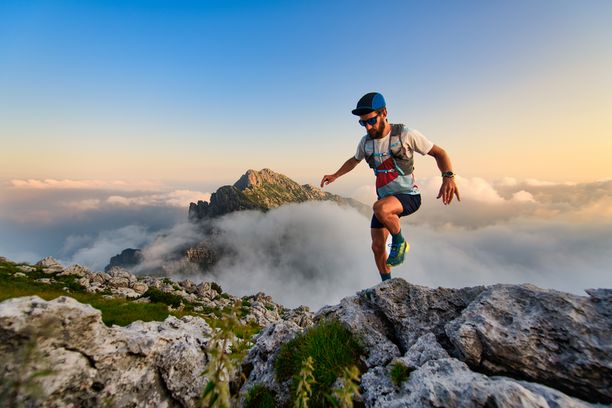
94% of researchers rate our articles as excellent or good
Learn more about the work of our research integrity team to safeguard the quality of each article we publish.
Find out more
MINI REVIEW article
Front. Aging Neurosci., 05 June 2018
Sec. Neurocognitive Aging and Behavior
Volume 10 - 2018 | https://doi.org/10.3389/fnagi.2018.00170
This article is part of the Research TopicMetabolic and Vascular Imaging Biomarkers for Brain Aging and Alzheimer’s DiseaseView all 20 articles
Alzheimer’s disease (AD) is associated with well-established macrostructural and cellular markers, including localized brain atrophy and deposition of amyloid. However, there is growing recognition of the link between cerebrovascular dysfunction and AD, supported by continuous experimental evidence in the animal and human literature. As a result, neuroimaging studies of AD are increasingly aiming to incorporate vascular measures, exemplified by measures of cerebrovascular reactivity (CVR). CVR is a measure that is rooted in clinical practice, and as non-invasive CVR-mapping techniques become more widely available, routine CVR mapping may open up new avenues of investigation into the development of AD. This review focuses on the use of MRI to map CVR, paying specific attention to recent developments in MRI methodology and on the emerging stimulus-free approaches to CVR mapping. It also summarizes the biological basis for the vascular contribution to AD, and provides critical perspective on the choice of CVR-mapping techniques amongst frail populations.
The brain’s energy needs are mainly met by neurovascular regulation of cerebral blood flow (CBF) (Roy and Sherrington, 1890; Duling and Berne, 1970), realized by the neurovascular unit (NVU). The NVU consists of arterial/arteriolar vascular smooth-muscle cells (VMSCs), endothelial cells, neuroglia (notably astrocytes), and pericytes. Pericytes play a crucial role in the formation and functionality of the selectively permeable space that is the blood–brain barrier (BBB), and BBB disruption is a classic marker of vascular dysfunction. Neurovascular dysfunction leads to failure to meet neuronal energy needs, which leads to oxidative stress and eventual neuronal death.
While the 𝜀4 allele of the apolipoprotein E (APOE) gene is an acknowledged genetic risk factor found in 40–80% of Alzheimer’s disease (AD) patients (Strittmatter et al., 1993), and amyloid plaques are a hallmark of AD, an approximated 60–90% of AD patients also exhibit cerebrovascular pathologies (Bell and Zlokovic, 2009), supporting the vascular theory of AD. In brief, the current understanding is that genetic, environmental, and lifestyle factors may all predispose individuals to damage to the NVU (Figure 1). Soluble amyloid beta (Aβ), which predates Aβ plaques, deregulates cerebrovascular function by activating a free-radical cascade (Park et al., 2008), leading to compromised microvascular integrity (Dorr et al., 2012), and reduced CBF. Aβ is known to interact with endothelin-1 (Kawanabe and Nauli, 2011) and myocardin (Ramanathan et al., 2015) to promote vascular hypercontractility. Moreover, the cholinergic deficit in AD can result in a reduction of cholinergic input to cortical blood vessels (Claassen and Zhang, 2011). Furthermore, Aβ-mediated pericyte degeneration leads to BBB breakdown, increasing the perivascular accumulation of neurotoxins. The various pathways of vascular dysfunction can lead to increasing vascular tortuosity and decreasing vascular reactivity (Black et al., 2009), compromising Aβ clearance and eventually lead to neuronal death. Paradoxically, tau pathology has been associated with an increase in regional vascular reactivity (Wells et al., 2015), a controversy that is still under investigation.
FIGURE 1. Vascular involvement in AD, as depicted in a “2-hit model” of neurovascular dysfunction. Genetic risk (including due to APOE 𝜀4) predispose individuals to amyloid deposition. Modified from Kisler et al. (2017) with permission.
Irrespective of the specific disease mechanism, vascular deficits have been demonstrated as a promising early indicator of AD. Non-invasive functional magnetic-resonance imaging (MRI) has provided strong evidence that CBF can be used to distinguish between at-risk individuals, patients and normal controls (Johnson et al., 2005). In addition, perfusion deficits have been associated with decreased functional connectivity despite maintained glucose metabolism (Göttler et al., 2018). Interventions to re-establish perfusion have been advocated as a promising preventative treatment (de la Torre, 2016). However, perfusion is a mixture of neuronal and vascular contributions, and unraveling the vascular mechanisms of AD etiology requires a more vascularly specific and routinely adoptable vascular marker. In this respect, there is early evidence that deficits in cerebrovascular reactivity (CVR) are detectable before those in CBF (Yezhuvath et al., 2012). Indeed, this was demonstrated through quantitative cerebrovascular resistance, defined as the ratio of mean-arterial blood pressure to CBF (Yew et al., 2017). Compared to CBF, resistance was found to be more sensitive at distinguishing amyloid-positive from amyloid-negative older populations as well as being more predictive of dementia conversion.
A recent review by Glodzik et al. (2013) provides an excellent overview of CVR measurement in AD using carbon-dioxide (CO2) challenges with various imaging modalities. CVR is a vasodilatory or constrictive reaction of a blood vessel to a stimulus. CVR is a well-established indicator of vascular reserve and autoregulatory efficiency. CVR decline has been associated with normal aging (Lu et al., 2011), and is the most reliable neuroimaging predictor of impending cerebrovascular disease (Pillai and Mikulis, 2015).
