- Department of Biology, University of Maryland, College Park, MD, United States
The thalamocortical circuit is of central importance in relaying information to the cortex. In development, subplate neurons (SPNs) form an integral part of the thalamocortical pathway. These early born cortical neurons are the first neurons to receive thalamic inputs and excite neurons in the cortical plate. This feed-forward circuit topology of SPNs supports the role of SPNs in shaping the formation and plasticity of thalamocortical connections. Recently it has been shown that SPNs also receive inputs from the developing cortical plate and project to the thalamus. The cortical inputs to SPNs in early ages are mediated by N-methyl-D-aspartate (NMDA)-receptor only containing synapses while at later ages α-amino-3-hydroxy-5-methyl-4-isoxazolepropionic acid (AMPA)-receptors are present. Thus, SPNs perform a changing integrative function over development. NMDA-receptor only synapses are crucially influenced by the resting potential and thus insults to the developing brain that causes depolarizations, e.g., hypoxia, can influence the integrative function of SPNs. Since such insults in humans cause symptoms of neurodevelopmental disorders, NMDA-receptor only synapses on SPNs might provide a crucial link between early injuries and later circuit dysfunction. We thus here review subplate associated circuits, their changing functions, and discuss possible roles in development and disease.
Introduction
The mammalian cerebral cortex is unique in that it is a laminated structure which is thought to support hierarchical information processing. The transmission of information from the thalamus to the cortex via thalamocortical circuits is of central importance for information processing in the mammalian brain. In the adult, thalamic input to the cerebral cortex mainly arrives in layer 4 but inputs to other layers can exist (Meyer et al., 2010; Bruno, 2011; Sherman, 2012, 2017; Constantinople and Bruno, 2013; Ji et al., 2016; Sun et al., 2016).
Given the laminar nature of the cerebral cortex, the development of the cortical layers is a protracted process. Curiously, the development of the cortical layers occurs in an inside-out fashion such that deeper layers are generated before superficial layers. The first postmitotic neurons from the ventricular zone and the germinative zone of the rostromedial telencephalic wall migrate radially and form the so-called preplate. Subsequent rounds of cell division give rise to the excitatory neurons of the cortical plate. Since these neurons migrate towards the pia, they divide the preplate into the superficial marginal zone and the deep subplate zone (Supèr et al., 1998; Kanold and Luhmann, 2010; Pedraza et al., 2014). Even though the subplate zone is only present in the brain transiently, it plays an important role in cortical development.
Studies both in cats and rodents have shown that subplate neurons (SPNs) are responsible for the functional maturation and segregation of the thalamocortical connections (Ghosh et al., 1990; Ghosh and Shatz, 1992; McConnell et al., 1994; Kanold et al., 2003; Tolner et al., 2012), as well as for the maturation of cortical GABAergic inhibition (Kanold and Shatz, 2006), and critical period plasticity (Kanold and Shatz, 2006). This key role of SPNs is thought to stem from the fact that SPNs are the first neurons to receive thalamic inputs (Friauf and Shatz, 1991; Hanganu et al., 2002; Higashi et al., 2002, 2005; Molnár et al., 2003; Zhao et al., 2009), respond to sensory stimuli (Wess et al., 2017) and relay this information to the developing layer 4 (L4) (Kanold, 2009; Zhao et al., 2009; Butts and Kanold, 2010; Kanold and Luhmann, 2010; Deng et al., 2017; Figure 1A). Thus, the subplate might form a functional protomap of the future cortical organization (O’Leary and Borngasser, 2006; O’Leary et al., 2007; Wess et al., 2017).
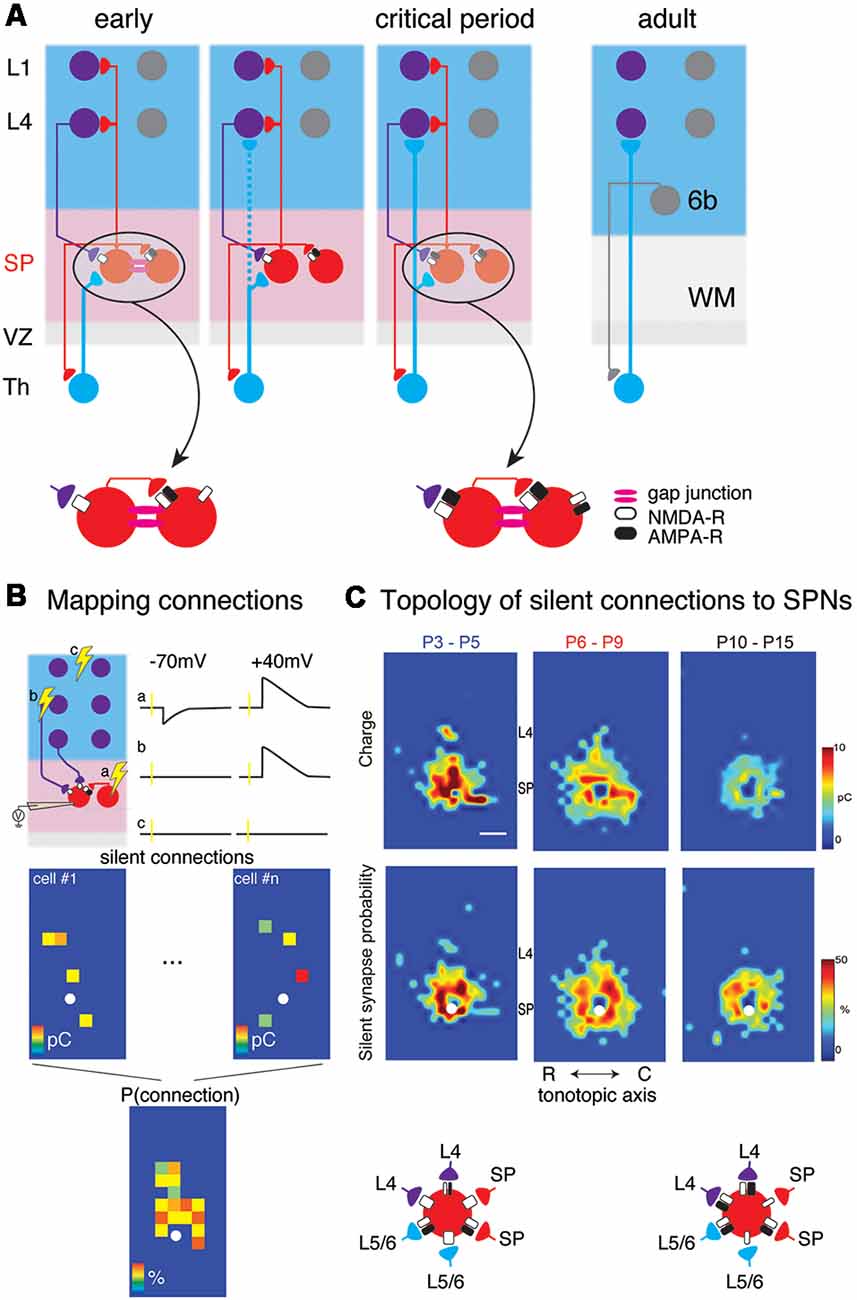
Figure 1. (A) Changing circuits between thalamus (Th), subplate (SP) and cortical layers 4 (L4) and 1 (L1). Insets show the existence of NMDA-R only “silent” synapses at early ages. (B) Cartoon illustration mapping of connections to subplate neurons (SPNs) via laser-scanning photostimulation (LSPS). Patch clamp recordings in voltage clamp are made from SPNs and locations are selectively stimulated. The SPN is recorded at −70 mV and +40 mV membrane potential at each stimulation location. Traces (a–c) indicate three potential outcomes of stimulation at the respective locations. (a) Stimulation of presynaptic locations that were connected to the SPN with AMPA and NMDARs resulted in excitatory postsynaptic currents (EPSCs) at both −70 mV and +40 mV. (b) Stimulation of presynaptic locations that were connected to the SPN with only NMDARs resulted in EPSCs at only +40 mV. (c) Stimulation of presynaptic locations that were not connected to the SPN resulted in no EPSCs at either −70 mV and +40 mV. Bottom shows calculating the spatial connection probability from maps of NMDAR-only connections (e.g., sites similar to site b). White circle indicates soma location of SPN. Maps of individual neurons are aligned to the soma and the probability of observing an EPSC is calculated for all relative spatial locations. (C) Changing topology of silent synapses over development. Shown are average connection probability and mean charge maps from LSPS experiments (from Meng et al., 2014). Cartoon below summarizes these data. At early ages, silent synapses are present between L4, L6/6, SP and SP and have a large synaptic strength. Silent synapses are most abundant at P6-P9 and at older ages, the strength of silent synapses has decreased.
Given the key position of SPNs in the thalamocortical pathway and their demonstrated role in cortical development, we here aim to review the changing circuits associated with SPNs and their possible functional relevance.
The Subplate Zone Contains a Diverse Population of Neurons
Both glutamatergic and GABAergic neurons are found in the subplate zone. The majority of the cortex projecting SPNs are glutamatergic in ferret, while some of them are positive for GABAergic interneuron markers (Finney et al., 1998). GABAergic SPNs are also found in mouse, cat, and primate (Chun and Shatz, 1989; Antonini and Shatz, 1990; Meinecke and Rakic, 1992; Higo et al., 2007; Kanold and Luhmann, 2010). SPNs have diverse dendritic and axonal morphology. Several distinct morphological types such as bitufted and monotufted horizontal, multipolar, inverted pyramidal, polymorphous, and fusiform SPNs have been identified (Mrzljak et al., 1988; Hanganu et al., 2002; Marx et al., 2017). Some SPNs have extensive dendritic trees in the cortical plate (Friauf et al., 1990; Finney et al., 1998; Clancy and Cauller, 1999; Piñon et al., 2009; Viswanathan et al., 2012), suggesting that they are integrating synaptic information from other sources. Such sources could originate locally within the cortical plate close to the SPN soma or from distant sources, e.g., other cortical or subcortical regions. Molecular analysis has shown that SPNs express a variety of molecular markers such as CTGF, Nurr1, Cplx3, as well as others (Hoerder-Suabedissen et al., 2009, 2013; Viswanathan et al., 2012). Molecularly defined classes of SPNs have been shown to have dendritic trees in the cortical plate (Viswanathan et al., 2012) suggesting that molecular cellular identity might co-vary with morphology (reviewed in Luhmann et al., 2018).
Outputs: Synaptic Targets of SPNs
While the subplate zone contains both glutamatergic and GABAergic neurons, ventricular zone derived SPNs are glutamatergic. SPNs send excitatory projections into the developing cortical plate and axons from SPNs target excitatory and GABAergic cells in L4 (Zhao et al., 2009; Deng et al., 2017; Figure 1A). Besides L4, SP axons are found in sub- as well as supragranular layers including layer 1 (L1) where they can potentially activate L1 neurons as well as apical dendrites of many other cortical cells (Clancy and Cauller, 1999; Viswanathan et al., 2017). Moreover, Cajal Retzius cells in L1 have been shown to receive GABAergic inputs from subplate (Myakhar et al., 2011), thus SPNs potentially target cells across the cortical column.
At young ages, SPNs are also coupled to each other as well as to some cortical plate neurons via gap junctions (Figure 1A; Dupont et al., 2006). In the adult cerebral cortex gap junction coupling is mostly present between GABAergic neurons (Galarreta and Hestrin, 1999, 2001, 2002; Gibson et al., 1999; Venance et al., 2000; Deans et al., 2001; Connors and Long, 2004; Butovas et al., 2006; Fukuda et al., 2006; Ma et al., 2011) while in development gap junction coupling more widespread (Niculescu and Lohmann, 2014). Gap junction coupling could synchronize neuronal activity, cause network oscillations, and contribute to circuit formation and plasticity (Peinado et al., 1993a,b; Roerig and Feller, 2000; Connors and Long, 2004; Cruikshank et al., 2005; Kotak et al., 2007; Postma et al., 2011; Niculescu and Lohmann, 2014; Yao et al., 2016). Thus, gap junctions between SPNs could synchronize local groups of SPNs and thereby amplify their effects on synaptic targets.
