- 1Department of Animal Physiology and Molecular Biomedicine, Justus-Liebig-University Giessen, Giessen, Germany
- 2Departamento de Biodiversidad y Biología Experimental, IBBEA-CONICET, Universidad de Buenos Aires, Buenos Aires, Argentina
- 3Department of Biology, Stanford University, Stanford, CA, United States
The glomerular array in the olfactory bulb of many vertebrates is segregated into molecularly and anatomically distinct clusters linked to different olfactory functions. In anurans, glomerular clustering is so far only described in Xenopus laevis. We traced olfactory projections to the bulb in tadpoles belonging to six distantly related anuran species in four families (Pipidae, Hylidae, Bufonidae, Dendrobatidae) and found that glomerular clustering is remarkably conserved. The general bauplan consists of four unequally sized glomerular clusters with minor inter-species variation. During metamorphosis, the olfactory system undergoes extensive remodeling. Tracings in metamorphotic and juvenile Dendrobates tinctorius and Xenopus tropicalis suggest a higher degree of variation in the glomerular organization after metamorphosis is complete. Our study highlights, that the anatomical organization of glomeruli in the main olfactory bulb (MOB) is highly conserved, despite an extensive ecomorphological diversification among anuran tadpoles, which suggests underlying developmental constraints.
Introduction
As in most tetrapods, the olfactory periphery of anuran amphibians is segregated into a main olfactory epithelium (MOE) and a vomeronasal organ (VNO; Eisthen, 1997; Reiss and Eisthen, 2008). Also, several smaller olfactory surfaces have been characterized as specific adaptations to either aquatic olfaction (recessus olfactorius and middle chamber epithelium of the pipid frogs; Helling, 1938; Nowack et al., 2013; Jungblut et al., 2017), aerial olfaction (eminentia olfactoria; Helling, 1938) or possible feeding mechanisms (buccal exposed epithelium; Jungblut et al., 2017). Odorant detection in vertebrates relies on ciliated or microvillous olfactory receptor neurons (ORNs) expressing a single allele belonging to one of several multigene families coding for G-protein coupled olfactory receptors (Buck and Axel, 1991; Dulac and Axel, 1995; Herrada and Dulac, 1997; Liberles and Buck, 2006; Rivière et al., 2009; Greer et al., 2016). In amphibians, each ORN residing in the MOE projects its axon towards one or multiple spheroid neuropil structures (glomeruli) in the main olfactory bulb (MOB; Hassenklöver and Manzini, 2013; Weiss et al., 2020), where synapses with postsynaptic projection neurons are formed.
The glomerular array of many vertebrates is organized in anatomical and functional clusters (Baier and Korsching, 1994; Frontini et al., 2003; Gaudin and Gascuel, 2005; Braubach et al., 2012). A detailed account of glomerular organization in anurans is available only from the fully aquatic Xenopus laevis (Manzini and Schild, 2010). X. laevis tadpoles have at least two separate odor processing streams from the MOE to spatially segregated glomerular clusters in the MOB. These streams rely on different ORN types, second messenger cascades, and odorant receptor types (Manzini et al., 2002; Gliem et al., 2013). Comparative studies with other anuran species are necessary to understand the relevance of this organization.
The lifecycle of most anurans contains an aquatic larva transforming into an adult frog that dwells on trees, in the water or underground (Duellman and Trueb, 1994; Wells, 2007). However, tadpoles have also diversified and adapted to a variety of aquatic and semiaquatic habitats (Altig and McDiarmid, 1999; Roelants et al., 2011). One distinctive feature among tadpole morphotypes is the oral apparatus and in particular the presence or absence of keratinized mouthparts, which has an impact on the trophic niche occupied by the animals (Orton, 1953; Altig and McDiarmid, 1999). Integrating morphological traits and habitat choice led to the categorization of tadpoles into ecomorphological guilds (Altig and Johnston, 1989). However, little is known about how the sensory system anatomy and function are adapted to the specific demands presented by various habitats.
We analyzed and compared the glomerular organization in the MOB of larval anurans belonging to six different species of four families (species overview in Figure 1). Glomeruli of all examined tadpoles showed anatomical segregation into distinct glomerular clusters. The conserved olfactory bulb architecture between the two members of early-diverging pipid frogs and later diverging neobatrachian frogs suggests an evolutionary constraint in the glomerular configuration in anuran tadpoles. Furthermore, we provide an outlook, that the organization of glomerular clusters in postmetamorphotic frogs might be more variable than in tadpoles.
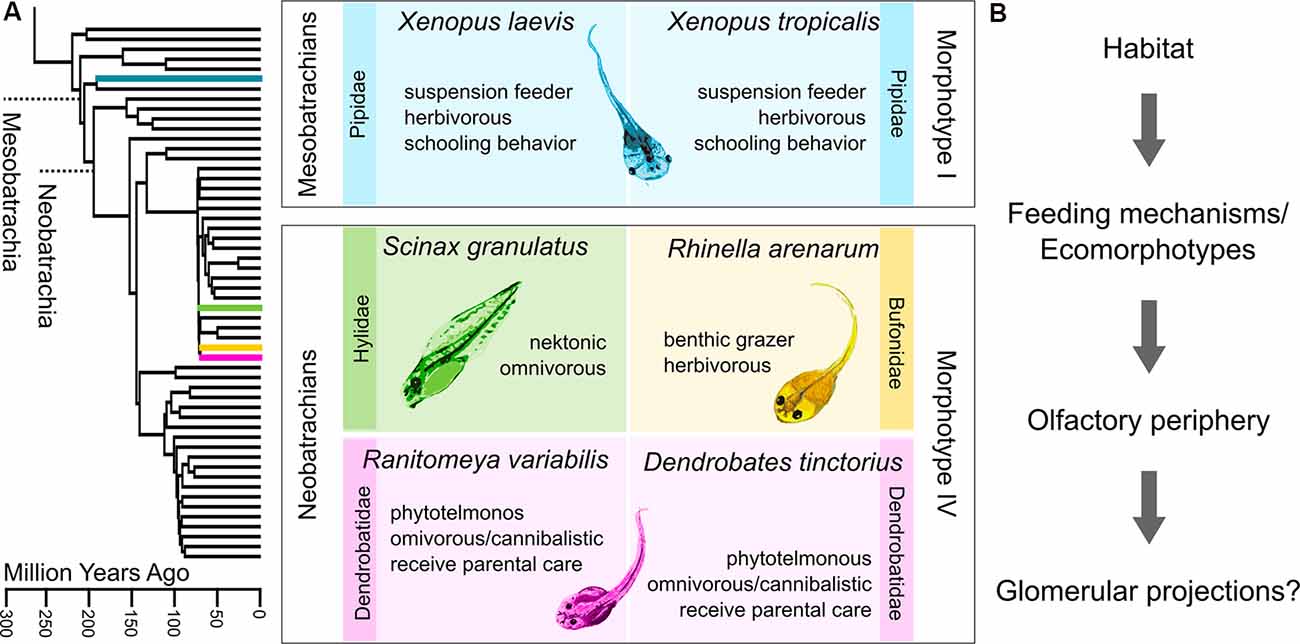
Figure 1. Diversity of anuran tadpoles used in this study. (A) The phylogenetic tree on the left is pruned from Pyron (2014), which originally includes 3,309 species. The four families to which the six examined species belong are highlighted. The middle panel describes the six species based on phylogeny, ecology, and morphology. Both Xenopus species belong to earlier diverging mesobatrachians, lack keratinized mouthparts (morphotype I), and are thus obligate suspension feeders. The four neobatrachian species can all be classified as morphotype IV. Their developed mouthparts enable them to scrape off food from the substrate. The two dendrobatid species both receive parental care and mostly live in pools in leaf axils or bromeliads. Morphotype distinction follows (Orton, 1953) and ecomorphotypic categorizations are based on (Altig and Johnston, 1989). (B) Experimental concept of this study. We tried to examine whether the glomerular organization in the main olfactory bulb (MOB) of tadpoles is influenced by the respective habitat or feeding mechanisms in the distantly related species.