Qualitative CVR information can be gleaned from the functional MRI (fMRI) response to any task (Dumas et al., 2012), but when quantitative CVR values are desired, vascular agents are generally required. Strong CBF responses can be induced by intravascular CO2 alterations, with CO2 inspiration thought as the optimal form of stimulus (Fierstra et al., 2013). While breathing and blood flow can both be regulated through the midbrain CO2 chemoreceptors, CO2-related blood pH changes are also actively regulated as part of maintaining homeostasis. Thus, hypercapnic challenges, in which the arterial CO2 content is increased, activate VMSC potassium channels (Ainslie and Duffin, 2009), leading to large CBF increases without a significant concomitant increase in metabolic rate (Chen and Pike, 2010; Jain et al., 2011). In addition, nitric oxide, which is synthesized locally following glutamate receptor activity, has also been implicated in the modulation of vasodilatory effects produced by CO2 (Iadecola et al., 1994).
End-tidal CO2 pressure (PETCO2) is an easily measured surrogate for arterial CO2 (PaCO2) (Battisti-Charbonney et al., 2011). PETCO2 is measured as the peak expired CO2, typically 35–40 mmHg in healthy individuals, and directly reflects alveolar CO2. CBF increases by 3–4% per mmHg increase in PETCO2, reaching its highest level when PETCO2 is elevated by 10–20 mmHg above normal resting value (Brugniaux et al., 2007). PETCO2 reductions result in CBF decline by approximately 3% per 1 mmHg change (Ito et al., 2005).
The clinical utility of CO2-based CVR quantification was established using transcranial Doppler ultrasound (TCD) (Ainslie and Duffin, 2009), positron-emission tomography (Ito et al., 2001) and dynamic X-ray computed tomography (Chen et al., 2006). While fMRI is not the most established method of assessing CVR, it offers marked advantages including richer spatial information and minimal invasiveness (Iannetti and Wise, 2007). CVR has been reliably assessed using CO2 fMRI in both gray and white matter (Thomas et al., 2014). In the absence of a CO2 delivery apparatus, breathing challenges such as breath-holding (Bright and Murphy, 2013; Pinto et al., 2016) and cued deep breathing (Bright et al., 2009) have been proposed as alternative ways to modulate intravascular CO2 (see Table 1). A comparison of breath-holding and inhaled-CO2 approaches reveals important CVR dependence on methodology (Tancredi and Hoge, 2013), but the reproducibility of both approaches has been established in healthy young controls (Kassner et al., 2010; Bright and Murphy, 2013).
CO2-based CVR measured using fMRI has been widely applied and extensively cross-validated (Herzig et al., 2008). Robust hypercapnia can be induced through manually adjusted administration of blended gases (Cohen et al., 2004), end-tidal forcing (Poulin et al., 1996) or more recently, computerized PETCO2 targeting (Slessarev et al., 2007; Mark et al., 2010). The latter method entails the most lengthy set up but also provides immediate and robust PETCO2 suppression (hypocapnia) (Blockley et al., 2011), and has been proposed as part of a rapid CVR-mapping protocol for routine use (Blockley et al., 2011, 2017).
In the clinical realm, the main considerations in choosing a CVR-mapping methodology are: (1) How to assess CVR in the most non-invasive manner? (2) How to interpret the CVR information?
As one of the earliest ways to induce PETCO2 elevation (Ratnatunga and Adiseshiah, 1990), breath-holding typically does not allow the calculation of quantitative CVR, as all participants are assumed to perform breath-holds in similar manners and the actual PETCO2 cannot be monitored during the challenge. The lack of PETCO2 monitoring is particularly concerning, as the actual change in PETCO2 achieved by a breath-hold depends on multiple factors, including the resting metabolic rate of the subject, lung size, recent ventilation history and whether the breath-hold is post-inspiration or post-expiration. Moreover, as typical breath-holds last 15–20 s, there are reports of poor subject compliance (Jahanian et al., 2017), particularly when elderly participants are involved. Despite these drawbacks, breath-holding-based CVR mapping has a key advantage of requiring the least instrumentation, thus allowing it to be implemented in almost any MRI scan session. Ongoing research aims to improve the robustness of breath-hold CVR mapping (Bright and Murphy, 2013), although clinical validation remains far from extensive.
Even less invasive than breath-holding, resting-state fMRI has offered a unique window to glean CVR information. Notably, Kannurpatti et al. (2014) reported a comparison of the resting-state fMRI fluctuation amplitude (voxel-wise temporal standard-deviation) as a CVR surrogate. This type of “unconstrained” or “task-free” CVR protocol does not require cooperation from participants, and is thus a promising direction of research that will likely attract tremendous attention from clinical studies. This topic will be further discussed as part of a proposed future trend.
Currently, the de-facto standard protocol to quantitative CVR mapping with MRI remains CO2 inhalation, notably controlled using computerized targeting (Kassner et al., 2010; Fierstra et al., 2013; Sobczyk et al., 2014, 2015; Poublanc et al., 2015; Sam et al., 2016; Fisher et al., 2017). Despite the complex set up, this approach has been extensively used and validated clinically. The use of modern breathing circuits also allows the CO2 challenge to follow nearly any shape. However, there has yet to be a consensus as to the level, duration and pattern of PETCO2 perturbation. As different stimulus designs likely have different vaso-stimulating capacities and hence may reveal different CVR patterns, the choice of challenge will be critical, not only in comparing across studies but also across the same individuals over time (Fierstra et al., 2013).
