- Instituto de Tecnologia Química e Biológica António Xavier, Universidade Nova de Lisboa, Oeiras, Portugal
Small non-coding RNAs (sRNAs) are critical post-transcriptional regulators of gene expression. Distinct RNA-binding proteins (RBPs) influence the processing, stability and activity of bacterial small RNAs. The vast majority of bacterial sRNAs interact with mRNA targets, affecting mRNA stability and/or its translation rate. The assistance of RNA-binding proteins facilitates and brings accuracy to sRNA-mRNA basepairing and the RNA chaperones Hfq and ProQ are now recognized as the most prominent RNA matchmakers in bacteria. These RBPs exhibit distinct high affinity RNA-binding surfaces, promoting RNA strand interaction between a trans-encoding sRNA and its mRNA target. Nevertheless, some organisms lack ProQ and/or Hfq homologs, suggesting the existence of other RBPs involved in sRNA function. Along this line of thought, the global regulator CsrA was recently shown to facilitate the access of an sRNA to its target mRNA and may represent an additional factor involved in sRNA function. Ribonucleases (RNases) can be considered a class of RNA-binding proteins with nucleolytic activity that are responsible for RNA maturation and/or degradation. Presently RNase E, RNase III, and PNPase appear to be the main players not only in sRNA turnover but also in sRNA processing. Here we review the current knowledge on the most important bacterial RNA-binding proteins affecting sRNA activity and sRNA-mediated networks.
Introduction
The majority of small non-coding RNAs (sRNAs) interact with a complementary mRNA through an antisense mechanism, leading to the formation of a duplex sRNA-mRNA region. Consequently, expression from the target mRNA is affected and frequently repressed (Storz et al., 2011). sRNA-mediated networks are cost efficient and often more rapid in the reprogramming of gene expression than pathways that rely exclusively on regulatory proteins (Shimoni et al., 2007). However, the interaction between sRNAs and RNA-binding proteins (RBPs) is often critical for the regulatory activity of sRNAs. RNA-binding proteins are a diverse class of proteins ubiquitously found in all living organisms and that control all steps of the life of an RNA (Smirnov et al., 2017a). The capacity of these proteins to recognize and bind RNA molecules arises from the presence of well-defined RNA-binding domains, such as the canonical S1 domain, cold shock domain (CSD), K homology (KH) domain, amongst others (Holmqvist and Vogel, 2018). Additional regions may also contribute to RNA-protein interactions, like the disordered regions that confer flexibility to proteins. The overall fold of the protein and the recognition of different RNA-binding motifs determines the interaction with RNA in a sequence- and/or structure-specific dependent manner. RBPs and sRNAs networks have been extensively studied in Eukarya and Bacteria, with a current lack of information about this regulation in Archaea (Gelsinger and DiRuggiero, 2018). Though many RBPs can be found in bacteria only few have been shown to associate with sRNAs. However, these participate in a variety of reactions that affect the catalytic and molecular recognition properties of sRNAs.
RNA chaperones constitute a specific group of RBPs that transiently bind and induce structural changes in RNA substrates by melting RNA secondary structures (Woodson et al., 2018). Such structural rearrangements influence not only the stability of sRNA and mRNA molecules but also facilitate the basepairing of sRNAs and mRNAs. Moreover, RNA chaperones that bind simultaneously the sRNA and the target mRNA, bring them closely together in a complex, promoting the annealing and formation of stable RNA-RNA interactions. Though sRNA-mRNA basepairing can occur in the absence of RNA chaperones, their presence greatly accelerates this process (Rajkowitsch and Schroeder, 2007; Panja et al., 2013). Three major RNA chaperones that assist sRNA function in bacteria are currently known: the Sm family member Hfq (Santiago-Frangos and Woodson, 2018), the FinO family member ProQ (Smirnov et al., 2016) and the prototype of its family CsrA (Müller et al., 2019). Despite being widespread, these RBPs are not evenly present in bacteria and the interactome studies of these RNA chaperones indicate they preferably bind different sRNAs (Figure 1), suggesting more specialized roles for each of them (Holmqvist et al., 2016, 2018; Smirnov et al., 2016; Melamed et al., 2019).
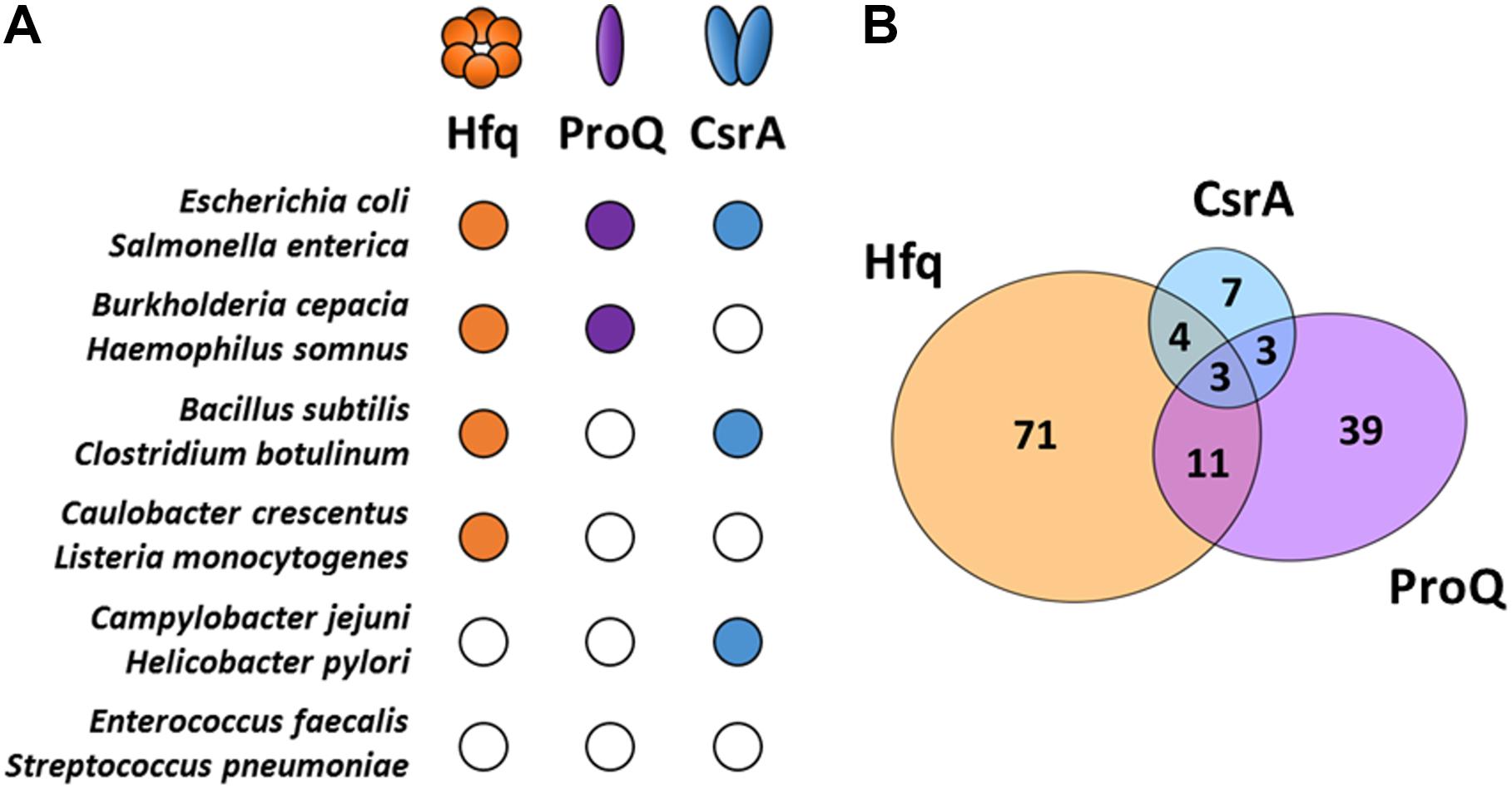
Figure 1. Major RNA chaperones in bacteria. (A) RNA chaperone distribution among representative bacteria. The Escherichia coli RNA chaperones Hfq, ProQ, and CsrA sequences were used as reference for comparison with other species using the co-occurrence analysis of the STRING database (Szklarczyk et al., 2017). Representative organisms were selected to illustrate the multiple combinations of RNA chaperones expression among bacteria. Close/open circles indicate presence/absence, respectively. (B) Venn diagram showing the intersection of sRNA substrates for each one of the RNA chaperones (Hfq, ProQ, and CsrA) in Salmonella enterica. Information retrieved from CLIP-seq datasets from Holmqvist et al. (2016, 2018).
