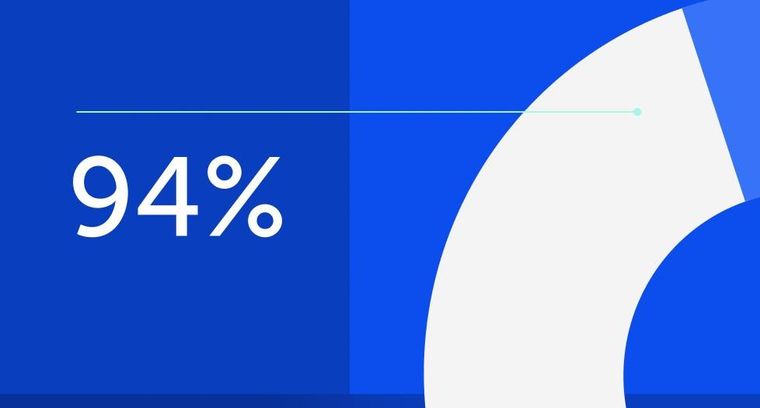
94% of researchers rate our articles as excellent or good
Learn more about the work of our research integrity team to safeguard the quality of each article we publish.
Find out more
MINI REVIEW article
Front. Neuroanat., 09 July 2019
Volume 13 - 2019 | https://doi.org/10.3389/fnana.2019.00071
This article is part of the Research TopicBrain Adaptative Changes in Mammalian HibernationView all 7 articles
Hibernation is a natural phenomenon in many species which helps them to survive under extreme ambient conditions, such as cold temperatures and reduced availability of food in the winter months. It is characterized by a dramatic and regulated drop of body temperature, which in some cases can be near 0°C. Additionally, neural control of hibernation is maintained over all phases of a hibernation bout, including entrance into, during and arousal from torpor, despite a marked decrease in overall neural activity in torpor. In the present review, we provide an overview on what we know about neuronal activity in the hibernating brain focusing on cold-induced adaptations. We discuss pioneer and more recent in vitro and in vivo electrophysiological data and molecular analyses of activity markers which strikingly contributed to our understanding of the brain’s sensitivity to dramatic changes in temperature across the hibernation cycle. Neuronal activity is markedly reduced with decreasing body temperature, and many neurons may fire infrequently in torpor at low brain temperatures. Still, there is convincing evidence that specific regions maintain their ability to generate action potentials in deep torpor, at least in response to adequate stimuli. Those regions include the peripheral system and primary central regions. However, further experiments on neuronal activity are needed to more precisely determine temperature effects on neuronal activity in specific cell types and specific brain nuclei.
Hibernation is an adaptation to extreme environmental conditions, including cold temperatures and reduced food availability. In small mammalian hibernators, it is characterized by reduced metabolism, by severely decreased heart and respiratory rates and by dramatic lowering of body temperatures to few degrees above ambient temperature (Nedergaard and Cannon, 1990; Nurnberger, 1995). Also, the brain temperature drops to 2–3°C above ambient temperature (Strumwasser, 1959). Still, hibernators are able to arouse from the state of torpor without any damage. The mechanisms underlying the entrance into and arousal from torpor state are not fully clear. However, the central nervous system (CNS) is supposed to be a fundamental key player in its regulation (Heller, 1979). This presumes that brain function is maintained under extreme hypothermia during deep torpor, though the membrane potential of neurons, the properties of ion channels and receptors, the efficiency of synaptic transmission and the conduction velocity of neuronal activity are strongly dependent on temperature, and significant, functional disruptions are already observed few degrees below physiological tissue temperature (Hodgkin and Huxley, 1952; Westerfield et al., 1978; Schiff and Somjen, 1985; Kufel and Wojcik, 2018). How does the brain of a hibernator deal with the low levels of brain temperatures? What changes in neuronal signal processing occur when the brain temperature drops to values near or below freezing point? Various approaches have been used to unravel the physiology of neurons and synapses in the hibernating brain. We presently provide a compact overview on in vivo and in vitro electrophysiological data as well as histochemical, molecular and biochemical results comprising the evaluation of activity markers. We refer to both earlier and more recent data, thereby focusing on the temperature-dependency of neuronal activity during hibernation. Structural plasticity which underlies functional changes (e.g., synapse and spine degradation, protein synthesis) is not considered here, but was intensively discussed in two recent reviews (Arendt and Bullmann, 2013; Horowitz and Horwitz, 2019).
Brain activity was reported to dramatically change during hibernation. Electroencephalograms (EEGs) that serve as a measure of synchronous firing of a population of neurons were nearly isoelectric (i.e., no significant changes in voltages) in hibernating ground squirrels and hamsters at lowest body temperature during torpor state (Chatfield et al., 1951; Mihailovic, 1972). Single-unit recordings obtained by chronically implanted electrodes in the lateral posterior thalamus and posterior thalamic nuclear group of ground squirrels confirmed these observations: firing rates of spontaneous neuronal activity were systematically reduced with decreasing body temperature, and neurons eventually stopped firing at body temperatures of 15–18°C, remained silent for 10–28 h (deep torpor), and only began firing again when body temperature increased (Krilowicz et al., 1988). Along with the reduction in firing rate, the waveform of the action potential dramatically changed with amplitude becoming smaller and spike width being increased at lower body temperatures (Krilowicz et al., 1989). These data imply that neuronal activity in hibernating animals is highly dependent on body and brain temperature.
