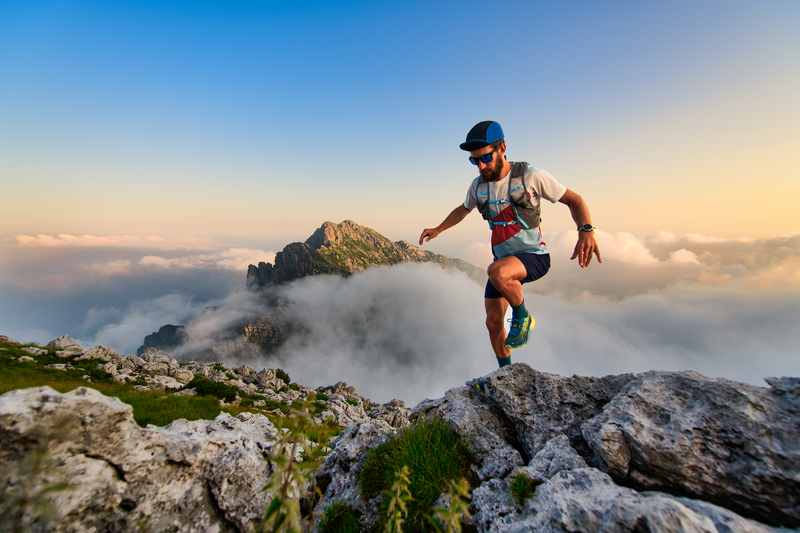
94% of researchers rate our articles as excellent or good
Learn more about the work of our research integrity team to safeguard the quality of each article we publish.
Find out more
REVIEW article
Front. Aging Neurosci. , 21 June 2018
Sec. Alzheimer's Disease and Related Dementias
Volume 10 - 2018 | https://doi.org/10.3389/fnagi.2018.00191
This article is part of the Research Topic Metabolic and Vascular Imaging Biomarkers for Brain Aging and Alzheimer’s Disease View all 20 articles
People with Down syndrome (DS) are at high risk for developing Alzheimer disease (AD). Neuropathology consistent with AD is present by 40 years of age and dementia may develop up to a decade later. In this review, we describe metabolic and vascular neuroimaging studies in DS that suggest these functional changes are a key feature of aging, linked to cognitive decline and AD in this vulnerable cohort. FDG-PET imaging in DS suggests systematic reductions in glucose metabolism in posterior cingulate and parietotemporal cortex. Magentic resonance spectroscopy studies show consistent decreases in neuronal health and increased myoinositol, suggesting inflammation. There are few vascular imaging studies in DS suggesting a gap in our knowledge. Future studies would benefit from longitudinal measures and combining various imaging approaches to identify early signs of dementia in DS that may be amenable to intervention.
The life expectancy of people with Down syndrome (DS) continues to increase due to improved health care and management of co-occurring illnesses (Bittles and Glasson, 2004). Consequently there are more people with DS and the population has grown from 49,923 in 1950 to 206, 336 in 2010 (de Graaf et al., 2017). However, mortality rate is higher in people with DS in older ages relative to the general population (Ng et al., 2017) and further, some deaths such as those due to respiratory disorders and epilepsy may be amenable to medical intervention (Hosking et al., 2016). As with the general population, the risk of developing health-related problems increases as people with DS get older. In particular, people with DS are at a high risk of developing cognitive impairment and clinical dementia after the age of 50 years (Zigman et al., 1996; Sinai et al., 2018). Virtually all adults with DS develop the neuropathology for a brain-based Alzheimer disease (AD) diagnosis by the age of 40 years (reviewed in Head et al., 2016). This is thought to be due to the lifelong overexpression of the APP gene on chromosome 21 leading to early onset and rapid accumulation of beta-amyloid (Aβ) with age (Head et al., 2016). Thus, by studying individuals with DS across the lifespan it is possible to identify early biomarkers of AD pathogenesis that may not be feasible in the general population as the age of onset of AD varies tremendously (e.g., 50-over 100 years). As will be discussed later in this review, cerebrovascular pathology may help to accelerate AD in DS and be an important contributor to dementia. Interestingly, a subset of adults with DS never develops dementia even in the presence of this AD pathology (Franceschi et al., 1990; Schupf and Sergievsky, 2002; Head et al., 2012a,b).
Neuroimaging studies help us detect structural, connectivity, activity, neurochemical, and vascular/metabolic functional changes with age and with the development of AD. As the development of AD neuropathology in DS is strongly age-dependent, we can learn about early changes associated with the progression of AD through neuroimaging studies in DS (Neale et al., 2018). These studies not only help us understand aging and AD in people with DS but can translate to our understanding of AD in the general population. For example, Jack and Holtzman (2013) proposed a hypothetical series of biomarker events including neuroimaging outcomes that may occur prior to changes in cognition and be predictive of decline in the general population. For example, Jack and colleagues suggest that Aβ can be detected prior to brain structure changes, which in turn are detectable prior to mild cognitive impairment and dementia. A similar series of biomarker events can be hypothetically applied for people with DS but using age as opposed to the clinical disease stage as the time axis. Whereas in the general population, biomarker changes reflecting progressive AD are plotted as a function of the cognitive continiuum, in DS we can use age as a representative to AD pathogenesis. It is clear from this hypothetical model that neuroimaging can provide early markers of dementia-associated brain changes and the inclusion of vascular or metabolic imaging may play an important role by providing even earlier information regarding AD pathogenesis.
