- 1Department of Mathematical and Life Sciences, Graduate School of Science, Hiroshima University, Higashi-Hiroshima, Japan
- 2Graduate School of Integrated Sciences for Life, Hiroshima University, Higashi-Hiroshima, Japan
- 3Research Center for the Mathematics on Chromatin Live Dynamics (RcMcD), Hiroshima University, Higashi-Hiroshima, Japan
- 4RIKEN Center for Biosystems Dynamics Research (BDR), Higashi-Hiroshima, Japan
Nucleosomes are structural units of the chromosome consisting of DNA wrapped around histone proteins, and play important roles in compaction and regulation of the chromatin structure. While the structure and dynamics of canonical nucleosomes have been studied extensively, those of nucleosomes in intermediate states, that occur when their structure or positioning is modulated, have been less understood. In particular, the dynamic features of partially disassembled nucleosomes have not been discussed in previous studies. Using all-atom molecular dynamics simulations, in this study, we investigated the dynamics and stability of nucleosome structures lacking a histone-dimer. DNA in nucleosomes lacking a histone H2A/H2B dimer was drastically deformed due to loss of local interactions between DNA and histones. In contrast, conformation of DNA in nucleosomes lacking H3/H4 was similar to the canonical nucleosome, as the H2A C-terminal domain infiltrated the space originally occupied by the dissociated H3/H4 histones and restricted DNA dynamics in close proximity. Our results suggest that, besides histone chaperones, the intrinsic dynamics of nucleosomes support the exchange of H2A/H2B, which is significantly more frequent than that of H3/H4.
1. Introduction
Eukaryotic genomic DNA is compacted into a hierarchical chromatin structure that is confined within the nucleus. Basic units constituting chromatin are complexes of DNA and histone proteins, called nucleosomes (Davey et al., 2002). A nucleosome consists of approximately 147 base pairs (bps) of DNA and 8 histone proteins, namely H2A, H2B, H3, and H4. In the canonical nucleosome structure, two H2A/H2B and two H3/H4 hetero-dimers form a histone octamer (Davey et al., 2002). The DNA wraps around the histone proteins in the nucleosome, forming a spool-like structure. Amino-acid sequences of these histones are highly conserved among eukaryotes. The nucleosomal structures are stable and are supported by electrostatic interactions between DNA and histone.
Nucleosomes are strongly associated with chromatin structure formation and the genome function. Gene expression involves nucleosomal rearrangement. In turn, changes in nucleosome positioning regulate the accessibility of proteins to DNA and hence modulate gene expression, which is also regulated based largely on cell species and cell cycle (Hogan et al., 2006; Jiang and Pugh, 2009; Bai and Morozov, 2010). Eviction of histones and reconstruction of nucleosomes occur frequently upon rearrangement. These structural changes have been associated with the interaction of nucleosome and histone chaperones (Loyola and Almouzni, 2004; Mazurkiewicz et al., 2006; Formosa, 2008), and often occur upon transcription. FACT (FAcilitates Chromatin Transcription) is a well-known histone chaperone and is highly conserved among eukaryotes (Formosa, 2008; Hondele et al., 2013; Kemble et al., 2015). Recent reports have postulated mechanisms of histone chaperones interacting with nucleosomes (Vlijm et al., 2015; Aguilar-Gurrieri et al., 2016; Tsunaka et al., 2016).
In these processes, nucleosomes may transiently lack some histone proteins (e.g., hexasome, Arimura et al., 2012). For example, during transcription, nucleosomes must at least be repositioned (Kujirai et al., 2018). Consequently, structural conflicts between neighboring nucleosomes may result in partial histone dissociation, manifesting in vitro as overlapping dinucleosomes (Kato et al., 2017). These partially disassembled nucleosomes (intermediate nucleosomes; or “partially assembled nucleosome” coined by Rychkov et al., 2017) have been reported in nucleosome structure modulation, histone assembly and disassembly (Mazurkiewicz et al., 2006; Formosa, 2008; Arimura et al., 2012; Aguilar-Gurrieri et al., 2016). For example, the histone variant H2A.Z is widely distributed in higher eukaryotic genomes and regulates genome dynamics (Fan et al., 2002; Li et al., 2005). H2A.Z is inserted into H2A/H2B-lacking nucleosomes with the help of histone chaperones. In some cases, partially disassembled nucleosomes facilitate binding of proteins to DNA (Kireeva et al., 2002). Such attachment and detachment of histones occur over the entire chromosomes, and partially disassembled nucleosomes may occur upon detachment of any of the constituent histones (Zlatanova et al., 2009). In fact, the dissociation frequency of histone H2A/H2B is significantly higher than that of H3/H4 (Kimura and Cook, 2001), and nucleosomes lacking only H3/H4 have not been experimentally observed. Therefore, most intermediate nucleosomes are considered to be hexasomes lacking H2A/H2B (Arimura et al., 2012).
Considering their importance, the structures of these intermediate nucleosomes have been extensively explored of late by crystallography and cryo-EM methods (Zlatanova et al., 2009; Arimura et al., 2012). However, their structural dynamics have not been fully elucidated. In this research, we focused on the intermediate nucleosome dynamics using molecular dynamics (MD) simulation. We considered nucleosome structures that lack one histone dimer, as well as the canonical nucleosome, and analyzed the differences in their dynamics. Our aim is to investigate the intrinsic dynamics of the intermediate nucleosomes at the configuration close to the intact nucleosome (octasome), not the equilibrium distribution. Hence, we constructed hexasome models by artificially deleting an H2A/H2B dimer from the canonical nucleosome structure. We also studied nucleosome models lacking an H3/H4 dimer for comparison, although hypothetical, to characterize the temporal behavior caused by the loss of histones. We found that deletion of a histone dimer had only little influence on other histones. In contrast, DNA dynamics was drastically affected, based on the eliminated histone type. These results are consistent with previous findings in nucleosome modulation, and suggest the importance of transient dynamics of intermediate nucleosomes in chromatin remodeling.