Although electrical activity is greatly reduced and almost silent at the lowest body temperature in torpor, this does not mean that information processing is suspended. Rather, sensory stimulation was shown to elicit specific responses in the CNS, even at very low body temperatures (<10°C). Peripheral stimulation of hibernating hamsters led to electrical responses in the cerebral cortex at a body temperature of 9.1°C (Chatfield et al., 1951). Similar observations were made in the ground squirrel where touching the torpid animal with a glass rod induced activity in the brainstem reticular formation, partly followed by arousal of the animals (Strumwasser, 1959). Also, acoustic stimulation of ground squirrels was reported to evoke a behavioral response or even a complete arousal (Strumwasser, 1959). Auditory brainstem recordings (ABRs) in ground squirrels during arousal revealed that acoustic stimulation indeed elicits stimulus-driven responses even at body temperatures as low as 9.8°C (Hamill et al., 1989). In the woodchuck, auditory stimulation resulted in specific ABR responses, although only waves 1 and 2 (representing the 8th nerve and the cochlear nucleus) were detectable at lowest body temperature (Katbamna et al., 1992). These data imply that peripheral nerves and primary central brain regions might be more resistant to hypothermia-induced activity changes compared to higher central brain regions.
Reduction of brain activity as the animal enters torpor follows a regional pattern, with EEG activity being reduced first in the neocortex and then in the reticular formation, followed by thalamocortical structures and the limbic system, with activity ceasing last in the hippocampus, if at all. During arousal, activity reappears and increases in reverse order: i.e., first in the limbic system (hippocampus) and then in the hypothalamus, thalamus, reticular formation, and cortex (South et al., 1968; Mihailovic, 1972; Heller, 1979). Notably, the neocortex is the first structure where activity is depressed during entrance into torpor and the last to regain activity on arousal, a finding that has focused studies on information processing during torpor to subcortical brain regions.
Taken together, in vivo studies (Figure 1) convincingly show that although neuronal activity is attenuated throughout the brain in torpor, some brain regions are more active than others. These data led to the hypothesis that the limbic system-midbrain circuitry (interconnections between the limbic system, hypothalamus and brainstem reticular formation) contributes to neural control of entrance into and arousal from torpor (Heller, 1979; Beckman and Stanton, 1982).
Figure 1. Temperature-dependent changes in neuronal activity during the hibernation cycle. Changes in body temperature during one torpor-arousal cycle are schematically illustrated in the upper graph.During torpor, body temperature is regulated at <10°C, but is generally ~2°C warmer than the ambient temperature when ambient temperature is >0°C. When ambient temperature falls below 0°C, central nervous system (CNS) signals activate thermogenic mechanisms and body temperature remains regulated near ~1°C (Richter et al., 2015). During entrance, torpor, and arousal, significant changes in neuronal activity occur. These changes have been evaluated by in vivo and in vitro electrophysiology as well as by documentation of c-Fos expression profiles and glucose utilization rate. Firing rates decrease; action potentials become smaller and broader with decreasing temperature during entrance into torpor; and they fully recover with increasing body temperature during arousal. Below 15–18°C, spontaneous activity ceases in vivo, but sensory stimulation leads to detectable responses in the CNS, even below 10°C body temperature. Accordingly, in vitro electrophysiology has shown: (i) cessation of spontaneous and electrically evoked activity at <5–16.6°C; and (ii) loss of LTP in the hippocampus at <15°C. The temperature-dependent changes in neuronal activity could be confirmed by equivalent changes in the expression of the activity marker c-Fos and reduced levels of glucose utilization. Abbreviations: AP, action potential; EEG, electroencephalogram; LTP, long-term potentiation; MPOA, medial preoptic area; SCN, suprachiasmatic nucleus.
Over the past 20 years, there has been a shift from electrophysiological in vivo studies to in vitro studies. In vitro approaches (brain slices) have the advantage that cellular mechanisms of neuronal activity in specific types of neurons can be more easily studied. Horowitz and colleagues (Hooper et al., 1985; Eckerman et al., 1990; Horrigan and Horowitz, 1990; Krelstein and Horowitz, 1990; Krelstein et al., 1990; Horowitz and Horwitz, 2019) and Igelmund and colleagues (Igelmund and Heinemann, 1995; Igelmund, 1995; Spangenberger et al., 1995) were pioneers investigating the temperature-dependent properties of CA1 hippocampal pyramidal neurons in brain slices. This early work included the finding that generation of long-term potentiation (LTP) in hamster brain slices is strongly dependent on temperature: i.e., it can be readily elicited above 20°C but not below 15°C (e.g., Krelstein et al., 1990). Similar observations were made for hippocampal long-term depression (LTD; Arant et al., 2011), leading to the view that neuroplasticity mechanisms contribute to hippocampal memory formation in euthermic but not in hibernating hamsters. That is, hamster LTP and LTD generation in CA1 hippocampal pyramidal cells appear silenced at and below 15°C (see Bronson et al., 2006; Hamilton et al., 2017) albeit CA1 hippocampal pyramidal cells continue to respond to afferent signals and generate action potentials at low slice temperatures.