Metabolic imaging using positron emission tomography (PET) has consistently shown reductions in glucose utilization in vulnerable brain regions in sporadic AD (Silverman and Phelps, 2001). In particular, the precuneus, posterior cingulate cortex and posterior parietotemporal lobes may be the earliest site of reduced glucose metabolism (FDG-PET) prior to onset of symptoms (Minoshima et al., 1997). In AD, the extent of cerebral metabolic rate of glucose from FDG-PET studies (CMRglu) is correlated with the severity of dementia (Minoshima et al., 1997). As AD progresses, more brain regions show declines in CMRglu such as the frontal cortex. CMRglu from FDG-PET can also predict AD neuropathology with 84–93% sensitivity and 73% specificity (Silverman et al., 2001; Jagust et al., 2007).
There are relatively few FDG-PET studies in DS with the majority being acquired under resting conditions (Table 1 summarizes studies since 1983). However the results of these studies have been relatively consistent. First, younger individuals with DS (without dementia) show increased glucose metabolism (Schwartz et al., 1983; Cutler, 1986; Azari et al., 1994a; Haier et al., 2003, 2008; Lengyel et al., 2006; Matthews et al., 2016) relative to age matched controls in all but one study (Schapiro et al., 1992b). The regions that show hypermetabolism include the prefrontal cortex, sensorimotor cortex, thalamus, inferior temporal/entorhinal cortex. Interestingly, increased glucose metabolic rate is associated with decreased gray matter volume in the temporal cortex including the parahippocampus/hippocampus suggesting that hypermetabolism is a compensatory response (Haier et al., 2008). Indeed, autopsy studies of the same brain region in a case series of DS shows evidence of neuronal sprouting positive for tau phosphorylation suggesting a mechanistic basis for increased glucose metabolism in middle age (Head et al., 2003). Other molecular events may also underlie this phenomenon (reviewed in Head et al., 2007).
In contrast, older individuals with DS and particularly those with dementia show hypometabolism in multiple studies (Schwartz et al., 1983; Cutler, 1986; Schapiro et al., 1992a; Azari et al., 1994a,b; Rafii et al., 2015; Sabbagh et al., 2015; Matthews et al., 2016). Brain regions that appear to be systematically affected under either resting or active conditions include the posterior cingulate cortex, hippocampus, parietal and temporal cortex consistent with reports in sporadic AD (Minoshima et al., 1997; Pietrini et al., 1997; Silverman and Phelps, 2001). Further, in a 45 year old female with mosaic/translation DS with clinical signs of early dementia, a pattern of hypometabolism similar to that of sporadic AD was observed (Schapiro et al., 1992a).
Reduced glucose metabolism in older adults with DS and dementia is associated with decreased cortical volumes (Matthews et al., 2016), increased amyloid binding with florbetapir (Matthews et al., 2016) and increased tau binding using AV-1451 (Rafii et al., 2017). Some studies report associations between cognition and glucose metabolism (Haier et al., 2008; Sabbagh et al., 2015; Matthews et al., 2016) but not all (Rafii et al., 2015), with variable results likely due to smaller sample sizes. In one of the only longitudinal studies that was found, Dani and colleagues reported stable glucose metabolic rates over a 7 year period of time unless clinical dementia had evolved (occurred in one person with DS) leading to rapid glucose metabolic decline in parietal and temporal cortices (Dani et al., 1996).
Reductions in glucose metabolism may lead to or reflect neuronal loss, synapse loss, and/or mitochondrial dysfunction. Given that all these events are thought to occur with age and dementia in DS (Head et al., 2016), PET imaging can provide useful information with respect to brain function but there is a clear need for more longitudinal studies that includes measures of cognition. It is also notable that despite the posterior cingulate cortex being an early site of glucose metabolic losses, there are few studies of AD neuropathology in this region in DS. The use of FDG-PET to capture information about metabolism requires the use of intravenous injections of radioactive ligands. This procedure may be problematic for some participants, their families and particularly for those with dementia. However, as an outcome measure that may reflect a rapid response to treatment that is targeting metabolism, FDG-PET has utility. In future, similar outcomes reflecting metabolic changes such as blood flow, may be obtainable using relatively short MR protocols such as arterial spin labeling (7 min). Further, as will be discussed next, magnetic resonance spectroscopy, which is also a relatively short protocol (5 min) that may be useful for a broader spectrum of participants can provide specific metabolic markers that could help dissect the different neuronal/glial pathways that signal onset of dementia.
Proton magnetic resonance spectroscopy (1H-MRS) has been widely used to characterize the neurochemistry of brain health and disease. In particular, the neuronal markers of N-acetylaspartate (NAA) and glutamate-glutamine complex (Glx) decrease, and the glial marker of myo-inositol (MI) increases, both correlate with clinical variables in aging and AD (Parnetti et al., 1997; Lin and Rothman, 2014). It is thought that lower levels of NAA or Glx reflects neuronal loss or injury; neuroinflammation is associated with activated astrocytes and microglial cells leading to increased MI (Chang et al., 2013). The ratio of NAA to MI can also be used to distinguish non-demented from demented people (cf. Lin et al., 2005).
In DS, there have been several studies using MRS with assessments done for posterior cingulate cortex, hippocampus, frontal cortex, occipital cortex, and parietal cortex with comparisons to age matched controls (Table 2). Decreased NAA and increased MI is observed relatively consistently across studies in non-demented adults with DS compared with age matched non-DS controls (Shonk and Ross, 1995; Berry et al., 1999; Huang et al., 1999; Beacher et al., 2005; Lamar et al., 2011; Lin et al., 2016) with a few exceptions (Murata et al., 1993; Smigielska-Kuzia et al., 2010). Hippocampal Glx was not different in people with DS from controls (Tan et al., 2014). It may not be surprising that MI levels are generally higher in people with DS as the MI cotransporter (SLC5A3) gene is on chromosome 21 and is overexpressed in DS (Berry et al., 1995). Further, synaptojanin 1 (gene also on chromosome 21) can lead to increased gliosis (Herrera et al., 2009) and thus possibly, higher MI levels.