SPNs are also thought to pioneer the corticothalamic pathway (McConnell et al., 1994) and as such SPNs have been shown to send projections to the thalamus (Viswanathan et al., 2017; Hoerder-Suabedissen et al., 2018). The described thalamic projecting SPNs have targeted higher-order thalamic nuclei (Viswanathan et al., 2017; Hoerder-Suabedissen et al., 2018), which develop early and also support multisensory processing in early development (Henschke et al., 2018). Thus, SPNs might play a role in early multisensory integration or synchronization.
Inputs: Subplate Neurons Integrate Cortical Information via a Varying Set of Synapses
Functional sources of cortical inputs to SPNs have been identified by studies in brain slices of the developing mouse auditory cortex (Viswanathan et al., 2012; Meng et al., 2014). These studies have shown that SPNs receive excitatory AMPA receptor-mediated and GABAergic inputs from the cortical plate (Viswanathan et al., 2012). In particular, these inputs originated in deep cortical layers, but subpopulations of SPNs also received inputs from L4. SPNs receiving L4 input were more prominent after thalamocortical connections to L4 were established roughly around ear opening at ~P11 in the mouse (Figure 1C).
Besides glutamatergic and GABAergic inputs, SPNs also receive neuromodulatory inputs from a variety of sources. For example, SPNs have both acetylcholine (Ach; Hanganu et al., 2002, 2009; Hanganu and Luhmann, 2004) and serotonin receptors (Kanold and Luhmann, 2010; Liao and Lee, 2011, 2014), and cholinergic as well as serotonergic fibers are present in the developing subplate (Krmpotić-Nemanić et al., 1983; Voigt and de Lima, 1991; Nakazawa et al., 1992; Mechawar and Descarries, 2001) suggesting that they form functional circuits with SPNs.
Subplate Neurons Receive Cortical Information via Silent Synapses
Ionotropic glutamatergic neural transmission is the major form of excitatory neural transmission in the central nervous system. Presynaptic release of glutamate is sensed by AMPA receptors (AMPARs) and also by NMDA receptors (NMDARs) on the postsynaptic sites. Binding of glutamate alone is not enough to make NMDARs active. Membrane depolarization is also required due to Mg2+ block of NMDARs (Nowak et al., 1984). Thus, when glutamatergic synapses conducting currents only at depolarized membrane potential were identified, they were named as “silent synapses” (Isaac et al., 1995, 1997; Liao et al., 1995; Durand et al., 1996; Isaac, 2003). This silent synapse phenomenon is most likely due to lacking AMPARs on the postsynaptic site, but other mechanisms could exist (Kullmann and Asztely, 1998; Kullmann, 2003).
Due to the blockage by Mg2+ and lack of AMPARs in early development many glutamatergic synapses in several brain regions are mediated by silent synapses and the abundance of silent synapses in cells decreases over development (Isaac et al., 1997; Rumpel et al., 1998, 2004; Deng et al., 2017). Silent synapses can be turned into AMPAR-containing synapses through long-term potentiation protocols. Together, they support the hypothesis that neural activity unsilencing silent synapses is a mechanism for building mature neural circuits (Kerchner and Nicoll, 2008; Hanse et al., 2013). Indeed, the glutamatergic connections from layer 2/3 (L2/3) to L5 pyramidal neurons in the cortex are mediated by silent synapses during the first postnatal week. These NMDAR-only connections can be turned into AMPAR-containing connections by elevated network activity (Anastasiades and Butt, 2012).
In vitro studies in slices of neonatal mouse auditory cortex using Laser Scanning Photostimulation (LSPS; Figure 1B) showed that cortical input to SPNs were mediated by silent synapses for most of SPNs from postnatal day 3 (P3) to P15 (Meng et al., 2014), which encompasses the period from before the formation of thalamocortical synapses past the critical period. In these experiments, presynaptic neurons were activated by uncaging glutamate sequentially in a grid over the A1, while GABAergic transmission was blocked. By holding SPNs either at −70 mV or +40 mV the nature of the connection between the activated presynaptic neuron at each stimulus location and the recorded SPN could be probed. Presence of AMPARs results in excitatory postsynaptic currents (EPSCs) at both −70 mV and +40 mV (e.g., from within SP at stimulation site a in Figure 1B). In contrast, when only NMDARs are present, the connection is “silent” at −70 mV and EPSCs are only observed at +40 mV (stimulation site b in Figure 1B). By sequentially stimulating many locations to cover the extent of the slice, maps of NMDAR-only (“silent”) connections can be obtained. For each stimulation site, the size of the evoked EPSCs is quantified. By mapping multiple neurons, the probability of having NMDAR-only connections from each spatial location can be calculated (Figure 1B bottom). Mapping inputs to SPNs across age and calculating the spatial connection probability as well as the average strength of such a connection shows that circuits to SPNs change over development (Figure 1C). SPNs had connections that could either be NMDAR-only or AMPAR-containing. Overall, SPNs at young ages have silent connections mediating inputs from cortical cells located at larger distances from the SPN soma (Figure 1C). Many of these silent connections at early ages originated in L4. Comparing the abundance of silent connections, a transient increase in silent connections from the cortical plate occurred from P6 to P9 suggesting the formation of glutamatergic connections during this time period. A population analysis revealed that SPNs could be categorized into four different groups based on their functional connectivity patterns and in particular, their inputs from L4 (Meng et al., 2014; Figures 2A,B). Moreover, groups of SPNs varied in their amount and extent of intralaminar integration along the rostro-caudal direction, which in A1 is the dominant tonotopic axis. For example, SPNs in both groups 2 and 4 receive “silent” L4 input but vary in the amount of rostro-caudal integration and thus presumably in their functional integration across the frequency axis. These studies indicate that SPNs form a diverse integrative neural circuit and that the nature of integration varies with age. In particular, feedback inputs from L4 are mediated by NMDARs at early ages but contain AMPARs at older ages.
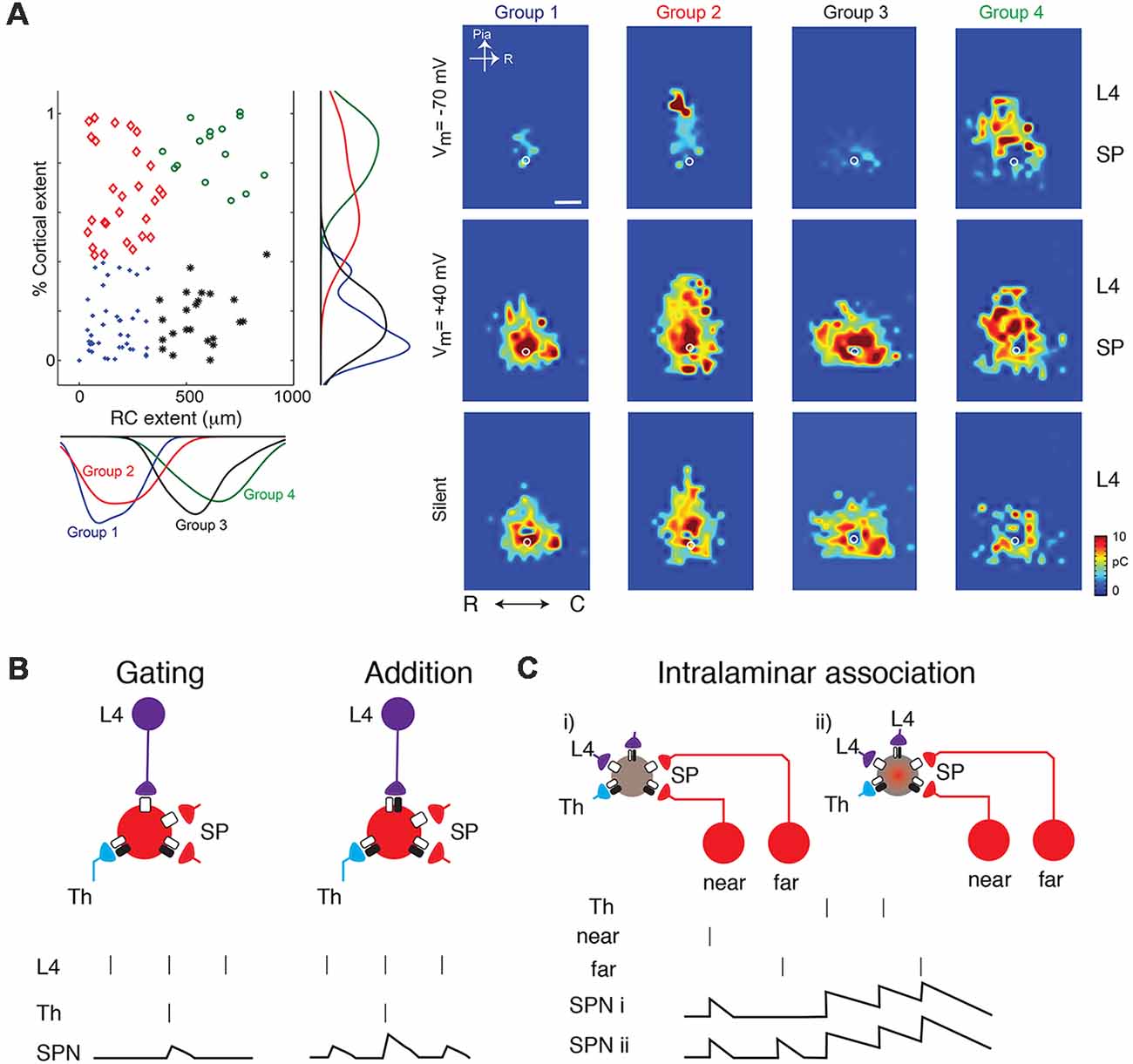
Figure 2. (A) SPNs can be classified into four groups based on their inputs from L4 (from Meng et al., 2014; no copyright permission as required for use of this image). Some classes show silent or AMPAR-containing inputs from L4 while others broadly integrate within the subplate (e.g., group 3). (B) Cartoon illustration how the changing presence of silent synapses on L4 inputs can change the functional association between thalamic inputs and L4 inputs. Vertical bars indicate presynaptic spikes and SPN membrane potential indicated below. At young ages, thalamic inputs might gate L4 inputs such that an SPN depolarization is only present when both thalamic and L4 activity occurs. At older aged SPN depolarizations occur when either is active and inputs sum. (C) Silent synapses might enable the conditional association of inputs e.g., from different locations within the same lamina.
SPNs are not the only cerebral cortical neurons sporting silent synapses. GABAergic neurons have also been shown to have silent synapses and some of these silent synapses originate from connections from SPNs (Deng et al., 2017). Thus, the presence of silent synapses on cortical neurons seems to be a ubiquitous feature of the developing cerebral cortex.
How Then Are Silent Connections to Spns Unsilenced?
The neural activity necessary to depolarize SPNs in order to displace the Mg2+ could come from depolarizing network activity during development. There are multiple non-exclusive sources of depolarizing inputs: (i) ascending sensory inputs; (ii) depolarizing GABAergic inputs; and (iii) depolarizing neuromodulatory inputs.
Ascending sensory activity mediated by AMPARs at thalamocortical terminals could provide depolarizing neural activity to SPNs and regulate the unsilencing of silent synapses on SPNs. Perturbing sensory activity resulted in the prolonged existence of silent synapses in the visual and somatosensory cortices (Ashby and Isaac, 2011; Funahashi et al., 2013; Han et al., 2017). Similarly, removing auditory inputs by cochlear ablation in early life altered the spatial distribution of AMPAR- and NMDAR-mediated connections on GABAergic neurons in the auditory cortex (Deng et al., 2017). In deaf mice, AMPAR-mediated connections were present for more distal connections from L2/3 neurons while L5/6 connections originated from more proximal locations. The spatial pattern of NMDAR-only connections was also altered in deaf animals. This suggests that glutamatergic synapse maturation is regulated with input specificity but that both extrinsic and intrinsic cortical activity such as sensory-evoked activity as well as endogenous cortical activity might play a role.