Notably, at low slice temperatures, excitability of CA1 pyramidal neurons can still be increased by serotonin (Horrigan and Horowitz, 1990) and histamine (Nikmanesh et al., 1996; Hamilton et al., 2017). These studies implied that neuromodulators increased firing rates in specific classes of neurons so they could more effectively contribute to signal processing in torpor. In addition, in vivo histamine infusion into hippocampi of hibernating ground squirrels prolonged hibernation bouts (Sallmen et al., 2003b). Thus, in vivo studies and in vitro slice studies imply that histamine is an effective neuromodulator in hippocampal pyramidal neurons at low temperatures. Further support of a role for histamine in torpor was the observation of increased expression of histamine H1 and H2 receptors in the hippocampi of hibernating compared to euthermic ground squirrels (Sallmen et al., 2003a). Taken together, these data indicate that histaminergic modulation affects limbic system-midbrain circuitry in torpor. Specifically, histamine enhancement of CA1 pyramidal cell excitability may lead to inhibition of neurons in the brainstem reticular formation and prolongation of torpor bouts (Sallmen et al., 2003a; Hamilton et al., 2017).
Hibernating species have adaptations that greatly increase their tolerance to glucose and oxygen deprivation as first reported in the major study by Frerichs and Hallenbeck (1998), fully confirmed by Drew and colleagues (e.g., Ross et al., 2006), and reflected in sustained hamster hippocampal neural activity (Mikhailova et al., 2016). Still, it needs to be considered that ketones are preferred as energy supply in the brain during torpor (Krilowicz, 1985; Andrews et al., 2009). In addition, hippocampal slices of hibernating ground squirrels after 24 h in culture were shown to be more resistant to high doses of NMDA and KCl in comparison to slices of euthermic and non-hibernating animals (Ross et al., 2006). Also, inhibition of Na+/K+ pump did not lead to increased cell death in hippocampal slices of hibernating animals (Ross et al., 2006). It was therefore proposed that ion flux and the activity of the Na+/K+ pump is highly reduced during hibernation, leading to channel arrest and saving of energy. More recent data, however, indicate that in primary neurons of the somatosensory system (dorsal root ganglion), ion gradients, and membrane potential remain stable at 10°C in torpid animals (Hoffstaetter et al., 2018). Furthermore, a modification of NMDA receptor function was discussed to prevent neurons from tonic depolarization and exocytotoxic levels of intracellular calcium, which is presumed to be a consequence of inhibition of Na+/K+ pump and decrease of Na+ gradients (Ross et al., 2006; Zhao et al., 2006). In this context, it was proposed that the ion composition of the cerebrospinal fluid might change during hibernation to facilitate the depression of neuronal and synaptic activity (Igelmund and Heinemann, 1995; Igelmund, 1995).
Although most of the earlier and more recent in vitro studies focused only on the hippocampus, the influence of hypothermia in a hibernating species was also investigated in neurons of the medial preoptic area (MPOA), the suprachiasmatic nucleus (SCN) and the nucleus tractus solitarius (NTS), all of which are brain regions potentially being involved in neuronal control of hibernation. The MPOA controls thermoregulation and was considered to be especially important for the entrance into hibernation (Satinoff, 1967; Hashimoto et al., 1998). Consistent with the results obtained in the hippocampus, spontaneous neuronal activity of MPOA neurons recorded extracellularly in hamster brain slices significantly changed with decreasing temperature, resulting in reduced firing rate and spike amplitude as well as increased spike width (Hashimoto et al., 1998). Almost half of the recorded neurons (42%) were still active below 10°C with an average cutoff temperature of 4.9°C. Neurons of the rat MPOA exhibited similar results with 40% of neurons being active below 10°C, though the average cutoff temperature of 7.9°C was significantly higher compared to hamsters (Hashimoto et al., 1998). Extracellularly recorded spontaneous activity in the SCN also revealed temperature-dependent changes of neuronal activity. The majority of neurons were warm-sensitive, i.e., their firing rate decreased with decreasing temperature and the minimum temperature at which activity could be observed was 16.6°C (Miller et al., 1994). The NTS is located in the brainstem and receives peripheral input from the cardiovascular and pulmonary systems. Its 2nd order neurons support cardiovascular and respiratory reflexes (Taylor et al., 1999). Whole-cell patch-clamp recordings showed that these neurons generate less spikes with smaller amplitude and increased spike width after current injection at 15°C compared to 33°C. Similar observations were made for synaptically-driven activity (miniature and evoked EPSCs; Sekizawa et al., 2012). A very recent precise study of Hoffstaetter et al. (2018) presented whole-cell patch-clamp recordings of dorsal root ganglion neurons isolated from torpid squirrels, suggesting that the neuronal activity of these primary neurons of the somatosensory system is less sensitive to temperature-dependent changes than are neurons isolated from active squirrels. These results clearly demonstrate that action potentials could be generated through current injection at 10°C, though firing pattern changed from tonic to irregular or single spiking. The authors also showed that the membrane potential and input resistance was not affected at 10°C suggesting that ionic gradients and electric potentials are maintained in the dorsal root ganglion during hibernation.