Materials and Methods
Experimental Animals
Wild type and albino tadpoles of Xenopus laevis and larvae of wild type Xenopus tropicalis were bred and reared at the Institute of Animal Physiology, Justus-Liebig-University Giessen and kept in water tanks at a water temperature of 20°C and 25°C respectively. Tadpoles were fed with algae until the end of metamorphosis. Ranitomeya variabilis larvae and larvae and juveniles of Dendrobates tinctorius were bred and reared in the Department of Biology at Stanford University, Palo Alto, CA, USA. Individual tadpoles were kept separately after hatching at a water temperature of 25°C and fed with brine shrimp flakes and tadpole pellets, juveniles were kept in terraria and fed with flies. Rhinella arenarum tadpoles were obtained by in vitro fertilization from a colony at the Faculdad de Ciencias Exactas y Naturales of the University of Buenos Aires. Larvae of Scinax granulatus were collected from temporary ponds in the surroundings of the Campus of the University of Buenos Aires. All larvae were kept in tanks of dechlorinated water at 22°C and fed with chard leaves. The sex of the developing gonad in the tadpoles and juveniles was not determined in any of the species.
An overview of the quantitatively analyzed samples of premetamorphotic larvae (Figure 2) is shown in Table 1. Experiments in higher staged tadpoles and juveniles were conducted for Dendrobates tinctorius (one animal stage 41, and two juveniles stage 45 after; Gosner, 1960) and Xenopus tropicalis (seven metamorphotic animals staged 55, 56, 58, 58, 61, 63 and 65 after Nieuwkoop and Faber, 1994).
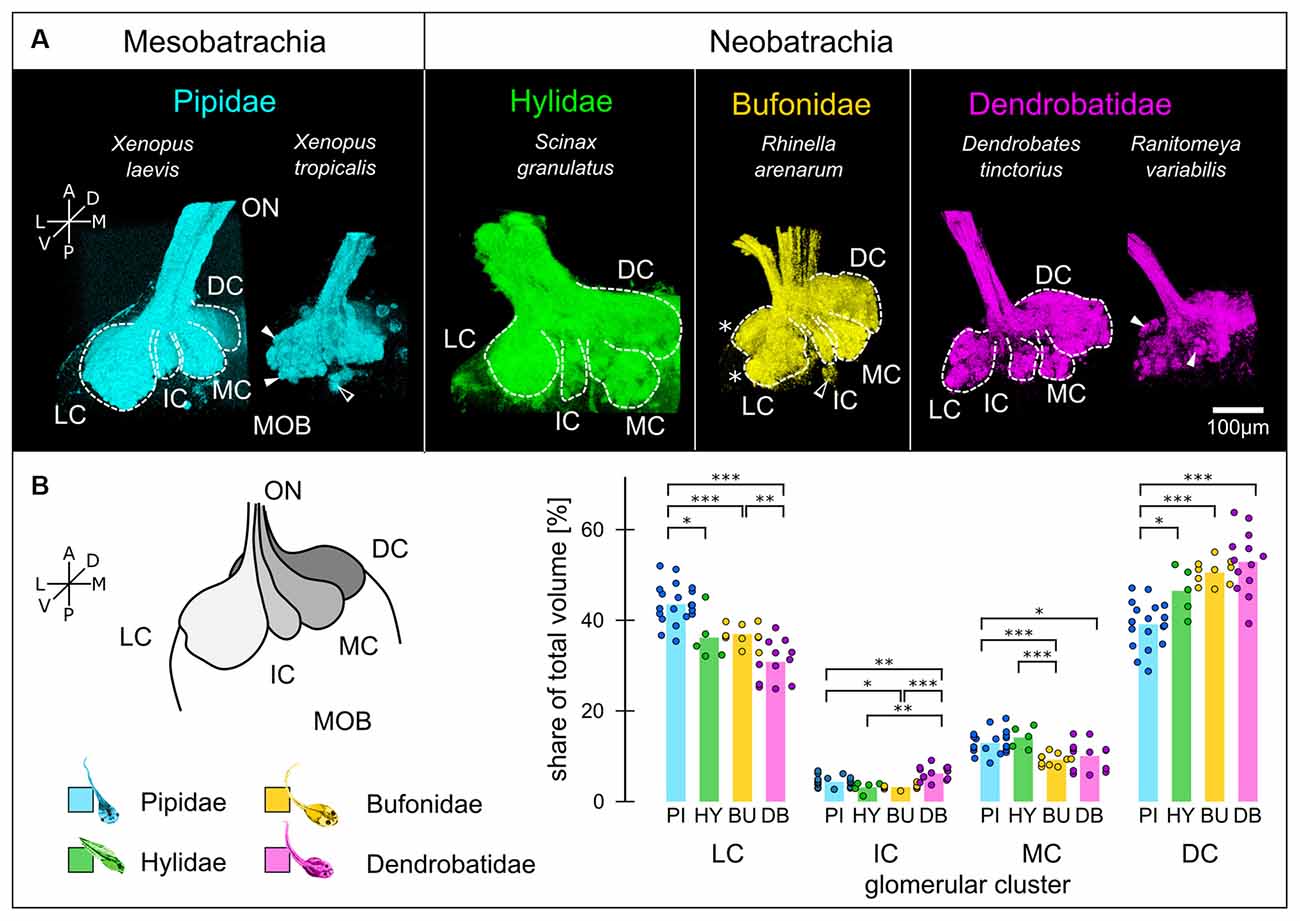
Figure 2. Glomerular clustering in the MOB is conserved among anuran tadpoles. (A) Glomeruli in the MOB of all species can be segregated into three ventrally (LC, IC, MC) and one dorso-medially located clusters (DC). White dotted lines—cluster outlines, filled arrowheads—glomeruli, empty arrowhead—small glomerular cluster, asterisks—ventral and dorsal lobes of the LC. (B) The relative volume of the clusters (schematically shown on the left) varies between the four families. The volumes of the clusters relative to the total glomerular volume for each family are shown. Each dot represents one MOB hemisphere and species of the same family are grouped. Significance levels: ***p < 0.001, **p < 0.01, *p < 0.05. A, anterior; P, posterior; L, lateral; M, medial; D, dorsal; V, ventral; ON, olfactory nerve; MOB, main olfactory bulb; LC, lateral cluster; IC, intermediate cluster; MC, medial cluster; DC, dorsomedial cluster; PI, Pipidae; HY, Hylidae; BU, Bufonidae; DB, Dendrobatidae.
