10.1007/s00787-005-0435-1
Heinrichs, S. C., Menzaghi, F., Merlo Pich, E., Britton, K. T., and Koob, G. F. (1995). The role of CRF in behavioral aspects of stress. Ann. N Y Acad. Sci. 771, 92–104. doi: 10.1111/j.1749-6632.1995.tb44673.x
Heinrichs, S. C., Stenzel-Poore, M. P., Gold, L. H., Battenberg, E., Bloom, F. E., Koob, G. F., et al. (1996). Learning impairment in transgenic mice with central overexpression of corticotropin-releasing factor. Neuroscience 74, 303–311. doi: 10.1016/0306-4522(96)00140-6
Helmstetter, F. J. (1993). Stress-induced hypoalgesia and defensive freezing are attenuated by application of diazepam to the amygdala. Pharmacol. Biochem. Behav. 44, 433–438. doi: 10.1016/0091-3057(93)90487-e
Henke, P. G. (1990). Limbic system modulation of stress ulcer development. Ann. N Y Acad. Sci. 597, 201–206. doi: 10.1111/j.1749-6632.1990.tb16168.x
Herry, C., and Garcia, R. (2002). Prefrontal cortex long-term potentiation, but not long-term depression, is associated with the maintenance of extinction of learned fear in mice. J. Neurosci. 22, 577–583. doi: 10.1523/JNEUROSCI.22-02-00577.2002
Het, S., Ramlow, G., and Wolf, O. T. (2005). A meta-analytic review of the effects of acute cortisol administration on human memory. Psychoneuroendocrinology 30, 771–784. doi: 10.1016/j.psyneuen.2005.03.005
Hötting, K., Schickert, N., Kaiser, J., Röder, B., and Schmidt-Kassow, M. (2016). The effects of acute physical exercise on memory, peripheral BDNF, and cortisol in young adults. Neural Plast. 2016:6860573. doi: 10.1155/2016/6860573
Izquierdo, A., Wellman, C. L., and Holmes, A. (2006). Brief uncontrollable stress causes dendritic retraction in infralimbic cortex and resistance to fear extinction in mice. J. Neurosci. 26, 5733–5738. doi: 10.1523/JNEUROSCI.0474-06.2006
Kempermann, G., Gast, D., and Gage, F. H. (2002). Neuroplasticity in old age: sustained fivefold induction of hippocampal neurogenesis by long-term environmental enrichment. Ann. Neurol. 52, 135–143. doi: 10.1002/ana.10262
Kim, D. D., Barr, A. M., Honer, W. G., and Procyshyn, R. M. (2018). Exercise-induced hippocampal neurogenesis: 5-HT3 receptor antagonism by antipsychotics as a potential limiting factor in Schizophrenia. Mol. Psychiatry 23, 2252–2253. doi: 10.1038/s41380-018-0022-8
Kim, J. J., and Diamond, D. M. (2002). The stressed hippocampus, synaptic plasticity and lost memories. Nat. Rev. Neurosci. 3, 453–462. doi: 10.1038/nrn849
Kim, J. J., and Haller, J. (2007). Glucocorticoid hyper- and hypofunction: stress effects on cognition and aggression. Ann. N Y Acad. Sci. 1113, 291–303. doi: 10.1196/annals.1391.014
Kim, E. J., Kim, E. S., Park, M., Cho, J., and Kim, J. J. (2012). Amygdalar stimulation produces alterations on firing properties of hippocampal place cells. J. Neurosci. 32, 11424–11434. doi: 10.1523/JNEUROSCI.1108-12.2012
Kim, J. J., Koo, J. W., Lee, H. J., and Han, J. S. (2005). Amygdalar inactivation blocks stress-induced impairments in hippocampal long-term potentiation and spatial memory. J. Neurosci. 25, 1532–1539. doi: 10.1523/JNEUROSCI.4623-04.2005
Kim, J. J., Lee, H. J., Han, J. S., and Packard, M. G. (2001). Amygdala is critical for stress-induced modulation of hippocampal long-term potentiation and learning. J. Neurosci. 21, 5222–5228. doi: 10.1523/JNEUROSCI.21-14-05222.2001