Taken together, in vitro experiments are consistent with the in vivo experiments cited in the preceding section indicating that neural activity is markedly decreased in torpor. They further suggest that within a single neuron, some cellular mechanisms may be silenced in torpor (e.g., LTP and LTD), that firing rates in one type of neuron may decrease more rapidly than in other types within a specific nucleus (e.g., the NTS), and that neurons have protective adaptations sustaining neural activity in torpor.
In the early 1990s, evaluation of gene and protein expression of c-Fos, a proto-oncogene and immediate early gene product that is expressed upon neuronal activation was introduced as a new, innovative approach for indirect assessment of brain activity (Hoffman et al., 1993). Northern analysis or in situ hybridization of c-Fos RNA as well as immunhistochemical localization of c-Fos protein in brain slices have been proven as suitable tools to analyze neuronal activity. C-Fos RNA levels estimated by Northern analysis were reported to undergo dramatic changes throughout the hibernation cycle in virtually all parts of the brain, including hypothalamus, thalamus, cortex, midbrain, pons and medulla. In general, in euthermic animals and in early torpor, c-Fos RNA levels are low, slightly increase during deep torpor, peak in early arousal, and finally drop to low levels again in late arousal animals (Bitting et al., 1994; O’Hara et al., 1997, 1999). C-Fos in situ hybridization in coronal brain sections of ground squirrels helped to further specify these observations by neuroanatomical identification of relevant brain regions. Strong changes in c-Fos mRNA levels during the different phases of hibernation were found in the SCN, in the MPOA, and in the reticular thalamic nucleus (Bitting et al., 1994; Bratincsák et al., 2007). In the SCN and the MPOA, c-Fos mRNA changes are in accordance with the temporal profile of c-Fos expression revealed by Northern Analysis, confirming low levels of c-Fos expression during early torpor. In contrast, the reticular thalamic nucleus, which controls the communication between thalamus and cortex, exhibited an opposed profile with high c-Fos mRNA expression in the torpor state (Bratincsák et al., 2007). This is thought to be linked to inhibition of cortical activity supporting maintenance of torpor. Although this view is supported by low c-Fos levels (Bratincsák et al., 2007), there is increasing evidence that c-Fos might not be a reliable marker for neuronal activity during hibernation because transcription of genes and protein synthesis are profoundly depressed in deep torpor (van Breukelen and Martin, 2001, 2002).
A more direct approach to assess neuronal activity during hibernation is estimation of glucose metabolism. The glucose utilization rate in deep torpor is reduced to 1%–2% of the values of active animals (Frerichs et al., 1995). These data clearly confirm that neuronal activity is low in torpor, although, there are regional variations in the change of glucose uptake in the brain across the hibernation bout (Kilduff et al., 1990).
While in in vivo studies, the physiological state of the hibernating brain is assumed to be preserved (except in the case of anesthesia usage), in vitro protocols only approximately simulate the physiological state which is—at the current state of knowledge—far from being standardized. One critical point is the preservation of brain temperature during all steps of preparation and handling of slices prior to recording (Pakhotin et al., 1993). Another point is that brains were often isolated from aroused/active hibernators with hypothermia data collected during exposure of brain slices to cold temperatures. There might be substantial differences when performing the same experiment in brains isolated from torpid animals (Hoffstaetter et al., 2018). Also, the composition of ACSF seems to be critical, because it may change across hibernation cycle (Pakhotin et al., 1993; Igelmund, 1995). Still, in vitro electrophysiology is indispensable for identifying the mechanisms underlying neuronal excitability in the hibernating brain. A comparable insight into cellular processes can hardly be achieved with common in vivo electrophysiological recording techniques (or indirect techniques). To compensate for hibernation-induced limitations in standard in vitro electrophysiology, a future alternative might be in vivo intracellular single-unit recordings in unanesthetized hibernators chronically implanted with specific recording devices that have been successfully conducted in rodents (Lee et al., 2006, 2009; Petersen, 2017).
The various approaches that have been used to characterize neuronal activity in the hibernating brain agree in one aspect: brain activity dramatically declines with decreasing body temperature (Figure 1). Neurons draw on glucose to support neuronal activity and in torpor, CNS glucose utilization has been lowered to 1%–2% of that of active animals. Still, the putatively most intriguing question (precisely what percentage of CNS neurons in various brain regions essentially suspend operating and seldom generate few action potentials in deep torpor?) evades a direct answer due to limitations of currently available experimental preparations and techniques. Present data imply that a low level of activity is maintained even in deep torpor, with many neurons generating action potentials infrequently. Interestingly, a variety of observations, from 2-deoxyglucose staining to single-unit recording indicate that neural activity varies in different brain regions and cell types. Moreover, in the hypothalamus and brainstem, neuronal activity, while low, maintains homeostatic temperature and cardiorespiratory regulation and responsiveness to alarm signals. For example, in deep torpor, a slow decline in ambient temperature to −30°C evokes a steady increase in activation of brown adipose tissue thermogenesis, keeping body temperature at ~1°C in Arctic ground squirrels (Richter et al., 2015) whereas a rapid fall in ambient temperature (an alarm signal) arouses the squirrel from hibernation. CNS regulation of the cardiorespiratory system ensures that all cells throughout the body receive oxygen, but not at a rate that would waste energy and defeat the entire advantage of hibernation. Taken together, despite a fundamental constraint on central signal processing in deep torpor (low brain temperature, Figure 1), evolutionary adaptations have configured critical subcortical signal processing (Table 1) to dependably support CNS function in a cold environment where food is scarce.