2. Materials and Methods
2.1. Nucleosome Models and Simulation Procedures
We employed all-atom MD simulations for nucleosome dynamics in solution. We constructed 5 nucleosome models, using the crystal structure of a nucleosome (PDB ID: 1KX5) (Davey et al., 2002). The canonical nucleosome corresponds to the entire crystal structure. The others are models of partially disassembled nucleosomes, constructed by removing one of the two H2A/H2B hetero-dimers or one of the two H3/H4 hetero-dimers from the canonical nucleosome; which we named ΔH3/H4, ΔH2A/H2B, ΔH3'/H4', and ΔH2A'/H2B' (Figure 1; these histones are indexed as chains A to H in the PDB file). Then, these models were soaked into a 153 Å × 197 Å × 103 Å water box, neutralized by K+, and added 150 mM KCl. TIP3P water model was employed. All histidine residues were configured as ϵ-protonated. VMD (Humphrey et al., 1996) was used to infer missing atom coordinates, solvate the model, and visualize the structure throughout the study. Note that the nucleosome structure is viewed from the side of chains A to D (H3, H4, H2A, and H2B) in Figures 1, 4, 5, and from the side of chains E to H (H3', H4', H2A', and H2B') in Figure S3.
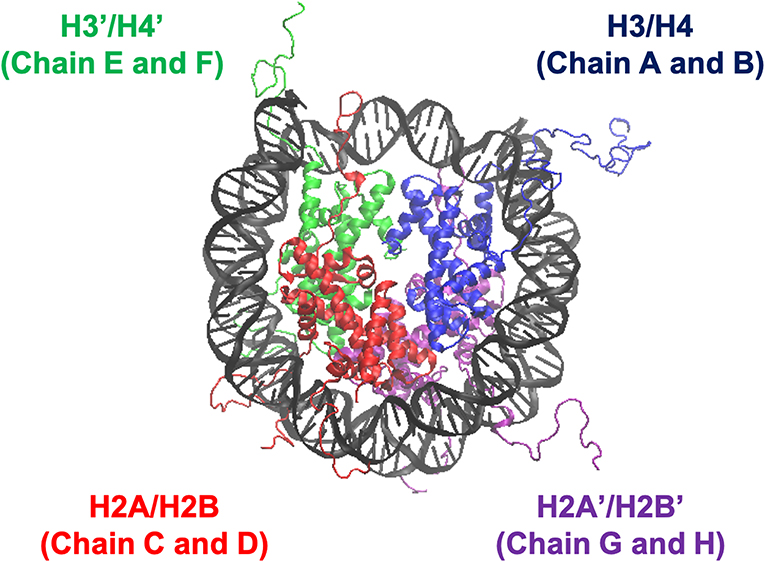
Figure 1. Nucleosome structure and histone indices. PDB ID: 1KX5. Histones H3/H4 (Chain A and B), H2A/H2B (Chain C and D), H3'/H4' (Chain E and F), and H2A'/H2B' (Chain G and H) are shown in blue, red, green, and purple, respectively. Following these indices, we named each nucleosome lacking one of the histone-dimers as ΔH3/H4, ΔH2A/H2B, ΔH3'/H4', and ΔH2A'/H2B'.
All simulations were carried out using NAMD (version 2.12 multi-core with CUDA) (Phillips et al., 2005). CHARMM36 force-field (July 2017 update) was used. Periodic boundary condition with Particle-Mesh Ewald electrostatics was employed. Cutoff 12 Å (with switching from 10 Å) was used for non-bonded interactions. Temperature and pressure were set at 310 K and 1 atm, respectively; Langevin thermostat (damping coefficient: 5/ps) and Langevin-piston barostat were adopted. After energy minimization with harmonic restraints on Cα of amino acids and C1' of nucleotides (spring constant: 100 pN/Å; 10,000 steps), the system was simulated in equilibrium for 100 ns (time-step: 2 fs). The structures were sampled at 10 ps intervals, and each nucleosome model was simulated 10 times.
2.2. Root Mean Square Displacement (RMSD)
The coordinate of atom i is denoted by xi(t). We used heavy atoms except the histone tails (Table 1) for calculation, as these tails are highly disordered (same for RMSF and PCA). First, we aligned the nucleosome by eliminating the translational and rotational motions to minimize RMSD (i), and then calculated RMSD. Additionally, to evaluate the structural changes of DNA, we aligned the nucleosome according to only the core regions of histones (i.e., except DNA) (ii), and calculated RMSD likewise. Below, the coordinates after the alignment in the case of (i) and (ii) are denoted by and , respectively.
2.3. Root Mean Square Fluctuation (RMSF) of DNA
Root mean square fluctuation of nucleotide i was defined as . In this case, is the C1' coordinate of nucleotide i after alignment. Note that 〈·〉time denotes averaging over the latter half of the simulation (50.01–100 ns) and 10 trials for the same nucleosome model.
2.4. Principal Component Analysis (PCA) of DNA
Principal component analysis was employed to analyze histone-wrapped DNA dynamics. PCA is often used in similar cases to evaluate protein dynamics (Balsera et al., 1996). In the current case, each nucleotide was represented by the C1' coordinate. Displacement S(τ, mode): = (X(τ) − 〈X 〉 time) · vmode was evaluated where , and vj is j-th eigenvector of covariance matrix of X. As stated in section 2.3, 〈·〉time denotes averaging over the latter half of the simulation and 10 trials for the same model.
2.5. Secondary Structure Determination
To determine the secondary structures in the histones, we employed the DSSP program (Kabsch and Sander, 1983). In the canonical nucleosome, each histone is composed of only several α-helices (Davey et al., 2002). We determined α-helix formation and the occurrence at each residue was calculated for the latter half of the simulation trajectory.
2.6. Contact Frequency of Nucleotides and Amino Acids
Contact frequency between DNA and histone tails were evaluated as follows. The distance between C1' of each nucleotide and Cα of each amino acid was examined. The molecules were regarded in contact if the distance was less than 10 Å. The contact frequency, defined as the ratio of samples in which the pair was in contact, was averaged over the latter half of the simulation and 10 trials for each nucleosome model.
3. Results
3.1. Structures of Histone Proteins
First, we analyzed the effects of partial histone deletion on the secondary structure of other histones. The occurrence ratio of α-helix formation is shown in Figure 2 and Figure S1. Each nucleosome lacking one histone dimer shows a profile of secondary structure formation similar to the canonical nucleosome. Removal of a histone dimer has little influence on the secondary structure of other remaining histone proteins. On the other hand, α-helix formation was occasionally observed in histone tail residues; which agrees with previous reports suggesting that histone-tail regions show transient α-helix formation when interacting with DNA (Wang et al., 2000; Moriwaki et al., 2016).