Kim, J. J., Lee, H. J., Welday, A. C., Song, E., Cho, J., Sharp, P. E., et al. (2007). Stress-induced alterations in hippocampal plasticity, place cells, and spatial memory. Proc. Natl. Acad. Sci. U S A 104, 18297–18302. doi: 10.1073/pnas.0708644104
Kim, E. J., Pellman, B., and Kim, J. J. (2015). Stress effects on the hippocampus: a critical review. Learn. Mem. 22, 411–416. doi: 10.1101/lm.037291.114
King, J. A., Mandansky, D., King, S., Fletcher, K. E., and Brewer, J. (2001). Early sexual abuse and low cortisol. Psychiatry Clin. Neurosci. 55, 71–74. doi: 10.1046/j.1440-1819.2001.00787.x
Kuo, T., McQueen, A., Chen, T. C., and Wang, J. C. (2015). Regulation of glucose homeostasis by glucocorticoids. Adv. Exp. Med. Biol. 872, 99–126. doi: 10.1007/978-1-4939-2895-8_5
Lemieux, A. M., and Coe, C. L. (1995). Abuse-related posttraumatic stress disorder: evidence for chronic neuroendocrine activation in women. Psychosom. Med. 57, 105–115. doi: 10.1097/00006842-199503000-00002
Lupien, S. J., and Lepage, M. (2001). Stress, memory and the hippocampus: can’t live with it, can’t live without it. Behav. Brain Res. 127, 137–158. doi: 10.1016/s0166-4328(01)00361-8
Maes, M., Lin, A., Bonaccorso, S., van Hunsel, F., Van Gastel, A., Delmeire, L., et al. (1998). Increased 24-hour urinary cortisol excretion in patients with post-traumatic stress disorder and patients with major depression, but not in patients with fibromyalgia. Acta Psychiatr. Scand. 98, 328–335. doi: 10.1111/j.1600-0447.1998.tb10092.x
Maier, S. F., Amat, J., Baratta, M. V., Paul, E., and Watkins, L. R. (2006). Behavioral control, the medial prefrontal cortex, and resilience. Dialogues Clin. Neurosci. 8, 397–406.
Maier, S. F., and Watkins, L. R. (2005). Stressor controllability and learned helplessness: the roles of the dorsal raphe nucleus, serotonin, and corticotropin-releasing factor. Neurosci. Biobehav. Rev. 29, 829–841. doi: 10.1016/j.neubiorev.2005.03.021
Masters, J. N., Finch, C. E., and Sapolsky, R. M. (1989). Glucocorticoid endangerment of hippocampal neurons does not involve deoxyribonucleic acid cleavage. Endocrinology 124, 3083–3088. doi: 10.1210/endo-124-6-3083
McEwen, B. S. (1999). Stress and hippocampal plasticity. Annu. Rev. Neurosci. 22, 105–122. doi: 10.1146/annurev.neuro.22.1.105
McEwen, B. S. (2013). Neuroscience. Hormones and the social brain. Science 339, 279–280. doi: 10.1126/science.1233713
McEwen, B. S., and Sapolsky, R. M. (1995). Stress and cognitive function. Curr. Opin. Neurobiol. 5, 205–216. doi: 10.1016/0959-4388(95)80028-X
Mello-Carpes, P. B., and Izquierdo, I. (2013). The nucleus of the solitary tract –> nucleus paragigantocellularis –> locus coeruleus –> CA1 region of dorsal hippocampus pathway is important for consolidation of object recognition memory. Neurobiol. Learn. Mem. 100, 56–63. doi: 10.1016/j.nlm.2012.12.002
Milad, M. R., and Quirk, G. J. (2002). Neurons in medial prefrontal cortex signal memory for fear extinction. Nature 420, 70–74. doi: 10.1038/nature01138