Both authors have made a substantial, direct and intellectual contribution to the work, and approved it for publication.
The authors acknowledge support from the German Research Foundation (DFG) and Leipzig University within the program of Open Access Publishing.
The authors declare that the research was conducted in the absence of any commercial or financial relationships that could be construed as a potential conflict of interest.
We are grateful to the reviewers for valuable comments which greatly improved the manuscript.
Andrews, M. T., Russeth, K. P., Drewes, L. R., and Henry, P.-G. (2009). Adaptive mechanisms regulate preferred utilization of ketones in the heart and brain of a hibernating mammal during arousal from torpor. Am. J. Physiol. Regul. Integr. Comp. Physiol. 296, R383–R393. doi: 10.1152/ajpregu.90795.2008
Arant, R. J., Goo, M. S., Gill, P. D., Nguyen, Y., Watson, K. D., Hamilton, J. S., et al. (2011). Decreasing temperature shifts hippocampal function from memory formation to modulation of hibernation bout duration in Syrian hamsters. Am. J. Physiol. Regul. Integr. Comp. Physiol. 301, R438–R847. doi: 10.1152/ajpregu.00016.2011
Arendt, T., and Bullmann, T. (2013). Neuronal plasticity in hibernation and the proposed role of the microtubule-associated protein tau as a “master switch” regulating synaptic gain in neuronal networks. Am. J. Physiol. Regul. Integr. Comp. Physiol. 305, R478–R489. doi: 10.1152/ajpregu.00117.2013
Beckman, A. L., and Stanton, T. L. (1982). “Properties of the CNS during the state of hibernation,” in Neural Basis of Behavior, ed. A. Beckman (Jamaica, NY: Spectrum Publications), 19–45.
Beckman, A. L., Satinoff, E., and Stanton, T. L. (1976). Characterization of midbrain component of the trigger for arousal from hibernation. Am. J. Physiol. 230, 368–375. doi: 10.1152/ajplegacy.1976.230.2.368
Bitting, L., Sutin, E. L., Watson, F. L., Leard, L. E., O’Hara, B. F., Heller, H. C., et al. (1994). C-fos mRNA increases in the ground squirrel suprachiasmatic nucleus during arousal from hibernation. Neurosci. Lett. 165, 117–121. doi: 10.1016/0304-3940(94)90723-4
Bratincsák, A., McMullen, D., Miyake, S., Tóth, Z. E., Hallenbeck, J. M., and Palkovits, M. (2007). Spatial and temporal activation of brain regions in hibernation: c-fos expression during the hibernation bout in thirteen-lined ground squirrel. J. Comp. Neurol. 505, 443–458. doi: 10.1002/cne.21507
Bronson, N. W., Piro, J. B., Hamilton, J. S., Horowitz, J. M., and Horwitz, B. A. (2006). Temperature modifies potentiation but not depotentiation in bidirectional hippocampal plasticity of Syrian hamsters (Mesocricetus auratus). Brain Res. 1098, 61–70. doi: 10.1016/j.brainres.2006.03.125
Chatfield, P. O., Lyman, C. P., and Purpura, D. P. (1951). The effects of temperature on the spontaneous and induced electrical activity in the cerebral cortex of the golden hamster. Electroencephalogr. Clin. Neurophysiol. 3, 225–230. doi: 10.1016/0013-4694(51)90015-6
Eckerman, P., Scharruhn, K., and Horowitz, J. M. (1990). Effects of temperature and acid-base state on hippocampal population spikes in hamsters. Am. J. Physiol. 258, R1140–R1146. doi: 10.1152/ajpregu.1990.258.5.r1140
Florant, G. L., and Heller, H. C. (1977). CNS regulation of body temperature in euthermic and hibernating marmots (Marmota flaviventris). Am. J. Physiol. 232, R203–R208. doi: 10.1152/ajpregu.1977.232.5.r203
Frerichs, K. U., Dienel, G. A., Cruz, N. F., Sokoloff, L., and Hallenbeck, J. M. (1995). Rates of glucose utilization in brain of active and hibernating ground squirrels. Am. J. Physiol. 268, R445–R453. doi: 10.1152/ajpregu.1995.268.2.r445
Frerichs, K. U., and Hallenbeck, J. M. (1998). Hibernation in ground squirrels induces state and species-specific tolerance to hypoxia and aglycemia: an in vitro study in hippocampal slices. J. Cereb. Blood Flow Metab. 18, 168–175. doi: 10.1097/00004647-199802000-00007
Hamill, N. J., McGinn, M. D., and Horowitz, J. M. (1989). Auditory brainstem responses in ground squirrels arousing from hibernation. J. Comp. Physiol. B Biochem. Syst. Environ. Physiol. 159, 167–172. doi: 10.1007/bf00691737