With age, older people with DS show higher MI and lower NAA than younger people with DS. MI was higher in the occipital and parietal cortex of older DS subjects relative to younger people with DS (Huang et al., 1999). In the hippocampus of older adults with dementia with DS, MI is also higher and NAA lower when compared to non-demented people with DS (Lamar et al., 2011) but Glx is unchanged (Tan et al., 2014). In the posterior cingulate cortex, MI was not significantly different in people with DS who were demented compared with those who were not demented, but NAA was significantly decreased (Lin et al., 2016). However, there is a case report of an individual with DS who was demented showing higher MI and lower NAA relative to non-demented DS individuals (Shonk and Ross, 1995). Further increases in MI reported in some studies with aging and dementia may reflect increased neuroinflammation that has been observed with aging in DS (Wilcock, 2012).
Thus, MRS provides novel information and unique signatures for DS (e.g., higher MI) but also may be amenable to future treatment studies as metabolic outcomes measured by MRS may be rapidly modifiable as opposed to outcomes reflecting brain structure. Comparing MRS outcomes in different affected brain regions in people with DS (e.g., comparing hippocampus, frontal cortex, cingulate cortex) may provide novel links between the presence of in vivo amyloid by PET imaging and glial/neuronal consequences. For example, as amyloid PiB binding increases with age, how does NAA or MI decrease or increase correspondingly? These studies may lead us to novel interventions in future for DS with outcome measures and a further examination of the link between MRS outcomes, brain region, and cognition will be useful in future.
Cerebrovascular pathology occurs in over 85% of autopsy cases presenting with AD neuropathology and is associated with impaired cognition (Arvanitakis et al., 2016). One form of this pathology, cerebral amyloid angiopathy (CAA) is present in near all brains of people with AD (Viswanathan and Greenberg, 2011). Thus, there is an increasing recognition that along with the development of Aβ plaques and neurofibrillary tangles, vascular neuropathology may also affect cognition and the progression of dementia (White et al., 2002). Interestingly, in DS, there is significant cerebrovascular neuropathology in the form of CAA, primarily due to the overexpression of APP and Aβ (Ikeda et al., 1994; Iwatsubo et al., 1995; Mendel et al., 2010; Head et al., 2017; Zis and Strydom, 2018).
Extensive CAA is associated with microhemorrhages and strokes in general (Arvanitakis et al., 2017; Banerjee et al., 2017) although in DS, stroke is relatively rare (Buss et al., 2016). Nonetheless, CAA may have a significant impact on blood vessel function. CAA can lead to deficits in cerebrovascular regulation (Grinberg et al., 2012) and reduced blood flow may lead to impaired perivascular clearance of Aβ. Impaired clearance will in turn lead to additional accumulation of Aβ (Banerjee et al., 2017).
Neuroimaging of CAA is typically by GRE or T2*-weighted MRI (Fazekas et al., 1999). There is only one neuroimaging study using T2* to observe the extent of CAA in vivo in older adults with DS (Carmona-Iragui et al., 2017). CAA was observed in 31% of cognitively impaired people with DS, which is similar to early onset AD (38%) and higher than sporadic AD (12%). In addition, 15.4% of people with DS had evidence of intracerebral hemorrhages. Thus, CAA is a consistent feature of aging and dementia in DS and may serve as a future target for clinical trials.
While PET-based studies in DS show metabolic differences that mirror AD in the general population, changes in blood flow may also be seen in DS. For example, single photon emission computed tomography (SPECT) patterns in younger individuals with DS reveal perfusion changes in temporal, parietal, and occipital regions (Kao et al., 1993) that are also reminiscent of those seen in AD (DeKosky et al., 1990). However, these regional differences in perfusion may reflect the added impact of CAA-associated or other cerebrovascular mechanisms in DS.
Cerebrovascular dysfunction measured in vivo may be critical for understanding not only the aging process and progression to AD in DS but treatment that rely on and are also relatively short MR protocols (T2*~7 min, FLAIR~4.5 min) (Figure 1). Immunotherapy trials in patients with AD suggest that cerebrovascular adverse effects can occur and are visualized with FLAIR (Sperling et al., 2012). The possibility of a similar outcome in DS is as yet unknown. Intervention studies that target Aβ or other pathways may be less effective in people with DS with significant cerebrovascular pathology and can confound the opportunity to observe benefits in clinical trials. Characterizing the extent of cerebrovascular pathology may serve as an exclusion/inclusion criteria or included as a covariate so as not to obscure positive clinical outcomes.
Figure 1. Representative examples of neuroimaging protocols acquired in Down syndrome. Panels (A–D) show MR imaging in a 57 year old male and 59 year old male imaged with T2* (A, C) and FLAIR (B, D) showing the presence of microbleeds. Arrows distinguish edema and hemosiderin positive microbleeds. Panel (E) shows examples of amyloid PiB-PET imaging (top) and FDG-PET imaging (bottom). Images from (A–D) were modified from Figure 5 with permission of Elsevier Press (Head et al., 2018). Images from (E) were modified from Figure 1 reproduced with permission from Dr. M. Rafii and under the Creative Commons Attribution License (CC BY) (Rafii et al., 2015).