Based on prospective targeting of stepwise PETCO2 changes, researchers at Toronto Western Hospital (TWH) pioneered the use of an uneven task design – one short block followed by longer block (Spano et al., 2013), both typically elevating PETCO2 by 10 mmHg. This design is motivated by the desire to derive more accurate estimates of CVR response time, and (Duffin et al., 2015; Poublanc et al., 2015), which may reflect regional arterial-transit time. Additionally, the same group proposed the use of progressive hypercapnia (CO2 ramps) (Fisher et al., 2017), in which both hypercapnia and hypocapnia are progressively induced through a ramp stimulus. It has been demonstrated that different segments of the ramp, which resulted in PETCO2 values of 30–50 mmHg, reveal different spatial patterns in CVR that could complement the conventional CVR information (Fisher et al., 2017). Alternatively, the use of a sinusoidal pattern allows direct estimation of response delay (as the phase in the corresponding sinusoidal CVR response), and has allowed the development of a CVR protocol as short as 5 min (Blockley et al., 2017). Such a design makes use of both hypercapnia and hypocapnia for CVR estimation, rendering estimates more robust against biases due to basal vascular tone (Halani et al., 2015). Further reducing scan time is a 1-min blended-gas protocol with 5% CO2 (Yezhuvath et al., 2009; Blockley et al., 2017), which has compared favorably against longer designs. These stimulation designs are summarized in Figure 2, and research is ongoing to validate the unique utility of each design, and it is likely that CVR measurements produced by these various methods are not directly comparable.
Concurrently, the emergence of arterial-spin labeling (ASL) MRI for CBF-based CVR mapping has added a new dimension to the choice of methods. In particular, CBF-based maps, while lower in signal-to-noise ratios (SNRs), can in fact provide more vascular-driven and thus less biased CVR quantification than BOLD fMRI (Halani et al., 2015), as demonstrated by comparisons with TCD (Gao et al., 2013). Great strides have been made in extending the use of ASL-based CVR mapping into aging research (Leoni et al., 2017), and ASL is now ubiquitously used in the study of AD (Alsop et al., 2014).
Cerebrovascular reactivity compromises in the middle-cerebral artery in AD, mainly measured using blended-CO2 method, is a well-established TCD-based finding (Lee et al., 2007; Sabayan et al., 2012; Viticchi et al., 2012; Hajjar et al., 2015). While the use of MRI-based CVR mapping in AD is still limited, its adoption is on the cusp of expansion due to rapid methodological developments.
Using MRI, such CVR reductions have been localized to the prefrontal, anterior cingulate and insular regions (Yezhuvath et al., 2012). Interestingly, while this pattern overlapped little with that of CBF deficits (found in the temporal and parietal regions), it agreed with the localization of amyloid deposition (Yezhuvath et al., 2012), suggesting that CVR has unique sensitivity to AD pathology (Figure 3A). Moreover, cortical and white-matter CVR deficits have been linked to the incidence of leukoaraiosis (Yezhuvath et al., 2012; Sam et al., 2016). Such reductions in CVR echo postmortem observations of vascular dysfunction (Chow et al., 2007), and can be the result of a number of structural changes in the vasculature, including cerebral amyloid angiopathy (CAA), astrocytic end-feet swelling, pericyte degeneration, basement-membrane hypertrophy and endothelial-cell metabolic abnormalities (Hashimura et al., 1991; Miyakawa et al., 1997).
FIGURE 3. MRI-based CVR maps in AD and APOE gene carriers. (A) Frontal, cingular, and insular CVR deficits are found in AD patients. Figure is modified from Yezhuvath et al. (2012) with permission. (B) Young 𝜀4 carriers manifest widespread CVR deficits compared to 𝜀3 homozygotes. Figure is modified from Suri et al. (2014) with permission.
Cerebrovascular reactivity deficits have been discovered amongst young APOE 𝜀4 gene carriers (Hajjar et al., 2015), even when compared to 𝜀3 homozygotes (Suri et al., 2014) (Figure 3B). Such deficits are found to be widespread, notably in the prefrontal and parahippocampal regions, bolstering the hypothesis that genetic predisposition to vascular disease contributes to the vulnerability of 𝜀4-carriers to late-life pathology (Kisler et al., 2017).
It is increasingly recognized that vascular deficits may be the most accessible physiological treatment target in the effort to delay dementia onset, and approaches that enhance perfusion have demonstrated potential therapeutic value (de la Torre, 2016). Predicting progression of preclinical AD amongst mild-cognitive impaired (MCI) individuals has been a key research focus. Using breath-hold TCD, the predictive value of CVR (Sato and Morishita, 2013) in terms of MCI-to-AD conversions has been demonstrated (Buratti et al., 2015).
In light of the overwhelming influence of vascular risk factors in AD progression, the lines between vascular deficits in AD and other types of dementia can become blurred in later stages of the disease, as will be discussed in later sections. As a case in point, given the rampant occurrence of CAA amongst suspected AD patients, the vascular dysfunction can produce deleterious oxidative stress that can promote ischemia and accelerate AD progression (Girouard and Iadecola, 2006; Bookheimer and Burggren, 2009). Furthermore, CVR may be a more sensitive early marker of AD severity (Yezhuvath et al., 2012). It is conceivable that a diseased vasculature may sustain normal perfusion but reveal an abnormal response to a stress test such as used in CVR mapping (Fierstra et al., 2013). Nonetheless, as an increasing amount of CVR data is generated using BOLD fMRI, it is also important to note that microvascular CVR is more reflective of AD severity (Jellinger and Attems, 2006), while the BOLD fMRI signal is generally dominated by large-vessels. This is true at clinical field strengths (1.5 or 3 Tesla) and using either gradient- or spin-echo BOLD. ASL, on the other hand, is likely more sensitive to capillaries and arterioles, and should be the most natural alternative for CVR mapping.
There are numerous fMRI studies that report age-related differences in the BOLD response amplitude or extent, but as the BOLD response to neuronal stimuli is intrinsically modulated by CVR, one must be cautioned against interpreting age-related BOLD differences as neuronal differences. This is also true of resting-state fMRI, where functional connectivity has been found to vary with CVR (Golestani et al., 2016a; Lajoie et al., 2017; Chu et al., 2018).