A second source of depolarizing activity might be provided by GABAergic inputs. When silent synapses are gradually replaced by AMPAR-containing synapses, the dominant form of cortical network activity is driven by depolarizing GABA (Ben-Ari et al., 1997; Ben-Ari, 2002; Allène et al., 2008). This suggests that depolarizing GABA may provide the neural activity for the unsilencing of silent synapses (Ben-Ari et al., 1997; Ben-Ari, 2002). Indeed GABA receptor-mediated depolarization is required for the unsilencing of silent synapses in the developing neocortex (Wang and Kriegstein, 2008) and during the development of adult-generated dentate granule cells (Chancey et al., 2013), but silent synapses can also be unsilenced by seizure activity (Zhou et al., 2011; Sun et al., 2018).
Besides the depolarizing action of GABA, neuromodulators which depolarize SPNs could also play a role in unsilencing silent connections. SPNs have both ACh (Hanganu et al., 2002; Hanganu and Luhmann, 2004) as well as serotonin receptors (Kanold and Luhmann, 2010; Liao and Lee, 2011, 2014) which can act depolarizing. Thus, the actions of these neuromodulators could contribute to the functional unsilencing of silent connections to SPNs.
Thus, in summary, there are multiple non-exclusive sources of depolarizing inputs that might individually or jointly act in unsilencing silent connections to SPNs.
What Are Silent Synapses Good for? What do They Tell us?
NMDAR-mediated connections are not only present in the developing mammalian cerebral cortex but also in other structures. Thus, the computational roles of such connections might be similar. First, it is well-established that NMDAR-only mediated synaptic connections can function as a coincidence detector. The activation of NMDAR not only requires glutamate binding but also membrane depolarization to remove the Mg2+ from the channel pore. One role of a coincidence detector is to enable the refinement of topographic connections as illustrated in the retinogeniculate system. During early development, structured spontaneous activity is present in the retina (Galli and Maffei, 1988; Meister et al., 1991; Feller et al., 1996; Firth et al., 2005) and competition between the two eyes drives retino-geniculate (Penn et al., 1998) and retino-collicular (Shah and Crair, 2008) segregation. Blockade of NMDA signaling alters the segregation of retinal ganglion afferents in both the lateral geniculate nucleus as well as the optic tectum (Hahm et al., 1991; Ruthazer et al., 2003; Ben Fredj et al., 2010).
However, the functional contribution of silent synapses to these processes remains unclear possibly because few retino-geniculate connections are truly “silent” (Hohnke et al., 2000). Moreover, it seems that at least in the retino-geniculate system, silent synapses peak after the increase in sensory-driven activity, thus might play a role at slightly later stages in development (Lu and Constantine-Paton, 2004).
A second potential role of NMDAR-only mediated synaptic connections is to integrate multiple subthreshold EPSCs over time and improve the synchrony between different cells due to a longer activation time scale. This is consistent with the role of NMDARs in supporting synchronized oscillations in the rodent somatosensory cortex (Dupont et al., 2006; Yang et al., 2009).
A third role of silent synapses has been shown recently in the mammalian retina to enhance motion processing by enabling differential processing of a single source of glutamate by two cells (Sethuramanujam et al., 2017).
Given the fact that activity in developing networks is slow and adapting, it seems likely that silent synapses on SPNs are involved in associating activity over longer time scales but do not enhance processing of stimuli, activity, or coincidence on fast time scales.
Moreover, since silent synapses can be unsilenced by correlated activity thus, by extension, the pattern of unsilenced, AMPAR- and NMDAR-containing, connections are in effect a readout of the past amount of correlated activity of inputs to a particular neuron. However, since unsilencing requires coincidence, the pattern of unsilenced neurons might not necessarily reflect the amount of activity per se. Thus, the presence of AMPAR-containing synapses on connections from deep layers indicates that those layers were likely correlated with SPNs. The emergence of AMPAR-containing synapses from L4 to SPNs after ear opening in the auditory cortex (Meng et al., 2014) indicates that L4 activity likely was correlated with SPN activity just before ear opening (Figure 1B). Such an interpretation would be consistent with the increased evoked correlated activity between SPNs and L4 at similar stages of development in ferret (Wess et al., 2017). Interestingly, just before ear opening, correlated activity is largest in the beta band, possibly indicating that such activity might be effective in unsilencing of synapses. Moreover, the spatial pattern of unsilenced synapses from the cortical plate shows a columnar pattern indicating synchronous activity across a cortical column consistent with microelectrode studies (Yang et al., 2009, 2013; Colonnese and Khazipov, 2010; Minlebaev et al., 2011).
SPN Might Enable the Generation of Normal Activity Patterns in the Developing Cortex
The existence of abundant NMDAR-only silent synapses in SPNs and other cortical neurons suggests that most excitatory activity during early development is mediated by NMDARs. Accordingly, cortical network activity in vitro during the first postnatal week can be almost completely blocked by application of the NMDAR antagonist AP5 (Allène et al., 2008). These results suggest that NMDAR-only “silent” synapses are in fact not “silent” but conduct current and regulate cortical network activity. Thus, NMDAR-only synapses are not truly “silent” but represent a unique early developmental mode of activity generation and propagation.
The electrical activity in the developing brain and especially the cortex is dominated by oscillations. These oscillations can be present spontaneously, that is without any overt external trigger, or they can be evoked by sensory stimuli such as light flashes, motor twitches, or sounds (Khazipov et al., 2004; Hanganu et al., 2006; Marcano-Reik and Blumberg, 2008; Yang et al., 2009; Colonnese and Khazipov, 2010; Chipaux et al., 2013; Blumberg et al., 2015). Moreover, spontaneous activity can be generated endogenously in the cortex (Garaschuk et al., 2000; Adelsberger et al., 2005; Siegel et al., 2012) or even in cortical slice culture (Stewart and Plenz, 2008) as well as be reflective of spontaneously generated activity in the sensory periphery e.g., the retina or cochlea (Galli and Maffei, 1988; Meister et al., 1991; Feller et al., 1996; Firth et al., 2005; Tritsch et al., 2007; Siegel et al., 2012; Wang and Bergles, 2015).
These activity patterns are complex and are comprised of fast nested oscillatory patterns, the so-called “spindles,” and slower transients, “SATs” (Vanhatalo et al., 2002; Tolonen et al., 2007; Benders et al., 2015). Centrally and peripherally generated spontaneous activity show different amounts of synchronizing, wave progression, and developmental profile (Siegel et al., 2012). These early activity patterns are thought to be crucially required for the functional maturation of cortical circuits as they enable high local synchrony of activity (Vanhatalo and Kaila, 2006; Tolonen et al., 2007; Kilb et al., 2011; Siegel et al., 2012; Khazipov et al., 2013; Winnubst et al., 2015; Yang et al., 2016; Luhmann and Khazipov, 2018). Many activity patterns can be replicated using in vitro preparations indicating that at minimum cortical circuits and thalamocortical inputs are required (Sun and Luhmann, 2007; Sun et al., 2010). The origin of early cortical oscillations is not known but likely involve the complex interplay of ascending thalamocortical and descending corticothalamic circuits (Yang et al., 2013, 2016; Murata and Colonnese, 2016). Due to their location at the center of thalamocortical and corticothalamic circuits, SPNs can influence cortical activity patterns. Indeed, electrical stimulation of SPNs in an in vitro preparation could cause oscillations (Sun and Luhmann, 2007) and removal of SPNs in vitro abolishes oscillations (Dupont et al., 2006). Further evidence for the crucial role of SPNs in the development of cortical oscillations came from selective ablation studies (Tolner et al., 2012). These in vivo ablation studies showed that SPNs are required for both spontaneous and sensory-evoked oscillations (Tolner et al., 2012). Together, these observations point to a key role of SPNs in the generation or transmission of cortical oscillations. Since, during the time when spontaneous activity is present, many connections to SPNs are NMDAR-only, this suggests that NMDAR-only synapses on SPNs might play a key role in controlling cortical oscillations. Indeed, the synchronized oscillations in developing somatosensory cortex are mediated through NMDARs (Dupont et al., 2006; Minlebaev et al., 2009; Yang et al., 2009).
SPNs Might Conditionally Integrate Ascending and Intracortical Activity via Silent Synapses
The above reflections and the emerging connectivity diagram suggest that the presence of abundant silent and non-silent synapses on SPNs enables SPNs to play important associative roles during development. As mentioned above, SPNs are heterogenous and can functionally be classified into two main groups based on their amount of L4 input but which can further be subdivided based on the amount of intralaminar connectivity which in auditory cortex likely reflects integration across the frequency axis (Meng et al., 2014). One group of SPNs (Figure 2A groups 1 and 3) does not receive L4 inputs and thus primarily depends on feed-forward inputs. The second group (Figure 2A groups 2 and 4) does not only integrate feed-forward thalamocortical inputs from MGB but also receives significant feedback inputs from L4. Thus, both feed-forward and feed-back inputs are affected by the resting potential. Both groups of SPNs can be further subdivided based on the amount of lateral intralaminar integration. Given the heterogeneity, the role of silent connections likely differs between these subgroups of SPNs.
On one hand, SPNs receive early spontaneous and sensory-evoked inputs via the thalamus and on the other hand, they receive early cortical inputs. While sensory stimulation can lead to activity in the matching cortical area (e.g., sounds in auditory cortex or touch in somatosensory cortex), other sources of intracortical activity can also be present such as activity, e.g., multisensory, originating in other cortical areas or intrinsically generated non-sensory evoked activity (Garaschuk et al., 2000; Adelsberger et al., 2005; Khazipov and Luhmann, 2006; Siegel et al., 2012). Thus, by virtue of their position between ascending thalamic circuits and cortical circuits, SPNs can potentially associate ascending and intracortical activity. Moreover, the presence of NMDARs, and not AMPARs, allows SPNs to directly gate and associate cortical activity with ascending thalamic information (Figure 2B). This is due to the fact that NMDARs will not signal unless SPNs are depolarized. Ascending thalamic input can depolarize SPNs and thus remove the Mg2+ block from NMDARs. If sufficient cortical activity is present at the same time then cortico-SP synapses will signal, enhancing the SPN response. Since SPNs innervate L4 and beyond, a temporal coincidence of cortical activity with the ascending activity will boost the ascending thalamic signal. Moreover, because SPNs also project to the thalamus (Viswanathan et al., 2017; Hoerder-Suabedissen et al., 2018), which is involved in generating oscillatory activity, boosted SPN activity has the potential to influence the generation of thalamocortical oscillations. Thus, over time this scenario likely leads to an association or synchronization of both sources of activity.
The changing presence of silent synapses changes the association between thalamic and cortical inputs. The addition of AMPARs at intracortical inputs at later ages transforms the initial conditional or gating association of thalamic and cortical inputs into an additive association (Figure 2B).
Besides ascending thalamic inputs other sources of activity can activate silent synapses and change the associative properties of SPNs. Because developing SPNs might have a slightly depolarized resting potential (Zhao et al., 2009), NMDAR-mediated synapses can potentially be activated by slight depolarizations from the resting potentials. In particular, the GABAR-mediated inputs to SPNs can be depolarizing due to high intracellular Cl− level at early ages (Luhmann et al., 2016). Thus, depolarizing GABA might be able to activate the silent synapses on SPNs. Besides, since developing SPNs tend to have higher membrane resistances (Zhao et al., 2009), which leads to longer time constants, weak depolarizing inputs could be integrated in SPNs and potentially activate the NMDAR-only mediated inputs.