Structural differences in childhood and early adulthood suggest that some brain regions (e.g.,) are smaller in people with DS whereas others (parahippocampal gyrus) may be larger (Kesslak et al., 1994; Teipel and Hampel, 2006). It will be important to consider additional volumetric tissue losses using structural MRI when interpreting reductions in vascular flow or metabolic outcomes. Additional atrophy occurs with aging in DS and with the development of dementia with posterior cingulate, parietal, temporal, and frontal regions being affected (summarized nicely in Neale et al., 2018).
In studies of people with DS, sample sizes are typically smaller. This is due to challenges with recruitment, the ability of people to be scanned (e.g., fear) or to stay motionless (movement artifacts, people with dementia; Neale et al., 2018). Obesity or being overweight can lead to discomfort in the scanner and in some cases, may preclude a person from participating. The neck and facial structure of people with DS also can lead to discomfort in the prone position. For these situations, there are methods to provide additional padding and support along with frequent pauses in the procedures. Unfortunately, in many studies this leads to small sample sizes of demented individuals with DS, which may skew our results. Anxiolytics can be helpful when obtaining structural images but may interfere with functional measures. In our own experiences, we have found that repeated visits leads to greater successes with our volunteers participating in the scanning procedures and the option of anxiolytics has been helpful. Age of the participant also influences success with scanning. Estimates from our cohort suggest that a full set of images (MPRAGE, FLAIR, T2*, ASL, MRS, DTI ~50 min), 92% of people 25–37 years, 82% of 37–50 year olds and 40% of 50–65 year olds can be successfully scanned (unpublished observations from the University of Kentucky Down syndrome and Aging study). However, there are fewer sets of full imaging protocols we can acquire with increasing age as our participants may not be able to stay in the scanner as long as we require.
Longitudinal studies in virtually all of the imaging parameters discussed here are critical. There are few longitudinal studies of metabolic and vascular neuroimaging changes with age in DS. In studies of structural imaging some show progressive atrophy (reviewed in Neale et al., 2018). Over a 3 year period of time, studies in non-demented adults with DS report an increasing number of individuals developing amyloid by PET (PiB binding) and those with existing amyloid binding showed an increasing number of brain regions affected along with increased accumulation within affected brain regions (Hartley et al., 2017; Lao et al., 2017; also reviewed in Neale et al., 2018) (Figure 1). It is interesting to note that PiB tends to bind more mature amyloid deposits (LeVine et al., 2017) in vitro consistent with the typical age of onset of PiB binding in DS being after 40 years of age. In summary, neuroimaging is a powerful tool to detect structural, metabolic and vascular changes with age and dementia in DS but there are still important gaps in our knowledge remaining. Feasibility concerns may be overcome with the use of mock scanners, increasing sample sizes (based upon estimates of scan success as a function of age and dementia) and reducing scan times. Given that neuroimaging outcomes could be critically important in future clinical trials, it will be important to encourage further studies for people with DS.
All authors listed have made a substantial, direct, and intellectual contribution to the work, and approved it for publication.
The authors declare that the research was conducted in the absence of any commercial or financial relationships that could be construed as a potential conflict of interest.
The authors are supported by NIH/NICHD R01HD064993. The authors thank Dr. M. Rafii for kindly allowing us to reproduce the figure of Amyloid and FDG PET imaging.
Arvanitakis, Z., Capuano, A. W., Leurgans, S. E., Bennett, D. A., and Schneider, J. A. (2016). Relation of cerebral vessel disease to Alzheimer's disease dementia and cognitive function in elderly people: a cross-sectional study. Lancet Neurol. 15, 934–943. doi: 10.1016/S1474-4422(16)30029-1
Arvanitakis, Z., Capuano, A. W., Leurgans, S. E., Buchman, A. S., Bennett, D. A., and Schneider, J. A. (2017). The relationship of cerebral vessel pathology to brain microinfarcts. Brain Pathol. 27, 77–85. doi: 10.1111/bpa.12365
Azari, N. P., Horwitz, B., Pettigrew, K. D., Grady, C. L., Haxby, J. V., Giacometti, K. R., et al. (1994a). Abnormal pattern of cerebral glucose metabolic rates involving language areas in young adults with Down syndrome. Brain Lang. 46, 1–20. doi: 10.1006/brln.1994.1001
Azari, N. P., Pettigrew, K. D., Pietrini, P., Horwitz, B., and Schapiro, M. B. (1994b). Detection of an Alzheimer disease pattern of cerebral metabolism in Down syndrome. Dementia 5, 69–78. doi: 10.1159/000106700
Banerjee, G., Carare, R., Cordonnier, C., Greenberg, S. M., Schneider, J. A., Smith, E. E., et al. (2017). The increasing impact of cerebral amyloid angiopathy: essential new insights for clinical practice. J. Neurol. Neurosurg. Psychiatr. 88, 982–994. doi: 10.1136/jnnp-2016-314697