Hamilton, J. S., Chau, S. M., Malins, K. J., Ibanez, G. G., Horowitz, J. M., and Horwitz, B. A. (2017). Syrian hamster neuroplasticity mechanisms fail as temperature declines to 15 degrees C, but histaminergic neuromodulation persists. J. Comp. Physiol. B 187, 779–791. doi: 10.1007/s00360-017-1078-5
Hashimoto, M., Schmid, H. A., Ohwatari, N., and Pleschka, K. (1998). Spontaneous activity of preoptic neurons in slice preparations of the hypothalamus of European hamsters (Cricetus cricetus) and Wistar rats under different states of hypothermia. Cryobiology 37, 254–262. doi: 10.1006/cryo.1998.2122
Heller, H. C. (1979). Hibernation: neural aspects. Annu. Rev. Physiol. 41, 305–321. doi: 10.1146/annurev.ph.41.030179.001513
Hodgkin, A. L., and Huxley, A. F. (1952). A quantitative description of membrane current and its application to conduction and excitation in nerve. J. Physiol. 117, 500–544. doi: 10.1113/jphysiol.1952.sp004764
Hoffman, G. E., Smith, M. S., and Verbalis, J. G. (1993). c-Fos and related immediate early gene products as markers of activity in neuroendocrine systems. Front. Neuroendocrinol. 14, 173–213. doi: 10.1006/frne.1993.1006
Hoffstaetter, L. J., Mastrotto, M., Merriman, D. K., Dib-Hajj, S. D., Waxman, S. G., Bagriantsev, S. N., et al. (2018). Somatosensory neurons enter a state of altered excitability during hibernation. Curr. Biol. 28, 2998.e3–3004.e3. doi: 10.1016/j.cub.2018.07.020
Hooper, D. C., Martin, S. M., and Horowitz, J. M. (1985). Temperature effects on evoked potentials of hippocampal slices from euthermic chipmunks, hamsters and rats. J. Therm. Biol. 10, 35–40. doi: 10.1016/0306-4565(85)90008-7
Horowitz, J. M., and Horwitz, B. A. (2019). Extreme neuroplasticity of hippocampal CA1 pyramidal neurons in hibernating mammalian species. Front. Neuroanat. 13:9. doi: 10.3389/fnana.2019.00009
Horrigan, D. J., and Horowitz, J. M. (1990). Thermal dependence of serotonergic modulation of neural activity in the hamster. J. Comp. Physiol. A 167, 79–88. doi: 10.1007/bf00192408
Horwitz, B. A., Chau, S. M., Hamilton, J. S., Song, C., Gorgone, J., Saenz, M., et al. (2013). Temporal relationships of blood pressure, heart rate, baroreflex function and body temperature change over a hibernation bout in Syrian hamsters. Am. J. Physiol. Regul. Integr. Comp. Physiol. 305, R759–R768. doi: 10.1152/ajpregu.00450.2012
Igelmund, P. (1995). Modulation of synaptic transmission at low temperatures by hibernation-related changes in ionic microenvironment in hippocampal slices of golden hamsters. Cryobiology 32, 334–343. doi: 10.1006/cryo.1995.1034
Igelmund, P., and Heinemann, U. (1995). Synaptic transmission and paired-pulse behaviour of CA1 pyramidal cells in hippocampal slices from a hibernator at low temperature: importance of ionic environment. Brain Res. 689, 9–20. doi: 10.1016/0006-8993(95)00524-t
Ikeno, T., Williams, C. T., Buck, C. L., Barnes, B. M., and Yan, L. (2017). Clock gene expression in the suprachiasmatic nucleus of hibernating arctic ground squirrels. J. Biol. Rhythms 32, 246–256. doi: 10.1177/0748730417702246
Katbamna, B., Thodi, C., Senturia, J. B., and Metz, D. A. (1992). Auditory-evoked brainstem responses in the hibernating woodchuck Marmota monax. Comp. Biochem. Physiol. Comp. Physiol. 102, 513–517. doi: 10.1016/0300-9629(92)90203-3
Kilduff, T. S., Miller, J. D., Radeke, C. M., Sharp, F. R., and Heller, H. C. (1990). 14C-2-deoxyglucose uptake in the ground squirrel brain during entrance to and arousal from hibernation. J. Neurosci. 10, 2463–2475. doi: 10.1523/JNEUROSCI.10-07-02463.1990
Krelstein, M. S., and Horowitz, J. M. (1990). Tetanus during a high extracellular calcium pulse overrides the block of long-term potentiation seen at 20 degrees C in the hamster hippocampal slice. Brain Res. 536, 105–113. doi: 10.1016/0006-8993(90)90014-3
Krelstein, M. S., Thomas, M. P., and Horowitz, J. M. (1990). Thermal effects on long-term potentiation in the hamster hippocampus. Brain Res. 520, 115–122. doi: 10.1016/0006-8993(90)91696-e
Krilowicz, B. L. (1985). Ketone body metabolism in a ground squirrel during hibernation and fasting. Am. J. Physiol. 249, R462–R470. doi: 10.1152/ajpregu.1985.249.4.r462
Krilowicz, B. L., Edgar, D. M., and Heller, H. C. (1989). Action potential duration increases as body temperature decreases during hibernation. Brain Res. 498, 73–80. doi: 10.1016/0006-8993(89)90400-9