Tracing of Olfactory Projections
Animals were anesthetized in 0.02% MS-222 (ethyl 3-aminobenzoate methanesulfonate; Sigma–Aldrich, St. Louis, MO, USA) for approx. 5 min and placed on a wet paper towel under a stereoscope with fluorescent illumination. Olfactory projections from the nasal cavity to the MOB were labeled using Wheat-Germ agglutinin (WGA)-coupled fluorophores (10 μg/μl, WGA-Alexa Fluor 594/488, Thermo Fisher Scientific, Waltham, MA, USA) dissolved in frog Ringer (in mM: 98 NaCl, 2 KCl, 1 CaCl2, 2 MgCl2, 5 glucose, 5 Na-pyruvate, 10 Hepes, pH 7.8). The WGA-fluorophore solution (3–5 μl) was pipetted into the nostrils using a microloader and left to be taken up by ORNs for 10 min, while the animal was kept moist. Subsequently, the remaining solution was washed off and the animal transferred to a glass beaker to recover. After at least 24 h the animals were again anesthetized and killed by severing the spinal cord at the level of the brainstem. The olfactory nerves were transected close to the nose and the brain containing the olfactory nerves and the bulbs were dissected out of the tissue. The samples were either scanned immediately without fixation or fixed in 4% PFA in PBS for 1 h and imaged at a later timepoint.
Imaging and Image Processing
The olfactory bulbs were imaged with the ventral surface up and fixed with nylon-stringed frames in a recording chamber. Image stacks were recorded at a z-resolution of 3 μm using multiphoton microscopy (upright Nikon A1R-MP and upright Leica SP5 multiphoton microscopes; excitation wavelength 780 nm) and processed in ImageJ (Schindelin et al., 2012). Since only one fluorophore was introduced per sample, we recorded pigmentation-derived auto-fluorescence by simultaneously recording with detectors of different emission-wavelength. In animal species with high pigmentation, we mathematically subtracted this auto-fluorescent signal from the images using the Image calculator function in ImageJ. Brightness and contrast were adjusted, and median filters were applied where necessary. Images showing both olfactory bulbs were stitched together using the stitching algorithm developed by Preibisch et al. (2009). All images presented are rendered in 3D using the 3D viewer plugin implemented in ImageJ.
Volume Measurements and Statistics
Glomerular clusters were manually identified, contoured on various z-planes of the image stacks, and interpolated using the Segmentation Editor in ImageJ. The contours of a cluster were drawn according to the following criteria: clusters are spatially separated and connect to the olfactory nerve via axon fascicles. No fascicles between clusters were observed, thus resulting in a gap between two clusters. The segments labeled and measured did not include the nerve fibers projecting to these clusters. The volumes of the labeled clusters were analyzed using Python and their relative share of the total glomerular volume is presented in percentages. Averaged data are presented as mean ± standard deviation. For statistical analysis, the relative volumes of olfactory projections in each bulb hemisphere were considered as independent samples. Statistical significance was assessed using a One-Way ANOVA separately for each of the four clusters followed by Student’s t-tests for multiple comparisons. To control the familywise error, a Holm-Bonferroni correction was applied.
Results
We first compared the larval glomerular organization by tracing the projections of ORNs from the MOE to the MOB (Figure 2). Wheat-Germ-Agglutinin (WGA) tracings of the most distal part of the left olfactory nerve (ON) and the glomeruli for each species are shown. Glomeruli can be discerned as spheroid accumulations of WGA (white arrowheads, Figure 2A). The glomerular array in all species is segregated into four unequally sized clusters (white dotted lines, Figure 2B), best described by their location as lateral (LC), intermediate (IC), medial (MC) and dorsomedial cluster (DC; Nezlin et al., 2003; Gaudin and Gascuel, 2005; Manzini et al., 2007). This organization is conserved between all species with only minor differences. The LC in Rhinella arenarum tadpoles show a clear bipartition into a dorsal and ventral lobe (white asterisk, bufonid tracings, Figure 2A). This bipartition is also present in the two Xenopus species, but not equally apparent as in R. arenarum. In addition to the four bigger clusters, a few ventro-posterior glomeruli could be observed in all species but were not clearly identifiable in all samples (empty arrowheads, Figure 2A; Brinkmann and Schild, 2016). Glomeruli in the DC were more clearly discernible in the Neobatrachians compared to Xenopus.
We then measured the percentual share of the clusters relative to the total glomerular volume (schematically shown in Figure 2B). Data from species belonging to the same family were pooled together. Across all species, the LC and the DC were most prominent with a combined relative volume of approx. 80%. In pipid tadpoles (n = 20 olfactory bulbs/11 animals; cyan in Figure 2B), the LC is slightly bigger than the DC, with 43.6 ± 4.3% and 39.1 ± 4.9% of the total volume, respectively. Contrastingly, in tadpoles of the other families (Hylidae: n = 5/3, green; Bufonidae: n = 10/6, yellow; Dendrobatidae: n = 13/7, magenta), the DC is bigger than the LC, with 46.5 ± 5.2% compared to 36.2 ± 5.4% in Hylidae, 50.6 ± 2.7% to 37 ± 2.1% in Bufonidae and 52.9 ± 6.9% to 30.8 ± 4.4% in Dendrobatidae. The percentual share of the LC in the Pipidae is significantly higher than in the other families (Figure 2B, left) and DC is significantly smaller (Figure 2B, right). The IC and the MC in all species are smaller than the other two clusters with approx. 5 and 10% of the total glomerular volume, respectively. The IC is biggest in the dendrobatid tadpoles (6.2 ± 1.6%) and significantly bigger than the IC in the other families (Pipidae: 4.4 ± 1.2%; Hylidae 3.2 ± 1.2, Bufonidae: 3.2 ± 0.5). The MC is smallest in the Bufonidae (9.3 ± 1.3%; Figure 2B) and biggest in hylid tadpoles (14.2 ± 2.4%). The MC of the dendrobatid tadpoles (10.1 ± 3.2%) is also smaller than in the pipids (12.9 ± 2.5%).