Ribonucleases (RNases) are another group of specific RBPs that interact with sRNAs. These enzymes are responsible for the catalytic cleavage of all classes of RNA (Arraiano et al., 2010). The stability of sRNAs results from the interplay between RNA chaperones and RNases with the sRNAs, as RNA chaperones may protect or expose the sRNAs to the nucleolytic action of RNases (Holmqvist and Vogel, 2018). The main RNases implicated in sRNA turnover are the endonucleases RNase E and RNase III and the exonuclease PNPase (Saramago et al., 2014). In this mini review, we summarize the current information on the major RNA chaperones and RNases governing the activity of bacterial sRNAs.
RNA Chaperones
Hfq
Hfq is widely recognized as a global regulator and key element of sRNA-based networks. Hundreds of sRNA molecules have been reported in Escherichia coli, and ∼30% rely on Hfq to carry on their functions (Vogel and Luisi, 2011). Hfq is particularly important for the action of trans-encoded sRNAs (which are expressed from a different genomic region than their mRNA targets), stabilizing the imperfect basepairing between sRNA/mRNA pairs. At least in Gram-negative bacteria, Hfq primary role is to promote the annealing of sRNA-mRNA duplexes, acting as a molecular “matchmaker,” but its role in Gram-positive bacteria is more controversial (Woodson et al., 2018; Dos Santos et al., 2019). Interestingly, Hfq can bind other substrates including rRNA (Andrade et al., 2018), tRNA (Lee and Feig, 2008), and even DNA molecules (Cech et al., 2016). The wide substrate selection of Hfq suggests additional functions for this protein in the cell, such as its involvement in ribosome biogenesis and translation fidelity (Andrade et al., 2018), and DNA compaction (Jiang et al., 2015; Malabirade et al., 2017). Hfq is also involved in many protein-protein interactions (Caillet et al., 2019), namely with proteins involved in RNA degradation, such as RNase E (Morita et al., 2005), PNPase (Mohanty et al., 2004), and Poly(A) Polymerase (Le Derout et al., 2003). These Hfq-based complexes hint a close relationship between Hfq and the RNA degradation machinery.
Interactome studies identified thousands of Hfq-bound RNA pairs, dominated by mRNA-sRNA pairs exhibiting sequence complementarity (Holmqvist et al., 2016; Melamed et al., 2016; Mihailovic et al., 2018). Hfq binds single-stranded RNA showing a preference for (ARN)n motifs frequently found on mRNA (Link et al., 2009) and short poly(U) tails present at the 3′-end of sRNAs (Sauer and Weichenrieder, 2011; Sauer et al., 2012). Hfq binds transiently to sRNA and mRNA, dissociating from the RNA duplex soon after basepairing occurs (Fender et al., 2010; Hwang et al., 2011). This widely conserved protein is composed by two N-terminal structural motifs (Sm1 and Sm2) and a variable intrinsically disordered C-terminal tail. The Hfq Sm motifs are characteristic of the Sm/Lsm family of RNA-binding proteins (Møller et al., 2002). Members of this family typically adopt a multimer ring-like architecture, with Hfq assembling into an homohexamer. Multiple RNA-binding surfaces are consequently present in the Hfq ring: the proximal face, the distal face, the lateral rim and the C-terminal tail. The RNA is wrapped around the ring, causing reshaping of the RNA’s secondary structure and/or bringing two different strands of RNA into close proximity (Woodson et al., 2018).
sRNAs have been classified in two major classes according to their dependence on Hfq contact surfaces. The vast majority of bacterial sRNAs belong to class I. Hfq contacts these transcripts through binding of its proximal face to the unstructured U-rich stretches present at the 3′-end of sRNAs. On the other hand, the distal face of the Hfq ring preferentially interacts with ARN motifs in mRNAs. The basic patched rim surface may then interact with UA-rich sites present in both RNAs, coordinating the successful annealing between the sRNA-mRNA pair (Panja et al., 2013; Zhang et al., 2013; Schu et al., 2015). As an example, the iron-responsive class I sRNA RyhB relies on Hfq for successful interaction with its targets (Massé et al., 2003). The less abundant class II sRNAs bind Hfq more tightly, as the interaction is done via the proximal and distal faces of the ring (Panja et al., 2013). Class II sRNAs also use Hfq as a hub for promoting duplex formation to their target mRNAs. This is the case for the MgrR sRNA regulation of the eptB and ygdQ transcripts (Kwiatkowska et al., 2018). In both sRNA classes, the C-terminal acidic region of Hfq is suggested to help displace unmatched sRNA-mRNA by transiently competing with the core binding surfaces (Woodson et al., 2018). This competition not only allows for a rapid cycling through the pool of cellular RNAs, but also seems to drive substrate RNA specificity in different bacteria (Santiago-Frangos et al., 2017, 2019).
Hfq remodels RNA conformation by disrupting secondary structures, without the need to hydrolyze ATP. This intrinsic RNA chaperone capability is important to unfold structured RNAs, exposing unpaired RNA stretches for basepairing between complementary strands. One of the best characterized Hfq-dependent sRNAs is MicA, which was found to target the ompA mRNA (Udekwu et al., 2005). The RNA chaperone activity of Hfq is also important for remodeling MicA structural elements, altering its stability and binding specificities. Hfq binding rearranges MicA fold to allow exposure of the ompA-binding site for pairing that leads to translation repression (Andrade et al., 2013; Henderson et al., 2013). Interestingly, target downregulation may require both Hfq and sRNA independently of an sRNA/Hfq complex formation. The two-step regulation of the dgcM mRNA was firstly shown to require Hfq to unfold a 5′-end secondary structure that otherwise occludes the binding sites for the OmrA and OmrB sRNAs. Successful binding of OmrA/B to the early coding sequence of dgcM results in translation inhibition of the target mRNA (Hoekzema et al., 2019).
ProQ
ProQ is a recently identified RNA chaperone of the FinO family of RNA-binding proteins commonly found in Proteobacteria (Olejniczak and Storz, 2017). Most of the work on ProQ RNA substrates came from studies performed in E. coli, Salmonella enterica, and Legionella pneumophila, which identified a hundred mRNA transcripts and more than fifty sRNAs as ProQ ligands (Attaiech et al., 2016; Smirnov et al., 2016; Holmqvist et al., 2018; Westermann et al., 2019). ProQ is a monomeric protein with 25 kDa, composed of a α-helical N-terminal domain similar to the RNA-binding domain FinO and a β-sheet C-terminal region partially resembling the eukaryotic Tudor domain. Both regions are connected by a highly flexible and extended linker that is thought to allow the binding and protection of a class of sRNAs that form extended duplexes (Attaiech et al., 2017; Gonzalez et al., 2017; Olejniczak and Storz, 2017). Although both domains contribute to the pairing of complementary RNA molecules, the C-terminal is critical for the RNA strand exchange activity (Chaulk et al., 2011). Unlike Hfq, ProQ binding to RNA is sequence-independent but shows structure preference. ProQ binds double-stranded RNA and prefers highly structured RNAs (Smirnov et al., 2017b; Melamed et al., 2019). The FinO-like domain of ProQ is responsible for this substrate preference (Holmqvist et al., 2018).
Most sRNAs that bind ProQ have unknown functions so far. In contrast to Hfq, the majority of known ProQ-associated sRNAs act in cis promoting extensive perfect basepairing with the target mRNA encoded on the opposite strand (Smirnov et al., 2017b). However, ProQ was also found to regulate trans-acting sRNAs, assisting the imperfect basepairing with their target mRNAs. Two well characterized examples in Salmonella are known: the RaiZ sRNA-hupA mRNA and STnc540 sRNA-mgtB mRNA (Smirnov et al., 2017b; Westermann et al., 2019). ProQ binds RaiZ through its 3′-terminal stem-loops and promotes interaction of a linear region of this sRNA with the hupA mRNA. This three-partner ProQ/RaiZ/hupA mRNA complex results in impairment of hupA mRNA translation by preventing loading of the 30S ribosome subunit (Smirnov et al., 2017b). STnc540 sRNA also represses the expression of its target mRNA in a ProQ-dependent manner (Westermann et al., 2019). In both examples, ProQ is absolutely required for stability of the sRNAs, affecting their abundance.