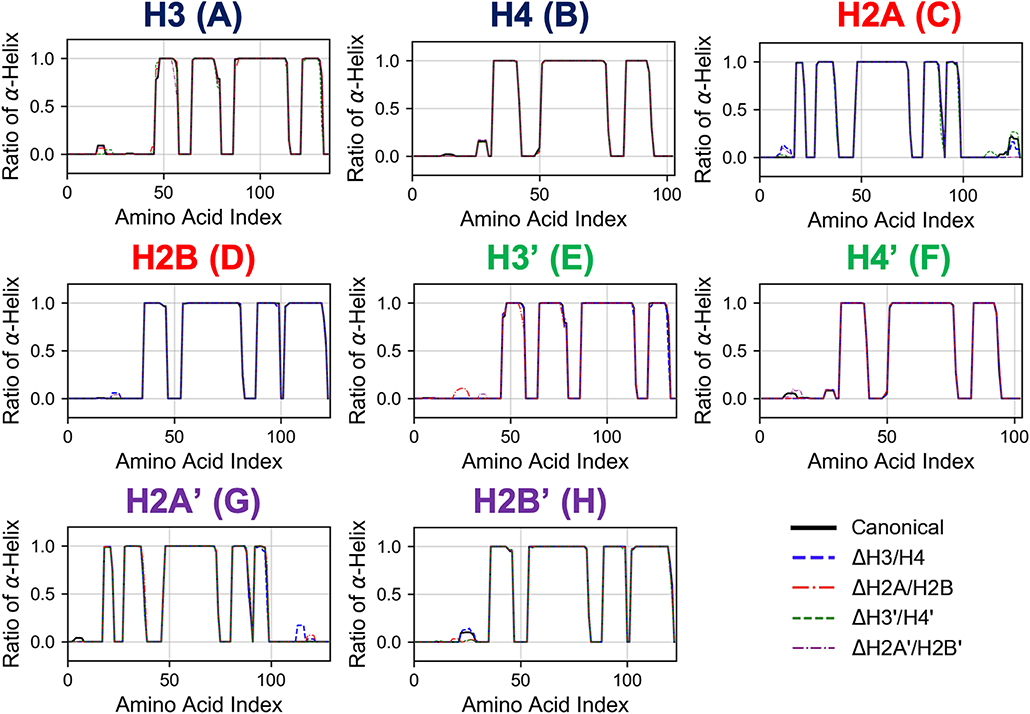
Figure 2. Secondary structure stability of histones in each nucleosome. Averaged over 10 trials (each trial is shown in Figure S1). Horizontal and vertical axes of each graph show amino acid index and ratio of α-helix formation in respective histones as indicated in figure.
Next, we evaluated the time series of RMSD of histones (except the histone tails; see section 2.2) in each nucleosome, to analyze their tertiary structures (Figure 3 and Figure S2). In this case, RMSD was defined as , where 〈〉atom means averaging over the constituent heavy atoms. Similar to the results of secondary structure analysis, each histone displayed similar profiles across all nucleosome models. Again, the absence of a histone dimer induced negligible effect on the other histones, which is consistent also with experimental observations (Arimura et al., 2012; Bilokapic et al., 2018).
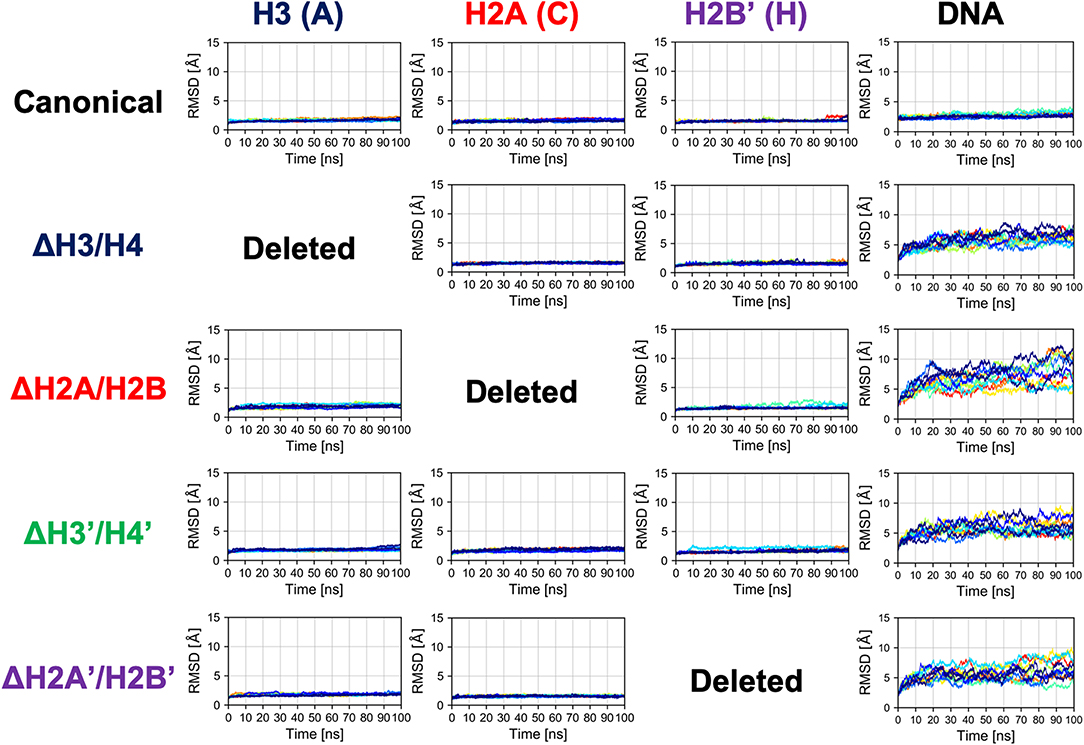
Figure 3. Time series of RMSD of histone and DNA in each nucleosome. Colors show different simulation trajectories (total 10).