As stated earlier, the most commonly reported challenge in acquiring CVR maps in clinical research pertains to the need for subject cooperation. This is true for all of the stimulus designs described thus far, imposing a fundamental limitation on the routine use of CVR mapping amongst patients. Very recently, resting-state methods that do not require CO2 perturbation have flourished (Golestani et al., 2016b; Jahanian et al., 2017; Liu et al., 2017). Resting-state CVR methods rely on intrinsic fluctuations in the BOLD fMRI signal, and may significantly broaden the accessibility of CVR mapping to clinical researchers. Additionally, beyond the magnitude of CVR, the dynamic features of the fMRI response can also provide useful information. A slowing of the CVR response has been shown to characterize vascular lesions (Poublanc et al., 2015), adding a dimension to the utility of CVR mapping.
The response of the cerebral circulation to a changing arterial CO2 concentration is not linear – the circulatory response follows a sigmoidal shape, and is greater for hypercapnia than to hypocapnia (Ogoh et al., 2008; Peebles et al., 2008; Rodell et al., 2012). Moreover, it is critical to note that while CVR is traditionally defined as a blood-flow response (as is the case in TCD, PET, and CT), the BOLD signal is not a direct measure of CBF. Rather, BOLD is modulated by CBF, CBV, and baseline oxidative metabolism, not to mention a series of field-dependent physical variables. Thus, the assumption of a linear relationship between the BOLD and CBF responses to CO2 is likely tenuous. Specifically, it is widely known that the BOLD response varies with CBF in a non-linear fashion (Hoge et al., 1999). This non-linearity is superimposed in the inherently sigmoidal vascular response to CO2 (Battisti-Charbonney et al., 2011). Such non-linear CVR changes have been demonstrated through a comparison with CBF-based CVR measurements at various vascular baselines (Halani et al., 2015), and may in a small part underlie the BOLD response behavior in the “vascular steal” phenomenon (Sobczyk et al., 2014). This limitation will require careful consideration in the presence of known vascular dysfunction (Battisti-Charbonney et al., 2011).
A critical assumption for CVR mapping is that PETCO2 represents PaCO2. However, PaCO2 is determined by both inhaled CO2 and the minute ventilation. Low cardiac output can increase alveolar dead space, which would increase the difference between PaCO2 and PETCO2 (Shibutani et al., 1992), leading to underestimations of PETCO2-based CVR. In addition, PETCO2 is shown to overestimate PaCO2 during exercise in young adults, but not in older adults (Williams and Babb, 1997). Moreover, PETCO2-related CVR is known to follow a circadian rhythm, increasing with the level of alertness (Ainslie and Duffin, 2009). These factor contribute to inter-cohort, inter-sessional and inter-subject variability in CVR estimates that must be accounted for when assessing true differences in CVR.
In this regard, an emerging research direction is building normative CVR atlases that allow the significance of CVR deviations to be assessed (Sobczyk et al., 2015). Such atlases would ideally encompass not only quantitative CVR values but also CVR-timing information (van Niftrik et al., 2017). This is a critical step in expanding the clinical utility of CVR maps, and atlases will likely need to be specific to the CO2 delivery method, stimulation design, study objectives and MRI system used.
The above research gaps pertain not only to AD but to other cerebrovascular diseases also. The increasing awareness of the vascular etiology of various forms of dementia will highlight these limitations and prompt more focused validation studies.
The author confirms being the sole contributor of this work and approved it for publication.
This work was supported by funding from the Canadian Institutes of Health Research (CIHR FRN#126164 and 148398) as well as by the Natural Sciences and Engineering Research Council of Canada (NSERC FRN# 418443).
The author declares that the research was conducted in the absence of any commercial or financial relationships that could be construed as a potential conflict of interest.
Ainslie, P. N., and Duffin, J. (2009). Integration of cerebrovascular CO2 reactivity and chemoreflex control of breathing: mechanisms of regulation, measurement, and interpretation. Am. J. Physiol. Regul. Integr. Comp. Physiol. 296, R1473–R1495. doi: 10.1152/ajpregu.91008.2008
Alsop, D. C., Detre, J. A., Golay, X., Gunther, M., Hendrikse, J., Hernandez-Garcia, L., et al. (2014). Recommended implementation of arterial spin-labeled perfusion MRI for clinical applications: a consensus of the ISMRM perfusion study group and the european consortium for ASL in dementia. Magn. Reson. Med. doi: 10.1002/mrm.25197 [Epub ahead of print].
Battisti-Charbonney, A., Fisher, J., and Duffin, J. (2011). The cerebrovascular response to carbon dioxide in humans. J. Physiol. 589, 3039–3048. doi: 10.1113/jphysiol.2011.206052
Bell, R. D., and Zlokovic, B. V. (2009). Neurovascular mechanisms and blood-brain barrier disorder in Alzheimer’s Disease. Acta Neuropathol. 118, 103–113. doi: 10.1007/s00401-009-0522-3
Black, S., Gao, F., and Bilbao, J. (2009). Understanding white matter disease: imaging-pathological correlations in vascular cognitive impairment. Stroke 3(Suppl.), S48–S52. doi: 10.1161/STROKEAHA.108.537704
Blockley, N. P., Driver, I. D., Francis, S. T., Fisher, J. A., and Gowland, P. A. (2011). An improved method for acquiring cerebrovascular reactivity maps. Magn. Reson. Med. 65, 1278–1286. doi: 10.1002/mrm.22719
Blockley, N. P., Harkin, J. W., and Bulte, D. P. (2017). Rapid cerebrovascular reactivity mapping: enabling vascular reactivity information to be routinely acquired. Neuroimage 159, 214–223. doi: 10.1016/j.neuroimage.2017.07.048
Bookheimer, S., and Burggren, A. (2009). APOE-4 genotype and neurophysiological vulnerability to Alzheimer’s and cognitive aging. Annu. Rev. Clin. Psychol. 5, 343–362. doi: 10.1146/annurev.clinpsy.032408.153625
Bright, M. G., Bulte, D. P., Jezzard, P., and Duyn, J. H. (2009). Characterization of regional heterogeneity in cerebrovascular reactivity dynamics using novel hypocapnia task and BOLD fMRI. Neuroimage 48, 166–175. doi: 10.1016/j.neuroimage.2009.05.026
Bright, M. G., and Murphy, K. (2013). Reliable quantification of BOLD fMRI cerebrovascular reactivity despite poor breath-hold performance. Neuroimage 83, 559–568. doi: 10.1016/j.neuroimage.2013.07.007
Brugniaux, J. V., Hodges, A. N., Hanly, P. J., and Poulin, M. J. (2007). Cerebrovascular responses to altitude. Respir. Physiol. Neurobiol. 158, 212–223. doi: 10.1016/j.resp.2007.04.008
Buratti, L., Balestrini, S., Altamura, C., Viticchi, G., Falsetti, L., Luzzi, S., et al. (2015). Markers for the risk of progression from mild cognitive impairment to Alzheimer’s Disease. J. Alzheimer’s Dis. 45, 883–890. doi: 10.3233/JAD-143135
Chen, A., Shyr, M.-H., Chen, T.-Y., Lai, H.-Y., Lin, C.-C., and Yen, P.-S. (2006). Dynamic CT perfusion imaging with acetazolamide challenge for evaluation of patients with unilateral cerebrovascular steno-occlusive disease. AJNR Am. J. Neuroradiol. 27, 1876–1881.