Beacher, F., Simmons, A., Daly, E., Prasher, V., Adams, C., Margallo-Lana, M. L., et al. (2005). Hippocampal myo-inositol and cognitive ability in adults with Down syndrome: an in vivo proton magnetic resonance spectroscopy study. Arch. Gen. Psychiatry 62, 1360–1365. doi: 10.1001/archpsyc.62.12.1360
Berry, G. T., Mallee, J. J., Kwon, H. M., Rim, J. S., Mulla, W. R., Muenke, M., et al. (1995). The human osmoregulatory Na+/myo-inositol cotransporter gene SLC5A3): molecular cloning and localization to chromosome 21. Genomics 25, 507–513. doi: 10.1016/0888-7543(95)80052-N
Berry, G. T., Wang, Z. J., Dreha, S. F., Finucane, B. M., and Zimmerman, R. A. (1999). In vivo brain myo-inositol levels in children with Down syndrome. J. Pediatr. 135, 94–97. doi: 10.1016/S0022-3476(99)70334-3
Bittles, A. H., and Glasson, E. J. (2004). Clinical, social, and ethical implications of changing life expectancy in Down syndrome. Dev. Med. Child Neurol. 46, 282–286. doi: 10.1111/j.1469-8749.2004.tb00483.x
Buss, L., Fisher, E., Hardy, J., Nizetic, D., Groet, J., Pulford, L., et al. (2016). Intracerebral haemorrhage in Down syndrome: protected or predisposed? F1000Res. 5:F1000 Faculty Rev-876. doi: 10.12688/f1000research.7819.1
Carmona-Iragui, M., Balasa, M., Benejam, B., Alcolea, D., Fernández, S., Videla, L., et al. (2017). Cerebral amyloid angiopathy in Down syndrome and sporadic and autosomal-dominant Alzheimer's disease. Alzheimers. Dement. 13, 1251–1260. doi: 10.1016/j.jalz.2017.03.007
Chang, L., Munsaka, S. M., Kraft-Terry, S., and Ernst, T. (2013). Magnetic resonance spectroscopy to assess neuroinflammation and neuropathic pain. J. Neuroimmune Pharmacol. 8, 576–593. doi: 10.1007/s11481-013-9460-x
Cutler, N. R. (1986). Cerebral metabolism as measured with positron emission tomography (PET) and [18F] 2-deoxy-D-glucose: healthy aging, Alzheimer's disease and Down syndrome. Prog. Neuropsychopharmacol. Biol. Psychiatry 10, 309–321. doi: 10.1016/0278-5846(86)90010-2
Dani, A., Pietrini, P., Furey, M. L., McIntosh, A. R., Grady, C. L., Horwitz, B., et al. (1996). Brain cognition and metabolism in Down syndrome adults in association with development of dementia. Neuroreport 7, 2933–2936. doi: 10.1097/00001756-199611250-00026
de Graaf, G., Buckley, F., and Skotko, B. G. (2017). Estimation of the number of people with Down syndrome in the United States. Genet. Med. 19, 439–447. doi: 10.1038/gim.2016.127
DeKosky, S. T., Shih, W. J., Schmitt, F. A., Coupal, J., and Kirkpatrick, C. (1990). Assessing utility of single photon emission computed tomography (SPECT) scan in Alzheimer disease: correlation with cognitive severity. Alzheimer Dis. Assoc. Disord. 4, 14–23. doi: 10.1097/00002093-199040100-00002
Fazekas, F., Kleinert, R., Roob, G., Kleinert, G., Kapeller, P., Schmidt, R., et al. (1999). Histopathologic analysis of foci of signal loss on gradient-echo T2*-weighted MR images in patients with spontaneous intracerebral hemorrhage: evidence of microangiopathy-related microbleeds. AJNR Am. J. Neuroradiol. 20, 637–642.
Franceschi, M., Comola, M., Piattoni, F., Gualandri, W., and Canal, N. (1990). Prevalence of dementia in adult patients with trisomy 21. Am. J. Med. Genet. 7, 306–308. doi: 10.1002/ajmg.1320370760
Grinberg, L. T., Korczyn, A. D., and Heinsen, H. (2012). Cerebral amyloid angiopathy impact on endothelium. Exp. Gerontol. 47, 838–842. doi: 10.1016/j.exger.2012.08.005
Haier, R. J., Alkire, M. T., White, N. S., Uncapher, M. R., Head, E., Lott, I. T., et al. (2003). Temporal cortex hypermetabolism in Down syndrome prior to the onset of dementia. Neurology 61, 1673–1679. doi: 10.1212/01.WNL.0000098935.36984.25
Haier, R. J., Head, K., Head, E., and Lott, I. T. (2008). Neuroimaging of individuals with Down's syndrome at-risk for dementia: evidence for possible compensatory events. Neuroimage 39, 1324–1332. doi: 10.1016/j.neuroimage.2007.09.064
Hartley, S. L., Handen, B. L., Devenny, D., Mihaila, I., Hardison, R., Lao, P. J., et al. (2017). Cognitive decline and brain amyloid-beta accumulation across 3 years in adults with Down syndrome. Neurobiol. Aging 58, 68–76. doi: 10.1016/j.neurobiolaging.2017.05.019
Head, E., Helman, A. M., Powell, D., and Schmitt, F. A. (2018). Down syndrome, beta-amyloid and neuroimaging. Free Radic. Biol. Med. 114, 102–109. doi: 10.1016/j.freeradbiomed.2017.09.013
Head, E., Lott, I. T., Hof, P. R., Bouras, C., Su, J. H., Kim, R., et al. (2003). Parallel compensatory and pathological events associated with tau pathology in middle aged individuals with down syndrome. J. Neuropath. Exp. Neurol. 62, 917–926. doi: 10.1093/jnen/62.9.917
Head, E., Lott, I. T., Patterson, D., Doran, E., and Haier, R. J. (2007). Possible compensatory events in adult Down syndrome brain prior to the development of Alzheimer disease neuropathology: targets for nonpharmacological intervention. J. Alzheimers. Dis. 11, 61–76. doi: 10.3233/JAD-2007-11110
Head, E., Lott, I. T., Wilcock, D. M., and Lemere, C. A. (2016). Aging in Down Syndrome and the Development of Alzheimer's Disease Neuropathology. Curr. Alzheimer Res. 13, 18–29. doi: 10.2174/1567205012666151020114607
Head, E., Phelan, M. J., Doran, E., Kim, R. C., Poon, W. W., Schmitt, F. A., et al. (2017). Cerebrovascular pathology in Down syndrome and Alzheimer disease. Acta Neuropathol. Commun. 5:93. doi: 10.1186/s40478-017-0499-4
Head, E., Powell, D., Gold, B. T., and Schmitt, F. A. (2012a). Alzheimer's disease in Down syndrome. Eur. J. Neurodegenerative Dis. 1, 353–364.