Recent work in E. coli explores the RNA-RNA interactomes of Hfq and ProQ chaperones using RIL-seq (Melamed et al., 2019). Even though the interactome of ProQ was smaller than the one of Hfq, about a third of the RNA-RNA interactions were common between the two RNA chaperones, with examples like RybB and MalM sRNAs. This suggests complementary or competitive roles for these RBPs. An additional example is found in Salmonella, in the regulation mediated by the SraL sRNA. This sRNA binds to the 5′-UTR of the rho mRNA, an interaction that can be mediated by ProQ and/or Hfq (Silva et al., 2019). However, the RNAs bound by ProQ generally differ from those bound by Hfq. RIL-seq data revealed that while Hfq-bound RNAs were enriched in both sRNAs and mRNAs, ProQ-bound RNAs were mainly enriched for coding sequences (Melamed et al., 2019). This suggests that the RNA-RNA matchmaking activity of ProQ may not be generalized, unlike observed with Hfq that is primarily involved in sRNA-mediated regulation of mRNA translation. Additional roles for ProQ may include RNA protection from RNase attack or a participation in RNA modification.
CsrA
The CsrA protein was first discovered in E. coli and its function attributed to carbon storage and glycogen production, acting as a translational repressor of the glgC mRNA (Romeo et al., 1993; Romeo and Babitzke, 2018). In Pseudomonas aeruginosa the homolog protein is termed RsmA (for regulator of secondary metabolism) with paralogs (RsmF/N, RsmE, and RsmI) found in different Pseudomonas species (Reimmann et al., 2005; Marden et al., 2013; Morris et al., 2013). Members of the CsrA/RsmA family are conserved among Gammaproteobacteria and have been described as global bacterial regulators (Vakulskas et al., 2015). Here we will focus on the information available on the enterobacterial CsrA.
The E. coli CsrA is a ∼7 kDa RNA-binding protein and consists of a homodimer, each subunit with five β-strands, one α-helix and an unstructured C-terminal (Gutiérrez et al., 2005; Duss et al., 2014a). The recognition motif is the AUGGA sequence typically localized in the loop of a stem-loop, as determined by SELEX and confirmed through CLIP-seq (Dubey et al., 2005; Duss et al., 2014a; Holmqvist et al., 2016). The most well characterized activity of CsrA is the binding of mRNA, resulting in repression or activation of translation. Typically, CsrA binding occurs in the RBS sequence or overlaps with the initiation codon, leading to a direct inhibition of translation. CsrA can also regulate transcript stability, either by promoting or blocking the access of the mRNA to ribonucleases (Dubey et al., 2005; Schubert et al., 2007; Yakhnin et al., 2013). CsrA also protects sRNAs from RNase E-mediated degradation, as it was shown for the small RNAs CsrB and CsrC (Weilbacher et al., 2003; Vakulskas et al., 2016). Interestingly, CsrA activity on target mRNAs is mostly regulated by the action of the CsrB and CsrC sRNAs (RsmY and RsmZ in Pseudomonads). These highly structured sRNAs are composed of repetitive sequence elements of the recognition motif GGA (22 per molecule in CsrB and 13 in CsrC) with high affinity for the CsrA binding site (Liu et al., 1997; Weilbacher et al., 2003; Duss et al., 2014b). Consequently, CsrB and CsrC act as “sponges” that sequester CsrA protein and prevent its activity (Romeo et al., 2013). The sRNA McaS is also able to modulate CsrA activity though it contains only two recognition sites (Jørgensen et al., 2013). Transcriptomic studies performed in E. coli showed that CsrA affects the abundance of 11 sRNAs, including CsrB and CsrC. Additionally, CLIP-seq analysis followed by in vitro studies confirmed CsrA binds other sRNAs with high affinity (Potts et al., 2017). In particular, the interaction of CsrA with the sRNAs GadY, Spot 42, and GcvB was shown to significantly overlap with known basepairing regions for these sRNA–mRNA pairs, suggesting that CsrA binding inhibits formation of these RNA duplexes.
CsrA was recently described to act as an RNA chaperone that indirectly promotes the basepairing between the trans-acting SR1 sRNA and its primary target the ahrC mRNA, which encodes the transcription activator of the arginine catabolic operons in Bacillus subtilis (Müller et al., 2019). In vitro binding studies demonstrated that CsrA binds these RNAs with high affinity, in the nanomolar range, even in the presence of an mRNA competitor. Further mutational analysis of the SR1 sRNA and the ahrC mRNA confirmed binding of CsrA to both transcripts. CsrA facilitates the binding of the SR1 sRNA downstream the start codon of the ahrC mRNA and induces conformational changes in the RBS preventing its translation (Müller et al., 2019). Importantly, Hfq was not found to catalyze this interaction and ProQ is not expressed in B. subtilis. Interestingly, this suggests that CsrA may act as an alternative RNA chaperone to Hfq and ProQ in assisting sRNA-mRNA basepairing.
RNA- and DNA-Binding Multifunctional Proteins as RNA Chaperones
While Hfq, ProQ, and CsrA may be considered the major RNA chaperones interacting with sRNAs, additional RBPs are known to assist RNA folding and bind sRNAs. Two of such examples include the cold shock proteins (CSPs) and the StpA protein. CSPs are a group of small proteins that display the RNA-binding cold shock domain (CSD) (Phadtare and Severinov, 2010) and can passively remodel RNA structures (Woodson et al., 2018). The major cold shock protein of E. coli is CspA that binds RNA with low sequence specificity and in a cooperative fashion (Jiang et al., 1997). CspA activity results in the melting of RNA secondary structures, which favors the unfolded state of transcripts enabling their translation (Rennella et al., 2017). In Staphylococcus aureus, a RIP-chip assay identified the RNA targets of CspA, which included several sRNAs (Caballero et al., 2018). Accordingly, it is likely that CspA assists sRNA-mediated regulation. Though not all members of the CSP family are induced by cold, they may be relevant for adaptation to other stresses (Yamanaka et al., 1998). For example, CspC and CspE stimulate translation of rpoS (encoding the stress sigma factor S) possibly by altering the secondary structure of the rpoS mRNA in E. coli (Phadtare and Inouye, 2001; Phadtare et al., 2006) and affect virulence in Salmonella (Michaux et al., 2017). E. coli StpA is another example of an RNA chaperone that remodels RNA structures without hydrolyzing ATP. StpA has RNA annealing and RNA strand displacement activities (Zhang et al., 1995, 1996). It binds weakly to RNA with preference for unstructured molecules, promoting RNA conformational changes by loosening RNA secondary structures (Mayer et al., 2007). Importantly, the RNA chaperone StpA was found to interact with the small RNA MicF. StpA regulates the stability of MicF sRNA and accelerates its base pairing with the target ompF mRNA, acting as a major regulator of the OmpF porin expression (Deighan et al., 2000).
RNAses
RNase E
Homologs of E. coli RNase E have been identified in the majority of Proteobacteria classes (Aït-Bara and Carpousis, 2015). This endoribonuclease is composed by a conserved N-terminal catalytic region with an embedded RNA-binding S1 domain, and the unstructured C-terminal non-catalytic region (Bandyra and Luisi, 2018). RNase E cleaves single-stranded RNA, preferably enriched in A/U nucleotides with a stem-loop upstream (Del Campo et al., 2015). Although it prefers substrates with 5′-end monophosphorylated, in vivo it is also functional in a 5′-monophosphate-independent pathway (Clarke et al., 2014). Upon Hfq dissociation from the sRNA-mRNA pairs, RNase E can reach and cleave the target mRNA in a linear stretch at the 3′-end of the duplex region (Waters et al., 2017). The sRNAs RyhB and GcvB are typical examples in which sRNA pairing with the coding region promotes mRNA decay via the recruitment of RNase E (Massé et al., 2003; Morita et al., 2005; Lalaouna et al., 2019). Often the base paired sRNA is also degraded with the mRNA. McaS sRNA bound to Hfq interacts with both RNase E and its substrate csgD mRNA, leading to the cleavage of both RNAs (Andreassen et al., 2018). RNase E is also critical for the processing of sRNAs from the 3′ UTR of mRNAs, including the release of the CpxQ sRNA from the 3′-end of the cpxP mRNA (Chao and Vogel, 2016) and the processing of the precursor RNA to originate the functional ArcZ (Chao et al., 2017). A paralog named RNase G that contains only the catalytic domain of RNase E is also present in E. coli and other bacteria (Aït-Bara and Carpousis, 2015). Although this non-essential enzyme shares common activities with RNase E, including rRNA processing and mRNA turnover, no role has been ascribed for RNase G in sRNA processing (Mackie, 2013). Interestingly, RNase E activity on sRNAs can be modulated by other RNA-binding proteins. This is the case of RapZ, an RBP that functions as an adaptor protein in E. coli. RapZ binds to the central stem loop of the sRNA GlmZ and RNase E is then recruited for the processing of this sRNA (Göpel et al., 2013), which regulates the glmS mRNA encoding the glucosamine-6-phosphate (GlcN6P) synthase. RapZ was recently found to be the receptor for GlcN6P (Khan et al., 2020).