3.2. Structural Deformation of Nucleosomal DNA
In contrast, as shown in Figure 3, dynamics of nucleosomal DNA was dramatically affected. RMSD of DNA in nucleosomes lacking any histone dimer was increased, compared to the canonical nucleosome; i.e., DNA was deformed from the native structure by the partial disassembly.
Then, we evaluated the deformation in each part of DNA, to determine which part was affected by the loss of histones. RMSF profiles of DNA are shown in Figure 4. Fluctuations were increased only in nucleotides adjacent to the removed histone. Consistently, drastic deformations (superior modes of PCA) were observed in the region that lost interactions with histones (Figure 5 and Figure S3). These deformations mostly correspond to the breathing motion of DNA outward from the nucleosome.
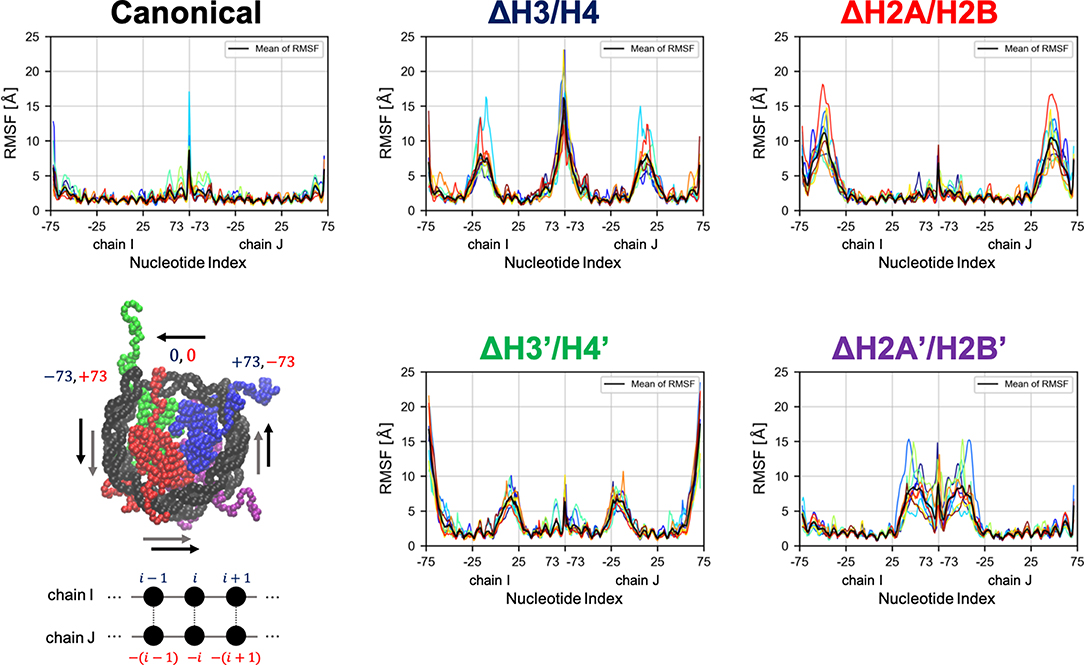
Figure 4. Root mean square fluctuation (RMSF) of DNA. Each graph shows RMSF profiles of respective nucleosomal DNA. Horizontal axis shows nucleotide indices i. Colors show different simulation trajectories (total 10). The nucleotide indices are indicated in the bottom-left panel (each particle represents a nucleotide or an amino acid); the indices in chains I and J (−73 to 73 each in the PDB file) are shown in blue and red, respectively.
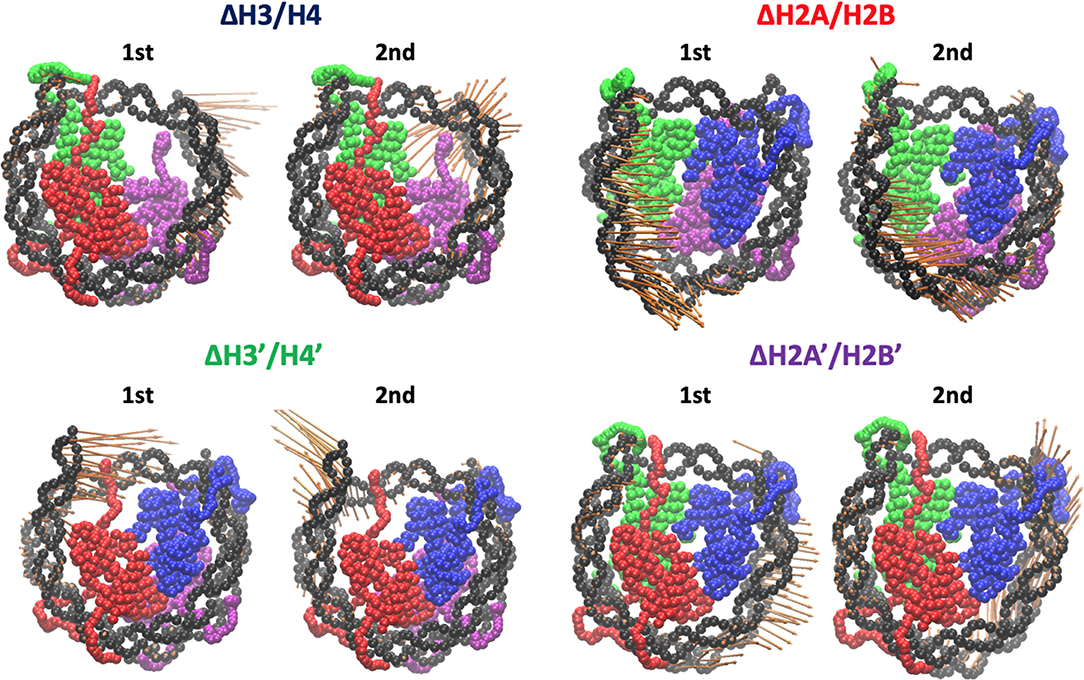
Figure 5. Effective directions of DNA deformation (front view). Visualized from the side of chains A–D (H3, H4, H2A, and H2B). Nucleotide (C1' of each nucleotide) and amino acid (Cα of each amino acid) coordinates are shown by spheres. Orange arrows show the vectors of the 1st and 2nd PC modes. Arrow lengths indicate the norm of the vector.