Chen, J. J., and Pike, G. B. (2010). Global cerebral oxidative metabolism during hypercapnia and hypocapnia in humans: implications for BOLD fMRI. J. Cereb. Blood Flow Metab. 30, 1094–1099. doi: 10.1038/jcbfm.2010.42
Chow, N., Bell, R. D., Deane, R., Streb, J. W., Chen, J., and Brooks, A. (2007). Serum response factor and myocardin mediate arterial hypercontractility and cerebral blood flow dysregulation in Alzheimer’s Phenotype. Proc. Natl. Acad. Sci. U.S.A. 104, 823–828. doi: 10.1073/pnas.0608251104
Chu, P. P. W., Golestani, A. M., Kwinta, J. B., Khatamian, Y. B., and Chen, J. J. (2018). Characterizing the modulation of resting-state fMRI Metrics by baseline physiology. Neuroimage 173, 72–87. doi: 10.1016/j.neuroimage.2018.02.004
Claassen, J. A., and Zhang, R. (2011). Cerebral Autoregulation in Alzheimer’s Disease. J. Cereb. Blood Flow Metab. 31, 1572–1577. doi: 10.1038/jcbfm.2011.69
Cohen, E. R., Rostrup, E., Sidaros, K., Lund, T. E., Paulson, O. B., Ugurbil, K., et al. (2004). Hypercapnic normalization of BOLD fMRI: comparison across field strengths and pulse sequences. Neuroimage 23, 613–624. doi: 10.1016/j.neuroimage.2004.06.021
de la Torre, J. C. (2016). Cerebral perfusion enhancing interventions: a new strategy for the prevention of Alzheimer dementia. Brain Pathol. 26, 618–631. doi: 10.1111/bpa.12405
Dorr, A., Sahota, B., Chinta, L. V., Brown, M. E., Lai, A. Y., and Ma, K. (2012). Amyloid-Beta-dependent compromise of microvascular structure and function in a model of Alzheimer’s Disease. Brain 135, 3039–3050. doi: 10.1093/brain/aws243
Duffin, J., Sobczyk, O., Crawley, A. P., Poublanc, J., Mikulis, D. J., and Fisher, J. A. (2015). The dynamics of cerebrovascular reactivity shown with transfer function analysis. Neuroimage 114, 207–216. doi: 10.1016/j.neuroimage.2015.04.029
Duling, B. R., and Berne, R. M. (1970). Longitudinal gradients in periarteriolar oxygen tension: a possible mechanism for the participation of oxygen in local regulation of blood flow. Circ. Res. 27, 669–678. doi: 10.1161/01.RES.27.5.669
Dumas, A., Dierksen, G. A., Gurol, M. E., Halpin, A., Martinez-Ramirez, S., Schwab, K., et al. (2012). Functional magnetic resonance imaging detection of vascular reactivity in cerebral amyloid angiopathy. Ann. Neurol. 72, 76–81. doi: 10.1002/ana.23566
Fierstra, J., Sobczyk, O., Battisti-Charbonney, A., Mandell, D. M., Poublanc, J., Crawley, A. P., et al. (2013). Measuring cerebrovascular reactivity: what stimulus to use? J. Physiol. 591, 5809–5821. doi: 10.1113/jphysiol.2013.259150
Fisher, J. A., Sobczyk, O., Crawley, A., Poublanc, J., Dufort, P., Venkatraghavan, L., et al. (2017). Assessing cerebrovascular reactivity by the pattern of response to progressive hypercapnia. Hum. Brain Mapp. doi: 10.1002/hbm.23598 [Epub ahead of print].
Gao, Y.-Z., Zhang, J.-J., Liu, H., Wu, G.-Y., Xiong, L., and Shu, M. (2013). Regional cerebral blood flow and cerebrovascular reactivity in Alzheimer’s disease and vascular dementia assessed by arterial spinlabeling magnetic resonance imaging. Curr. Neurovasc. Res. 10, 49–53. doi: 10.2174/156720213804806016
Girouard, H., and Iadecola, C. (2006). Neurovascular coupling in the normal brain and in hypertension, stroke, and Alzheimer Disease. J. Appl. Physiol. 100, 328–335. doi: 10.1152/japplphysiol.00966.2005
Glodzik, L., Randall, C., Rusinek, H., and de Leon, M. J. (2013). Cerebrovascular reactivity to carbon dioxide in Alzheimer’s Disease. J. Alzheimer’s Dis. JAD 35, 427–440.
Golestani, A. M., Kwinta, J. B., Strother, S. C., Khatamian, Y. B., and Chen, J. J. (2016a). The association between cerebrovascular reactivity and resting-state fMRI functional connectivity in healthy adults: the influence of basal carbon dioxide. Neuroimage 132, 301–313. doi: 10.1016/j.neuroimage.2016.02.051
Golestani, A. M., Wei, L. L., and Chen, J. J. (2016b). Quantitative mapping of cerebrovascular reactivity using resting-state BOLD fMRI: validation in healthy adults. Neuroimage 138, 147–163. doi: 10.1016/j.neuroimage.2016.05.025
Göttler, J., Preibisch, C., Riederer, I., Pasquini, L., Alexopoulos, P., Bohn, K. P., et al. (2018). Reduced blood oxygenation level dependent connectivity is related to hypoperfusion in Alzheimer’s Disease. J. Cereb. Blood Flow Metab. doi: 10.1177/0271678X18759182 [Epub ahead of print].