RNase III
RNase III is a widely distributed endoribonuclease involved in the processing of double-stranded RNAs (dsRNAs). E. coli RNase III acts as a 52 kDa homodimer, with the catalytic N-terminal domain connected by a short linker to the C-terminal dsRNA-binding domain (Li and Nicholson, 1996). RNase III can cleave the duplex RNA formed between complementary regions of sRNA and its target mRNA (Lybecker et al., 2014; Altuvia et al., 2018). The target-coupled pathway for RNase III degradation mediated via sRNAs is commonly observed in bacteria. In Salmonella, RNase III is responsible for the degradation of the dsRNA formed between MicA and its target ompA mRNA upon basepairing (Viegas et al., 2011). In B. subtilis, the 3′-end of the antitoxin RatA sRNA forms a large duplex with the txpA mRNA that is cleaved by RNase III and prevents the translation of TxpA toxin (Durand et al., 2012). In Streptococcus pyogenes, the type II CRISPR-Cas system depends on the maturation of CRISPR RNA by RNase III (Deltcheva et al., 2011).
PNPase
Polynucleotide phosphorylase (PNPase) is a highly conserved 3′–5′ exoribonuclease that processively degrades RNA (Saramago et al., 2014; Dos Santos et al., 2018). PNPase adopts a homotrimeric organization with a ring-like structure, each monomer having a molecular weight of 78 kDa and holding two RNA-binding domains, KH and S1, on the C-terminal (Shi et al., 2008). In E. coli, PNPase is the main enzyme involved in the degradation of sRNAs that are not bound to Hfq, as shown for the regulation of different Hfq-dependent sRNAs, such as MicA, GlmY, RyhB, and SgrS levels (Andrade et al., 2012, 2013). This effect is growth-phase regulated and agrees with previous work in which PNPase was found to degrade sRNAs in the absence of their primary target mRNAs (Andrade and Arraiano, 2008). Additionally, PNPase has an unexpected role in Listeria monocytogenes, being responsible for the correct processing of an orphan CRISPR RNA (Sesto et al., 2014).
Although to a lesser extent, additional RNases are involved in the regulation of sRNAs. The degradative enzymes YbeY and RNase R are illustrative examples. YbeY is a highly conserved endoribonuclease commonly associated with rRNA processing (Davies et al., 2010). However, YbeY was shown to bind sRNAs and regulate the levels of sRNAs and mRNAs (Pandey et al., 2011). In Sinorhizobium meliloti it was shown that YbeY could cleave sRNA-mRNA pairs (Saramago et al., 2017). Inactivation of YbeY in E. coli cells exposed to hydroxyurea resulted in the upregulation of many sRNAs involved in the adaptation to oxidative stress (Pandey et al., 2014). Additionally, Vibrio cholerae YbeY was found to regulate the abundance of the sRNAs Qrr1-4 (Vercruysse et al., 2014), which are involved in quorum-sensing and virulence (Tu and Bassler, 2007). RNase R is a unique 3′–5′ exoribonuclease able to degrade highly structured RNAs (Dos Santos et al., 2018). There are few described examples of RNase R involved in the regulation of sRNAs. During cold shock in E. coli, RNase R is required for the correct processing of the sRNA SsrA/tmRNA (transfer-messenger RNA), involved in protein quality control and ribosome recycling (Cairrão et al., 2003). RNase R was also described to control sRNA stability of the sRNA SR4 and its target bsrG mRNA that together constitute a temperature-dependent type I toxin/antitoxin system in Bacillus subtilis (Jahn et al., 2012).
Conclusion
RNA chaperones can modify sRNA structure, facilitate the basepairing of sRNAs to their target mRNAs and together with RNases control sRNA stability (Figure 2). However, some RNA chaperones seem to be specific of some species and the activities performed by these regulators may be compensated by other still unidentified RNA-binding proteins. Several RBPs with unorthodox RNA-binding domains have been identified in humans, expanding the number of proteins that can associate with RNA (Castello et al., 2016). Therefore, it is also possible that additional and probably unconventional RBPs interacting with sRNA are going to be discovered in bacteria. The RNA chaperone ProQ offers us a good example of this potential. A new RNA-seq methodology associated to sample fractionation (Grad-seq) contributed to the identification of ProQ as a novel RBP interacting with sRNAs (Smirnov et al., 2016). Application of this and similar methods may contribute to expand the number of sRNA-protein partners and helps to shed light on the many still unknown functions and physiological roles of sRNAs.
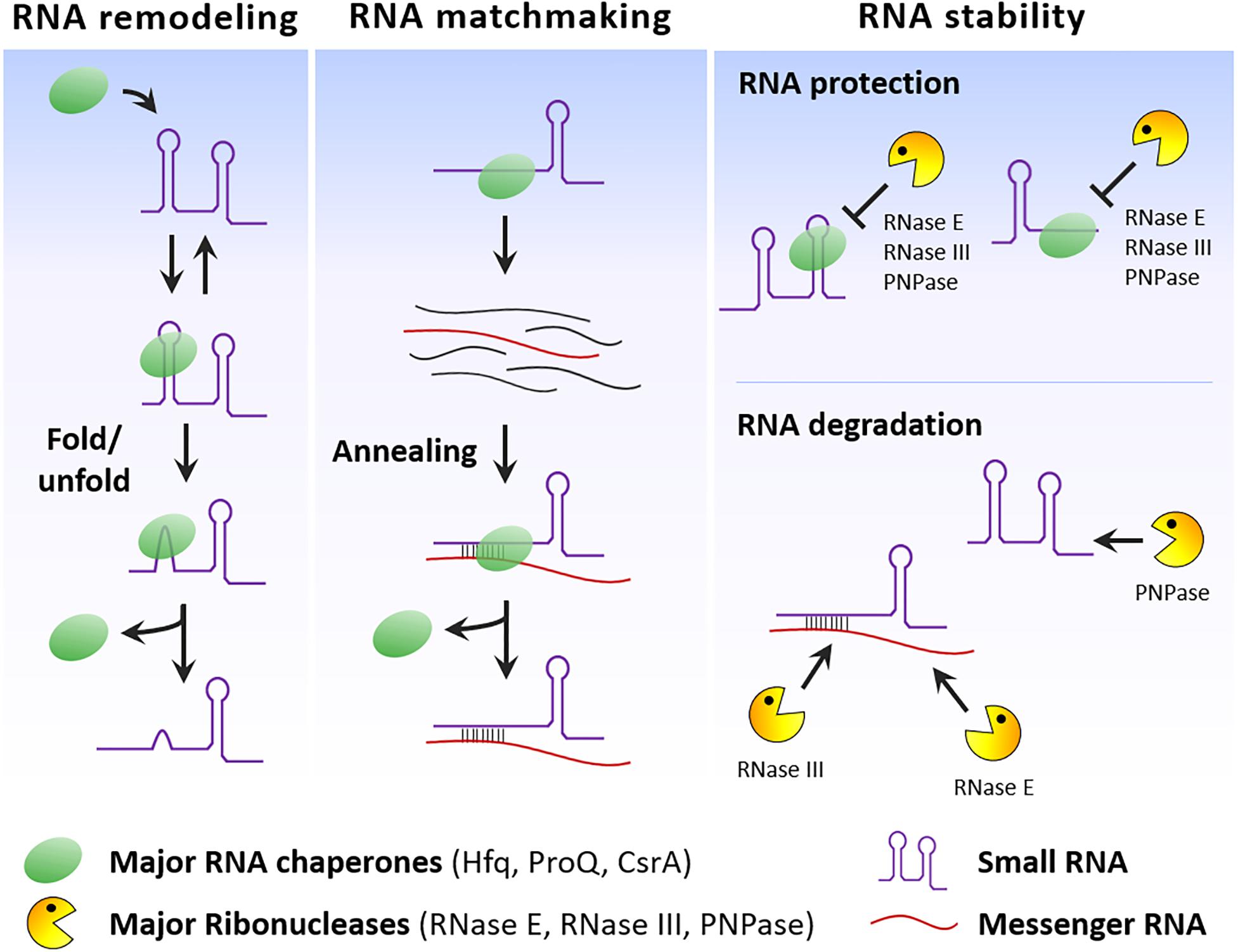
Figure 2. RNA chaperone and RNase activities on sRNAs. Simplified scheme that illustrates the effect of these RNA-binding proteins in different aspects of the sRNA lifetime, namely on the folding of sRNA secondary structures, promotion of sRNA/mRNA basepairing and control of sRNA stability.
Author Contributions
JA outlined the manuscript. AQ, AS, and RS prepared the figures. CA and JA supervised the work. All authors wrote and participated in preparation of the final manuscript.