To capture effective structural changes of DNA, we estimated free energy profiles projected to principal modes obtained from PCA analysis (see section 2.4). We also focused on trajectories projected onto this space, to evaluate DNA dynamics (Figure 6).
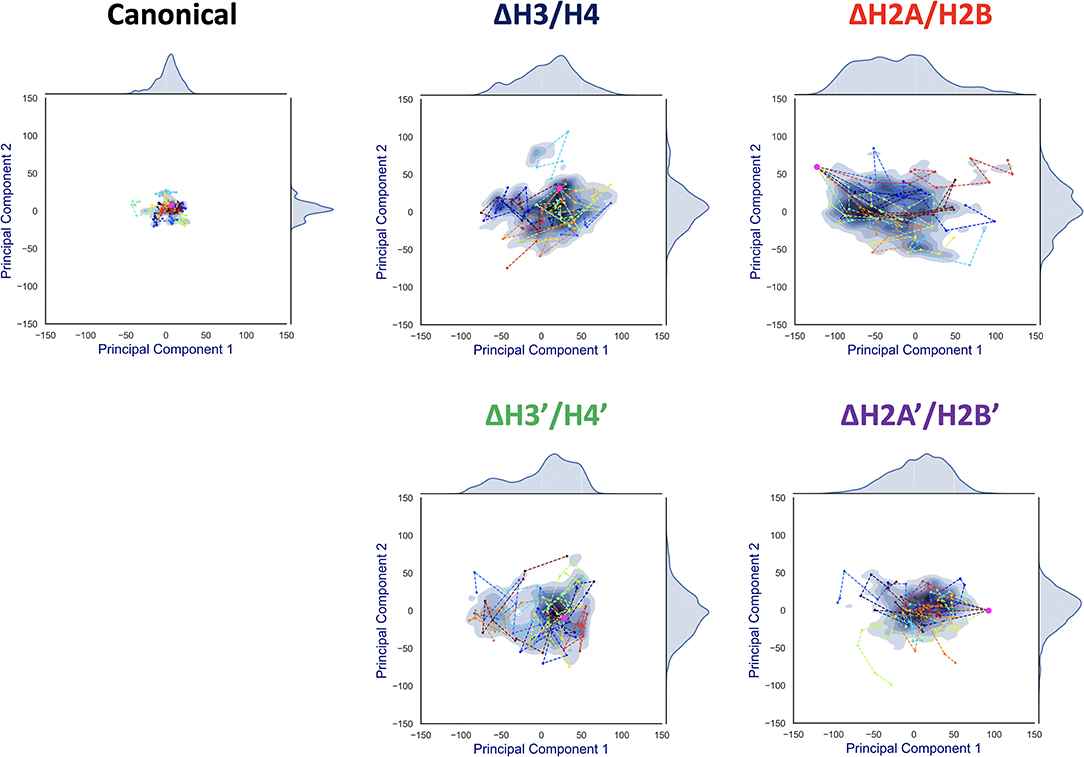
Figure 6. Free energy landscape of DNA dynamics to 1st and 2nd PC modes. The axes correspond to deviations (S(t, mode)) to the 1st and 2nd PC vector directions from the averaged structure (= S(t, 1) or S(t, 2)). The color (blue) shows the probability density of S. Each profile was fitted by kernel density estimation (KDE). Magenta dot corresponds to the initial conformation (S(0, 1), S(0, 2)), and each line (in different colors) shows a simulation trajectory (total 10; plotted at 10 ns intervals).
Contrasting results depending on the removed histone dimer were obtained in the PCA. In the case of nucleosomes lacking H2A/H2B (ΔH2A/H2B and ΔH2A'/H2B'), the initial structure (magenta point) was distant from the area with high probability density, and each simulation trajectory drifted from the initial structure (Figure 6). This suggested that nucleosomes lacking H2A/H2B could not maintain their canonical histone-wrapped conformation, and deformed drastically. In contrast, in the case of H3/H4 lacking nucleosomes (ΔH3/H4 and ΔH3'/H4'), the initial coordinate (magenta point) locates around the high probability density area, and the trajectories were distributed around the initial coordinate (Figure 6). While the loss of H3/H4 affects the nucleosome structure stability (Figure 3), conformational changes often occur only around the initial structure.
3.3. Interactions Between Histone Tails and DNA
We considered that the difference in the free energy profiles of DNA deformation was induced by physical restraints on DNA from histone proteins, and thus focused on physical interactions between DNA and histones. However, as shown in Figure 3, structures of core histones changed only slightly. We also confirmed that the interaction between DNA and core histones did not show a clear difference. Hence, we evaluated contact frequency between the DNA and histone tails (Figure S4). It is to be noted that the histone tails were not counted in the analysis of RMSD, RMSF, and PCA above (see section 2).
Comparing the contact matrices of the canonical nucleosome and others, no significant changes were observed in the majority of components. It is consistent with a previous study by MD simulations showing that histone tails were trapped by the adjacent DNA (Li and Kono, 2016). However, in the nucleosomes lacking H3/H4 (ΔH3/H4 and ΔH3'/H4'), H2A C-terminal region adjacent to the dissociated H3/H4 showed enhanced contact with DNA (Blue rectangles in Figure S4), which were not observed in the nucleosomes lacking H2A/H2B. Therefore, we concluded that the difference of free energy profiles (Figure 6) were closely related to these histone tails.
Increase of contact frequency of nucleosomes lacking H3/H4 corresponds to the invasion of H2A C-terminal region to internal space originally occupied by the removed H3/H4 (Figure 7). The H2A C-terminal interacts with the H3/H4 in the canonical nucleosome (Davey et al., 2002), and the contact frequency between the H2A C-terminal and DNA was increased by the invasion upon loss of H3/H4. These additional interactions restrict DNA deformation and breathing motion, and consequently the histone-wrapping DNA conformation was retained (Figure 6). In contrast, in the nucleosomes lacking H2A/H2B, DNA neighboring the removed histone dimer shows drastic deformations (Figure 7). The behavior of the H3/H4 tails is similar to previous study (Kono et al., 2015), and not altered by the loss of H2A/H2B.