Hajjar, I., Sorond, F., and Lipsitz, L. A. (2015). Apolipoprotein E, carbon dioxide vasoreactivity, and cognition in older adults: effect of hypertension. J. Am. Geriatr. Soc. 63, 276–281. doi: 10.1111/jgs.13235
Halani, S., Kwinta, J. B., Golestani, A. M., Khatamian, Y. B., and Chen, J. J. (2015). Comparing cerebrovascular reactivity measured using BOLD and cerebral blood flow MRI: the effect of basal vascular tension on vasodilatory and vasoconstrictive reactivity. Neuroimage 110, 110–123. doi: 10.1016/j.neuroimage.2015.01.050
Hashimura, T., Kimura, T., and Miyakawa, T. (1991). Morphological changes of blood vessels in the brain with Alzheimer’s Disease. Jpn. J. Psychiatry Neurol. 45, 661–665. doi: 10.1111/j.1440-1819.1991.tb01187.x
Herzig, R., Hlustík, P., Skoloudík, D., Sanák, D., Vlachová, I., Herman, M., et al. (2008). Assessment of the cerebral vasomotor reactivity in internal carotid artery occlusion using a transcranial doppler sonography and functional MRI. J. Neuroimaging 18, 38–45. doi: 10.1111/j.1552-6569.2007.00168.x
Hoge, R. D., Atkinson, J., Gill, B., Crelier, G. R., Marrett, S., and Pike, G. B. (1999). Investigation of BOLD signal dependence on CBF and CMRO: the deoxyhemoglobin dilution model. Magn. Reson. Med. 42, 849–863. doi: 10.1002/(SICI)1522-2594(199911)42:5<849::AID-MRM4>3.0.CO;2-Z
Iadecola, C., Pelligrino, D. A., Moskowitz, M. A., and Lassen, N. A. (1994). Nitric oxide synthase inhibition and cerebrovascular regulation. J. Cereb. Blood Flow Metab. 14, 175–192. doi: 10.1038/jcbfm.1994.25
Iannetti, G. D., and Wise, R. G. (2007). BOLD functional MRI in disease and pharmacological studies: room for improvement? Magn. Reson. Imaging 25, 978–988. doi: 10.1016/j.mri.2007.03.018
Ito, H., Kanno, I., and Fukuda, H. (2005). Human cerebral circulation: positron emission tomography studies. Ann. Nucl. Med. 19, 65–74. doi: 10.1007/BF03027383
Ito, H., Takahashi, K., Hatazawa, J., Kim, S.-G., and Kanno, I. (2001). Changes in human regional cerebral blood flow and cerebral blood volume during visual stimulation measured by positron emission tomography. J. Cereb. Blood Flow Metab. 21, 608–612. doi: 10.1097/00004647-200105000-00015
Jahanian, H., Christen, T., Moseley, M. E., Pajewski, N. M., Wright, C. B., Tamura, M. K., et al. (2017). Measuring vascular reactivity with resting-state blood oxygenation level-dependent (BOLD) signal fluctuations: a potential alternative to the breath-holding challenge? J. Cereb. Blood Flow Metab. 37, 2526–2538. doi: 10.1177/0271678X16670921
Jain, V., Langham, M. C., Floyd, T. F., Jain, G., Magland, J. F., and Wehrli, F. W. (2011). Rapid magnetic resonance measurement of global cerebral metabolic rate of oxygen consumption in humans during rest and hypercapnia. J. Cereb. Blood Flow Metab. 31, 1504–1512. doi: 10.1038/jcbfm.2011.34
Jellinger, K. A., and Attems, J. (2006). Prevalence and impact of cerebrovascular pathology in Alzheimer’s Disease and Parkinsonism. Acta Neurol. Scand. 114, 38–46. doi: 10.1111/j.1600-0404.2006.00665.x
Johnson, N. A., Jahng, G. H., Weiner, M. W., Miller, B. L., Chui, H. C., Jagust, W. J., et al. (2005). Pattern of cerebral hypoperfusion in Alzheimer Disease and mild cognitive impairment measured with arterial spin-labeling MR imaging: initial experience. Radiology 234, 851–859. doi: 10.1148/radiol.2343040197
Kannurpatti, S. S., Motes, M. A., Biswal, B. B., and Rypma, B. (2014). Assessment of unconstrained cerebrovascular reactivity marker for large age-range FMRI studies. PLoS One 9:e88751. doi: 10.1371/journal.pone.0088751
Kassner, A., Winter, J. D., Poublanc, J., Mikulis, D. J., and Crawley, A. P. (2010). Blood-Oxygen level dependent MRI measures of cerebrovascular reactivity using a controlled respiratory challenge: reproducibility and gender differences. J. Magn. Reson. Imaging 31, 298–304. doi: 10.1002/jmri.22044
Kawanabe, Y., and Nauli, S. M. (2011). Endothelin. Cell Mol. Life Sci. 68, 195–203. doi: 10.1007/s00018-010-0518-0
Kisler, K., Nelson, A. R., Montagne, A., and Zlokovic, B. V. (2017). Cerebral blood flow regulation and neurovascular dysfunction in Alzheimer Disease. Nat. Rev. Neurosci. 18, 419–434. doi: 10.1038/nrn.2017.48
Lajoie, I., Nugent, S., Debacker, C., Dyson, K., Tancredi, F. B., Badhwar, A., et al. (2017). Application of calibrated fMRI in Alzheimer’s Disease. Neuroimage Clin. 15, 348–358. doi: 10.1016/j.nicl.2017.05.009
Lee, S.-T., Jung, K.-H., and Lee, Y.-S. (2007). Decreased vasomotor reactivity in Alzheimer’s Disease. J. Clin. Neurol. 3, 18–23. doi: 10.3988/jcn.2007.3.1.18
Leoni, R. F., Oliveira, I. A., Pontes-Neto, O. M., Santos, A. C., and Leite, J. P. (2017). Cerebral blood flow and vasoreactivity in aging: an arterial spin labeling study. Braz. J. Med. Biol. Res. 50:e5670. doi: 10.1590/1414-431X20175670