In most anurans, metamorphosis is accompanied by major habitat changes, which also impacts the olfactory system. We labeled glomeruli of the pipid Xenopus tropicalis and the dendrobatid Dendrobates tinctorius in different larval stages during metamorphosis and in early postmetamorphotic animals (Figure 3). In premetamorphotic tadpoles, the glomerular clusters of the left and the right MOB are separated at the interhemispheric midline (top images, Figure 3). At that stage, tadpoles of D. tinctorius already have a prominent dorsal glomerular region that extends towards the midline, while this region is less visible in X. tropicalis tadpoles. During metamorphosis, the dorsal glomerular regions of both sides start to medially fuse in both examined species (middle images, Figure 3). The ventral clusters (LC; IC; MC) are still present and remain unchanged. In both species, a bundle of axons bypasses the glomerular clusters, terminates in higher brain centers, or crosses the midline more caudally (white arrows, Figure 3). After the completion of metamorphosis (bottom images, Figure 3), the ventral clusters in X. tropicalis are unchanged, while they are reduced in D. tinctorius (asterisks, Figure 3). The LC is still clearly discernable in both species, while especially the IC and MC of D. tinctorius are not clearly delineated anymore.
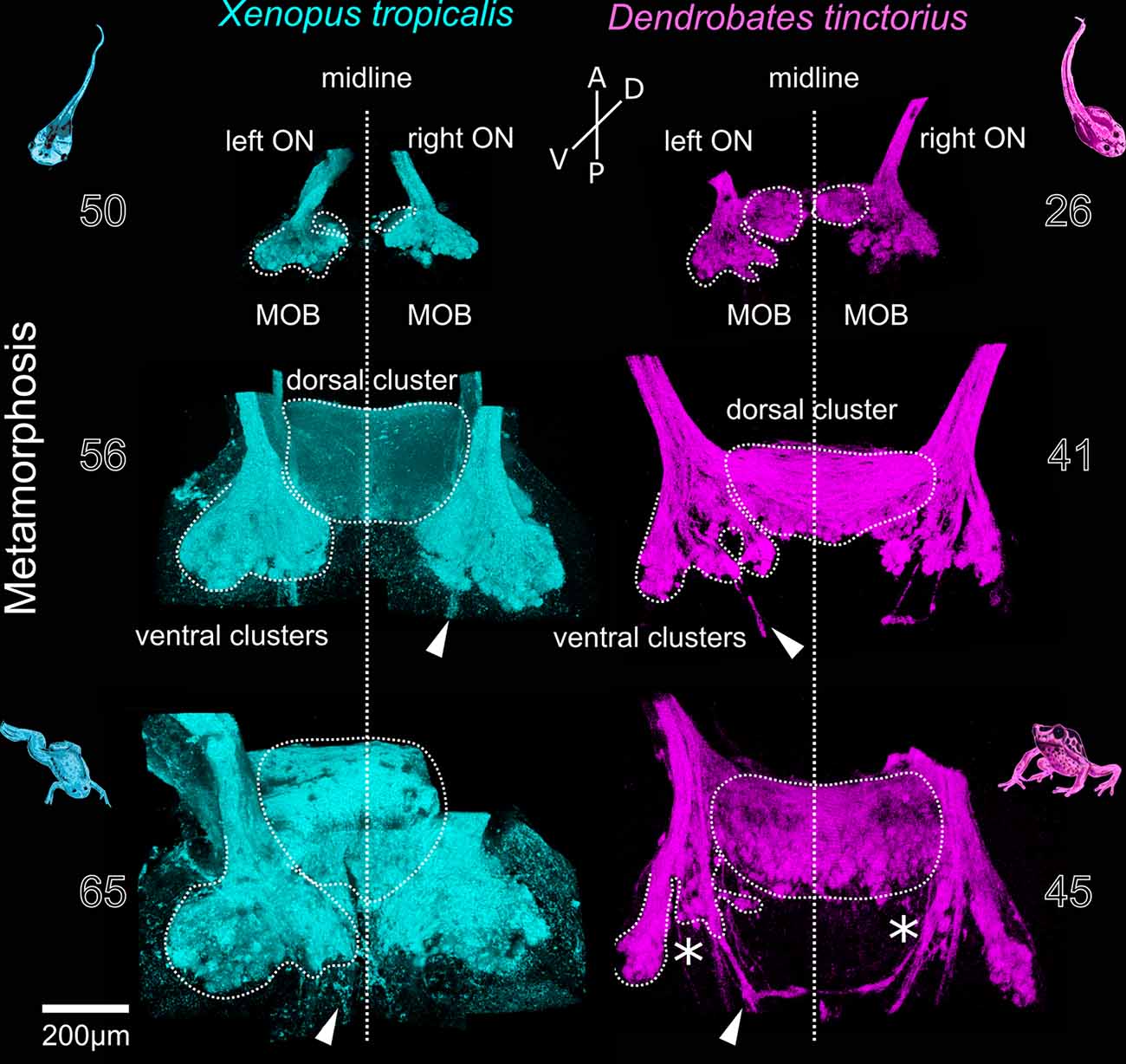
Figure 3. Metamorphotic changes of glomerular clusters in Xenopus tropicalis and Dendrobates tinctorius. Before metamorphosis (top), the left and right glomerular projections in the MOB are separated at the midline (vertical line). During metamorphosis, the dorsomedial components form an unpaired dorsal cluster (middle). In the late phases of metamorphosis (bottom), the ventral glomerular clusters in X. tropicalis are unchanged, while they are reduced in D. tinctorius (asterisks). Arrowheads—extrabulbar fibers. The numbers next to the images indicate the developmental stages after Nieuwkoop and Faber for X. tropicalis and Gosner for D.tinctorius. A, anterior; P, posterior; D, dorsal; V, ventral; ON, olfactory nerve; MOB, main olfactory bulb.