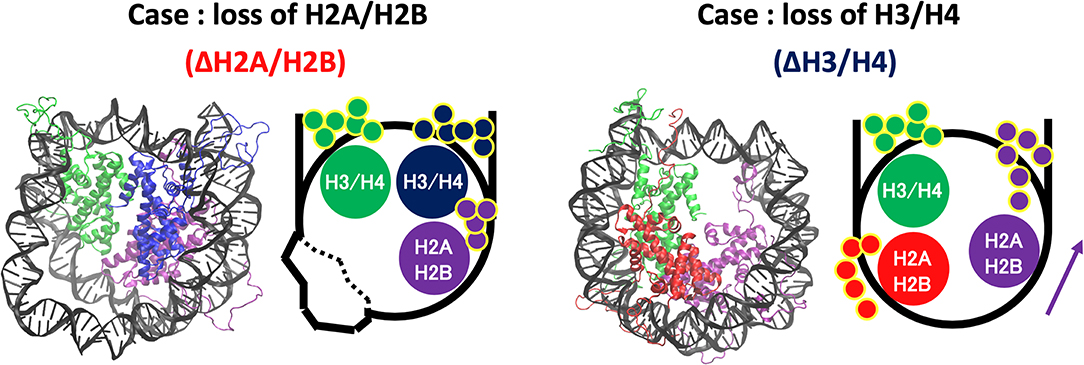
Figure 7. Histone tail dynamics in ΔH3/H4 and ΔH2A/H2B. Snapshots from simulation trajectories and schematics of ΔH3/H4 and ΔH2A/H2B are shown. Histone tails are represented by small circles in the schematics.
4. Discussion
In the partially disassembled nucleosomes, the core histones showed no significant deformation. Indeed, RMSD of the hexasome (ΔH2A/H2B and ΔH2A'/H2B'), which was also experimentally observed, was very small (Figure 3). We could not find any clear difference between the hexasome part in the overlapping di-nucleosome structure obtained by experiment (PDB ID: 5GSE) (Kato et al., 2017), and the nucleosome lacking H2A/H2B constructed from the canonical nucleosome structure (PDB ID: 1KX5). RMSD between them is 2.554 Å. Therefore, in partially disassembled nucleosomes, drastic structural deformation occurs only in the DNA, while histone structures are similar to the canonical crystal structure during attachment and detachment of histones. Note that unlike coarse-grained models depending on a reference structure, the force-field used in the all-atom simulations does not depend on a specific reference conformation.
On the other hand, DNA dynamics of nucleosomes lacking any histone dimer were statistically different depending on dissociated histones (Figure 6). Individual histones did not show obvious structural deformation (Figure 3). When an H2A/H2B was removed (ΔH2A/H2B and ΔH2A'/H2B'), N-terminal long tails of H3 were trapped around the dyad part and the ends of nucleosomal DNA (Figure 7). In previous MD studies, it was shown that histone tails keep the DNA from being peeled off in the canonical nucleosome (Biswas et al., 2011; Ettig et al., 2011; Voltz et al., 2012; Li and Kono, 2016). Particularly, the role of N-terminal tail of H3 in the stabilization was discussed. Our result is consistent with these studies. Due to the H3 tail trapping, free space induced by histone deletion was not filled, and DNA adjacent to the space was highly variable (Figure 7). Similar DNA-histone interactions may exist after the H2A/H2B release induced by spontaneous breathing and partial unwrapping (Bilokapic et al., 2018; Gansen et al., 2018; Winogradoff and Aksimentiev, 2019), which may play a role in further nucleosome deformation and histone exchange. Furthermore, asymmetric unwrapping of nucleosomal DNA was suggested by previous studies (Chen et al., 2014, 2017). Although our simulation without H2A/H2B corresponds only to a short time, our finding might be related to those results (Chen et al., 2014, 2017; Bilokapic et al., 2018; Gansen et al., 2018; Winogradoff and Aksimentiev, 2019). The DNA fluctuations observed in our study may help histone chaperones to intrude between DNA and histones, and hence may be relevant to chaperone-assisted disassembly and reassembly. Single-molecule experiments using e.g., Förster resonance energy transfer (FRET) (Gansen et al., 2009, 2018) would be effective for verification of such changes in DNA dynamics.
Note that our aim is to consider the intrinsic and transient dynamics around the intact octasome configuration. The hexasome structure may show further transitions to more stable conformations, e.g., with more tightly (~110 bp) wrapped DNA as previously suggested (Arimura et al., 2012; Rychkov et al., 2017) by sliding of DNA. It is however expected to be beyond the time-scale of our simulation; although large breathing motion of DNA observed in our study is analogous to the broad distribution of the inter-dye distance in FRET (Gansen et al., 2009; Rychkov et al., 2017), they are not directly comparable.
In contrast, when an H3/H4 was removed (ΔH3/H4 and ΔH3'/H4'), negligible deviation from the initial structure was observed (Figure 6). DNA that originally interacted with the H3/H4 in turn interacted with the H2A C-terminal region adjacent to the H3/H4, which restricted the DNA dynamics by electrostatic interaction (Figure 7). Due to the invasion and transient interaction of the H2A C-terminal, the nucleosome showed conformation relatively close to the canonical nucleosome.
From the results above, the following scenario for nucleosome remodeling was suggested. The lack of H2A/H2B induces only DNA deformation. Then, reinsertion of H2A/H2B into such a nucleosome would be relatively easy, as DNA may become more accessible because of its structural flexibility (Figure S5). In contrast, without H3/H4, additional interaction between DNA and H2A C-terminal tail is induced (Figure 7). This interaction prevents DNA deformation, and keeps the structure similar to the canonical one, which makes reinsertion difficult due to the structural restriction of DNA. For reversible attachment and detachment of histone proteins, H2A/H2B has a dynamic advantage. In fact, nucleosomal structure modulation by histone chaperones has been experimentally observed only for the case of H2A/H2B dissociation. Such selectivity may arise from the physical property. It may also be relevant to localization of histone variants, as the exchange (i.e., attachment and detachment) of H2A/H2B seems relatively easy. Although it requires further investigation, the observed nucleosome dynamics may relate to the selectivity of histones and acquisition of biochemical functions.