Liu, P., Li, Y., Pinho, M., Park, D. C., Welch, B. G., and Lu, H. (2017). Cerebrovascular reactivity mapping without gas challenges. Neuroimage 146, 320–326. doi: 10.1016/j.neuroimage.2016.11.054
Lu, H., Xu, F., Rodrigue, K. M., Kennedy, K. M., Cheng, Y., Flicker, B., et al. (2011). Alterations in cerebral metabolic rate and blood supply across the adult lifespan. Cereb. Cortex 21, 1426–1434. doi: 10.1093/cercor/bhq224
Mark, C. I., Slessarev, M., Ito, S., Han, J., Fisher, J. A., and Pike, G. B. (2010). Precise control of end-tidal carbon dioxide and oxygen improves BOLD and ASL cerebrovascular reactivity measures. Magn. Reson. Med. 64, 749–756. doi: 10.1002/mrm.22405
Miyakawa, T., Katsuragi, S., Higuchi, Y., Yamashita, K., Kimura, T., Teraoka, K., et al. (1997). Changes of microvessels in the brain with Alzheimer’s Disease. Ann. N. Y. Acad. Sci. 826, 428–432. doi: 10.1111/j.1749-6632.1997.tb48497.x
Ogoh, S., Hayashi, N., Inagaki, M., Ainslie, P. N., and Miyamoto, T. (2008). Interaction between the ventilatory and cerebrovascular responses to hypo- and hypercapnia at rest and during exercise. J. Physiol. 586, 4327–4338. doi: 10.1113/jphysiol.2008.157073
Park, L., Zhou, P., Pitstick, R., Capone, C., Anrather, J., Norris, E. H., et al. (2008). Nox2-Derived radicals contribute to neurovascular and behavioral dysfunction in mice overexpressing the amyloid precursor protein. Proc. Natl. Acad. Sci. U.S.A. 105, 1347–1352. doi: 10.1073/pnas.0711568105
Peebles, K. C., Mark Richards, A., Celi, L., McGrattan, K., Murrell, C. J., and Ainslie, P. N. (2008). Human cerebral arteriovenous vasoactive exchange during alterations in arterial blood gases. J. Appl. Physiol. 105, 1060–1068. doi: 10.1152/japplphysiol.90613.2008
Pillai, J. J., and Mikulis, D. J. (2015). Cerebrovascular reactivity mapping: an evolving standard for clinical functional imaging. AJNR Am. J. Neuroradiol. 36, 7–13. doi: 10.3174/ajnr.A3941
Pinto, J., Jorge, J., Sousa, I., Vilela, P., and Figueiredo, P. (2016). Fourier modeling of the BOLD response to a breath-hold task: optimization and reproducibility. Neuroimage 135, 223–231. doi: 10.1016/j.neuroimage.2016.02.037
Poublanc, J., Crawley, A. P., Sobczyk, O., Montandon, G., Sam, K., Mandell, D. M., et al. (2015). Measuring cerebrovascular reactivity: the dynamic response to a step hypercapnic stimulus. J. Cereb. Blood Flow Metab. 35, 1746–1756. doi: 10.1038/jcbfm.2015.114
Poulin, M. J., Liang, P. J., and Robbins, P. A. (1996). Dynamics of the cerebral blood flow response to step changes in end-tidal PCO2 and PO2 in humans. J. Appl. Physiol. 81, 1084–1095. doi: 10.1152/jappl.1996.81.3.1084
Ramanathan, A., Nelson, A. R., Sagare, A. P., and Zlokovic, B. V. (2015). Impaired vascular-mediated clearance of brain amyloid beta in Alzheimer’s disease: the role, regulation and restoration of LRP1. Front. Aging Neurosci. 7:136. doi: 10.3389/fnagi.2015.00136
Ratnatunga, C., and Adiseshiah, M. (1990). Increase in middle cerebral artery velocity on breath holding: a simplified test of cerebral perfusion reserve. Eur. J. Vasc. Surg. 4, 519–523. doi: 10.1016/S0950-821X(05)80795-9
Rodell, A. B., Aanerud, J., Braendgaard, H., and Gjedde, A. (2012). Low residual CBF variability in Alzheimer’s disease after correction for CO2 effect. Front. Neuroenerg. 4:8. doi: 10.3389/fnene.2012.00008
Roy, C. S., and Sherrington, C. S. (1890). On the regulation of the blood supply of the brain. J. Physiol. 11, 85–105. doi: 10.1113/jphysiol.1890.sp000321
Sabayan, B., Jansen, S., Oleksik, A. M., van Osch, M. J. P., van Buchem, M. A., van Vliet, P., et al. (2012). Cerebrovascular hemodynamics in Alzheimer’s Disease and vascular dementia: a meta-analysis of transcranial doppler studies. Ageing Res. Rev. 11, 271–277. doi: 10.1016/j.arr.2011.12.009
Sam, K., Crawley, A. P., Poublanc, J., Conklin, J., Sobczyk, O., Mandell, D. M., et al. (2016). Vascular dysfunction in leukoaraiosis. AJNR Am. J. Neuroradiol. 37, 2258–2264. doi: 10.3174/ajnr.A4888
Sato, N., and Morishita, R. (2013). Roles of vascular and metabolic components in cognitive dysfunction of Alzheimer disease: short- and long-term modification by non-genetic risk factors. Front. Aging Neurosci. 5:64. doi: 10.3389/fnagi.2013.00064
Shibutani, K., Shirasaki, S., Kubal, K., and Gupte, P. (1992). Changes in cardiac output affect PETCO2 AND CO2 transport during unsteady state in man. Anesthesiology 77(Suppl.):A468. doi: 10.1097/00000542-199209001-00468
Slessarev, M., Han, J., Mardimae, A., Prisman, E., Preiss, D., Volgyesi, G., et al. (2007). Prospective targeting and control of end-tidal CO2 and O2 concentrations. J. Physiol. 581(Pt 3), 1207–1219.