Krilowicz, B. L., Glotzbach, S. F., and Heller, H. C. (1988). Neuronal activity during sleep and complete bouts of hibernation. Am. J. Physiol. 255, R1008–R1019. doi: 10.1152/ajpregu.1988.255.6.r1008
Kufel, D. S., and Wojcik, G. M. (2018). Analytical modelling of temperature effects on an AMPA-type synapse. J. Comput. Neurosci. 44, 379–391. doi: 10.1007/s10827-018-0684-x
Lee, A. K., Epsztein, J., and Brecht, M. (2009). Head-anchored whole-cell recordings in freely moving rats. Nat. Protoc. 4, 385–392. doi: 10.1038/nprot.2009.5
Lee, A. K., Manns, I. D., Sakmann, B., and Brecht, M. (2006). Whole-cell recordings in freely moving rats. Neuron 51, 399–407. doi: 10.1016/j.neuron.2006.07.004
Mihailovic, L. J. T. (1972). “Cortical and subcortical electrical activity in hibernation and hypothermia,” in Hibernation and Hypothermia, Perspectives and Challenges, eds F. E. South, J. P. Hannon, J. R. Willis, E. T. Pengelley and N. R. Alpert (Amsterdam: Elsevier), 487–534.
Mikhailova, A., Mack, J., Vitagliano, N., Hamilton, J. S., Horowitz, J. M., and Horwitz, B. A. (2016). Recovery of Syrian hamster hippocampal signaling following its depression during oxygen-glucose deprivation is enhanced by cold temperatures and by hibernation. Neurosci. Lett. 621, 98–103. doi: 10.1016/j.neulet.2016.04.011
Miller, J. D., Cao, V. H., and Heller, H. C. (1994). Thermal effects on neuronal activity in suprachiasmatic nuclei of hibernators and nonhibernators. Am. J. Physiol. 266, R1259–R1266. doi: 10.1152/ajpregu.1994.266.4.r1259
Morrison, S. F., Madden, C. J., and Tupone, D. (2014). Central neural regulation of brown adipose tissue thermogenesis and energy expenditure. Cell Metab. 19, 741–756. doi: 10.1016/j.cmet.2014.02.007
Nedergaard, J., and Cannon, B. (1990). Mammalian hibernation. Philos. Trans. R. Soc. Lond. B Biol. Sci. 326, 669–685, discussion 685–686. doi: 10.1098/rstb.1990.0038
Nikmanesh, F. G., Spangenberger, H., and Igelmund, P. (1996). Histamine enhances synaptic transmission in hippocampal slices from hibernating and warm-acclimated Turkish hamsters. Neurosci. Lett. 210, 119–120. doi: 10.1016/0304-3940(96)12672-0
Nurnberger, F. (1995). The neuroendocrine system in hibernating mammals: present knowledge and open questions. Cell Tissue Res. 281, 391–412. doi: 10.1007/s004410050437
O’Hara, B. F., Watson, F. L., Andretic, R., Wiler, S. W., Young, K. A., Bitting, L., et al. (1997). Daily variation of CNS gene expression in nocturnal vs. diurnal rodents and in the developing rat brain. Mol. Brain Res. 48, 73–86. doi: 10.1016/s0169-328x(97)00084-3
O’Hara, B. F., Watson, F. L., Srere, H. K., Kumar, H., Wiler, S. W., Welch, S. K., et al. (1999). Gene expression in the brain across the hibernation cycle. J. Neurosci. 19, 3781–3790. doi: 10.1523/JNEUROSCI.19-10-03781.1999
Pakhotin, P. I., Pakhotina, I. D., and Belousov, A. B. (1993). The study of brain slices from hibernating mammals in vitro and some approaches to the analysis of hibernation problems in vivo. Prog. Neurobiol. 40, 123–161. doi: 10.1016/0301-0082(93)90021-j
Petersen, C. C. H. (2017). Whole-cell recording of neuronal membrane potential during behavior. Neuron 95, 1266–1281. doi: 10.1016/j.neuron.2017.06.049
Richter, M. M., Williams, C. T., Lee, T. N., Tøien, Ø., Florant, G. L., Barnes, B. M., et al. (2015). Thermogenic capacity at subzero temperatures: how low can a hibernator go? Physiol. Biochem. Zool. 88, 81–89. doi: 10.1086/679591
Ross, A. P., Christian, S. L., Zhao, H. W., and Drew, K. L. (2006). Persistent tolerance to oxygen and nutrient deprivation and N-methyl-D-aspartate in cultured hippocampal slices from hibernating Arctic ground squirrel. J. Cereb. Blood Flow Metab. 26, 1148–1156. doi: 10.1038/sj.jcbfm.9600271
Sallmen, T., Lozada, A. F., Anichtchik, O. V., Beckman, A. L., Leurs, R., and Panula, P. (2003a). Changes in hippocampal histamine receptors across the hibernation cycle in ground squirrels. Hippocampus 13, 745–754. doi: 10.1002/hipo.10120
Sallmen, T., Lozada, A. F., Beckman, A. L., and Panula, P. (2003b). Intrahippocampal histamine delays arousal from hibernation. Brain Res. 966, 317–320. doi: 10.1016/s0006-8993(02)04235-x