5. Conclusion
We analyzed dynamical behavior of partially disassembled nucleosomes using MD simulations. We observed that removal of an H2A/H2B dimer from the canonical nucleosome induces large fluctuations of nucleosomal DNA, while keeping the space for the histone dimer unoccupied. In contrast, when an H3/H4 was removed, the space for the dimer was invaded by the C-terminal tail of H2A, which mitigated the DNA fluctuations. The observed behavior may support frequent exchange of H2A/H2B, as well as specific molecular mechanisms such as histone chaperones.
Data Availability Statement
The datasets generated for this study can be found in the Zenodo repository doi: 10.5281/zenodo.2548539.
Author Contributions
TK, AA, and YT conceived and designed the research, analyzed the data, and wrote the paper. TK and YT performed the simulations.
Funding
This work was supported by JSPS KAKENHI Grant Numbers JP17K05614 (to AA), JP16H01408, JP18H04720, and by JSPS and NRF under the Japan-Korea Basic Scientific Cooperation Program.
Conflict of Interest
The authors declare that the research was conducted in the absence of any commercial or financial relationships that could be construed as a potential conflict of interest.
Acknowledgments
The authors are grateful to K. Saikusa, R. Kawasaki, N. Sakamoto, and H. Nishimori for fruitful discussions.
Supplementary Material
The Supplementary Material for this article can be found online at: https://www.frontiersin.org/articles/10.3389/fmolb.2019.00133/full#supplementary-material
References
Aguilar-Gurrieri, C., Larabi, A., Vinayachandran, V., Patel, N. A., Yen, K., Reja, R., et al. (2016). Structural evidence for Nap1-dependent H2A-H2B deposition and nucleosome assembly. EMBO J. 35, 1465–1482. doi: 10.15252/embj.201694105
Arimura, Y., Tachiwana, H., Oda, T., Sato, M., and Kurumizaka, H. (2012). Structural analysis of the hexasome, lacking one histone H2A/H2B dimer from the conventional nucleosome. Biochemistry 51, 3302–3309. doi: 10.1021/bi300129b
Bai, L., and Morozov, A. V. (2010). Gene regulation by nucleosome positioning. Trends Genet. 26, 476–483. doi: 10.1016/j.tig.2010.08.003
Balsera, M. A., Wriggers, W., Oono, Y., and Schulten, K. (1996). Principal component analysis and long time protein dynamics. J. Phys. Chem. 100, 2567–2572. doi: 10.1021/jp9536920
Bilokapic, S., Strauss, M., and Halic, M. (2018). Histone octamer rearranges to adapt to DNA unwrapping. Nat. Struct. Mol. Biol. 25:101. doi: 10.1038/s41594-017-0005-5
Biswas, M., Voltz, K., Smith, J. C., and Langowski, J. (2011). Role of histone tails in structural stability of the nucleosome. PLoS Comput. Biol. 7:e1002279. doi: 10.1371/journal.pcbi.1002279
Chen, Y., Tokuda, J. M., Topping, T., Meisburger, S. P., Pabit, S. A., Gloss, L. M., et al. (2017). Asymmetric unwrapping of nucleosomal DNA propagates asymmetric opening and dissociation of the histone core. Proc. Natl. Acad. Sci. U.S.A. 114, 334–339. doi: 10.1073/pnas.1611118114
Chen, Y., Tokuda, J. M., Topping, T., Sutton, J. L., Meisburger, S. P., Pabit, S. A., et al. (2014). Revealing transient structures of nucleosomes as DNA unwinds. Nucl. Acids Res. 42, 8767–8776. doi: 10.1093/nar/gku562
Davey, C. A., Sargent, D. F., Luger, K., Maeder, A. W., and Richmond, T. J. (2002). Solvent mediated interactions in the structure of the nucleosome core particle at 1.9 Å resolution. J. Mol. Biol. 319, 1097–1113. doi: 10.1016/S0022-2836(02)00386-8
Ettig, R., Kepper, N., Stehr, R., Wedemann, G., and Rippe, K. (2011). Dissecting DNA-histone interactions in the nucleosome by molecular dynamics simulations of DNA unwrapping. Biophys. J. 101, 1999–2008. doi: 10.1016/j.bpj.2011.07.057
Fan, J. Y., Gordon, F., Luger, K., Hansen, J. C., and Tremethick, D. J. (2002). The essential histone variant H2A.Z regulates the equilibrium between different chromatin conformational states. Nat. Struct. Biol. 9:172. doi: 10.1038/nsb767
Formosa, T. (2008). FACT and the reorganized nucleosome. Mol. Biosyst. 4, 1085–1093. doi: 10.1039/b812136b
Gansen, A., Felekyan, S., Kühnemuth, R., Lehmann, K., Tóth, K., Seidel, C. A. M., et al. (2018). High precision FRET studies reveal reversible transitions in nucleosomes between microseconds and minutes. Nat. Commun. 9:4628. doi: 10.1038/s41467-018-06758-1
Gansen, A., Valeri, A., Hauger, F., Felekyan, S., Kalinin, S., Tóth, K., et al. (2009). Nucleosome disassembly intermediates characterized by single-molecule FRET. Proc. Natl. Acad. Sci. U.S.A. 106, 15308–15313. doi: 10.1073/pnas.0903005106
Hogan, G. J., Lee, C.-K., and Lieb, J. D. (2006). Cell cycle-specified fluctuation of nucleosome occupancy at gene promoters. PLoS Genet. 2:e158. doi: 10.1371/journal.pgen.0020158
Hondele, M., Stuwe, T., Hassler, M., Halbach, F., Bowman, A., Zhang, E. T., et al. (2013). Structural basis of histone H2A-H2B recognition by the essential chaperone FACT. Nature 499:111. doi: 10.1038/nature12242
Humphrey, W., Dalke, A., and Schulten, K. (1996). VMD: visual molecular dynamics. J. Mol. Graph. 14, 33–38. doi: 10.1016/0263-7855(96)00018-5
Jiang, C., and Pugh, B. F. (2009). Nucleosome positioning and gene regulation: advances through genomics. Nat. Rev. Genet. 10:161. doi: 10.1038/nrg2522