Head, E., Silverman, W., Patterson, D., and Lott, I. T. (2012b). Aging and down syndrome. Curr. Gerontol. Geriatr. Res. 2012:412536. doi: 10.1155/2012/412536
Herrera, F., Chen, Q., Fischer, W. H., Maher, P., and Schubert, D. R. (2009). Synaptojanin-1 plays a key role in astrogliogenesis: possible relevance for Down's syndrome. Cell Death Differ. 16, 910–920. doi: 10.1038/cdd.2009.24
Horwitz, B., Schapiro, M. B., Grady, C. L., and Rapoport, S. I. (1990). Cerebral metabolic pattern in young adult Down's syndrome subjects: altered intercorrelations between regional rates of glucose utilization. J. Ment. Defic. Res. 34 (Pt 3), 237–252. doi: 10.1111/j.1365-2788.1990.tb01535.x
Hosking, F. J., Carey, I. M., Shah, S. M., Harris, T., DeWilde, S., Beighton, C., et al. (2016). Mortality among adults with intellectual disability in england: comparisons with the general population. Am. J. Public Health 106, 1483–1490. doi: 10.2105/AJPH.2016.303240
Huang, W., Alexander, G. E., Daly, E. M., Shetty, H. U., Krasuski, J. S., Rapoport, S. I., et al. (1999). High brain myo-inositol levels in the predementia phase of Alzheimer's disease in adults with Down's syndrome: a 1H MRS study. Am. J. Psychiatry 156, 1879–1886.
Ikeda, S., Tokuda, T., Yanagisawa, N., Kametani, F., Ohshima, T., and Allsop, D. (1994). Variability of beta-amyloid protein deposited lesions in Down's syndrome brains. Tohoku J. Exp. Med. 174, 189–198. doi: 10.1620/tjem.174.189
Iwatsubo, T., Mann, D. M., Odaka, A., Suzuki, N., and Ihara, Y. (1995). Amyloid beta protein (A beta) deposition: a beta 42(43) precedes A beta 40 in Down syndrome. Ann. Neurol. 37, 294–299. doi: 10.1002/ana.410370305
Jack, C. R., and Holtzman, D. M. (2013). Biomarker modeling of Alzheimer's disease. Neuron 80, 1347–1358. doi: 10.1016/j.neuron.2013.12.003
Jagust, W., Reed, B., Mungas, D., Ellis, W., and Decarli, C. (2007). What does fluorodeoxyglucose PET imaging add to a clinical diagnosis of dementia? Neurology 69, 871–877. doi: 10.1212/01.wnl.0000269790.05105.16
Kao, C. H., Wang, P. Y., Wang, S. J., Chou, K. T., Hsu, C. Y., Lin, W. Y., et al. (1993). Regional cerebral blood flow of Alzheimer's disease-like pattern in young patients with Down's syndrome detected by 99Tcm-HMPAO brain SPECT. Nucl. Med. Commun. 14, 47–51. doi: 10.1097/00006231-199301000-00010
Kesslak, J. P., Nagata, S. F., Lott, I., and Nalcioglu, O. (1994). Magnetic resonance imaging analysis of age-related changes in the brains of individuals with Down's syndrome. Neurology 44, 1039–1045. doi: 10.1212/WNL.44.6.1039
Lamar, M., Foy, C. M., Beacher, F., Daly, E., Poppe, M., Archer, N., et al. (2011). Down syndrome with and without dementia: an in vivo proton magnetic resonance spectroscopy study with implications for Alzheimer's disease. Neuroimage 57, 63–68. doi: 10.1016/j.neuroimage.2011.03.073
Lao, P. J., Handen, B. L., Betthauser, T. J., Mihaila, I., Hartley, S. L., Cohen, A. D., et al. (2018). Alzheimer-Like Pattern of hypometabolism emerges with elevated amyloid-beta burden in down syndrome. J. Alzheimers. Dis. 61, 631–644. doi: 10.3233/JAD-170720
Lao, P. J., Handen, B. L., Betthauser, T. J., Mihaila, I., Hartley, S. L., Cohen, A. D., et al. (2017). Longitudinal changes in amyloid positron emission tomography and volumetric magnetic resonance imaging in the nondemented Down syndrome population. Alzheimer's Dementia 9, 1–9. doi: 10.1016/j.dadm.2017.05.001
Lengyel, Z., Balogh, E., Emri, M., Szikszai, E., Kollar, J., Sikula, J., et al. (2006). Pattern of increased cerebral FDG uptake in down syndrome patients. Pediatr. Neurol. 34, 270–275. doi: 10.1016/j.pediatrneurol.2005.08.035
LeVine, H. 3rd, Spielmann, H. P., Matveev, S., Cauvi, F. M., Murphy, M. P., Beckett, T. L., et al. (2017). Down syndrome: age-dependence of PiB binding in postmortem frontal cortex across the lifespan. Neurobiol. Aging 54, 163–169. doi: 10.1016/j.neurobiolaging.2017.03.005
Lin, A. L., and Rothman, D. L. (2014). What have novel imaging techniques revealed about metabolism in the aging brain? Future Neurol. 9, 341–354. doi: 10.2217/fnl.14.13
Lin, A. L., Powell, D., Caban-Holt, A., Jicha, G., Robertson, W., Gold, B. T., et al. (2016). 1H-MRS metabolites in adults with Down syndrome: effects of dementia. NeuroImage Clin. 11, 728–735. doi: 10.1016/j.nicl.2016.06.001
Lin, A., Ross, B. D., Harris, K., and Wong, W. (2005). Efficacy of proton magnetic resonance spectroscopy in neurological diagnosis and neurotherapeutic decision making. NeuroRx 2, 197–214. doi: 10.1602/neurorx.2.2.197
Matthews, D. C., Lukic, A. S., Andrews, R. D., Marendic, B., Brewer, J., Rissman, R. A., et al. (2016). Dissociation of Down syndrome and Alzheimer's disease effects with imaging. Alzheimers Dement 2, 69–81. doi: 10.1016/j.trci.2016.02.004
Mendel, T., Bertrand, E., Szpak, G. M., Stepien, T., and Wierzba-Bobrowicz, T. (2010). Cerebral amyloid angiopathy as a cause of an extensive brain hemorrhage in adult patient with Down's syndrome - a case report. Folia Neuropathol. 48, 206–211.