Morales-Medina, J. C., Sanchez, F., Flores, G., Dumont, Y., and Quirion, R. (2009). Morphological reorganization after repeated corticosterone administration in the hippocampus, nucleus accumbens and amygdala in the rat. J. Chem. Neuroanat. 38, 266–272. doi: 10.1016/j.jchemneu.2009.05.009
Mumby, D. G. (2001). Perspectives on object-recognition memory following hippocampal damage: lessons from studies in rats. Behav. Brain Res. 127, 159–181. doi: 10.1016/s0166-4328(01)00367-9
Newcomer, J. W., Craft, S., Hershey, T., Askins, K., and Bardgett, M. E. (1994). Glucocorticoid-induced impairment in declarative memory performance in adult humans. J. Neurosci. 14, 2047–2053. doi: 10.1523/JNEUROSCI.14-04-02047.1994
Nicolaides, N. C., Pavlaki, A. N., Maria Alexandra, M. A., and Chrousos, G. P. (2000). “Glucocorticoid therapy and adrenal suppression,” in Endotext [Internet], eds K. R. Feingold, B. Anawalt, A. Boyce, G. Chrousos, K. Dungan, A. Grossman, J. M. Hershman, G. Kaltsas, C. Koch, P. Kopp, M. Korbonits, R. McLachlan, J. E. Morley, M. New, L. Perreault, J. Purnell, R. Rebar, F. Singer, D. L. Trence, A. Vinik and D. P. Wilson (South Dartmouth, MA: MDText.com, Inc.).
Oomen, C. A., Mayer, J. L., de Kloet, E. R., Joëls, M., and Lucassen, P. J. (2007). Brief treatment with the glucocorticoid receptor antagonist mifepristone normalizes the reduction in neurogenesis after chronic stress. Eur. J. Neurosci. 26, 3395–3401. doi: 10.1111/j.1460-9568.2007.05972.x
Oquendo, M. A., Echavarria, G., Galfalvy, H. C., Grunebaum, M. F., Burke, A., Barrera, A., et al. (2003). Lower cortisol levels in depressed patients with comorbid post-traumatic stress disorder. Neuropsychopharmacology 28, 591–598. doi: 10.1038/sj.npp.1300050
Pavlides, C., Ogawa, S., Kimura, A., and McEwen, B. S. (1996). Role of adrenal steroid mineralocorticoid and glucocorticoid receptors in long-term potentiation in the CA1 field of hippocampal slices. Brain Res. 738, 229–235. doi: 10.1016/s0006-8993(96)00776-7
Pavlides, C., Watanabe, Y., Magariños, A. M., and McEwen, B. S. (1995). Opposing roles of type I and type II adrenal steroid receptors in hippocampal long-term potentiation. Neuroscience 68, 387–394. doi: 10.1016/0306-4522(95)00151-8
Pitman, R. K., and Orr, S. P. (1990). Twenty-four hour urinary cortisol and catecholamine excretion in combat-related posttraumatic stress disorder. Biol. Psychiatry 27, 245–247. doi: 10.1016/0006-3223(90)90654-k
Radley, J. J., Rocher, A. B., Miller, M., Janssen, W. G., Liston, C., Hof, P. R., et al. (2006). Repeated stress induces dendritic spine loss in the rat medial prefrontal cortex. Cereb. Cortex 16, 313–320. doi: 10.1093/cercor/bhi104
Sapolsky, R. M. (2000). Stress hormones: good and bad. Neurobiol. Dis. 7, 540–542. doi: 10.1006/nbdi.2000.0350
Sapolsky, R. M., Krey, L. C., and McEwen, B. S. (1986). The neuroendocrinology of stress and aging: the glucocorticoid cascade hypothesis. Endocr. Rev. 7, 284–301. doi: 10.1210/edrv-7-3-284
Schaaf, M. J., De Kloet, E. R., and Vreugdenhil, E. (2000). Corticosterone effects on BDNF expression in the hippocampus. Implications for memory formation. Stress 3, 201–208. doi: 10.3109/10253890009001124
Schoenfeld, T. J., and Gould, E. (2012). Stress, stress hormones, and adult neurogenesis. Exp. Neurol. 233, 12–21. doi: 10.1016/j.expneurol.2011.01.008