Funding
This work was supported by Project LISBOA-01-0145-FEDER-007660 (Microbiologia Molecular, Estrutural e Celular) funded by FEDER through COMPETE2020 - Programa Operacional Competitividade e Internacionalização (POCI) and by FCT—Fundação para a Ciência e a Tecnologia (Portugal), including Program IF (IF/00961/2014) and Grants PTDC/IMI-MIC/4463/2014 and PTDC/BIA-MIC/32525/2017 to JA, Grant PTDC/BIA-MIC/1399/2014 to CA, the Doctoral fellowships PD/BD/135487/2018 to AQ, and PD/BD/146136/2019 to AS.
Conflict of Interest
The authors declare that the research was conducted in the absence of any commercial or financial relationships that could be construed as a potential conflict of interest.
References
Aït-Bara, S., and Carpousis, A. J. (2015). RNA degradosomes in bacteria and chloroplasts: classification, distribution and evolution of RNase E homologs. Mol. Microbiol. 97, 1021–1135 doi: 10.1111/mmi.13095
Altuvia, Y., Bar, A., Reiss, N., Karavani, E., Argaman, L., and Margalit, H. (2018). In vivo cleavage rules and target repertoire of RNase III in Escherichia coli. Nucleic Acids Res. 46, 10380–10394. doi: 10.1093/nar/gky684
Andrade, J. M., and Arraiano, C. M. (2008). PNPase is a key player in the regulation of small RNAs that control the expression of outer membrane proteins. RNA 14, 543–551. doi: 10.1261/rna.683308
Andrade, J. M., Dos Santos, R. F., Chelysheva, I., Ignatova, Z., and Arraiano, C. M. (2018). The RNA-binding protein Hfq is important for ribosome biogenesis and affects translation fidelity. EMBO J. 37:e97631. doi: 10.15252/embj.201797631
Andrade, J. M., Pobre, V., and Arraiano, C. M. (2013). Small RNA modules confer different stabilities and interact differently with multiple targets. PLoS One 8:e52866. doi: 10.1371/journal.pone.0052866
Andrade, J. M., Pobre, V., Matos, A. M., and Arraiano, C. M. (2012). The crucial role of PNPase in the degradation of small RNAs that are not associated with Hfq. RNA 18, 844–855. doi: 10.1261/rna.029413.111
Andreassen, P. R., Pettersen, J. S., Szczerba, M., Valentin-Hansen, P., Møller-Jensen, J., and Jørgensen, M. G. (2018). sRNA-dependent control of curli biosynthesis in Escherichia coli: McaS directs endonucleolytic cleavage of csgD mRNA. Nucleic Acids Res. 46, 6746–6760. doi: 10.1093/nar/gky479
Arraiano, C. M., Andrade, J. M., Domingues, S., Guinote, I. B., Malecki, M., Matos, R. G., et al. (2010). The critical role of RNA processing and degradation in the control of gene expression. FEMS Microbiol. Rev. 34, 883–923. doi: 10.1111/j.1574-6976.2010.00242.x
Attaiech, L., Boughammoura, A., Brochier-Armanet, C., Allatif, O., Peillard-Fiorente, F., Edwards, R. A., et al. (2016). Silencing of natural transformation by an RNA chaperone and a multitarget small RNA. Proc. Natl. Acad. Sci. U.S.A. 113, 8813–8818. doi: 10.1073/pnas.1601626113
Attaiech, L., Glover, J. N. M., and Charpentier, X. (2017). RNA chaperones step out of Hfq’s shadow. Trends Microbiol. 25, 247–249. doi: 10.1016/j.tim.2017.01.006
Bandyra, K. J., and Luisi, B. F. (2018). RNase E and the high-fidelity orchestration of RNA metabolism. Microbiol. Spectr. 6:RWR-0008-2017. doi: 10.1128/microbiolspec.RWR-0008-2017
Caballero, C. J., Menendez-Gil, P., Catalan-Moreno, A., Vergara-Irigaray, M., García, B., Segura, V., et al. (2018). The regulon of the RNA chaperone CspA and its auto-regulation in Staphylococcus aureus. Nucleic Acids Res. 46, 1345–1361. doi: 10.1093/nar/gkx1284
Caillet, J., Baron, B., Boni, I. V., Caillet-Saguy, C., and Hajnsdorf, E. (2019). Identification of protein-protein and ribonucleoprotein complexes containing Hfq. Sci. Rep. 9:14054. doi: 10.1038/s41598-019-50562-w
Cairrão, F., Cruz, A., Mori, H., and Arraiano, C. M. (2003). Cold shock induction of RNase R and its role in the maturation of the quality control mediator SsrA/tmRNA. Mol. Microbiol. 50, 1349–1360. doi: 10.1046/j.1365-2958.2003.03766.x
Castello, A., Fischer, B., Frese, C. K., Horos, R., Alleaume, A.-M., Foehr, S., et al. (2016). Comprehensive identification of RNA-binding domains in human cells. Mol. Cell 63, 696–710. doi: 10.1016/j.molcel.2016.06.029
Cech, G. M., Szalewska-Pałasz, A., Kubiak, K., Malabirade, A., Grange, W., Arluison, V., et al. (2016). The Escherichia coli Hfq protein: an unattended DNA-transactions regulator. Front. Mol. Biosci. 3:36. doi: 10.3389/fmolb.2016.00036
Chao, Y., Li, L., Girodat, D., Förstner, K. U., Said, N., Corcoran, C., et al. (2017). In vivo cleavage map illuminates the central role of RNase E in coding and non-coding RNA pathways. Mol. Cell 65, 39–51. doi: 10.1016/j.molcel.2016.11.002
Chao, Y., and Vogel, J. (2016). A 3’ UTR-derived small RNA provides the regulatory noncoding arm of the inner membrane stress response. Mol. Cell 61, 352–363. doi: 10.1016/j.molcel.2015.12.023
Chaulk, S. G., Smith Frieday, M. N., Arthur, D. C., Culham, D. E., Edwards, R. A., Soo, P., et al. (2011). ProQ is an RNA chaperone that controls ProP levels in Escherichia coli. Biochemistry 50, 3095–3106. doi: 10.1021/bi101683a
Clarke, J. E., Kime, L., Romero, A. D., and McDowall, K. J. (2014). Direct entry by RNase E is a major pathway for the degradation and processing of RNA in Escherichia coli. Nucleic Acids Res. 42, 11733–11751. doi: 10.1093/nar/gku808
Davies, B. W., Köhrer, C., Jacob, A. I., Simmons, L. A., Zhu, J., Aleman, L. M., et al. (2010). Role of Escherichia coli YbeY, a highly conserved protein, in rRNA processing. Mol. Microbiol. 78, 506–518. doi: 10.1111/j.1365-2958.2010.07351.x
Deighan, P., Free, A., and Dorman, C. J. (2000). A role for the Escherichia coli H-NS-like protein StpA in OmpF porin expression through modulation of micF RNA stability. Mol. Microbiol. 38, 126–139. doi: 10.1046/j.1365-2958.2000.02120.x
Del Campo, C., Bartholomäus, A., Fedyunin, I., and Ignatova, Z. (2015). Secondary structure across the bacterial transcriptome reveals versatile roles in mRNA regulation and function. PLoS Genet. 11:e1005613. doi: 10.1371/journal.pgen.1005613
Deltcheva, E., Chylinski, K., Sharma, C. M., Gonzales, K., Chao, Y., Pirzada, Z. A., et al. (2011). CRISPR RNA maturation by trans-encoded small RNA and host factor RNase III. Nature 471, 602–607. doi: 10.1038/nature09886
Dos Santos, R. F., Arraiano, C. M., and Andrade, J. M. (2019). New molecular interactions broaden the functions of the RNA chaperone Hfq. Curr. Genet. 65, 1313–1319. doi: 10.1007/s00294-019-00990-y
Dos Santos, R. F., Quendera, A. P., Boavida, S., Seixas, A. F., Arraiano, C. M., and Andrade, J. M. (2018). Major 3’-5’ exoribonucleases in the metabolism of coding and non-coding RNA. Prog. Mol. Biol. Transl. Sci. 159, 101–155. doi: 10.1016/bs.pmbts.2018.07.005
Dubey, A. K., Baker, C. S., Romeo, T., and Babitzke, P. (2005). RNA sequence and secondary structure participate in high-affinity CsrA-RNA interaction. RNA 11, 1579–1587. doi: 10.1261/rna.2990205