Sobczyk, O., Battisti-Charbonney, A., Fierstra, J., Mandell, D. M., Poublanc, J., Crawley, A. P., et al. (2014). A conceptual model for CO2-Induced redistribution of cerebral blood flow with experimental confirmation using BOLD MRI. Neuroimage 92, 56–68. doi: 10.1016/j.neuroimage.2014.01.051
Sobczyk, O., Battisti-Charbonney, A., Poublanc, J., Crawley, A. P., Sam, K., Fierstra, J., et al. (2015). Assessing cerebrovascular reactivity abnormality by comparison to a reference atlas. J. Cereb. Blood Flow Metab. 35, 213–220. doi: 10.1038/jcbfm.2014.184
Spano, V. R., Mandell, D. M., Poublanc, J., Sam, K., Battisti-Charbonney, A., Pucci, O., et al. (2013). CO2 blood oxygen level-dependent MR mapping of cerebrovascular reserve in a clinical population: safety, tolerability, and technical feasibility. Radiology 266, 592–598. doi: 10.1148/radiol.12112795
Strittmatter, W. J., Saunders, A. M., Schmechel, D., Pericak-Vance, M., and Enghild Salvesen, G. S. (1993). Apolipoprotein E: high-avidity binding to beta-amyloid and increased frequency of type 4 allele in late-onset familial Alzheimer Disease. Proc. Natl. Acad. Sci. U.S.A. 90, 1977–1981. doi: 10.1073/pnas.90.5.1977
Suri, S., Mackay, C. E., Kelly, M. E., Germuska, M., Tunbridge, E. M., Frisoni, G. B., et al. (2014). Reduced cerebrovascular reactivity in young adults carrying the APOE epsilon4 Allele. Alzheimer’s Dement. 11, 648.e1–657.e1. doi: 10.1016/j.jalz.2014.05.1755
Tancredi, F. B., and Hoge, R. D. (2013). Comparison of cerebral vascular reactivity measures obtained using breath-holding and CO2 inhalation. J. Cereb. Blood Flow Metab. 33, 1066–1074. doi: 10.1038/jcbfm.2013.48
Thomas, B. P., Liu, P., Park, D. C., van Osch, M. J., and Lu, H. (2014). Cerebrovascular reactivity in the brain white matter: magnitude, temporal characteristics, and age effects. J. Cereb. Blood Flow Metab. 34, 242–247. doi: 10.1038/jcbfm.2013.194
van Niftrik, C. H. B., Piccirelli, M., Bozinov, O., Pangalu, A., Fisher, J. A., Valavanis, A., et al. (2017). Iterative analysis of cerebrovascular reactivity dynamic response by temporal decomposition. Brain Behav. 7:e00705. doi: 10.1002/brb3.705
Viticchi, G., Falsetti, L., Vernieri, F., Altamura, C., Bartolini, M., Luzzi, S., et al. (2012). Vascular predictors of cognitive decline in patients with mild cognitive impairment. Neurobiol. Aging 33, 1127.e1–1127.e9. doi: 10.1016/j.neurobiolaging.2011.11.027
Wells, J. A., Holmes, H. E., O’Callaghan, J. M., Colgan, N., Ismail, O., Fisher, E. M., et al. (2015). Increased cerebral vascular reactivity in the tau expressing rTg4510 mouse: evidence against the role of tau pathology to impair vascular health in Alzheimer’s Disease. J. Cereb. Blood Flow Metab. 35, 359–362. doi: 10.1038/jcbfm.2014.224
Williams, J. S., and Babb, T. G. (1997). Differences between estimates and measured PaCO2 during rest and exercise in older subjects. J. Appl. Physiol. 83, 312–316. doi: 10.1152/jappl.1997.83.1.312
Yew, B., Nation, D. A., and Alzheimer’s Disease Neuroimaging Initiative (2017). Cerebrovascular resistance: effects on cognitive decline, cortical atrophy, and progression to dementia. Brain 140, 1987–2001. doi: 10.1093/brain/awx112
Yezhuvath, U. S., Lewis-Amezcua, K., Varghese, R., Xiao, G., and Lu, H. (2009). On the assessment of cerebrovascular reactivity using hypercapnia BOLD MRI. NMR Biomed. 22, 779–786. doi: 10.1002/nbm.1392
Keywords: cerebrovascular reactivity (CVR), Alzheimer’s disease, APOE, magnetic resonance imaging (MRI), functional MRI (fMRI), carbon dioxide (CO2), resting-state fMRI, mild-cognitive impairment
Citation: Chen JJ (2018) Cerebrovascular-Reactivity Mapping Using MRI: Considerations for Alzheimer’s Disease. Front. Aging Neurosci. 10:170. doi: 10.3389/fnagi.2018.00170
Received: 14 March 2018; Accepted: 18 May 2018;
Published: 05 June 2018.
Edited by:
Ai-Ling Lin, University of Kentucky, United StatesReviewed by:
Katherine Bangen, University of California, San Diego, United StatesCopyright © 2018 Chen. This is an open-access article distributed under the terms of the Creative Commons Attribution License (CC BY). The use, distribution or reproduction in other forums is permitted, provided the original author(s) and the copyright owner are credited and that the original publication in this journal is cited, in accordance with accepted academic practice. No use, distribution or reproduction is permitted which does not comply with these terms.
*Correspondence: J. J. Chen, amNoZW5AcmVzZWFyY2guYmF5Y3Jlc3Qub3Jn
Disclaimer: All claims expressed in this article are solely those of the authors and do not necessarily represent those of their affiliated organizations, or those of the publisher, the editors and the reviewers. Any product that may be evaluated in this article or claim that may be made by its manufacturer is not guaranteed or endorsed by the publisher.
Research integrity at Frontiers
Learn more about the work of our research integrity team to safeguard the quality of each article we publish.