Thus, the existence of silent synapses from spatially specific presynaptic cells suggests a very interesting hypothesis on the functional topology of the developing cortical circuit: the inter- and intra-columnar connections involving SPNs could be dynamic (Figure 2C). In other words, silent synapses can enable SPNs to integrate inputs from either close by or farther away in a state-dependent manner.
Since a crucial variable in acutely unsilencing silent synapses is the resting membrane potential, relatively slow acting neuromodulatory inputs could play a major role in determining the amount of integration taking place in SPNs. As mentioned above, SPNs have ACh as well as serotonin receptors (Hanganu et al., 2002, 2009; Hanganu and Luhmann, 2004; Kanold and Luhmann, 2010; Liao and Lee, 2011, 2014). Moreover, layer 6b neurons in adult, a subset of which represents surviving SPNs (Marx et al., 2017), are modulated by neurotensin controlling wakefulness (Case and Broberger, 2018). It is possible that SPNs are modulated by neuropeptides even at early ages and that their function varies thru the sleep-wake cycle due to differential recruitment of local AMPAR-mediated vs. distal NMDAR-only mediated inputs.
Disruption of SPN in Clinical Conditions and Potential Role of Silent Synapses
Given the central position of SPNs, disruption of SPNs can alter many aspects of cortical development (Ghosh et al., 1990; Ghosh and Shatz, 1992; McConnell et al., 1994; Kanold et al., 2003; Kanold and Shatz, 2006; Tolner et al., 2012). Because of their early maturation and connectivity, SPNs are also susceptible to injury and resulting malfunction. For example, neonatal hypoxic-ischemic (HI) injuries in animals can lesion SPNs or in milder forms cause hyperconnectivity of SPNs (McQuillen et al., 2003; Mikhailova et al., 2017; Sheikh et al., 2019; Figure 3A). Such injuries are associated with altered cortical function and plasticity (Failor et al., 2010; Ranasinghe et al., 2015) similar to those observed after SPN lesion (Kanold et al., 2003; Kanold and Shatz, 2006; Tolner et al., 2012) suggesting that SPN damage after the HI injury might be leading to the downstream effects in the cortical plate.
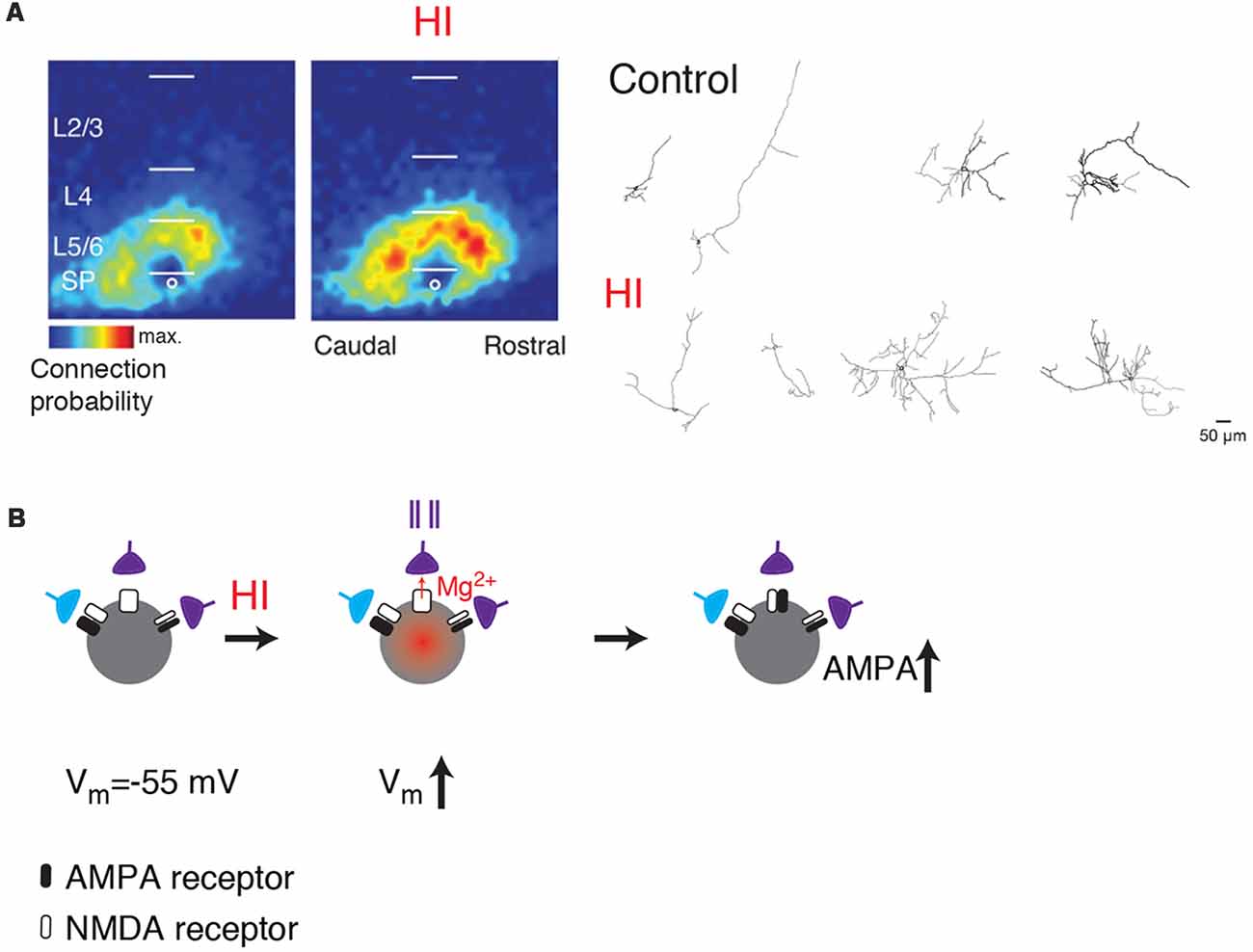
Figure 3. (A) SPNs show functional and morphological hyperconnectivity after hypoxic-ischemic (HI; from Sheikh et al., 2019; no copyright permission as required for use of this image). Shown are LSPS maps of connection probability and neurolucida reconstructions. (B) Cartoon illustrating how depolarization caused by HI can lead to unsilencing of synapses on SPNs (middle). If presynaptic cells are active (indicated by spike train), the coincidence between presynaptic activity and postsynaptic depolarization might result in a subsequent increase in AMPARs (right, black).
In humans, SPN generation begins around the fifth postconceptional week and fully formed between the 20–26th week (Kanold and Luhmann, 2010). Environmental insults that occur during the first trimester can increase the risk of autism. For instance, valproate (VPA) exposure during the first trimester correlates negatively with language outcome in children (Nadebaum et al., 2011). In mice, subplate birth occurs between E11–13, and full formation of subplate by E14 (Kanold and Luhmann, 2010). Although this is outside of the first “trimester,” maternal VPA exposure during this narrow time window results in autistic phenotypes in the offspring (Mabunga et al., 2015; Nicolini and Fahnestock, 2018). Recent in vitro studies in rodents have shown that such prenatal VPA exposure causes miswiring of circuits to SPNs (Nagode et al., 2017). While these circuit changes were detected shortly after birth (Nagode et al., 2017), earlier ages were not studied, thus it is possible that even earlier effects of VPA on SPNs are present. Together, these studies in animal models suggest that SPNs might form a key element in the progression from prenatal insult to disease phenotype in multiple neurodevelopmental disorders.
Because subplate disappears before the onset of ASD symptoms, no direct link has been made between subplate and ASD in humans. However, one of the more intriguing indirect links is the relatively consistent finding of a blurred gray-white matter boundary in the brains of ASD individuals (Avino and Hutsler, 2010; Hutsler and Casanova, 2016), possibly due to an increase in the number of interstitial neurons in the superficial white matter. Interstitial neurons are believed to be remnants of the fetal subplate (Chun and Shatz, 1989; Reep, 2000; Torres-Reveron and Friedlander, 2007; Suárez-Solá et al., 2009; Judaš et al., 2010), and their overabundance could reflect dysfunctional apoptotic mechanisms in ASD (Courchesne and Pierce, 2005; Avino and Hutsler, 2010; Courchesne et al., 2011; McFadden and Minshew, 2013). Autistic patients also show an altered columnar structure in frontal cortex (Buxhoeveden et al., 2006) and patches of disorganization are seen in L4 and L5 of the prefrontal cortex in children with autism (Stoner et al., 2014). Given the putative role of subplate in setting up protomaps of cortical organization (O’Leary and Borngasser, 2006; O’Leary et al., 2007; Wess et al., 2017; Kanold, 2019) and given that alterations are present in the target layers of SPNs, these observations are consistent with a potential role of early SPN dysfunction in the emergence of ASDs. However, the ultimate test of this possibility would require to reverse or to prevent the changes in the SPNs, which in turn could lead to treatment and prevention approaches in humans. The consequences of increased “adult subplate” or interstitial cells on circuit function are not known.
Additional indirect evidence points to a role of SPNs in ASDs. Expression profiling has identified an enrichment in subplate genes for association with ASDs (Hoerder-Suabedissen et al., 2013) but there are additional potential links between subplate damage and ASDs. Hypoxia/ischemia preferentially damages SPNs in rodents (McQuillen et al., 2003; Mikhailova et al., 2017; Sheikh et al., 2019). Birth injuries or preterm labor can lead to hypoxic situations, and both of these have been shown to increase the risk of ASD (reviewed in Rennie et al., 2007; Agrawal et al., 2018; Rogers et al., 2018). Perinatal anoxia in rats also causes ASD phenotypes (Laviola et al., 2004). Importantly, preterm birth is also associated with an increased risk of ASD, and this might be due to an increase in the incidence of pre-eclampsia in mother of preterm infants, among other things (Buchmayer et al., 2009).
Thus, while direct evidence of SPN disruptions in ASDs is currently lacking, multiple lines of evidence suggest that understanding SPN function and susceptibility will be important in understanding the etiology of many neurodevelopmental disorders. One common thread is the vulnerability of SPNs to hypoxia/ischemia. While the acute effects of hypoxic/ischemic injuries on SPNs are unknown, anoxia can induce depolarization in cortical pyramidal cells (Luhmann et al., 1993; Rosen and Morris, 1994; O’Reilly and Haddad, 1996; Pisani et al., 1997). Thus, hypoxia might be able to “unsilence” silent synapses onto SPNs leading to an increased co-activation of presynaptic neurons and postsynaptic SPNs (Figure 3B). Such co-activation would be expected to result in increased connectivity, which is experimentally seen after HI (Sheikh et al., 2019). Indeed, in vitro studies showed that combined oxygen and glucose deprivation depolarizes SPNs (Albrecht et al., 2005). Moreover, hypoxia-induced seizures can diminish silent synapses in hippocampus consistent with such a scenario (Zhou et al., 2011). Thus, activation of silent synapses on SPNs by hypoxia or other insults might be a key step in linking early insults to later circuit dysfunctions.
Conclusion and Open Questions
While SPNs as a population of neurons are coming into clearer view, many unknowns remain. SPNs as a group are both the target of initial thalamic inputs but also receive extensive cortical input. In particular, there is an extensive connectivity of SPNs with the cortical plate mediated by NMDAR-only containing “silent” synapses at the earliest ages. These synapses are very sensitive to the resting potential of the cell, thus subtle modulation of the resting potential can modulate their activity and thus their ability to integrate inputs. Activation of silent synapses on SPNs by hypoxia or other insults might be the initial key step in a cascade of events leading to the manifestation of neurodevelopmental disorders. Therefore, interventions that limit the amount of depolarization or NMDAR signaling might be effective in preventing this chain of events. Indeed, hypothermia has been shown to be effective in reducing the effects of hypoxia on cortical neurons (Hiramatsu et al., 1993; Rosen and Morris, 1994) and improves clinical outcomes (Gunn and Bennet, 2008; Jacobs et al., 2013; Papile et al., 2014).