Satinoff, E. (1967). Disruption of hibernation caused by hypothalamic lesions. Science 155, 1031–1033. doi: 10.1126/science.155.3765.1031
Schiff, S. J., and Somjen, G. G. (1985). The effects of temperature on synaptic transmission in hippocampal tissue slices. Brain Res. 345, 279–284. doi: 10.1016/0006-8993(85)91004-2
Sekizawa, S.-I., Horowitz, J. M., Horwitz, B. A., and Chen, C.-Y. (2012). Realignment of signal processing within a sensory brainstem nucleus as brain temperature declines in the Syrian hamster, a hibernating species. J. Comp. Physiol. A Neuroethol. Sens. Neural Behav. Physiol. 198, 267–282. doi: 10.1007/s00359-011-0706-x
South, F. E., Breazile, J. E., Dellman, H. D., and Epperly, A. D. (1968). “Sleep, hibernation, and hypothermia in the yellowbellied marmot (M. flaviventris),” in Depressed Metabolism, eds X. J. Musacchia and J. E. Saunders (New York, NY: Elsevier), 277–312.
Spangenberger, H., Nikmanesh, F. G., and Igelmund, P. (1995). Long-term potentiation at low temperature is stronger in hippocampal slices from hibernating Turkish hamsters compared to warm-acclimated hamsters and rats. Neurosci. Lett. 194, 127–129. doi: 10.1016/0304-3940(95)11723-a
Strumwasser, F. (1959). Regulatory mechanisms, brain activity and behavior during deep hibernation in the squirrel, Citellus beecheyi. Am. J. Physiol. 196, 23–30. doi: 10.1152/ajplegacy.1958.196.1.23
Taylor, E. W., Jordan, D., and Coote, J. H. (1999). Central control of the cardiovascular and respiratory systems and their interactions in vertebrates. Physiol. Rev. 79, 855–916. doi: 10.1152/physrev.1999.79.3.855
van Breukelen, F., and Martin, S. L. (2001). Translational initiation is uncoupled from elongation at 18 degrees C during mammalian hibernation. Am. J. Physiol. Regul. Integr. Comp. Physiol. 281, R1374–R1379. doi: 10.1152/ajpregu.2001.281.5.r1374
van Breukelen, F., and Martin, S. L. (2002). Reversible depression of transcription during hibernation. J. Comp. Physiol. B Biochem. Syst. Environ. Physiol. 172, 355–361. doi: 10.1007/s00360-002-0256-1
Webb, C. L., and Milsom, W. K. (2017). Effects of low temperature on breathing pattern and ventilatory responses during hibernation in the golden-mantled ground squirrel. J. Comp. Physiol. 187, 793–802. doi: 10.1007/s00360-017-1079-4
Westerfield, M., Joyner, R. W., and Moore, J. W. (1978). Temperature-sensitive conduction failure at axon branch points. J. Neurophysiol. 41, 1–8. doi: 10.1152/jn.1978.41.1.1
Williams, C. T., Radonich, M., Barnes, B. M., and Buck, C. L. (2017). Seasonal loss and resumption of circadian rhythms in hibernating arctic ground squirrels. J. Comp. Physiol. B 187, 693–703. doi: 10.1007/s00360-017-1069-6
Keywords: hibernation, hypothermia, neuronal activity, electrophysiology, c-Fos, firing rate homeostasis
Citation: Sonntag M and Arendt T (2019) Neuronal Activity in the Hibernating Brain. Front. Neuroanat. 13:71. doi: 10.3389/fnana.2019.00071
Received: 27 February 2019; Accepted: 26 June 2019;
Published: 09 July 2019.
Edited by:
Marcello Rosa, Monash University, AustraliaReviewed by:
Oscar Herreras, Spanish National Research Council (CSIC), SpainCopyright © 2019 Sonntag and Arendt. This is an open-access article distributed under the terms of the Creative Commons Attribution License (CC BY). The use, distribution or reproduction in other forums is permitted, provided the original author(s) and the copyright owner(s) are credited and that the original publication in this journal is cited, in accordance with accepted academic practice. No use, distribution or reproduction is permitted which does not comply with these terms.
*Correspondence: Mandy Sonntag, bWFuZHkuc29ubnRhZ0BtZWRpemluLnVuaS1sZWlwemlnLmRl
Disclaimer: All claims expressed in this article are solely those of the authors and do not necessarily represent those of their affiliated organizations, or those of the publisher, the editors and the reviewers. Any product that may be evaluated in this article or claim that may be made by its manufacturer is not guaranteed or endorsed by the publisher.
Research integrity at Frontiers
Learn more about the work of our research integrity team to safeguard the quality of each article we publish.