Discussion
During vertebrate evolution, a trend towards segregation into olfactory subsystems is apparent. Mammals possess several anatomically and molecularly distinct olfactory organs that project to different glomerular regions of the olfactory bulb (Munger et al., 2009; Bear et al., 2016). In fishes, ORNs expressing different odorant receptors are intermingled in a single olfactory surface (Hamdani and Døving, 2007). ORNs expressing receptors belonging to the same gene family often project to segregated glomerular clusters or regions in the olfactory bulb, putatively constituting functionally distinct odorant processing streams (Baier and Korsching, 1994; Hamdani et al., 2001; Frontini et al., 2003; Hansen et al., 2003, 2004; Sato, 2005; Braubach et al., 2012; Green et al., 2017). In anurans, a detailed analysis of the glomerular array is only available from the African Clawed frog Xenopus laevis (Gaudin and Gascuel, 2005; Manzini et al., 2007). Here we show that the organization of glomerular clusters is remarkably conserved between six distantly related anuran species despite a quite diverse eco-morphology of the examined tadpoles.
In larval X. laevis, glomerular clusters have been associated with a lateral and medial odorant processing stream (Manzini et al., 2002; Gliem et al., 2013). The medially located glomeruli in the MOB of X. laevis tadpoles are putatively innervated by ciliated ORNs expressing OR-type odorant receptors linked to Gα/olf and using the cAMP transduction pathway. The medial stream shows responses to alcohols, aldehydes, and ketones (Gliem et al., 2013). The lateral cluster on the other hand is highly responsive to amino acid stimulation and expresses Gα/oand Gα/i linked to a cAMP-independent transduction pathway (Manzini and Schild, 2003; Gliem et al., 2013). Vomeronasal-type receptors (V1Rs or V2Rs), as well as trace-amine-associated receptors (TAARs), have been proposed to mediate this lateral stream (Date-Ito et al., 2008; Gliem et al., 2013; Syed et al., 2013). Even though these pathways are described quite in detail, their functional and behavioral significance is so far unknown. Amino acids are generally known as a potent food stimulus in fishes (Hamdani and Døving, 2007) and a lateral processing stream linked to feeding behavior has been identified in the olfactory bulb of the carp (Hamdani et al., 2001). Whether the lateral glomerular cluster in tadpoles also mediates feeding or foraging behavior remains to be elucidated (Terni et al., 2018).
Tadpoles of the examined species vary extensively in their feeding behavior, as they have adapted to a variety of microhabitats by differentiating into several morphotypes (Orton, 1953; Roelants et al., 2011). Earlier diverging frogs, like most pipids, rely on a large buccopharyngeal space to pump water through their body (Orton’s morphotype I), trapping mostly algae or other small food particles (Seale, 1982). However, derived phenotypes are more specialized, where the development of keratinized mouthparts enables tadpoles of Orton’s morphotype IV to rasp food particles off the ground or prey animals (Altig and Johnston, 1989; Roelants et al., 2011). For example, tadpoles of many dendrobatid frogs like Ranitomeya variabilis inhabit small temporary pools in bromeliads or leave axils, which often is linked to a scarcity of food resources (Altig and McDiarmid, 1999; Brown et al., 2008) and facultative cannibalism (Masche et al., 2010). In addition to their feeding behavior, there are major differences in social interactions. While tadpoles of Xenopus or Rhinella arenarum tadpoles are often seen forming schools for protection against predators (Altig and McDiarmid, 1999; Wells, 2007), Dendrobates tinctorius tadpoles engage in aggressive behavior against conspecifics (Fischer et al., 2020) and are transported by their parents if the food resources in the pool are exhausted (Altig and McDiarmid, 1999; Brown et al., 2008; Roland and O’Connell, 2015). While tadpoles rely on olfaction for foraging and kin recognition (Waldman, 1991; Veeranagoudar et al., 2004; Villinger and Waldman, 2005), it is currently unclear whether the olfactory system shows adaptation to specific ecological niches.
R. arenarum, like other anuran larvae, also has a part of their MOE exposed to the buccal cavity (Jermakowicz et al., 2004; Benzekri and Reiss, 2012; Jungblut et al., 2017). This buccal exposed epithelium is hypothesized to be important to assess food quality in species that actively scrape food off the substrate and is absent in suspension feeders like X. laevis (Jungblut et al., 2017). The glomerular projection target of this buccal exposed epithelium is currently unknown. It takes up approx. 15–20% of the entire epithelial volume in R. arenarum tadpoles (Jungblut et al., 2017) and is present in Scinax granulatus like in other hylid tadpoles (Jungblut et al., 2017; Jungblut personal observation). The presence of the buccal exposed epithelium in tadpoles of the two dendrobatid species tadpoles to our knowledge. Despite the eco-morphological difference and differences in the structure of the peripheral olfactory organ of the examined species, the spatial organization of glomeruli in the MOB of all examined tadpoles was remarkably similar. It is however still unclear, whether the anatomically similar clusters are also functionally or molecularly equivalent. In larval R. arenarum, both Gα/oand Gα/olf are expressed in MOB glomeruli, but no clear segregation could be observed, in contrast to X. laevis tadpoles (Jungblut et al., 2009). It seems possible that the glomerular clusters are anatomically conserved but innervated by ORNs expressing receptors belonging to different receptor families, thus detecting different odorant cues. Whether the small variation in the relative volume of the described glomerular clusters e.g., the relatively bigger DC in the neobatrachians could be attributed to the presence of the buccal exposed epithelium or other ecological differences needs further experimental evidence.
After metamorphosis, the anatomy of the nose of different frog species is more variable than at the larval level (Helling, 1938). The larval MOE in the principal nasal cavity consisting of microvillous and ciliated ORNs transforms into the adult “air-nose,” solely consisting of ciliated olfactory ORNs (Föske, 1934; Bloom, 1954; Hansen et al., 1998; Reiss and Eisthen, 2008). In pipid frogs, a sensory epithelium consisting of both microvillous and ciliated ORNs forms in the middle cavity (Hansen et al., 1998) and seems to be a molecular and functional copy of the larval MOE (Hansen et al., 1998; Syed et al., 2017). In other anurans, a small patch of “aquatic” epithelium forms at the anterior bottom of the principal cavity, the recessus olfactorius, a putative homolog to the pipid middle cavity (Helling, 1938; Nowack et al., 2013). The middle cavity in these species is non-sensory (Reiss and Eisthen, 2008).