It is becoming clear that multiple classes of SPNs exist, but the functional roles of the subclasses are unknown. Moreover, given that SPNs are present in all cortical areas, it is likely that there are functional differences between SPNs in different cortical areas. This has not been explored at all. Most importantly, the role of SPNs in development has only been assessed in primary sensory areas, thus important roles of SPNs in other areas, e.g., frontal areas, that might relate to a potential role of SPNs in neurodevelopmental disorders is lacking. SPNs are present in all mammalian species but given that the size of the subplate zone varies between species, it is likely that there are species-dependent differences in SPN organization and possibly function. Such differences have not been elucidated. In particular, the function of SPNs in primates has not been studied. Thus, future studies need to address the functional diversity of SPN within but also across species. Moreover, there is growing evidence of SPN dysfunction in multiple neurodevelopmental disorders. What is lacking is direct functional evidence of SPN disruption in human patients at the relevant early developmental ages. Moreover, while SPNs have been studied in primary sensory areas the phenotypes of many neurodevelopmental disorders have been most prevalent in frontal areas leaving open the possibility that dysfunctions might be more widespread or that a selective vulnerability exists for circuits in frontal areas. Functional imaging or EEG methods might be able to provide such evidence.
Author Contributions
All authors made direct and intellectual contribution to the work and approved it for publication. PK wrote the manuscript with contributions from XM and RD.
Funding
This work was supported by the National Institute on Deafness and Other Communication Disorders (R01 DC009607) to PK.
Conflict of Interest Statement
The authors declare that the research was conducted in the absence of any commercial or financial relationships that could be construed as a potential conflict of interest.
Acknowledgments
We thank all past and current members of the Kanold lab as well as our collaborators for their contribution to a better understanding of the function of subplate neurons. We confirm that we read the Journal’s position on issues involved in ethical publication and affirm that this report is consistent with those guidelines.
References
Adelsberger, H., Garaschuk, O., and Konnerth, A. (2005). Cortical calcium waves in resting newborn mice. Nat. Neurosci. 8, 988–990. doi: 10.1038/nn1502
Agrawal, S., Rao, S. C., Bulsara, M. K., and Patole, S. K. (2018). Prevalence of autism spectrum disorder in preterm infants: a meta-analysis. Pediatrics 142:e20180134. doi: 10.1542/peds.2018-0134
Albrecht, J., Hanganu, I. L., Heck, N., and Luhmann, H. J. (2005). Oxygen and glucose deprivation induces major dysfunction in the somatosensory cortex of the newborn rat. Eur. J. Neurosci. 22, 2295–2305. doi: 10.1111/j.1460-9568.2005.04398.x
Allène, C., Cattani, A., Ackman, J. B., Bonifazi, P., Aniksztejn, L., Ben-Ari, Y., et al. (2008). Sequential generation of two distinct synapse-driven network patterns in developing neocortex. J. Neurosci. 28, 12851–12863. doi: 10.1523/JNEUROSCI.3733-08.2008
Anastasiades, P. G., and Butt, S. J. (2012). A role for silent synapses in the development of the pathway from layer 2/3 to 5 pyramidal cells in the neocortex. J. Neurosci. 32, 13085–13099. doi: 10.1523/JNEUROSCI.1262-12.2012
Antonini, A., and Shatz, C. J. (1990). Relation between putative transmitter phenotypes and connectivity of subplate neurons during cerebral cortical development. Eur. J. Neurosci. 2, 744–761. doi: 10.1111/j.1460-9568.1990.tb00465.x
Ashby, M. C., and Isaac, J. T. (2011). Maturation of a recurrent excitatory neocortical circuit by experience-dependent unsilencing of newly formed dendritic spines. Neuron 70, 510–521. doi: 10.1016/j.neuron.2011.02.057
Avino, T. A., and Hutsler, J. J. (2010). Abnormal cell patterning at the cortical gray-white matter boundary in autism spectrum disorders. Brain Res. 1360, 138–146. doi: 10.1016/j.brainres.2010.08.091
Ben-Ari, Y. (2002). Excitatory actions of gaba during development: the nature of the nurture. Nat. Rev. Neurosci. 3, 728–739. doi: 10.1038/nrn920
Ben-Ari, Y., Khazipov, R., Leinekugel, X., Caillard, O., and Gaiarsa, J. L. (1997). GABAA, NMDA and AMPA receptors: a developmentally regulated ‘ménage à trois’. Trends Neurosci. 20, 523–529. doi: 10.1016/s0166-2236(97)01147-8
Ben Fredj, N., Hammond, S., Otsuna, H., Chien, C. B., Burrone, J., and Meyer, M. P. (2010). Synaptic activity and activity-dependent competition regulates axon arbor maturation, growth arrest, and territory in the retinotectal projection. J. Neurosci. 30, 10939–10951. doi: 10.1523/JNEUROSCI.1556-10.2010
Benders, M. J., Palmu, K., Menache, C., Borradori-Tolsa, C., Lazeyras, F., Sizonenko, S., et al. (2015). Early brain activity relates to subsequent brain growth in premature infants. Cereb. Cortex 25, 3014–3024. doi: 10.1093/cercor/bhu097
Blumberg, M. S., Coleman, C. M., Sokoloff, G., Weiner, J. A., Fritzsch, B., and McMurray, B. (2015). Development of twitching in sleeping infant mice depends on sensory experience. Curr. Biol. 25, 656–662. doi: 10.1016/j.cub.2015.01.022
Bruno, R. M. (2011). Synchrony in sensation. Curr. Opin. Neurobiol. 21, 701–708. doi: 10.1016/j.conb.2011.06.003
Buchmayer, S., Johansson, S., Johansson, A., Hultman, C. M., Sparén, P., and Cnattingius, S. (2009). Can association between preterm birth and autism be explained by maternal or neonatal morbidity? JAMA 124, e817–e825. doi: 10.1542/peds.2008-3582
Butovas, S., Hormuzdi, S. G., Monyer, H., and Schwarz, C. (2006). Effects of electrically coupled inhibitory networks on local neuronal responses to intracortical microstimulation. J. Neurophysiol. 96, 1227–1236. doi: 10.1152/jn.01170.2005
Butts, D. A., and Kanold, P. O. (2010). The applicability of spike time dependent plasticity to development. Front. Synaptic Neurosci. 2:30. doi: 10.3389/fnsyn.2010.00030
Buxhoeveden, D. P., Semendeferi, K., Buckwalter, J., Schenker, N., Switzer, R., and Courchesne, E. (2006). Reduced minicolumns in the frontal cortex of patients with autism. Neuropathol. Appl. Neurobiol. 32, 483–491. doi: 10.1111/j.1365-2990.2006.00745.x
Case, L., and Broberger, C. (2018). Neurotensin broadly recruits inhibition via white matter neurons in the mouse cerebral cortex: synaptic mechanisms for decorrelation. Cereb. Cortex 28, 2711–2724. doi: 10.1093/cercor/bhx149
Chancey, J. H., Adlaf, E. W., Sapp, M. C., Pugh, P. C., Wadiche, J. I., and Overstreet-Wadiche, L. S. (2013). GABA depolarization is required for experience-dependent synapse unsilencing in adult-born neurons. J. Neurosci. 33, 6614–6622. doi: 10.1523/JNEUROSCI.0781-13.2013
Chipaux, M., Colonnese, M. T., Mauguen, A., Fellous, L., Mokhtari, M., Lezcano, O., et al. (2013). Auditory stimuli mimicking ambient sounds drive temporal “delta-brushes” in premature infants. PLoS One 8:e79028. doi: 10.1371/journal.pone.0079028
Chun, J. J., and Shatz, C. J. (1989). Interstitial cells of the adult neocortical white matter are the remnant of the early generated subplate neuron population. J. Comp. Neurol. 282, 555–569. doi: 10.1002/cne.902820407
Clancy, B., and Cauller, L. J. (1999). Widespread projections from subgriseal neurons (layer VII) to layer I in adult rat cortex. J. Comp. Neurol. 407, 275–286. doi: 10.1002/(sici)1096-9861(19990503)407:2<275::aid-cne8>3.0.co;2-0
Colonnese, M. T., and Khazipov, R. (2010). “Slow activity transients” in infant rat visual cortex: a spreading synchronous oscillation patterned by retinal waves. J. Neurosci. 30, 4325–4337. doi: 10.1523/JNEUROSCI.4995-09.2010
Committee on Fetus and Newborn, Papile, L. A., Baley, J. E., Benitz, W., Cummings, J., Carlo, W. A., et al. (2014). Hypothermia and neonatal encephalopathy. Pediatrics 133, 1146–1150. doi: 10.1542/peds.2014-0899
Connors, B. W., and Long, M. A. (2004). Electrical synapses in the mammalian brain. Annu. Rev. Neurosci. 27, 393–418. doi: 10.1146/annurev.neuro.26.041002.131128
Constantinople, C. M., and Bruno, R. M. (2013). Deep cortical layers are activated directly by thalamus. Science 340, 1591–1594. doi: 10.1126/science.1236425
Courchesne, E., Mouton, P. R., Calhoun, M. E., Semendeferi, K., Ahrens-Barbeau, C., Hallet, M. J., et al. (2011). Neuron number and size in prefrontal cortex of children with autism. JAMA 306, 2001–2010. doi: 10.1001/jama.2011.1638
Courchesne, E., and Pierce, K. (2005). Brain overgrowth in autism during a critical time in development: implications for frontal pyramidal neuron and interneuron development and connectivity. Int. J. Dev. Neurosci. 23, 153–170. doi: 10.1016/j.ijdevneu.2005.01.003
Cruikshank, S. J., Landisman, C. E., Mancilla, J. G., and Connors, B. W. (2005). Connexon connexions in the thalamocortical system. Prog. Brain Res. 149, 41–57. doi: 10.1016/s0079-6123(05)49004-4
Deans, M. R., Gibson, J. R., Sellitto, C., Connors, B. W., and Paul, D. L. (2001). Synchronous activity of inhibitory networks in neocortex requires electrical synapses containing connexin36. Neuron 31, 477–485. doi: 10.1016/s0896-6273(01)00373-7
Deng, R., Kao, J. P. Y., and Kanold, P. O. (2017). Distinct translaminar glutamatergic circuits to GABAergic interneurons in the neonatal auditory cortex. Cell Rep. 19, 1141–1150. doi: 10.1016/j.celrep.2017.04.044
Dupont, E., Hanganu, I. L., Kilb, W., Hirsch, S., and Luhmann, H. J. (2006). Rapid developmental switch in the mechanisms driving early cortical columnar networks. Nature 439, 79–83. doi: 10.1038/nature04264
Durand, G. M., Kovalchuk, Y., and Konnerth, A. (1996). Long-term potentiation and functional synapse induction in developing hippocampus. Nature 381, 71–75. doi: 10.1038/381071a0
Failor, S., Nguyen, V., Darcy, D. P., Cang, J., Wendland, M. F., Stryker, M. P., et al. (2010). Neonatal cerebral hypoxia-ischemia impairs plasticity in rat visual cortex. J. Neurosci. 30, 81–92. doi: 10.1523/JNEUROSCI.5656-08.2010