Durand, S., Gilet, L., Bessières, P., Nicolas, P., and Condon, C. (2012). Three essential ribonucleases-RNase Y, J1, and III-control the abundance of a majority of Bacillus subtilis mRNAs. PLoS Genet. 8:e1002520. doi: 10.1371/journal.pgen.1002520
Duss, O., Michel, E., Dit Konté, N. D., Schubert, M., and Allain, F. H. T. (2014a). Molecular basis for the wide range of affinity found in Csr/Rsm protein-RNA recognition. Nucleic Acids Res. 42, 5332–5346. doi: 10.1093/nar/gku141
Duss, O., Michel, E., Yulikov, M., Schubert, M., Jeschke, G., and Allain, F. H.-T. (2014b). Structural basis of the non-coding RNA RsmZ acting as a protein sponge. Nature 509, 588–592. doi: 10.1038/nature13271
Fender, A., Elf, J., Hampel, K., Zimmermann, B., and Wagner, E. G. H. (2010). RNAs actively cycle on the Sm-like protein Hfq. Genes Dev. 24, 2621–2626. doi: 10.1101/gad.591310
Gelsinger, D. R., and DiRuggiero, J. (2018). The non-coding regulatory RNA revolution in archaea. Genes (Basel) 9:141. doi: 10.3390/genes9030141
Gonzalez, G. M., Hardwick, S. W., Maslen, S. L., Skehel, J. M., Holmqvist, E., Vogel, J., et al. (2017). Structure of the Escherichia coli ProQ RNA-binding protein. RNA 23, 696–711. doi: 10.1261/rna.060343.116
Göpel, Y., Papenfort, K., Reichenbach, B., Vogel, J., and Görke, B. (2013). Targeted decay of a regulatory small RNA by an adaptor protein for RNase E and counteraction by an anti-adaptor RNA. Genes Dev. 27, 552–564. doi: 10.1101/gad.210112.112
Gutiérrez, P., Li, Y., Osborne, M. J., Pomerantseva, E., Liu, Q., and Gehring, K. (2005). Solution structure of the carbon storage regulator protein CsrA from Escherichia coli. J. Bacteriol. 187, 3496–3501. doi: 10.1128/JB.187.10.3496-3501.2005
Henderson, C. A., Vincent, H. A., Stone, C. M., Phillips, J. O., Cary, P. D., Gowers, D. M., et al. (2013). Characterization of MicA interactions suggests a potential novel means of gene regulation by small non-coding RNAs. Nucleic Acids Res. 41, 3386–3397. doi: 10.1093/nar/gkt008
Hoekzema, M., Romilly, C., Holmqvist, E., and Wagner, E. G. H. (2019). Hfq−dependent mRNA unfolding promotes sRNA −based inhibition of translation. EMBO J. 38:e101199. doi: 10.15252/embj.2018101199
Holmqvist, E., Li, L., Bischler, T., Barquist, L., and Vogel, J. (2018). Global maps of ProQ binding In Vivo reveal target recognition via RNA structure and stability control at mRNA 3’ ends. Mol. Cell 70, 971–982.e6. doi: 10.1016/j.molcel.2018.04.017
Holmqvist, E., and Vogel, J. (2018). RNA-binding proteins in bacteria. Nat. Rev. Microbiol. 16, 601–615. doi: 10.1038/s41579-018-0049-5
Holmqvist, E., Wright, P. R., Li, L., Bischler, T., Barquist, L., Reinhardt, R., et al. (2016). Global RNA recognition patterns of post-transcriptional regulators Hfq and CsrA revealed by UV crosslinking in vivo. EMBO J. 35, 991–1011. doi: 10.15252/embj.201593360
Hwang, W., Arluison, V., and Hohng, S. (2011). Dynamic competition of DsrA and rpoS fragments for the proximal binding site of Hfq as a means for efficient annealing. Nucleic Acids Res. 39, 5131–5139. doi: 10.1093/nar/gkr075
Jahn, N., Preis, H., Wiedemann, C., and Brantl, S. (2012). BsrG/SR4 from Bacillus subtilis - the first temperature-dependent type I toxin-antitoxin system. Mol. Microbiol. 83, 579–598. doi: 10.1111/j.1365-2958.2011.07952.x
Jiang, K., Zhang, C., Guttula, D., Liu, F., Van Kan, J. A., Lavelle, C., et al. (2015). Effects of Hfq on the conformation and compaction of DNA. Nucleic Acids Res. 43, 4332–4341. doi: 10.1093/nar/gkv268
Jiang, W., Hou, Y., and Inouye, M. (1997). CspA, the major cold-shock protein of Escherichia coli, is an RNA chaperone. J. Biol. Chem. 272, 196–202. doi: 10.1074/jbc.272.1.196
Jørgensen, M. G., Thomason, M. K., Havelund, J., Valentin-Hansen, P., and Storz, G. (2013). Dual function of the McaS small RNA in controlling biofilm formation. Genes Dev. 27, 1132–1145. doi: 10.1101/gad.214734.113
Khan, M. A., Durica-Mitic, S., Göpel, Y., Heermann, R., and Görke, B. (2020). Small RNA-binding protein RapZ mediates cell envelope precursor sensing and signaling in Escherichia coli. EMBO J. 39:e103848. doi: 10.15252/embj.2019103848
Kwiatkowska, J., Wroblewska, Z., Johnson, K. A., and Olejniczak, M. (2018). The binding of Class II sRNA MgrR to two different sites on matchmaker protein Hfq enables efficient competition for Hfq and annealing to regulated mRNAs. RNA 24, 1761–1784. doi: 10.1261/rna.067777.118
Lalaouna, D., Eyraud, A., Devinck, A., Prévost, K., and Massé, E. (2019). GcvB small RNA uses two distinct seed regions to regulate an extensive targetome. Mol. Microbiol. 111, 473–486. doi: 10.1111/mmi.14168
Le Derout, J., Folichon, M., Briani, F., Dehò, G., Régnier, P., and Hajnsdorf, E. (2003). Hfq affects the length and the frequency of short oligo(A) tails at the 3’ end of Escherichia coli rpsO mRNAs. Nucleic Acids Res. 31, 4017–4023. doi: 10.1093/nar/gkg456
Lee, T., and Feig, A. L. (2008). The RNA binding protein Hfq interacts specifically with tRNAs. RNA 14, 514–523. doi: 10.1261/rna.531408
Li, H., and Nicholson, A. W. (1996). Defining the enzyme binding domain of a ribonuclease III processing signal. Ethylation interference and hydroxyl radical footprinting using catalytically inactive RNase III mutants. EMBO J. 15, 1421–1433. doi: 10.1002/j.1460-2075.1996.tb00484.x
Link, T. M., Valentin-Hansen, P., and Brennan, R. G. (2009). Structure of Escherichia coli Hfq bound to polyriboadenylate RNA. Proc. Natl. Acad. Sci. U.S.A. 106, 19292–19297. doi: 10.1073/pnas.0908744106
Liu, M. Y., Gui, G., Wei, B., Preston, J. F., Oakford, L., Yüksel, U., et al. (1997). The RNA molecule CsrB binds to the global regulatory protein CsrA and antagonizes its activity in Escherichia coli. J. Biol. Chem. 272, 17502–17510. doi: 10.1074/jbc.272.28.17502
Lybecker, M., Zimmermann, B., Bilusic, I., Tukhtubaeva, N., and Schroeder, R. (2014). The double-stranded transcriptome of Escherichia coli. Proc. Natl. Acad. Sci. U.S.A. 111, 3134–3139. doi: 10.1073/pnas.1315974111
Mackie, G. A. (2013). RNase E: at the interface of bacterial RNA processing and decay. Nat. Rev. Microbiol. 11, 45–57. doi: 10.1038/nrmicro2930
Malabirade, A., Jiang, K., Kubiak, K., Diaz-Mendoza, A., Liu, F., van Kan, J. A., et al. (2017). Compaction and condensation of DNA mediated by the C-terminal domain of Hfq. Nucleic Acids Res. 45, 7299–7308. doi: 10.1093/nar/gkx431
Marden, J. N., Diaz, M. R., Walton, W. G., Gode, C. J., Betts, L., Urbanowski, M. L., et al. (2013). An unusual CsrA family member operates in series with RsmA to amplify posttranscriptional responses in Pseudomonas aeruginosa. Proc. Natl. Acad. Sci. U.S.A. 110, 15055–15060. doi: 10.1073/pnas.1307217110
Massé, E., Escorcia, F. E., and Gottesman, S. (2003). Coupled degradation of a small regulatory RNA and its mRNA targets in Escherichia coli. Genes Dev. 17, 2374–2383. doi: 10.1101/gad.1127103
Mayer, O., Rajkowitsch, L., Lorenz, C., Konrat, R., and Schroeder, R. (2007). RNA chaperone activity and RNA-binding properties of the E. coli protein StpA. Nucleic Acids Res. 35, 1257–1269. doi: 10.1093/nar/gkl1143