Shin, L. M., Whalen, P. J., Pitman, R. K., Bush, G., Macklin, M. L., Lasko, N. B., et al. (2001). An fMRI study of anterior cingulate function in posttraumatic stress disorder. Biol. Psychiatry 50, 932–942. doi: 10.1016/s0006-3223(01)01215-x
Starkman, M. N., Gebarski, S. S., Berent, S., and Schteingart, D. E. (1992). Hippocampal formation volume, memory dysfunction, and cortisol levels in patients with Cushing’s syndrome. Biol. Psychiatry 32, 756–765. doi: 10.1016/0006-3223(92)90079-f
Wiegert, O., Joels, M., and Krugers, H. (2006). Timing is essential for rapid effects of corticosterone on synaptic potentiation in the mouse hippocampus. Learn. Mem. 13, 110–113. doi: 10.1101/lm.87706
Woodson, J. C., Macintosh, D., Fleshner, M., and Diamond, D. M. (2003). Emotion-induced amnesia in rats: working memory-specific impairment, corticosterone-memory correlation, and fear versus arousal effects on memory. Learn. Mem. 10, 326–336. doi: 10.1101/lm.62903
Woolley, C. S., Gould, E., and McEwen, B. S. (1990). Exposure to excess glucocorticoids alters dendritic morphology of adult hippocampal pyramidal neurons. Brain Res. 531, 225–231. doi: 10.1016/0006-8993(90)90778-a
Yang, C. H., Huang, C. C., and Hsu, K. S. (2004). Behavioral stress modifies hippocampal synaptic plasticity through corticosterone-induced sustained extracellular signal-regulated kinase/mitogen-activated protein kinase activation. J. Neurosci. 24, 11029–11034. doi: 10.1523/JNEUROSCI.3968-04.2004
Yehuda, R. (2009). Status of glucocorticoid alterations in post-traumatic stress disorder. Ann. N Y Acad. Sci. 1179, 56–69. doi: 10.1111/j.1749-6632.2009.04979.x
Yehuda, R., Golier, J. A., and Kaufman, S. (2005). Circadian rhythm of salivary cortisol in Holocaust survivors with and without PTSD. Am. J. Psychiatry 162, 998–1000. doi: 10.1176/appi.ajp.162.5.998
Yoon, T., Okada, J., Jung, M. W., and Kim, J. J. (2008). Prefrontal cortex and hippocampus subserve different components of working memory in rats. Learn. Mem. 15, 97–105. doi: 10.1101/lm.850808
Keywords: stress, corticosterone, amygdala, prefrontal cortex, hippocampus, object recognition memory, learning, cognition
Citation: Kim EJ and Kim JJ (2019) Amygdala, Medial Prefrontal Cortex and Glucocorticoid Interactions Produce Stress-Like Effects on Memory. Front. Behav. Neurosci. 13:210. doi: 10.3389/fnbeh.2019.00210
Received: 24 June 2019; Accepted: 29 August 2019;
Published: 18 September 2019.
Edited by:
Jee Hyun Kim, Florey Institute of Neuroscience and Mental Health, AustraliaReviewed by:
Phillip R. Zoladz, Ohio Northern University, United StatesRyan McLaughlin, Washington State University, United States
Copyright © 2019 Kim and Kim. This is an open-access article distributed under the terms of the Creative Commons Attribution License (CC BY). The use, distribution or reproduction in other forums is permitted, provided the original author(s) and the copyright owner(s) are credited and that the original publication in this journal is cited, in accordance with accepted academic practice. No use, distribution or reproduction is permitted which does not comply with these terms.
*Correspondence: Eun Joo Kim, ZWpraW03MzFAdXcuZWR1; Jeansok J. Kim, amVhbnNva2tAdXcuZWR1