Feller, M. B., Wellis, D. P., Stellwagen, D., Werblin, F. S., and Shatz, C. J. (1996). Requirement for cholinergic synaptic transmission in the propagation of spontaneous retinal waves. Science 272, 1182–1187. doi: 10.1126/science.272.5265.1182
Finney, E. M., Stone, J. R., and Shatz, C. J. (1998). Major glutamatergic projection from subplate into visual cortex during development. J. Comp. Neurol. 398, 105–118. doi: 10.1002/(SICI)1096-9861(19980817)398:1<105::AID-CNE7>3.0.CO;2-5
Firth, S. I., Wang, C. T., and Feller, M. B. (2005). Retinal waves: mechanisms and function in visual system development. Cell Calcium 37, 425–432. doi: 10.1016/j.ceca.2005.01.010
Friauf, E., McConnell, S. K., and Shatz, C. J. (1990). Functional synaptic circuits in the subplate during fetal and early postnatal development of cat visual cortex. J. Neurosci. 10, 2601–2613. doi: 10.1523/JNEUROSCI.10-08-02601.1990
Friauf, E., and Shatz, C. J. (1991). Changing patterns of synaptic input to subplate and cortical plate during development of visual cortex. J. Neurophysiol. 66, 2059–2071. doi: 10.1152/jn.1991.66.6.2059
Fukuda, T., Kosaka, T., Singer, W., and Galuske, R. A. (2006). Gap junctions among dendrites of cortical GABAergic neurons establish a dense and widespread intercolumnar network. J. Neurosci. 26, 3434–3443. doi: 10.1523/JNEUROSCI.4076-05.2006
Funahashi, R., Maruyama, T., Yoshimura, Y., and Komatsu, Y. (2013). Silent synapses persist into adulthood in layer 2/3 pyramidal neurons of visual cortex in dark-reared mice. J. Neurophysiol. 109, 2064–2076. doi: 10.1152/jn.00912.2012
Galarreta, M., and Hestrin, S. (1999). A network of fast-spiking cells in the neocortex connected by electrical synapses. Nature 402, 72–75. doi: 10.1038/47029
Galarreta, M., and Hestrin, S. (2001). Electrical synapses between GABA-releasing interneurons. Nat. Rev. Neurosci. 2, 425–433. doi: 10.1038/35077566
Galarreta, M., and Hestrin, S. (2002). Electrical and chemical synapses among parvalbumin fast-spiking GABAergic interneurons in adult mouse neocortex. Proc. Natl. Acad. Sci. U S A 99, 12438–12443. doi: 10.1073/pnas.192159599
Galli, L., and Maffei, L. (1988). Spontaneous impulse activity of rat retinal ganglion cells in prenatal life. Science 242, 90–91. doi: 10.1126/science.3175637
Garaschuk, O., Linn, J., Eilers, J., and Konnerth, A. (2000). Large-scale oscillatory calcium waves in the immature cortex. Nat. Neurosci. 3, 452–459. doi: 10.1038/74823
Ghosh, A., Antonini, A., McConnell, S. K., and Shatz, C. J. (1990). Requirement for subplate neurons in the formation of thalamocortical connections. Nature 347, 179–181. doi: 10.1038/347179a0
Ghosh, A., and Shatz, C. J. (1992). Involvement of subplate neurons in the formation of ocular dominance columns. Science 255, 1441–1443. doi: 10.1126/science.1542795
Gibson, J. R., Beierlein, M., and Connors, B. W. (1999). Two networks of electrically coupled inhibitory neurons in neocortex. Nature 402, 75–79. doi: 10.1038/47035
Gunn, A. J., and Bennet, L. (2008). Brain cooling for preterm infants. Clin. Perinatol. 35, 735–748, vi–vii. doi: 10.1016/j.clp.2008.07.012
Hahm, J. O., Langdon, R. B., and Sur, M. (1991). Disruption of retinogeniculate afferent segregation by antagonists to NMDA receptors. Nature 351, 568–570. doi: 10.1038/351568a0
Han, K. S., Cooke, S. F., and Xu, W. (2017). Experience-dependent equilibration of AMPAR-mediated synaptic transmission during the critical period. Cell Rep. 18, 892–904. doi: 10.1016/j.celrep.2016.12.084
Hanganu, I. L., Ben-Ari, Y., and Khazipov, R. (2006). Retinal waves trigger spindle bursts in the neonatal rat visual cortex. J. Neurosci. 26, 6728–6736. doi: 10.1523/JNEUROSCI.0752-06.2006
Hanganu, I. L., Kilb, W., and Luhmann, H. J. (2002). Functional synaptic projections onto subplate neurons in neonatal rat somatosensory cortex. J. Neurosci. 22, 7165–7176. doi: 10.1523/JNEUROSCI.22-16-07165.2002
Hanganu, I. L., and Luhmann, H. J. (2004). Functional nicotinic acetylcholine receptors on subplate neurons in neonatal rat somatosensory cortex. J. Neurophysiol. 92, 189–198. doi: 10.1152/jn.00010.2004
Hanganu, I. L., Okabe, A., Lessmann, V., and Luhmann, H. J. (2009). Cellular mechanisms of subplate-driven and cholinergic input-dependent network activity in the neonatal rat somatosensory cortex. Cereb. Cortex 19, 89–105. doi: 10.1093/cercor/bhn061
Hanse, E., Seth, H., and Riebe, I. (2013). AMPA-silent synapses in brain development and pathology. Nat. Rev. Neurosci. 14, 839–850. doi: 10.1038/nrn3642
Henschke, J. U., Oelschlegel, A. M., Angenstein, F., Ohl, F. W., Goldschmidt, J., Kanold, P. O., et al. (2018). Early sensory experience influences the development of multisensory thalamocortical and intracortical connections of primary sensory cortices. Brain Struct. Funct. 223, 1165–1190. doi: 10.1007/s00429-017-1549-1
Higashi, S., Hioki, K., Kurotani, T., Kasim, N., and Molnár, Z. (2005). Functional thalamocortical synapse reorganization from subplate to layer IV during postnatal development in the reeler-like mutant rat (shaking rat Kawasaki). J. Neurosci. 25, 1395–1406. doi: 10.1523/JNEUROSCI.4023-04.2005
Higashi, S., Molnár, Z., Kurotani, T., and Toyama, K. (2002). Prenatal development of neural excitation in rat thalamocortical projections studied by optical recording. Neuroscience 115, 1231–1246. doi: 10.1016/s0306-4522(02)00418-9
Higo, S., Udaka, N., and Tamamaki, N. (2007). Long-range GABAergic projection neurons in the cat neocortex. J. Comp. Neurol. 503, 421–431. doi: 10.1002/cne.21395
Hiramatsu, K., Kassell, N. F., and Lee, K. S. (1993). Thermal sensitivity of hypoxic responses in neocortical brain slices. J. Cereb. Blood Flow Metab. 13, 395–401. doi: 10.1038/jcbfm.1993.53
Hoerder-Suabedissen, A., Hayashi, S., Upton, L., Nolan, Z., Casas-Torremocha, D., Grant, E., et al. (2018). Subset of cortical layer 6b neurons selectively innervates higher order thalamic nuclei in mice. Cereb. Cortex 28, 1882–1897. doi: 10.1093/cercor/bhy036
Hoerder-Suabedissen, A., Oeschger, F. M., Krishnan, M. L., Belgard, T. G., Wang, W. Z., Lee, S., et al. (2013). Expression profiling of mouse subplate reveals a dynamic gene network and disease association with autism and schizophrenia. Proc. Natl. Acad. Sci. U S A 110, 3555–3560. doi: 10.1073/pnas.1218510110
Hoerder-Suabedissen, A., Wang, W. Z., Lee, S., Davies, K. E., Goffinet, A. M., Rakić, S., et al. (2009). Novel markers reveal subpopulations of subplate neurons in the murine cerebral cortex. Cereb. Cortex 19, 1738–1750. doi: 10.1093/cercor/bhn195
Hohnke, C. D., Oray, S., and Sur, M. (2000). Activity-dependent patterning of retinogeniculate axons proceeds with a constant contribution from AMPA and NMDA receptors. J. Neurosci. 20, 8051–8060. doi: 10.1523/JNEUROSCI.20-21-08051.2000
Hutsler, J. J., and Casanova, M. F. (2016). Review: cortical construction in autism spectrum disorder: columns, connectivity and the subplate. Neuropathol. Appl. Neurobiol. 42, 115–134. doi: 10.1111/nan.12227
Isaac, J. T. (2003). Postsynaptic silent synapses: evidence and mechanisms. Neuropharmacology 45, 450–460. doi: 10.1016/s0028-3908(03)00229-6
Isaac, J. T., Crair, M. C., Nicoll, R. A., and Malenka, R. C. (1997). Silent synapses during development of thalamocortical inputs. Neuron 18, 269–280. doi: 10.1016/s0896-6273(00)80267-6
Isaac, J. T., Nicoll, R. A., and Malenka, R. C. (1995). Evidence for silent synapses: implications for the expression of LTP. Neuron 15, 427–434. doi: 10.1016/0896-6273(95)90046-2
Jacobs, S. E., Berg, M., Hunt, R., Tarnow-Mordi, W. O., Inder, T. E., and Davis, P. G. (2013). Cooling for newborns with hypoxic ischaemic encephalopathy. Cochrane Database Syst. Rev. 1:CD003311. doi: 10.1002/14651858.CD003311.pub3
Ji, X. Y., Zingg, B., Mesik, L., Xiao, Z., Zhang, L. I., and Tao, H. W. (2016). Thalamocortical innervation pattern in mouse auditory and visual cortex: laminar and cell-type specificity. Cereb. Cortex 26, 2612–2625. doi: 10.1093/cercor/bhv099
Judaš, M., Sedmak, G., Pletikos, M., and Jovanov-Milošević, N. (2010). Populations of subplate and interstitial neurons in fetal and adult human telencephalon. J. Anat. 217, 381–399. doi: 10.1111/j.1469-7580.2010.01284.x
Kanold, P. O. (2009). Subplate neurons: crucial regulators of cortical development and plasticity. Front. Neuroanat. 3:16. doi: 10.3389/neuro.05.016.2009
Kanold, P. O. (2019). The first cortical circuits: subplate neurons lead the way and shape cortical organization. Neuroforum 25, 15–23. doi: 10.1515/nf-2018-0010
Kanold, P. O., Kara, P., Reid, R. C., and Shatz, C. J. (2003). Role of subplate neurons in functional maturation of visual cortical columns. Science 301, 521–525. doi: 10.1126/science.1084152
Kanold, P. O., and Luhmann, H. J. (2010). The subplate and early cortical circuits. Annu. Rev. Neurosci. 33, 23–48. doi: 10.1146/annurev-neuro-060909-153244
Kanold, P. O., and Shatz, C. J. (2006). Subplate neurons regulate maturation of cortical inhibition and outcome of ocular dominance plasticity. Neuron 51, 627–638. doi: 10.1016/j.neuron.2006.07.008
Kerchner, G. A., and Nicoll, R. A. (2008). Silent synapses and the emergence of a postsynaptic mechanism for LTP. Nat. Rev. Neurosci. 9, 813–825. doi: 10.1038/nrn2501
Khazipov, R., and Luhmann, H. J. (2006). Early patterns of electrical activity in the developing cerebral cortex of humans and rodents. Trends Neurosci. 29, 414–418. doi: 10.1016/j.tins.2006.05.007
Khazipov, R., Minlebaev, M., and Valeeva, G. (2013). Early γ oscillations. Neuroscience 250, 240–252. doi: 10.1016/j.neuroscience.2013.07.019
Khazipov, R., Sirota, A., Leinekugel, X., Holmes, G. L., Ben-Ari, Y., and Buzsáki, G. (2004). Early motor activity drives spindle bursts in the developing somatosensory cortex. Nature 432, 758–761. doi: 10.1038/nature03132
Kilb, W., Kirischuk, S., and Luhmann, H. J. (2011). Electrical activity patterns and the functional maturation of the neocortex. Eur. J. Neurosci. 34, 1677–1686. doi: 10.1111/j.1460-9568.2011.07878.x