Melamed, S., Adams, P. P., Zhang, A., Zhang, H., and Storz, G. (2019). RNA-RNA Interactomes of ProQ and Hfq reveal overlapping and competing roles. Mol. Cell 77, 411–425.e7. doi: 10.1016/j.molcel.2019.10.022
Melamed, S., Peer, A., Faigenbaum-Romm, R., Gatt, Y. E., Reiss, N., Bar, A., et al. (2016). Global mapping of small RNA-target interactions in bacteria. Mol. Cell 63, 884–897. doi: 10.1016/j.molcel.2016.07.026
Michaux, C., Holmqvist, E., Vasicek, E., Sharan, M., Barquist, L., Westermann, A. J., et al. (2017). RNA target profiles direct the discovery of virulence functions for the cold-shock proteins CspC and CspE. Proc. Natl. Acad. Sci. U.S.A. 114, 6824–6829. doi: 10.1073/pnas.1620772114
Mihailovic, M. K., Vazquez-Anderson, J., Li, Y., Fry, V., Vimalathas, P., Herrera, D., et al. (2018). High-throughput in vivo mapping of RNA accessible interfaces to identify functional sRNA binding sites. Nat. Commun. 9:4084. doi: 10.1038/s41467-018-06207-z
Mohanty, B. K., Maples, V. F., and Kushner, S. R. (2004). The Sm-like protein Hfq regulates polyadenylation dependent mRNA decay in Escherichia coli. Mol. Microbiol. 54, 905–920. doi: 10.1111/j.1365-2958.2004.04337.x
Møller, T., Franch, T., Højrup, P., Keene, D. R., Bächinger, H. P., Brennan, R. G., et al. (2002). Hfq: a bacterial Sm-like protein that mediates RNA-RNA interaction. Mol. Cell 9, 23–30. doi: 10.1016/s1097-2765(01)00436-1
Morita, T., Maki, K., and Aiba, H. (2005). RNase E-based ribonucleoprotein complexes: mechanical basis of mRNA destabilization mediated by bacterial noncoding RNAs. Genes Dev. 19, 2176–2186. doi: 10.1101/gad.1330405
Morris, E. R., Hall, G., Li, C., Heeb, S., Kulkarni, R. V., Lovelock, L., et al. (2013). Structural rearrangement in an RsmA/CsrA Ortholog of Pseudomonas aeruginosa creates a dimeric RNA-binding protein, RsmN. Structure 21, 1659–1671. doi: 10.1016/j.str.2013.07.007
Müller, P., Gimpel, M., Wildenhain, T., and Brantl, S. (2019). A new role for CsrA: promotion of complex formation between an sRNA and its mRNA target in Bacillus subtilis. RNA Biol. 16, 972–987. doi: 10.1080/15476286.2019.1605811
Olejniczak, M., and Storz, G. (2017). ProQ/FinO-domain proteins: another ubiquitous family of RNA matchmakers? Mol. Microbiol. 104, 905–915. doi: 10.1111/mmi.13679
Pandey, S. P., Minesinger, B. K., Kumar, J., and Walker, G. C. (2011). A highly conserved protein of unknown function in Sinorhizobium meliloti affects sRNA regulation similar to Hfq. Nucleic Acids Res. 39, 4691–4708. doi: 10.1093/nar/gkr060
Pandey, S. P., Winkler, J. A., Li, H., Camacho, D. M., Collins, J. J., and Walker, G. C. (2014). Central role for RNase YbeY in Hfq-dependent and Hfq-independent small-RNA regulation in bacteria. BMC Genomics 15:121. doi: 10.1186/1471-2164-15-121
Panja, S., Schu, D. J., and Woodson, S. A. (2013). Conserved arginines on the rim of Hfq catalyze base pair formation and exchange. Nucleic Acids Res. 41, 7536–7546. doi: 10.1093/nar/gkt521
Phadtare, S., and Inouye, M. (2001). Role of CspC and CspE in regulation of expression of RpoS and UspA, the stress response proteins in Escherichia coli. J. Bacteriol. 183, 1205–1214. doi: 10.1128/JB.183.4.1205-1214.2001
Phadtare, S., and Severinov, K. (2010). RNA remodeling and gene regulation by cold shock proteins. RNA Biol. 7, 788–795. doi: 10.4161/rna.7.6.13482
Phadtare, S., Tadigotla, V., Shin, W. H., Sengupta, A., and Severinov, K. (2006). Analysis of Escherichia coli global gene expression profiles in response to overexpression and deletion of CspC and CspE. J. Bacteriol. 188, 2521–2527. doi: 10.1128/JB.188.7.2521-2527.2006
Potts, A. H., Vakulskas, C. A., Pannuri, A., Yakhnin, H., Babitzke, P., and Romeo, T. (2017). Global role of the bacterial post-transcriptional regulator CsrA revealed by integrated transcriptomics. Nat. Commun. 8:1596. doi: 10.1038/s41467-017-01613-1
Rajkowitsch, L., and Schroeder, R. (2007). Dissecting RNA chaperone activity. RNA 13, 2053–2060. doi: 10.1261/rna.671807
Reimmann, C., Valverde, C., Kay, E., and Haas, D. (2005). Posttranscriptional repression of GacS/GacA-controlled genes by the RNA-binding protein RsmE acting together with RsmA in the biocontrol strain Pseudomonas fluorescens CHA0. J. Bacteriol. 187, 276–285. doi: 10.1128/JB.187.1.276-285.2005
Rennella, E., Sára, T., Juen, M., Wunderlich, C., Imbert, L., Solyom, Z., et al. (2017). RNA binding and chaperone activity of the E. coli cold-shock protein CspA. Nucleic Acids Res. 45, 4255–4268. doi: 10.1093/nar/gkx044
Romeo, T., and Babitzke, P. (2018). Global Regulation by CsrA and Its RNA Antagonists. Microbiol. Spectr. 6:RWR–0009–2017. doi: 10.1128/microbiolspec.rwr-0009-2017
Romeo, T., Gong, M., Liu, M. Y., and Brun-Zinkernagel, A. M. (1993). Identification and molecular characterization of csrA, a pleiotropic gene from Escherichia coli that affects glycogen biosynthesis, gluconeogenesis, cell size, and surface properties. J. Bacteriol. 175, 4744–4755. doi: 10.1128/jb.175.15.4744-4755.1993
Romeo, T., Vakulskas, C. A., and Babitzke, P. (2013). Post-transcriptional regulation on a global scale: form and function of Csr/Rsm systems. Environ. Microbiol. 15, 313–324. doi: 10.1111/j.1462-2920.2012.02794.x
Santiago-Frangos, A., Fröhlich, K. S., Jeliazkov, J. R., Małecka, E. M., Marino, G., Gray, J. J., et al. (2019). Caulobacter crescentus Hfq structure reveals a conserved mechanism of RNA annealing regulation. Proc. Natl. Acad. Sci. U.S.A. 166, 10978–10987. doi: 10.1073/pnas.1814428116
Santiago-Frangos, A., Jeliazkov, J. R., Gray, J. J., and Woodson, S. A. (2017). Acidic C-terminal domains autoregulate the RNA chaperone Hfq. eLife 6:e27049. doi: 10.7554/eLife.27049
Santiago-Frangos, A., and Woodson, S. A. (2018). Hfq chaperone brings speed dating to bacterial sRNA. Wiley Interdiscip. Rev. RNA 9:e1475. doi: 10.1002/wrna.1475
Saramago, M., Bárria, C., Dos Santos, R. F., Silva, I. J., Pobre, V., Domingues, S., et al. (2014). The role of RNases in the regulation of small RNAs. Curr. Opin. Microbiol. 18, 105–115. doi: 10.1016/j.mib.2014.02.009
Saramago, M., Peregrina, A., Robledo, M., Matos, R. G., Hilker, R., Serrania, J., et al. (2017). Sinorhizobium meliloti YbeY is an endoribonuclease with unprecedented catalytic features, acting as silencing enzyme in riboregulation. Nucleic Acids Res. 45, 1371–1391. doi: 10.1093/nar/gkw1234
Sauer, E., Schmidt, S., and Weichenrieder, O. (2012). Small RNA binding to the lateral surface of Hfq hexamers and structural rearrangements upon mRNA target recognition. Proc. Natl. Acad. Sci. U.S.A. 109, 9396–9401. doi: 10.1073/pnas.1202521109
Sauer, E., and Weichenrieder, O. (2011). Structural basis for RNA 3’-end recognition by Hfq. Proc. Natl. Acad. Sci. U.S.A. 108, 13065–13070. doi: 10.1073/pnas.1103420108
Schu, D. J., Zhang, A., Gottesman, S., and Storz, G. (2015). Alternative Hfq-sRNA interaction modes dictate alternative mRNA recognition. EMBO J. 34, 2557–2573. doi: 10.15252/embj.201591569