Minoshima, S., Giordani, B., Berent, S., Frey, K. A., Foster, N. L., and Kuhl, D. E. (1997). Metabolic reduction in the posterior cingulate cortex in very early Alzheimer's disease. Ann. Neurol. 42, 85–94. doi: 10.1002/ana.410420114
Murata, T., Koshino, Y., Omori, M., Murata, I., Nishio, M., Horie, T., et al. (1993). In vivo proton magnetic resonance spectroscopy study on premature aging in adult Down's syndrome. Biol. Psychiatry 34, 290–297. doi: 10.1016/0006-3223(93)90086-S
Neale, N., Padilla, C., Fonseca, L. M., Holland, T., and Zaman, S. (2018). Neuroimaging and other modalities to assess Alzheimer's disease in Down syndrome. NeuroImage Clin. 17, 263–271. doi: 10.1016/j.nicl.2017.10.022
Ng, N., Flygare Wallen, E., and Ahlstrom, G. (2017). Mortality patterns and risk among older men and women with intellectual disability: a Swedish national retrospective cohort study. BMC Geriatr. 17:269. doi: 10.1186/s12877-017-0665-3
Parnetti, L., Tarducci, R., Presciutti, O., Lowenthal, D. T., Pippi, M., Palumbo, B., et al. (1997). Proton magnetic resonance spectroscopy can differentiate Alzheimer's disease from normal aging. Mech. Ageing Dev. 97, 9–14. doi: 10.1016/S0047-6374(97)01877-0
Pietrini, P., Dani, A., Furey, M. L., Alexander, G. E., Freo, U., Grady, C. L., et al. (1997). Low glucose metabolism during brain stimulation in older Down's syndrome subjects at risk for Alzheimer's disease prior to dementia. Am. J. Psychiatry 154, 1063–1069. doi: 10.1176/ajp.154.8.1063
Rafii, M. S., Lukic, A. S., Andrews, R. D., Brewer, J., Rissman, R. A., Strother, S. C., et al. (2017). PET Imaging of Tau Pathology and Relationship to Amyloid, Longitudinal, MRI and Cognitive Change in Down Syndrome: results From the Down Syndrome Biomarker Initiative (DSBI). J. Alzheimers Dis. 60, 439–450. doi: 10.3233/JAD-170390
Rafii, M. S., Wishnek, H., Brewer, J. B., Donohue, M. C., Ness, S., Mobley, W. C., et al. (2015). The down syndrome biomarker initiative (DSBI) pilot: proof of concept for deep phenotyping of Alzheimer's disease biomarkers in down syndrome. Front. Behav. Neurosci. 9:239. doi: 10.3389/fnbeh.2015.00239
Sabbagh, M. N., Chen, K., Rogers, J., Fleisher, A. S., Liebsack, C., Bandy, D., et al. (2015). Florbetapir, PET, FDG PET, and MRI in Down syndrome individuals with and without Alzheimer's dementia. Alzheimers Dement 11, 994–1004. doi: 10.1016/j.jalz.2015.01.006
Schapiro, M. B., Azari, N. P., Grady, C. L., Haxby, J. V., and Horwitz, B. (1992a). Down syndrome: differentiating mental retardation and dementia with brain imaging techniques. Prog. Clin. Biol. Res. 379, 103–122.
