- 1Laboratory of Applied Virology, Department of Microbiology, Immunology and Parasitology, Federal University of Santa Catarina, Florianópolis, Brazil
- 2Department of Chemical and Food Engineering, Federal University of Santa Catarina, Florianópolis, Brazil
- 3Laboratory of Microbiology and Bioprocesses, Federal University of Fronteira Sul (UFFS), Erechim, Brazil
- 4Contested University (PMPECSA), Concórdia, Brazil
- 5Laboratory of Environmental Virology, Environmental Research Division, Department of Water Pollution Research, National Research Centre, Giza, Egypt
- 6Division of Microbiology, Department of Biotechnology and Food Science, Universidad de Burgos, Burgos, Spain
- 7Centre for Emerging Pathogens and Global Health, Universidad de Burgos, Burgos, Spain
Bacteriophages are bacterial-specific viruses and the most abundant biological form on Earth. Each bacterial species possesses one or multiple bacteriophages and the specificity of infection makes them a promising alternative for bacterial control and environmental safety, as a biotechnological tool against pathogenic bacteria, including those resistant to antibiotics. This application can be either directly into foods and food-related environments as biocontrol agents of biofilm formation. In addition, bacteriophages are used for microbial source-tracking and as fecal indicators. The present review will focus on the uses of bacteriophages like bacterial control tools, environmental safety indicators as well as on their contribution to bacterial control in human, animal, and environmental health.
Introduction
Bacteriophages, also known as phages, are prokaryotes viruses, being the most abundant life form, present in all environments and the predominant entities in the sea (Boehme, 1993; Suttle, 2005). Several studies have demonstrated a 1:5 relative abundance between bacteria and bacteriophage (Fuhrman, 1999; Balter, 2000; Rohwer, 2003). They were discovered independently by Twort (1915), who isolated them from Staphylococcus spp., and from patients with dysentery. D’Herelle (1926) described bacteriophage as a virus that has the capability to parasitize bacteria (Twort, 1915; Delbruck, 1942). Bacteriophages vary greatly in morphology and replicative characteristics, containing either RNA or DNA, being these parameters currently used by the International Committee on Taxonomy of Viruses (ICTV) for bacteriophage classification (King et al., 2012; Table 1). However, the identification of bacteriophages is difficult since there are no universally conserved markers, unlike e.g., the bacterial 16S rRNA gene (Paul et al., 2002), with only minor parts of bacteriophage genomes being used to determine family specific makers, such as the viral capsid g20 of T4 (Fuller et al., 1998; Marston and Sallee, 2003; Sullivan et al., 2008).
Bacteriophages can present different life cycles: lytic, lysogenic, and chronic (Figure 1A). Lytic bactériophages, such as T4 and MS2, insert their genetic material inside the bacteria, forcing the cell to produce a large amount of bacteriophage copies. After replication the membrane is then ruptured, releasing the new bacteriophages. Lysogenic bacteriophages (such as T1) possesses an alternative sub-cycle, in which the virus may integrate its DNA in the bacterial genome, becoming non-infectious and replicating together with the bacterial chromosome; the bacteriophage then becomes a prophage, producing new bacteriophage particles under appropriate conditions. Finally, chronic bacteriophages (such as M13) preserve their genome in the bacterial cell, in which the release from the host occurs gradually with less damage to the cell, preserving it longer (Clokie et al., 2011; Cann, 2016; Janczuk et al., 2016). There is an intimate relation between bacteriophages and bacterial cell functions acquisition (Forterre, 1999; Filée et al., 2002, 2003). Bacteriophages can serve as points for genomic rearrangements due to their mosaic nature, with lysogenic bacteriophages even protecting bacteria from lytic infection in certain conditions (Brüssow et al., 2004; Tree et al., 2014; Penadés et al., 2015). While bacterial hosts can benefit from the presence of bacteriophages (as they can express important regulators for adaptation to specific niches by the addition of bacteriophage genes in the cell’s genome) bacteriophages can be involved in the transfer of virulence genes, producing proteins participating in invasion, immune evasion, and toxins related to toxin-mediated diseases (Brüssow et al., 2004; Boyd, 2012; Tree et al., 2014; Penadés et al., 2015).