Kotak, V. C., Sadahiro, M., and Fall, C. P. (2007). Developmental expression of endogenous oscillations and waves in the auditory cortex involves calcium, gap junctions, and GABA. Neuroscience 146, 1629–1639. doi: 10.1016/j.neuroscience.2007.03.039
Krmpotić-Nemanić, J., Kostović, I., Kelović, Z., Nemanić, D., and Mrzljak, L. (1983). Development of the human fetal auditory cortex: growth of afferent fibres. Acta Anat. 116, 69–73. doi: 10.1159/000145727
Kullmann, D. M. (2003). Silent synapses: what are they telling us about long-term potentiation? Philos. Trans. R. Soc. Lond. B Biol. Sci. 358, 727–733. doi: 10.1098/rstb.2002.1229
Kullmann, D. M., and Asztely, F. (1998). Extrasynaptic glutamate spillover in the hippocampus: evidence and implications. Trends Neurosci. 21, 8–14. doi: 10.1016/s0166-2236(97)01150-8
Laviola, G., Adriani, W., Rea, M., Aloe, L., and Alleva, E. (2004). Social withdrawal, neophobia, and stereotyped behavior in developing rats exposed to neonatal asphyxia. Psychopharmacology 175, 196–205. doi: 10.1007/s00213-004-1800-3
Liao, D., Hessler, N. A., and Malinow, R. (1995). Activation of postsynaptically silent synapses during pairing-induced LTP in CA1 region of hippocampal slice. Nature 375, 400–404. doi: 10.1038/375400a0
Liao, C. C., and Lee, L. J. (2011). Neonatal fluoxetine exposure affects the action potential properties and dendritic development in cortical subplate neurons of rats. Toxicol. Lett. 207, 314–321. doi: 10.1016/j.toxlet.2011.09.028
Liao, C. C., and Lee, L. J. (2014). Presynaptic 5-HT1B receptor-mediated synaptic suppression to the subplate neurons in the somatosensory cortex of neonatal rats. Neuropharmacology 77, 81–89. doi: 10.1016/j.neuropharm.2013.08.040
Lu, W., and Constantine-Paton, M. (2004). Eye opening rapidly induces synaptic potentiation and refinement. Neuron 43, 237–249. doi: 10.1016/j.neuron.2004.06.031
Luhmann, H. J., and Khazipov, R. (2018). Neuronal activity patterns in the developing barrel cortex. Neuroscience 368, 256–267. doi: 10.1016/j.neuroscience.2017.05.025
Luhmann, H. J., Kirischuk, S., and Kilb, W. (2018). The superior function of the subplate in early neocortical development. Front. Neuroanat. 12:97. doi: 10.3389/fnana.2018.00097
Luhmann, H. J., Kral, T., and Heinemann, U. (1993). Influence of hypoxia on excitation and GABAergic inhibition in mature and developing rat neocortex. Exp. Brain Res. 97, 209–224. doi: 10.1007/bf00228690
Luhmann, H. J., Sinning, A., Yang, J. W., Reyes-Puerta, V., Stüttgen, M. C., Kirischuk, S., et al. (2016). Spontaneous neuronal activity in developing neocortical networks: from single cells to large-scale interactions. Front. Neural Circuits 10:40. doi: 10.3389/fncir.2016.00040
Ma, Y., Hioki, H., Konno, M., Pan, S., Nakamura, H., Nakamura, K. C., et al. (2011). Expression of gap junction protein connexin36 in multiple subtypes of GABAergic neurons in adult rat somatosensory cortex. Cereb. Cortex 21, 2639–2649. doi: 10.1093/cercor/bhr051
Mabunga, D. F., Gonzales, E. L., Kim, J. W., Kim, K. C., and Shin, C. Y. (2015). Exploring the validity of valproic acid animal model of autism. Exp. Neurobiol. 24, 285–300. doi: 10.5607/en.2015.24.4.285
Marcano-Reik, A. J., and Blumberg, M. S. (2008). The corpus callosum modulates spindle-burst activity within homotopic regions of somatosensory cortex in newborn rats. Eur. J. Neurosci. 28, 1457–1466. doi: 10.1111/j.1460-9568.2008.06461.x
Marx, M., Qi, G., Hanganu-Opatz, I. L., Kilb, W., Luhmann, H. J., and Feldmeyer, D. (2017). Neocortical layer 6B as a remnant of the subplate—a morphological comparison. Cereb. Cortex 27, 1011–1026. doi: 10.1093/cercor/bhv279
McConnell, S. K., Ghosh, A., and Shatz, C. J. (1994). Subplate pioneers and the formation of descending connections from cerebral cortex. J. Neurosci. 14, 1892–1907. doi: 10.1523/JNEUROSCI.14-04-01892.1994
McFadden, K., and Minshew, N. J. (2013). Evidence for dysregulation of axonal growth and guidance in the etiology of ASD. Front. Hum. Neurosci. 7:671. doi: 10.3389/fnhum.2013.00671
McQuillen, P. S., Sheldon, R. A., Shatz, C. J., and Ferriero, D. M. (2003). Selective vulnerability of subplate neurons after early neonatal hypoxia-ischemia. J. Neurosci. 23, 3308–3315. doi: 10.1523/JNEUROSCI.23-08-03308.2003
Mechawar, N., and Descarries, L. (2001). The cholinergic innervation develops early and rapidly in the rat cerebral cortex: a quantitative immunocytochemical study. Neuroscience 108, 555–567. doi: 10.1016/s0306-4522(01)00389-x
Meinecke, D. L., and Rakic, P. (1992). Expression of GABA and GABAA receptors by neurons of the subplate zone in developing primate occipital cortex: evidence for transient local circuits. J. Comp. Neurol. 317, 91–101. doi: 10.1002/cne.903170107
Meister, M., Wong, R. O., Baylor, D. A., and Shatz, C. J. (1991). Synchronous bursts of action potentials in ganglion cells of the developing mammalian retina. Science 252, 939–943. doi: 10.1126/science.2035024
Meng, X., Kao, J. P., and Kanold, P. O. (2014). Differential signaling to subplate neurons by spatially specific silent synapses in developing auditory cortex. J. Neurosci. 34, 8855–8864. doi: 10.1523/jneurosci.0233-14.2014
Meyer, H. S., Wimmer, V. C., Hemberger, M., Bruno, R. M., de Kock, C. P., Frick, A., et al. (2010). Cell type-specific thalamic innervation in a column of rat vibrissal cortex. Cereb. Cortex 20, 2287–2303. doi: 10.1093/cercor/bhq069
Mikhailova, A., Sunkara, N., and McQuillen, P. S. (2017). Unbiased quantification of subplate neuron loss following neonatal hypoxia-ischemia in a rat model. Dev. Neurosci. 39, 171–181. doi: 10.1159/000460815
Minlebaev, M., Ben-Ari, Y., and Khazipov, R. (2009). NMDA receptors pattern early activity in the developing barrel cortex in vivo. Cereb. Cortex 19, 688–696. doi: 10.1093/cercor/bhn115
Minlebaev, M., Colonnese, M., Tsintsadze, T., Sirota, A., and Khazipov, R. (2011). Early γ oscillations synchronize developing thalamus and cortex. Science 334, 226–229. doi: 10.1126/science.1210574
Molnár, Z., Kurotani, T., Higashi, S., Yamamoto, N., and Toyama, K. (2003). Development of functional thalamocortical synapses studied with current source-density analysis in whole forebrain slices in the rat. Brain Res. Bull. 60, 355–371. doi: 10.1016/s0361-9230(03)00061-3
Mrzljak, L., Uylings, H. B., Kostovic, I., and Van Eden, C. G. (1988). Prenatal development of neurons in the human prefrontal cortex: I. A qualitative Golgi study. J. Comp. Neurol. 271, 355–386. doi: 10.1002/cne.902710306
Murata, Y., and Colonnese, M. T. (2016). An excitatory cortical feedback loop gates retinal wave transmission in rodent thalamus. Elife 5:e18816. doi: 10.7554/elife.18816
Myakhar, O., Unichenko, P., and Kirischuk, S. (2011). GABAergic projections from the subplate to Cajal-Retzius cells in the neocortex. Neuroreport 22, 525–529. doi: 10.1097/wnr.0b013e32834888a4
Nadebaum, C., Anderson, V. A., Vajda, F., Reutens, D. C., Barton, S., and Wood, A. G. (2011). Language skills of school-aged children prenatally exposed to antiepileptic drugs. Neurology 76, 719–726. doi: 10.1212/wnl.0b013e31820d62c7
Nagode, D. A., Meng, X., Winkowski, D. E., Smith, E., Khan-Tareen, H., Kareddy, V., et al. (2017). Abnormal development of the earliest cortical circuits in a mouse model of autism spectrum disorder. Cell Rep. 18, 1100–1108. doi: 10.1016/j.celrep.2017.01.006
Nakazawa, M., Koh, T., Kani, K., and Maeda, T. (1992). Transient patterns of serotonergic innervation in the rat visual cortex: normal development and effects of neonatal enucleation. Dev. Brain Res. 66, 77–90. doi: 10.1016/0165-3806(92)90143-k
Nicolini, C., and Fahnestock, M. (2018). The valproic acid-induced rodent model of autism. Exp. Neurol. 299, 217–227. doi: 10.1016/j.expneurol.2017.04.017
Niculescu, D., and Lohmann, C. (2014). Gap junctions in developing thalamic and neocortical neuronal networks. Cereb. Cortex 24, 3097–3106. doi: 10.1093/cercor/bht175
Nowak, L., Bregestovski, P., Ascher, P., Herbet, A., and Prochiantz, A. (1984). Magnesium gates glutamate-activated channels in mouse central neurones. Nature 307, 462–465. doi: 10.1038/307462a0
O’Leary, D. D., and Borngasser, D. (2006). Cortical ventricular zone progenitors and their progeny maintain spatial relationships and radial patterning during preplate development indicating an early protomap. Cereb. Cortex 16, i46–i56. doi: 10.1093/cercor/bhk019
O’Leary, D. D., Chou, S. J., Hamasaki, T., Sahara, S., Takeuchi, A., Thuret, S., et al. (2007). Regulation of laminar and area patterning of mammalian neocortex and behavioural implications. Novartis Found. Symp. 288, 141–159; discussion 159–164, 276–181. doi: 10.1002/9780470994030.ch11
O’Reilly, J. P., and Haddad, G. G. (1996). Chronic hypoxia in vivo renders neocortical neurons more vulnerable to subsequent acute hypoxic stress. Brain Res. 711, 203–210. doi: 10.1016/0006-8993(95)01396-2
Pedraza, M., Hoerder-Suabedissen, A., Albert-Maestro, M. A., Molnar, Z., and De Carlos, J. A. (2014). Extracortical origin of some murine subplate cell populations. Proc. Natl. Acad. Sci. U S A 111, 8613–8618. doi: 10.1073/pnas.1323816111
Peinado, A., Yuste, R., and Katz, L. C. (1993a). Gap junctional communication and the development of local circuits in neocortex. Cereb. Cortex 3, 488–498. doi: 10.1093/cercor/3.5.488
Peinado, A., Yuste, R., and Katz, L. C. (1993b). Extensive dye coupling between rat neocortical neurons during the period of circuit formation. Neuron 10, 103–114. doi: 10.1016/0896-6273(93)90246-n
Penn, A. A., Riquelme, P. A., Feller, M. B., and Shatz, C. J. (1998). Competition in retinogeniculate patterning driven by spontaneous activity. Science 279, 2108–2112. doi: 10.1126/science.279.5359.2108
Piñon, M. C., Jethwa, A., Jacobs, E., Campagnoni, A., and Molnar, Z. (2009). Dynamic integration of subplate neurons into the cortical barrel field circuitry during postnatal development in the Golli-tau-eGFP (GTE) mouse. J. Physiol. 587, 1903–1915. doi: 10.1113/jphysiol.2008.167767
Pisani, A., Calabresi, P., and Bernardi, G. (1997). Hypoxia in striatal and cortical neurones: membrane potential and Ca2+ measurements. Neuroreport 8, 1143–1147. doi: 10.1097/00001756-199703240-00017