In the adult Xenopus laevis, ORNs located in the de novo formed middle cavity epithelium project to the ventrally located glomerular clusters, while the remodeled principal cavity connects to the dorsomedial glomeruli in the MOB (Reiss and Burd, 1997; Gaudin and Gascuel, 2005). The ventrally located glomerular clusters have been shown to remain anatomically (Gaudin and Gascuel, 2005) and functionally (Weiss et al., 2020) stable during metamorphosis, constituting a channel for waterborne olfaction. The dorsomedial region grows extensively and fuses at the midline, forming a single dorsal MOB (Gaudin and Gascuel, 2005). We show that the development of the dorsomedial portion of the MOB is similar between Xenopus tropicalis and Dendrobates tinctorius (Figure 3). Contrastingly, the ventrally located glomerular clusters are reduced in D. tinctorius after metamorphosis. Since D. tinctorius juveniles and adults are terrestrial, it is not clear to what extent they still rely on aquatic olfaction, in contrast to the fully aquatic Xenopus. Comparative data from different terrestrial and aquatic adults are needed to fully understand adaptive traits in the glomerular organization.
In conclusion, we show that the organization of glomerular clusters in eco-morphologically diverse tadpoles of distantly related species is remarkably conserved. It remains to be shown if the segregation into glomerular clusters also represents functionally distinct subsystems and if the small inter-species variability reflects possible adaptation to specific microhabitats.
Data Availability Statement
The raw data supporting the conclusions of this article will be made available by the authors, without undue reservation.
Ethics Statement
All experiments followed the guidelines of Laboratory animal research of the Ethics Committee of the University of Buenos Aires (CD: 316/12, Protocol #22), Justus-Liebig-University Gießen (GI 15/7 Nr. G 2/2019, 932_GP), and Stanford University (APLAC-33016).
Author Contributions
LW, TH, and IM: conceptualization. LW: investigation, formal analysis, visualization, and writing. LW, LJ, AP, LO’C, TH, and IM: review and editing. LJ, AP, LO’C, TH, and IM: funding acquisition and resources. TH and IM: supervision.
Funding
This work was supported by Deutsche Forschungsgemeinschaft (DFG) Grant 4113/4-1, the cooperation program Consejo Nacional de Investigaciones Científicas y Técnicas (CONICET)-DFG-MINCYT 23120160100031CO, Secretaría de Ciencia y Técnica, Universidad de Buenos Aires (UBACyT) 20020170200191BA and by Award Number S10RR02557401 from the National Center for Research Resources (NCRR). Its contents are solely the responsibility of the authors and do not necessarily represent the official views of the NCRR. LW was granted a travel fellowship (JEBTF-180809) by the Company of Biologists.
Conflict of Interest
The authors declare that the research was conducted in the absence of any commercial or financial relationships that could be construed as a potential conflict of interest.
Acknowledgments
We thank all members of the Manzini laboratory for discussion, Anja Schnecko for technical assistance and Eva Fischer for support to set up experiments.
References
Altig, R., and Johnston, G. F. (1989). Guilds of anuran larvae: relationships among developmental modes, morphologies and habitats. Herpetol. Monogr. 3:81. doi: 10.2307/1466987
Altig, R., and McDiarmid, R. W. (1999). Tadpoles: The Biology of Anuran Larvae. Chicago and London: University of Chicago Press.
Baier, H., and Korsching, S. (1994). Olfactory glomeruli in the zebrafish form an invariant pattern and are identifiable across animals. J. Neurosci. 14, 219–230. doi: 10.1523/jneurosci.14-01-00219.1994
Bear, D. M., Lassance, J.-M., Hoekstra, H. E., and Datta, S. R. (2016). The evolving neural and genetic architecture of vertebrate olfaction. Curr. Biol. 26, R1039–R1049. doi: 10.1016/j.cub.2016.09.011
Benzekri, N. A., and Reiss, J. O. (2012). Olfactory metamorphosis in the coastal tailed frog Ascaphus truei (Amphibia, Anura, Leiopelmatidae). J. Morphol. 273, 68–87. doi: 10.1002/jmor.11008
Bloom, G. (1954). Studies on the olfactory epithelium of the frog and the toad with the aid of light and electron microscopy. Z. Zeitsch. Mikrosk. Anat. 41, 89–100. doi: 10.1007/bf00340285
Braubach, O. R., Fine, A., and Croll, R. P. (2012). Distribution and functional organization of glomeruli in the olfactory bulbs of zebrafish (Danio rerio). J. Comp. Neurol. 520, 2317–2339. doi: 10.1002/cne.23075
Brinkmann, A., and Schild, D. (2016). One special glomerulus in the olfactory bulb of Xenopus laevis tadpoles integrates a broad range of amino acids and mechanical stimuli. J. Neurosci. 36, 10978–10989. doi: 10.1523/jneurosci.4631-15.2016
Brown, J. L., Morales, V., and Summers, K. (2008). Divergence in parental care, habitat selection and larval life history between two species of Peruvian poison frogs: an experimental analysis. J. Evol. Biol. 21, 1534–1543. doi: 10.1111/j.1420-9101.2008.01609.x
Buck, L., and Axel, R. (1991). A novel multigene family may encode odorant receptors: a molecular basis for odor recognition. Cell 65, 175–187. doi: 10.1016/0092-8674(91)90418-x
Date-Ito, A., Ohara, H., Ichikawa, M., Mori, Y., and Hagino-Yamagishi, K. (2008). Xenopus V1R vomeronasal receptor family is expressed in the main olfactory system. Chem. Senses 33, 339–346. doi: 10.1093/chemse/bjm090
Duellman, W. E., and Trueb, L. (1994). Biology of Amphibians. Baltimore: John Hopkins University Press.
Dulac, C., and Axel, R. (1995). A novel family of genes encoding putative pheromone receptors in mammals. Cell 83, 195–206. doi: 10.1016/0092-8674(95)90161-2
Eisthen, H. L. (1997). Evolution of vertebrate olfactory systems. Brain. Behav. Evol. 50, 222–233. doi: 10.1159/000113336
Fischer, E. K., Alvarez, H., Lagerstrom, K. M., Petrillo, R., Ellis, G., and O’Connell, L. A. (2020). Neural correlates of winning and losing fights in poison frog tadpoles. Physiol. Behav. 223:112973. doi: 10.1016/j.physbeh.2020.112973
Föske, H. (1934). Das Geruchsorgan von Xenopus laevis. Z. Anat. Entwicklungsgesch. 103, 519–550. doi: 10.1007/bf02118933
Frontini, A., Zaidi, A. U., Hua, H., Wolak, T. P., Greer, C. A., Kafitz, K. W., et al. (2003). Glomerular territories in the olfactory bulb from the larval stage of the sea lamprey Petromyzon marinus. J. Comp. Neurol. 465, 27–37. doi: 10.1002/cne.10811
Gaudin, A., and Gascuel, J. (2005). 3D atlas describing the ontogenic evolution of the primary olfactory projections in the olfactory bulb of Xenopus laevis. J. Comp. Neurol. 489, 403–424. doi: 10.1002/cne.20655
Gliem, S., Syed, A. S., Sansone, A., Kludt, E., Tantalaki, E., Hassenklöver, T., et al. (2013). Bimodal processing of olfactory information in an amphibian nose: odor responses segregate into a medial and a lateral stream. Cell. Mol. Life Sci. 70, 1965–1984. doi: 10.1007/s00018-012-1226-8
Gosner, K. L. (1960). A simplified table for staging anuran embryos and larvae with notes on identification. Herpetologica 16, 183–190. Available online at: https://www.jstor.org/stable/3890061?seq=1.