Schapiro, M. B., Ball, M. J., Grady, C. L., Haxby, J. V., Kaye, J. A., and Rapoport, S. I. (1988). Dementia in Down's syndrome: cerebral glucose utilization, neuropsychological assessment, and neuropathology. Neurology 38, 938–942. doi: 10.1212/WNL.38.6.938
Schapiro, M. B., Grady, C. L., Kumar, A., Herscovitch, P., Haxby, J. V., Moore, A. M., et al. (1990). Regional cerebral glucose metabolism is normal in young adults with Down syndrome. J. Cereb. Blood Flow Metab. 10, 199–206. doi: 10.1038/jcbfm.1990.35
Schapiro, M. B., Haxby, J. V., and Grady, C. L. (1992b). Nature of mental retardation and dementia in Down syndrome: study with PET, CT, and neuropsychology. Neurobiol. Aging 13, 723–734. doi: 10.1016/0197-4580(92)90096-G
Schupf, N., and Sergievsky, G. H. (2002). Genetic and host factors for dementia in Down's syndrome. Br. J. Psychiatry 180, 405–410. doi: 10.1192/bjp.180.5.405
Schwartz, M., Duara, R., Haxby, J., Grady, C., White, B. J., Kessler, R. M., et al. (1983). Down's syndrome in adults: brain metabolism. Science 221, 781–783. doi: 10.1126/science.6224294
Shonk, T., and Ross, B. D. (1995). Role of increased cerebral myo-inositol in the dementia of Down syndrome. Magn. Reson. Med. 33, 858–861. doi: 10.1002/mrm.1910330619
Silverman, D. H., and Phelps, M. E. (2001). Application of positron emission tomography for evaluation of metabolism and blood flow in human brain: normal development, aging, dementia, and stroke. Mol. Genet. Metab. 74, 128–138. doi: 10.1006/mgme.2001.3236
Silverman, D. H., Small, G. W., Chang, C. Y., Lu, C. S., Kung De Aburto, M. A., Chen, Wm, et al. (2001). Positron emission tomography in evaluation of dementia: regional brain metabolism and long-term outcome. JAMA 286, 2120–2127. doi: 10.1001/jama.286.17.2120
Sinai, A., Mokrysz, C., Bernal, J., Bohnen, I., Bonell, S., Courtenay, K., et al. (2018). Predictors of age of diagnosis and survival of Alzheimer's Disease in down syndrome. J. Alzheimers. Dis. 61, 717–728. doi: 10.3233/JAD-170624
Smigielska-Kuzia, J., and Sobaniec, W. (2007). Brain metabolic profile obtained by proton magnetic resonance spectroscopy HMRS in children with Down syndrome. Adv. Med. Sci. 52 (Suppl. 1), 183–187.
Smigielska-Kuzia, J., Bockowski, L., Sobaniec, W., Kulak, W., and Sendrowski, K. (2010). Amino acid metabolic processes in the temporal lobes assessed by proton magnetic resonance spectroscopy (1H MRS) in children with Down syndrome. Pharmacol. Rep. 63, 1070–1077. doi: 10.1016/S1734-1140(10)70369-8
Sperling, R., Salloway, S., Brooks, D. J., Tampieri, D., Barakos, J., Fox, N. C., et al. (2012). Amyloid-related imaging abnormalities in patients with Alzheimer's disease treated with bapineuzumab: a retrospective analysis. Lancet Neurol. 11, 241–249. doi: 10.1016/S1474-4422(12)70015-7
Tan, G. M., Beacher, F., Daly, E., Horder, J., Prasher, V., Hanney, M. L., et al. (2014). Hippocampal glutamate-glutamine (Glx) in adults with Down syndrome: a preliminary study using in vivo proton magnetic resonance spectroscopy (1H MRS). J. Neurodev. Disord. 6:42. doi: 10.1186/1866-1955-6-42
Teipel, S. J., and Hampel, H. (2006). Neuroanatomy of Down syndrome in vivo: a model of preclinical Alzheimer's disease. Behav. Genet. 36, 405–415. doi: 10.1007/s10519-006-9047-x
Viswanathan, A., and Greenberg, S. M. (2011). Cerebral amyloid angiopathy in the elderly. Ann. Neurol. 70, 871–880. doi: 10.1002/ana.22516
White, L., Petrovitch, H., Hardman, J., Nelson, J., Davis, D. G., Ross, G. W., et al. (2002). Cerebrovascular pathology and dementia in autopsied Honolulu-Asia Aging Study participants. Ann. N. Y. Acad. Sci. 977, 9–23. doi: 10.1111/j.1749-6632.2002.tb04794.x
Wilcock, D. M. (2012). Neuroinflammation in the aging down syndrome brain; lessons from Alzheimer's disease. Curr. Gerontol. Geriatr. Res. 2012:170276. doi: 10.1155/2012/170276
Zigman, W. B., Schupf, N., Sersen, E., and Silverman, W. (1996). Prevalence of dementia in adults with and without Down syndrome. Am. J. Ment. Retard. 100, 403–412.
Keywords: dementia, FDG-PET, hypometabolism, hypermetabolism, myoinositol, MR spectroscopy, T2*, trisomy 21
Citation: Head E, Powell DK and Schmitt FA (2018) Metabolic and Vascular Imaging Biomarkers in Down Syndrome Provide Unique Insights Into Brain Aging and Alzheimer Disease Pathogenesis. Front. Aging Neurosci. 10:191. doi: 10.3389/fnagi.2018.00191
Received: 03 April 2018; Accepted: 06 June 2018;
Published: 21 June 2018.
Edited by:
Fahmeed Hyder, Yale University, United StatesReviewed by:
Andre Strydom, University College London, United KingdomCopyright © 2018 Head, Powell and Schmitt. This is an open-access article distributed under the terms of the Creative Commons Attribution License (CC BY). The use, distribution or reproduction in other forums is permitted, provided the original author(s) and the copyright owner are credited and that the original publication in this journal is cited, in accordance with accepted academic practice. No use, distribution or reproduction is permitted which does not comply with these terms.
*Correspondence: Elizabeth Head, ZWxpemFiZXRoLmhlYWRAdWt5LmVkdQ==
Disclaimer: All claims expressed in this article are solely those of the authors and do not necessarily represent those of their affiliated organizations, or those of the publisher, the editors and the reviewers. Any product that may be evaluated in this article or claim that may be made by its manufacturer is not guaranteed or endorsed by the publisher.
Research integrity at Frontiers
Learn more about the work of our research integrity team to safeguard the quality of each article we publish.