Postma, F., Liu, C. H., Dietsche, C., Khan, M., Lee, H. K., Paul, D., et al. (2011). Electrical synapses formed by connexin36 regulate inhibition- and experience-dependent plasticity. Proc. Natl. Acad. Sci. U S A 108, 13770–13775. doi: 10.1073/pnas.1100166108
Ranasinghe, S., Or, G., Wang, E. Y., Ievins, A., McLean, M. A., Niell, C. M., et al. (2015). Reduced cortical activity impairs development and plasticity after neonatal hypoxia ischemia. J. Neurosci. 35, 11946–11959. doi: 10.1523/jneurosci.2682-14.2015
Reep, R. L. (2000). Cortical layer VII and persistent subplate cells in mammalian brains. Brain Behav. Evol. 56, 212–234. doi: 10.1159/000047206
Rennie, J. M., Hagmann, C. F., and Robertson, N. J. (2007). Outcome after intrapartum hypoxic ischaemia at term. Semin. Fetal Neonatal Med. 12, 398–407. doi: 10.1016/j.siny.2007.07.006
Roerig, B., and Feller, M. B. (2000). Neurotransmitters and gap junctions in developing neural circuits. Brain Res. Rev. 32, 86–114. doi: 10.1016/s0165-0173(99)00069-7
Rogers, C. E., Lean, R. E., Wheelock, M. D., and Smyser, C. D. (2018). Aberrant structural and functional connectivity and neurodevelopmental impairment in preterm children. J. Neurodev. Disord. 10:38. doi: 10.1186/s11689-018-9253-x
Rosen, A. S., and Morris, M. E. (1994). Influence of temperature on anoxic responses of neocortical pyramidal neurons. Neuroscience 63, 949–955. doi: 10.1016/0306-4522(94)90563-0
Rumpel, S., Hatt, H., and Gottmann, K. (1998). Silent synapses in the developing rat visual cortex: evidence for postsynaptic expression of synaptic plasticity. J. Neurosci. 18, 8863–8874. doi: 10.1523/JNEUROSCI.18-21-08863.1998
Rumpel, S., Kattenstroth, G., and Gottmann, K. (2004). Silent synapses in the immature visual cortex: layer-specific developmental regulation. J. Neurophysiol. 91, 1097–1101. doi: 10.1152/jn.00443.2003
Ruthazer, E. S., Akerman, C. J., and Cline, H. T. (2003). Control of axon branch dynamics by correlated activity in vivo. Science 301, 66–70. doi: 10.1126/science.1082545
Sethuramanujam, S., Yao, X., deRosenroll, G., Briggman, K. L., Field, G. D., and Awatramani, G. B. (2017). “Silent” NMDA synapses enhance motion sensitivity in a mature retinal circuit. Neuron 96, 1099.e3–1111.e3. doi: 10.1016/j.neuron.2017.09.058
Shah, R. D., and Crair, M. C. (2008). Retinocollicular synapse maturation and plasticity are regulated by correlated retinal waves. J. Neurosci. 28, 292–303. doi: 10.1523/jneurosci.4276-07.2008
Sheikh, A., Meng, X., Liu, J., Mikhailova, A., Kao, J. P. Y., McQuillen, P. S., et al. (2019). Neonatal hypoxia-ischemia causes functional circuit changes in subplate neurons. Cereb. Cortex 29, 765–776. doi: 10.1093/cercor/bhx358
Sherman, S. M. (2012). Thalamocortical interactions. Curr. Opin. Neurobiol. 22, 575–579. doi: 10.1016/j.conb.2012.03.005
Sherman, S. M. (2017). Functioning of circuits connecting thalamus and cortex. Compr. Physiol. 7, 713–739. doi: 10.1002/cphy.c160032
Siegel, F., Heimel, J. A., Peters, J., and Lohmann, C. (2012). Peripheral and central inputs shape network dynamics in the developing visual cortex in vivo. Curr. Biol. 22, 253–258. doi: 10.1016/j.cub.2011.12.026
Stewart, C. V., and Plenz, D. (2008). Homeostasis of neuronal avalanches during postnatal cortex development in vitro. J. Neurosci. Methods 169, 405–416. doi: 10.1016/j.jneumeth.2007.10.021
Stoner, R., Chow, M. L., Boyle, M. P., Sunkin, S. M., Mouton, P. R., Roy, S., et al. (2014). Patches of disorganization in the neocortex of children with autism. N. Engl. J. Med. 370, 1209–1219. doi: 10.1056/NEJMoa1307491
Suárez-Solá, M. L., González-Delgado, F. J., Pueyo-Morlans, M., Medina-Bolívar, O. C., Hernández-Acosta, N. C., González-Gómez, M., et al. (2009). Neurons in the white matter of the adult human neocortex. Front. Neuroanat. 3:7. doi: 10.3389/neuro.05.007.2009
Sun, J. J., Kilb, W., and Luhmann, H. J. (2010). Self-organization of repetitive spike patterns in developing neuronal networks in vitro. Eur. J. Neurosci. 32, 1289–1299. doi: 10.1111/j.1460-9568.2010.07383.x
Sun, J. J., and Luhmann, H. J. (2007). Spatio-temporal dynamics of oscillatory network activity in the neonatal mouse cerebral cortex. Eur. J. Neurosci. 26, 1995–2004. doi: 10.1111/j.1460-9568.2007.05819.x
Sun, H., Takesian, A. E., Wang, T. T., Lippman-Bell, J. J., Hensch, T. K., and Jensen, F. E. (2018). Early seizures prematurely unsilence auditory synapses to disrupt thalamocortical critical period plasticity. Cell Rep. 23, 2533–2540. doi: 10.1016/j.celrep.2018.04.108
Sun, W., Tan, Z., Mensh, B. D., and Ji, N. (2016). Thalamus provides layer 4 of primary visual cortex with orientation- and direction-tuned inputs. Nat. Neurosci. 19, 308–315. doi: 10.1038/nn.4196
Supèr, H., Soriano, E., and Uylings, H. B. (1998). The functions of the preplate in development and evolution of the neocortex and hippocampus. Brain Res. Rev. 27, 40–64. doi: 10.1016/s0165-0173(98)00005-8
Tolner, E. A., Sheikh, A., Yukin, A. Y., Kaila, K., and Kanold, P. O. (2012). Subplate neurons promote spindle bursts and thalamocortical patterning in the neonatal rat somatosensory cortex. J. Neurosci. 32, 692–702. doi: 10.1523/jneurosci.1538-11.2012
Tolonen, M., Palva, J. M., Andersson, S., and Vanhatalo, S. (2007). Development of the spontaneous activity transients and ongoing cortical activity in human preterm babies. Neuroscience 145, 997–1006. doi: 10.1016/j.neuroscience.2006.12.070
Torres-Reveron, J., and Friedlander, M. J. (2007). Properties of persistent postnatal cortical subplate neurons. J. Neurosci. 27, 9962–9974. doi: 10.1523/jneurosci.1536-07.2007
Tritsch, N. X., Yi, E., Gale, J. E., Glowatzki, E., and Bergles, D. E. (2007). The origin of spontaneous activity in the developing auditory system. Nature 450, 50–55. doi: 10.1038/nature06233
Vanhatalo, S., and Kaila, K. (2006). Development of neonatal EEG activity: from phenomenology to physiology. Semin. Fetal Neonatal Med. 11, 471–478. doi: 10.1016/j.siny.2006.07.008
Vanhatalo, S., Tallgren, P., Andersson, S., Sainio, K., Voipio, J., and Kaila, K. (2002). DC-EEG discloses prominent, very slow activity patterns during sleep in preterm infants. Clin. Neurophysiol. 113, 1822–1825. doi: 10.1016/s1388-2457(02)00292-4
Venance, L., Rozov, A., Blatow, M., Burnashev, N., Feldmeyer, D., and Monyer, H. (2000). Connexin expression in electrically coupled postnatal rat brain neurons. Proc. Natl. Acad. Sci. U S A 97, 10260–10265. doi: 10.1073/pnas.160037097
Viswanathan, S., Bandyopadhyay, S., Kao, J. P., and Kanold, P. O. (2012). Changing microcircuits in the subplate of the developing cortex. J. Neurosci. 32, 1589–1601. doi: 10.1523/jneurosci.4748-11.2012
Viswanathan, S., Sheikh, A., Looger, L. L., and Kanold, P. O. (2017). Molecularly defined subplate neurons project both to thalamocortical recipient layers and thalamus. Cereb. Cortex 27, 4759–4768. doi: 10.1093/cercor/bhw271
Voigt, T., and de Lima, A. D. (1991). Serotoninergic innervation of the ferret cerebral cortex. J. Comp. Neurol. 314, 415–428. doi: 10.1002/cne.903140215
Wang, H. C., and Bergles, D. E. (2015). Spontaneous activity in the developing auditory system. Cell Tissue Res. 361, 65–75. doi: 10.1007/s00441-014-2007-5
Wang, D. D., and Kriegstein, A. R. (2008). GABA regulates excitatory synapse formation in the neocortex via NMDA receptor activation. J. Neurosci. 28, 5547–5558. doi: 10.1523/jneurosci.5599-07.2008
Wess, J. M., Isaiah, A., Watkins, P. V., and Kanold, P. O. (2017). Subplate neurons are the first cortical neurons to respond to sensory stimuli. Proc. Natl. Acad. Sci. U S A 114, 12602–12607. doi: 10.1073/pnas.1710793114
Winnubst, J., Cheyne, J. E., Niculescu, D., and Lohmann, C. (2015). Spontaneous activity drives local synaptic plasticity in vivo. Neuron 87, 399–410. doi: 10.1016/j.neuron.2015.06.029
Yang, J. W., An, S., Sun, J. J., Reyes-Puerta, V., Kindler, J., Berger, T., et al. (2013). Thalamic network oscillations synchronize ontogenetic columns in the newborn rat barrel cortex. Cereb. Cortex 23, 1299–1316. doi: 10.1093/cercor/bhs103
Yang, J. W., Hanganu-Opatz, I. L., Sun, J. J., and Luhmann, H. J. (2009). Three patterns of oscillatory activity differentially synchronize developing neocortical networks in vivo. J. Neurosci. 29, 9011–9025. doi: 10.1523/jneurosci.5646-08.2009
Yang, J. W., Reyes-Puerta, V., Kilb, W., and Luhmann, H. J. (2016). Spindle bursts in neonatal rat cerebral cortex. Neural Plast. 2016:3467832. doi: 10.1155/2016/3467832
Yao, X. H., Wang, M., He, X. N., He, F., Zhang, S. Q., Lu, W., et al. (2016). Electrical coupling regulates layer 1 interneuron microcircuit formation in the neocortex. Nat. Commun. 7:12229. doi: 10.1038/ncomms12229
Zhao, C., Kao, J. P., and Kanold, P. O. (2009). Functional excitatory microcircuits in neonatal cortex connect thalamus and layer 4. J. Neurosci. 29, 15479–15488. doi: 10.1523/jneurosci.4471-09.2009
Keywords: cortex, development, circuit, NMDA-receptor, silent synapse, subplate neuron
Citation: Kanold PO, Deng R and Meng X (2019) The Integrative Function of Silent Synapses on Subplate Neurons in Cortical Development and Dysfunction. Front. Neuroanat. 13:41. doi: 10.3389/fnana.2019.00041
Received: 04 February 2019; Accepted: 26 March 2019;
Published: 16 April 2019.
Edited by:
Shen-Ju Chou, Academia Sinica, TaiwanReviewed by:
Barry W. Connors, Brown University, United StatesMatthew T. Colonnese, George Washington University, United States
Zoltan Molnar, University of Oxford, United Kingdom
Copyright © 2019 Kanold, Deng and Meng. This is an open-access article distributed under the terms of the Creative Commons Attribution License (CC BY). The use, distribution or reproduction in other forums is permitted, provided the original author(s) and the copyright owner(s) are credited and that the original publication in this journal is cited, in accordance with accepted academic practice. No use, distribution or reproduction is permitted which does not comply with these terms.
*Correspondence: Patrick O. Kanold, cGthbm9sZEB1bWQuZWR1