Green, W. W., Boyes, K., McFadden, C., Daghfous, G., Auclair, F., Zhang, H., et al. (2017). Odorant organization in the olfactory bulb of the sea lamprey. J. Exp. Biol. 220, 1350–1359. doi: 10.1242/jeb.150466
Greer, P. L., Bear, D. M., Lassance, J. M., Bloom, M. L., Tsukahara, T., Pashkovski, S. L., et al. (2016). A family of non-GPCR chemosensors defines an alternative logic for mammalian olfaction. Cell 165, 1734–1748. doi: 10.1016/j.cell.2016.05.001
Hamdani, E. H., and Døving, K. B. (2007). The functional organization of the fish olfactory system. Prog. Neurobiol. 82, 80–86. doi: 10.1016/j.pneurobio.2007.02.007
Hamdani, E. H., Kasumyan, A., and Døving, K. B. (2001). Is feeding behaviour in Crucian Carp mediated by the lateral olfactory tract? Chem. Senses 26, 1133–1138. doi: 10.1093/chemse/26.9.1133
Hansen, A., Anderson, K. T., and Finger, T. E. (2004). Differential distribution of olfactory receptor neurons in goldfish: structural and molecular correlates. J. Comp. Neurol. 477, 347–359. doi: 10.1002/cne.20202
Hansen, A., Reiss, J. O., Gentry, C. L., and Burd, G. D. (1998). Ultrastructure of the olfactory organ in the clawed frog , Xenopus laevis, during larval development and metamorphosis. J. Comp. Neurol. 398, 273–288. doi: 10.1002/(sici)1096-9861(19980824)398:2<273::aid-cne8>3.0.co;2-y
Hansen, A., Rolen, S. H., Anderson, K., Morita, Y., Caprio, J., and Finger, T. E. (2003). Correlation between olfactory receptor cell type and function in the channel catfish. J. Neurosci. 23, 9328–9339. doi: 10.1523/jneurosci.23-28-09328.2003
Hassenklöver, T., and Manzini, I. (2013). Olfactory wiring logic in amphibians challenges the basic assumptions of the unbranched axon concept. J. Neurosci. 33, 17247–17252. doi: 10.1523/jneurosci.2755-13.2013
Helling, H. (1938). Das Geruchsorgan der Anuren, vergleichend-morphologisch betrachtet. Z. Anat. Entwicklungsgesch. 108, 587–643. doi: 10.1007/bf02118847
Herrada, G., and Dulac, C. (1997). A novel family of putative pheromone receptors in mammals with a topographically organized and sexually dimorphic distribution. Cell 90, 763–773. doi: 10.1016/s0092-8674(00)80536-x
Jermakowicz, W. J., Dorsey, D. A., Brown, A. L., Wojciechowski, K., Giscombe, C. L., Graves, B. M., et al. (2004). Development of the nasal chemosensory organs in two terrestrial anurans: the directly developing frog, Eleutherodactylus coqui (Anura: Leptodactylidae) and the metamorphosing toad, Bufo americanus (Anura: Bufonidae). J. Morphol. 261, 225–248. doi: 10.1002/jmor.10246
Jungblut, L. D., Paz, D. A., López-Costa, J. J., and Pozzi, A. G. (2009). Heterogeneous distribution of G protein alpha subunits in the main olfactory and vomeronasal systems of Rhinella (Bufo) arenarum tadpoles. Zoolog. Sci. 26, 722–728. doi: 10.2108/zsj.26.722
Jungblut, L. D., Reiss, J. O., Paz, D. A., and Pozzi, A. G. (2017). Quantitative comparative analysis of the nasal chemosensory organs of anurans during larval development and metamorphosis highlights the relative importance of chemosensory subsystems in the group. J. Morphol. 278, 1208–1219. doi: 10.1002/jmor.20705
Liberles, S. D., and Buck, L. B. (2006). A second class of chemosensory receptors in the olfactory epithelium. Nature 442, 645–650. doi: 10.1038/nature05066
Manzini, I., Heermann, S., Czesnik, D., Brase, C., Schild, D., and Rössler, W. (2007). Presynaptic protein distribution and odour mapping in glomeruli of the olfactory bulb of Xenopus laevis tadpoles. Eur. J. Neurosci. 26, 925–934. doi: 10.1111/j.1460-9568.2007.05731.x
Manzini, I., Rössler, W., and Schild, D. (2002). cAMP-independent responses of olfactory neurons in Xenopus laevis tadpoles and their projection onto olfactory bulb neurons. J. Physiol. 545, 475–484. doi: 10.1113/jphysiol.2002.031914
Manzini, I., and Schild, D. (2003). cAMP-independent olfactory transduction of amino acids in Xenopus laevis tadpoles. J. Physiol. 551, 115–123. doi: 10.1113/jphysiol.2003.043059
Manzini, I., and Schild, D. (2010). “Olfactory coding in larvae of the African Clawed frog Xenopus laevis,” in The Neurobiology of Olfaction, ed. A. Menini (Boca Raton, FL: CRC Press/Taylor and Francis), 114–126. Available online at: http://www.ncbi.nlm.nih.gov/books/NBK55981/. Accessed April 28, 2015.
Masche, S., Zimmermann, H., and Pröhl, H. (2010). Description and ecological observations of the tadpole of ranitomeya variabilis (Anura: Dendrobatidae). South Am. J. Herpetol. 5, 207–211. doi: 10.2994/057.005.0306
Munger, S. D., Leinders-Zufall, T., and Zufall, F. (2009). Subsystem organization of the mammalian sense of smell. Annu. Rev. Physiol. 71, 115–140. doi: 10.1146/annurev.physiol.70.113006.100608
Nezlin, L. P., Heermann, S., Schild, D., and Rössler, W. (2003). Organization of glomeruli in the main olfactory bulb of Xenopus laevis tadpoles. J. Comp. Neurol. 464, 257–268. doi: 10.1002/cne.10709
Nieuwkoop, D. P., and Faber, J. (1994). Normal Table of Xenopus laevis (Daudin). New York, NY: Garland.