Kabsch, W., and Sander, C. (1983). Dictionary of protein secondary structure: pattern recognition of hydrogen-bonded and geometrical features. Biopolymers 22, 2577–2637. doi: 10.1002/bip.360221211
Kato, D., Osakabe, A., Arimura, Y., Mizukami, Y., Horikoshi, N., Saikusa, K., et al. (2017). Crystal structure of the overlapping dinucleosome composed of hexasome and octasome. Science 356, 205–208. doi: 10.1126/science.aak9867
Kemble, D. J., McCullough, L. L., Whitby, F. G., Formosa, T., and Hill, C. P. (2015). FACT disrupts nucleosome structure by binding H2A-H2B with conserved peptide motifs. Mol. Cell 60, 294–306. doi: 10.1016/j.molcel.2015.09.008
Kimura, H., and Cook, P. R. (2001). Kinetics of core histones in living human cells: little exchange of H3 and H4 and some rapid exchange of H2B. J. Cell Biol. 153, 1341–1354. doi: 10.1083/jcb.153.7.1341
Kireeva, M. L., Walter, W., Tchernajenko, V., Bondarenko, V., Kashlev, M., and Studitsky, V. M. (2002). Nucleosome remodeling induced by RNA polymerase II: loss of the H2A/H2B dimer during transcription. Mol. Cell 9, 541–552. doi: 10.1016/S1097-2765(02)00472-0
Kono, H., Shirayama, K., Arimura, Y., Tachiwana, H., and Kurumizaka, H. (2015). Two arginine residues suppress the flexibility of nucleosomal DNA in the canonical nucleosome core. PLoS ONE 10:e0120635. doi: 10.1371/journal.pone.0120635
Kujirai, T., Ehara, H., Fujino, Y., Shirouzu, M., Sekine, S.-I., and Kurumizaka, H. (2018). Structural basis of the nucleosome transition during RNA polymerase II passage. Science 362, 595–598. doi: 10.1126/science.aau9904
Li, B., Pattenden, S. G., Lee, D., Gutiérrez, J., Chen, J., Seidel, C., et al. (2005). Preferential occupancy of histone variant H2AZ at inactive promoters influences local histone modifications and chromatin remodeling. Proc. Natl. Acad. Sci. U.S.A. 102, 18385–18390. doi: 10.1073/pnas.0507975102
Li, Z., and Kono, H. (2016). Distinct roles of histone H3 and H2A tails in nucleosome stability. Sci. Rep. 6:31437. doi: 10.1038/srep31437
Loyola, A., and Almouzni, G. (2004). Histone chaperones, a supporting role in the limelight. Biochim. Biophys. Acta 1677, 3–11. doi: 10.1016/j.bbaexp.2003.09.012
Mazurkiewicz, J., Kepert, J. F., and Rippe, K. (2006). On the mechanism of nucleosome assembly by histone chaperone NAP1. J. Biol. Chem. 281, 16462–16472. doi: 10.1074/jbc.M511619200
Moriwaki, Y., Yamane, T., Ohtomo, H., Ikeguchi, M., Kurita, J.-I., Sato, M., et al. (2016). Solution structure of the isolated histone H2A-H2B heterodimer. Sci. Rep. 6:24999. doi: 10.1038/srep24999
Phillips, J. C., Braun, R., Wang, W., Gumbart, J., Tajkhorshid, E., Villa, E., et al. (2005). Scalable molecular dynamics with NAMD. J. Comput. Chem. 26, 1781–1802. doi: 10.1002/jcc.20289
Rychkov, G. N., Ilatovskiy, A. V., Nazarov, I. B., Shvetsov, A. V., Lebedev, D. V., Konev, A. Y., et al. (2017). Partially assembled nucleosome structures at atomic detail. Biophys. J. 112, 460–472. doi: 10.1016/j.bpj.2016.10.041
Tsunaka, Y., Fujiwara, Y., Oyama, T., Hirose, S., and Morikawa, K. (2016). Integrated molecular mechanism directing nucleosome reorganization by human FACT. Genes Dev. 30, 673–686. doi: 10.1101/gad.274183.115
Vlijm, R., Lee, M., Lipfert, J., Lusser, A., Dekker, C., and Dekker, N. H. (2015). Nucleosome assembly dynamics involve spontaneous fluctuations in the handedness of tetrasomes. Cell Rep. 10, 216–225. doi: 10.1016/j.celrep.2014.12.022
Voltz, K., Trylska, J., Calimet, N., Smith, J. C., and Langowski, J. (2012). Unwrapping of nucleosomal DNA ends: a multiscale molecular dynamics study. Biophys. J. 102, 849–858. doi: 10.1016/j.bpj.2011.11.4028
Wang, X., Moore, S. C., Laszckzak, M., and Ausió, J. (2000). Acetylation increases the α-helical content of the histone tails of the nucleosome. J. Biol. Chem. 275, 35013–35020. doi: 10.1074/jbc.M004998200
Winogradoff, D., and Aksimentiev, A. (2019). Molecular mechanism of spontaneous nucleosome unraveling. J. Mol. Biol. 431, 323–335. doi: 10.1016/j.jmb.2018.11.013
Keywords: nucleosome, DNA, histone, chromatin, structural dynamics, hexasome, intermediate nucleosome, molecular dynamics simulation
Citation: Kameda T, Awazu A and Togashi Y (2019) Histone Tail Dynamics in Partially Disassembled Nucleosomes During Chromatin Remodeling. Front. Mol. Biosci. 6:133. doi: 10.3389/fmolb.2019.00133
Received: 31 July 2019; Accepted: 11 November 2019;
Published: 28 November 2019.
Edited by:
Andrzej Stasiak, Université de Lausanne, SwitzerlandReviewed by:
Wataru Kagawa, Meisei University, JapanLars Nordenskiöld, Nanyang Technological University, Singapore
Samuel Bowerman, University of Colorado Boulder, United States
Copyright © 2019 Kameda, Awazu and Togashi. This is an open-access article distributed under the terms of the Creative Commons Attribution License (CC BY). The use, distribution or reproduction in other forums is permitted, provided the original author(s) and the copyright owner(s) are credited and that the original publication in this journal is cited, in accordance with accepted academic practice. No use, distribution or reproduction is permitted which does not comply with these terms.
*Correspondence: Yuichi Togashi, dG9nYXNoaUBoaXJvc2hpbWEtdS5hYy5qcA==