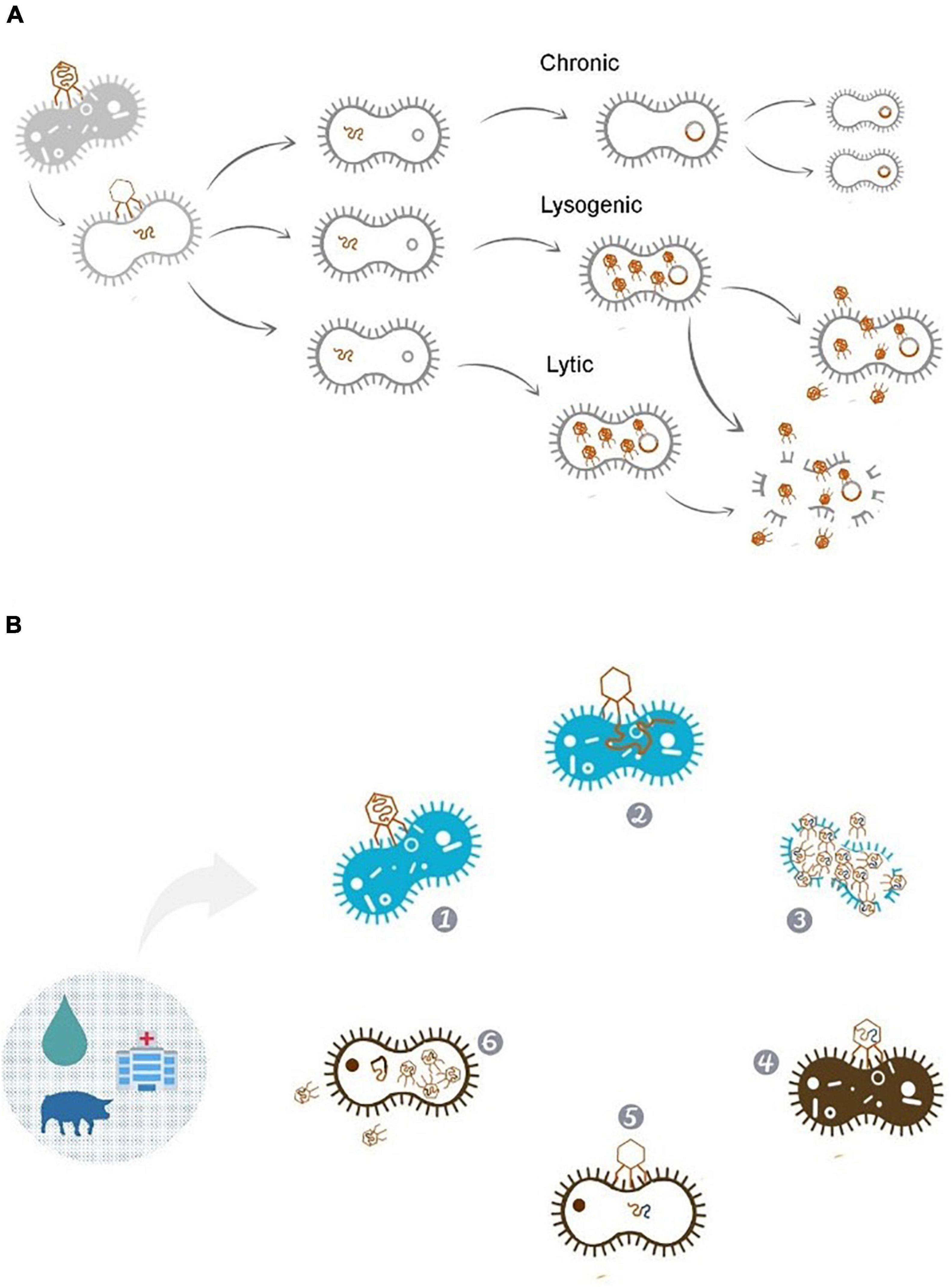
Figure 1. (A) Bacteriophage life cycle. Bacteriophages can replicate by lytic, lysogenic or remain in a chronic state. In the lytic cycle of replication the viruses are released from the host after completing their replication. Lysogenic cycle involves the inclusion of genetic material into the genome of host cells, this way phages can contribute to the transferring of tetracycline resistance plasmids to bacterial cells (Pratama and Van Elsas, 2017). This is evidenced considering bacteriophages genomes, which can represent up to 13 and 5% of the Escherichia coli O157:H7 and Salmonella Newport genomes, respectively (Bobay et al., 2013). (B) Phage’s journey through different cell lines in different environments: (1) Bacteriophage adhering to the resistant bacterial host. (2) Insertion of the phage genetic material. (3) New phages carrying resistance genes derived from the infected bacteria, being released into the environment. (4) Adhesion of the new phage on non-resistant bacteria. (5) Passage of resistance genes inserted into the phages genome to the bacteria. (6) After the genes insertion, the bacteria can enjoy the resistance genes acquired while new bacteriophages can be released keeping the journey of resistance genes on the environment.