Nowack, C., Jordan, S., and Wittmer, C. (2013). “The recessus olfactorius: a cryptic olfactory organ of anuran amphibians,” in Chemical Signals in Vertebrates, eds. M. L. East and M. Dehnhard (New York, NY: Springer Science+Business Media), 37–48.
Orton, G. L. (1953). The systematics of vertebrate larvae. Syst. Zool. 2, 63–75. doi: 10.2307/2411661
Preibisch, S., Saalfeld, S., and Tomancak, P. (2009). Globally optimal stitching of tiled 3D microscopic image acquisitions. Bioinformatics 25, 1463–1465. doi: 10.1093/bioinformatics/btp184
Pyron, R. A. (2014). Biogeographic analysis reveals ancient continental vicariance and recent oceanic dispersal in amphibians. Syst. Biol. 63, 779–797. doi: 10.1093/sysbio/syu042
Reiss, J. O., and Burd, G. D. (1997). Metamorphic remodeling of the primary olfactory projection in Xenopus: developmental independence of projections from olfactory neuron subclasses. J. Neurobiol. 32, 213–222. doi: 10.1002/(sici)1097-4695(199702)32:2<213::aid-neu6>3.0.co;2-b
Reiss, J. O., and Eisthen, H. L. (2008). “Comparative anatomy and physiology of chemical senses in amphibians,” in Sensory Evolution on the Threshold: Adaptations in Secondarily Aquatic Vertebrates, eds. J. Thewissen and S. Nummela (Oakland, CA: University of California Press), 43–63.
Rivière, S., Challet, L., Fluegge, D., Spehr, M., and Rodriguez, I. (2009). Formyl peptide receptor-like proteins are a novel family of vomeronasal chemosensors. Nature 459, 574–577. doi: 10.1038/nature08029
Roelants, K., Haas, A., and Bossuyt, F. (2011). Anuran radiations and the evolution of tadpole morphospace. Proc. Natl. Acad. Sci. U S A 108, 8731–8736. doi: 10.1073/pnas.1100633108
Roland, A. B., and O’Connell, L. A. (2015). Poison frogs as a model system for studying the neurobiology of parental care. Curr. Opin. Behav. Sci. 6, 76–81. doi: 10.1016/j.cobeha.2015.10.002
Sato, Y. (2005). Mutually exclusive glomerular innervation by two distinct types of olfactory sensory neurons revealed in transgenic zebrafish. J. Neurosci. 25, 4889–4897. doi: 10.1523/jneurosci.0679-05.2005
Schindelin, J., Arganda-Carreras, I., Frise, E., Kaynig, V., Longair, M., Pietzsch, T., et al. (2012). Fiji: an open-source platform for biological-image analysis. Nat. Methods 9, 676–682. doi: 10.1038/nmeth.2019
Seale, D. B. (1982). Obligate and facultative suspension feeding in anuran larvae: feeding regulation in Xenopus and Rana. Biol. Bull. 162, 214–231. doi: 10.2307/1540816
Syed, A. S., Sansone, A., Hassenklöver, T., Manzini, I., Korsching, S. I., Sciences, M. L., et al. (2017). Coordinated shift of olfactory amino acid responses and V2R expression to an amphibian water nose during metamorphosis. Cell. Mol. Life Sci. 74, 1711–1719. doi: 10.1007/s00018-016-2437-1
Syed, A. S., Sansone, A., Nadler, W., Manzini, I., and Korsching, S. I. (2013). Ancestral amphibian v2rs are expressed in the main olfactory epithelium. Proc. Natl. Acad. Sci. U S A 110, 7714–7719. doi: 10.1073/pnas.1302088110
Terni, B., Pacciolla, P., Perelló, M., and Llobet, A. (2018). Functional evaluation of olfactory pathways in living Xenopus tadpoles. J. Vis. Exp. 142:e58028. doi: 10.3791/58028
Veeranagoudar, D. K., Shanbhag, B. A., and Saidapur, S. K. (2004). Mechanism of food detection in the tadpoles of the bronze frog Rana temporalis. Acta Ethol. 7, 37–41. doi: 10.1007/s10211-004-0096-y
Villinger, J., and Waldman, B. (2005). MHC-based kin recognition in African claewd frog tadpoles. News Zeal. J. Zool. 32:230. doi: 10.1080/03014223.2005.9518413
Waldman, B. (1991). “Kin recognition in amphibians,” in Kin Recognit, ed P. Hepper (Cambridge, CA: Cambridge University Press), 162–219. doi: 10.1017/CBO9780511525414.009
Weiss, L., Jungblut, L. D., Pozzi, A. G., Zielinski, B. S., O’Connell, L. A., Hassenklöver, T., et al. (2020). Multi-glomerular projection of single olfactory receptor neurons is conserved among amphibians. J. Comp. Neurol. doi: 10.1002/cne.24887 [Epub ahead of print].
Keywords: amphibians, anura, olfaction, glomeruli, olfactory bulb, evolution
Citation: Weiss L, Jungblut LD, Pozzi AG, O’Connell LA, Hassenklöver T and Manzini I (2020) Conservation of Glomerular Organization in the Main Olfactory Bulb of Anuran Larvae. Front. Neuroanat. 14:44. doi: 10.3389/fnana.2020.00044
Received: 11 May 2020; Accepted: 30 June 2020;
Published: 24 July 2020.
Edited by:
James C. Vickers, University of Tasmania, AustraliaReviewed by:
Ruth Morona, Complutense University of Madrid, SpainMarissa Fabrezi, CONICET Instituto de Bio y Geociencias del NOA (IBIGEO), Argentina
Copyright © 2020 Weiss, Jungblut, Pozzi, O’Connell, Hassenklöver and Manzini. This is an open-access article distributed under the terms of the Creative Commons Attribution License (CC BY). The use, distribution or reproduction in other forums is permitted, provided the original author(s) and the copyright owner(s) are credited and that the original publication in this journal is cited, in accordance with accepted academic practice. No use, distribution or reproduction is permitted which does not comply with these terms.
*Correspondence: Lukas Weiss, bHVrYXMud2Vpc3NAcGh5c3pvb2wuYmlvLnVuaS1naWVzc2VuLmRl