Schubert, M., Lapouge, K., Duss, O., Oberstrass, F. C., Jelesarov, I., Haas, D., et al. (2007). Molecular basis of messenger RNA recognition by the specific bacterial repressing clamp RsmA/CsrA. Nat. Struct. Mol. Biol. 14, 807–813. doi: 10.1038/nsmb1285
Sesto, N., Touchon, M., Andrade, J. M., Kondo, J., Rocha, E. P. C., Arraiano, C. M., et al. (2014). A PNPase dependent CRISPR System in Listeria. PLoS Genet. 10:e1004065. doi: 10.1371/journal.pgen.1004065
Shi, Z., Yang, W.-Z., Lin-Chao, S., Chak, K.-F., and Yuan, H. S. (2008). Crystal structure of Escherichia coli PNPase: central channel residues are involved in processive RNA degradation. RNA 14, 2361–2371. doi: 10.1261/rna.1244308
Shimoni, Y., Friedlander, G., Hetzroni, G., Niv, G., Altuvia, S., Biham, O., et al. (2007). Regulation of gene expression by small non-coding RNAs: a quantitative view. Mol. Syst. Biol. 3:138. doi: 10.1038/msb4100181
Silva, I. J., Barahona, S., Eyraud, A., Lalaouna, D., Figueroa-Bossi, N., Massé, E., et al. (2019). SraL sRNA interaction regulates the terminator by preventing premature transcription termination of rho mRNA. Proc. Natl. Acad. Sci. U.S.A. 116, 3042–3051. doi: 10.1073/pnas.1811589116
Smirnov, A., Förstner, K. U., Holmqvist, E., Otto, A., Günster, R., Becher, D., et al. (2016). Grad-seq guides the discovery of ProQ as a major small RNA-binding protein. Proc. Natl. Acad. Sci. U.S.A. 113, 11591–11596. doi: 10.1073/pnas.1609981113
Smirnov, A., Schneider, C., Hör, J., and Vogel, J. (2017a). Discovery of new RNA classes and global RNA-binding proteins. Curr. Opin. Microbiol. 39, 152–160. doi: 10.1016/j.mib.2017.11.016
Smirnov, A., Wang, C., Drewry, L. L., and Vogel, J. (2017b). Molecular mechanism of mRNA repression in trans by a ProQ-dependent small RNA. EMBO J. 36, 1029–1045. doi: 10.15252/embj.201696127
Storz, G., Vogel, J., and Wassarman, K. M. (2011). Regulation by small RNAs in bacteria: expanding frontiers. Mol. Cell 43, 880–891. doi: 10.1016/j.molcel.2011.08.022
Szklarczyk, D., Morris, J. H., Cook, H., Kuhn, M., Wyder, S., Simonovic, M., et al. (2017). The STRING database in 2017: quality-controlled protein-protein association networks, made broadly accessible. Nucleic Acids Res. 45, D362–D368. doi: 10.1093/nar/gkw937
Tu, K. C., and Bassler, B. L. (2007). Multiple small RNAs act additively to integrate sensory information and control quorum sensing in Vibrio harveyi. Genes Dev. 21, 221–233. doi: 10.1101/gad.1502407
Udekwu, K. I., Darfeuille, F., Vogel, J., Reimegård, J., Holmqvist, E., and Wagner, E. G. H. (2005). Hfq-dependent regulation of OmpA synthesis is mediated by an antisense RNA. Genes Dev. 19, 2355–2366. doi: 10.1101/gad.354405
Vakulskas, C. A., Leng, Y., Abe, H., Amaki, T., Okayama, A., Babitzke, P., et al. (2016). Antagonistic control of the turnover pathway for the global regulatory sRNA CsrB by the CsrA and CsrD proteins. Nucleic Acids Res. 44, 7896–7910. doi: 10.1093/nar/gkw484
Vakulskas, C. A., Potts, A. H., Babitzke, P., Ahmer, B. M. M., and Romeo, T. (2015). Regulation of Bacterial Virulence by Csr (Rsm) Systems. Microbiol. Mol. Biol. Rev. 79, 193–224. doi: 10.1128/mmbr.00052-14
Vercruysse, M., Köhrer, C., Davies, B. W., Arnold, M. F. F., Mekalanos, J. J., RajBhandary, U. L., et al. (2014). The highly conserved bacterial RNase YbeY is essential in Vibrio cholerae, playing a critical role in virulence, stress regulation, and RNA processing. PLoS Pathog. 10:e1004175. doi: 10.1371/journal.ppat.1004175
Viegas, S. C., Silva, I. J., Saramago, M., Domingues, S., and Arraiano, C. M. (2011). Regulation of the small regulatory RNA MicA by ribonuclease III: a target-dependent pathway. Nucleic Acids Res. 39, 2918–2930. doi: 10.1093/nar/gkq1239
Vogel, J., and Luisi, B. F. (2011). Hfq and its constellation of RNA. Nat. Rev. Microbiol. 9, 578–589. doi: 10.1038/nrmicro2615
Waters, S. A., McAteer, S. P., Kudla, G., Pang, I., Deshpande, N. P., Amos, T. G., et al. (2017). Small RNA interactome of pathogenic E. coli revealed through crosslinking of RNase E. EMBO J. 36, 374–387. doi: 10.15252/embj.201694639
Weilbacher, T., Suzuki, K., Dubey, A. K., Wang, X., Gudapaty, S., Morozov, I., et al. (2003). A novel sRNA component of the carbon storage regulatory system of Escherichia coli. Mol. Microbiol. 48, 657–670. doi: 10.1046/j.1365-2958.2003.03459.x
Westermann, A. J., Venturini, E., Sellin, M. E., Förstner, K. U., Hardt, W.-D., and Vogel, J. (2019). The Major RNA-Binding Protein ProQ Impacts Virulence Gene Expression in Salmonella enterica Serovar Typhimurium. mBio 10:e02504-18. doi: 10.1128/mBio.02504-18
Woodson, S. A., Panja, S., and Santiago-Frangos, A. (2018). Proteins That Chaperone RNA Regulation. Microbiol. Spectr. 6, 385–397. doi: 10.1128/microbiolspec.RWR-0026-2018
Yakhnin, A. V., Baker, C. S., Vakulskas, C. A., Yakhnin, H., Berezin, I., Romeo, T., et al. (2013). CsrA activates flhDC expression by protecting flhDC mRNA from RNase E-mediated cleavage. Mol. Microbiol. 87, 851–866. doi: 10.1111/mmi.12136
Yamanaka, K., Fang, L., and Inouye, M. (1998). The CspA family in Escherichia coli: multiple gene duplication for stress adaptation. Mol. Microbiol. 27, 247–255. doi: 10.1046/j.1365-2958.1998.00683.x
Zhang, A., Derbyshire, V., Galloway Salvo, J. L., and Belfort, M. (1995). Escherichia coli protein StpA stimulates self-splicing by promoting RNA assembly in vitro. RNA 1, 783–793.
Zhang, A., Rimsky, S., Reaban, M. E., Buc, H., and Belfort, M. (1996). Escherichia coli protein analogs StpA and H-NS: regulatory loops, similar and disparate effects on nucleic acid dynamics. EMBO J. 15, 1340–1349. doi: 10.1002/j.1460-2075.1996.tb00476.x
Keywords: RNA-binding proteins, RNA chaperone, ribonucleases, small non-coding RNAs, CsrA, Hfq, ProQ
Citation: Quendera AP, Seixas AF, dos Santos RF, Santos I, Silva JPN, Arraiano CM and Andrade JM (2020) RNA-Binding Proteins Driving the Regulatory Activity of Small Non-coding RNAs in Bacteria. Front. Mol. Biosci. 7:78. doi: 10.3389/fmolb.2020.00078
Received: 20 January 2020; Accepted: 06 April 2020;
Published: 13 May 2020.
Edited by:
Olga N. Ozoline, Institute of Cell Biophysics (RAS), RussiaReviewed by:
Irina Masulis, Institute of Cell Biophysics (RAS), RussiaClaudio Valverde, National University of Quilmes, Argentina
Copyright © 2020 Quendera, Seixas, dos Santos, Santos, Silva, Arraiano and Andrade. This is an open-access article distributed under the terms of the Creative Commons Attribution License (CC BY). The use, distribution or reproduction in other forums is permitted, provided the original author(s) and the copyright owner(s) are credited and that the original publication in this journal is cited, in accordance with accepted academic practice. No use, distribution or reproduction is permitted which does not comply with these terms.
*Correspondence: José M. Andrade, YW5kcmFkZUBpdHFiLnVubC5wdA==