The specificity of bacteriophage infection allows their application in several areas such as biotechnology, ecology, health and environment (bacterial control), and as environmental monitoring agents (Armon and Kott, 1996; Leclerc et al., 2000; Arredondo-Hernandez et al., 2017; McMinn et al., 2017; Friedman and Lai, 2018; Vandamme and Mortelmans, 2019).
In this review, a vast amount of scientific literature has been reviewed on the application of phage-based products, discussing the benefits and limitations of the use of bacteriophages as bacterial control tools in the health, food, and environmental fields.
Bacteriophages Application
Fighting Bacterial Infections
Bacterial infections are a major public health concern worldwide, representing an enormous economical and medical burden with a fatal outcome in a significant proportion of those affected. Dysentery caused by Shigella spp., Salmonella spp., Proteus spp. Staphylococcus, Escherichia coli, and Pseudomonas, usually associated with contamination of food and water, is a serious health problem affecting millions of people annually in the world, with shigellosis, a disease caused by Shigella spp., resulting in approximately 600,000 deaths each year (Walker et al., 1990; World Health Organization [WHO], 2017).
Lytic bacteriophages are the main tools for phage therapy, for their capacity to invade the bacterial cell and kill it. Lysogenic bacteriophages could also have an application, the transduction mechanisms could allow the use of bacteriophages as genetic tools to increase bacterial susceptibility to antibiotics; however, this approach has not been widely studied (Lu and Collins, 2009; Edgar et al., 2012). The use of a bacteriophage cocktail for the treatment and prophylaxis of intestinal infections caused by Shigella resulted in the patient recovery in 9 days, while antibiotic chemotherapy revealed only a clinical improvement after 29 days (Kutateladze and Adamia, 2008). Similarly, many other bacterial infections can be alternatively treated with bacteriophages, including chronic otitis, chronic infections of wounds, cystic fibrosis-associated pulmonary infections, osteomyelitis, mastitis, chronic infections of the urinary tract, gastrointestinal infections, dental caries, and endodontic infections (Harada et al., 2018; Abedon, 2019).
There is evidence that bacteriophages can be effectively used against bacterial infections, including those that have proved to be resistant to treatments with antibiotics (Abedon, 2019). Staphylococcus aureus, for example, is reported to be resistant to methicillin (MRSA), vancomycin (VRSA), and vancomycin-intermediate sensitivity (VISA) (Fadlallah et al., 2015). Some studies have shown that bacteriophage therapy for the treatment of infections caused by such bacteria has been successful. Fadlallah et al. (2015) reported the treatment of corneal abscess caused by VISA using the bacteriophage S. aureus SATA-8505 (ATCC PTA-9476).
Although treatment with bacteriophages seems a promising advantage compared to conventional antibiotics and disinfectants, a major drawback of this approach is the need for identification of the specificity range against the pathogenic bacteria prior to starting the bacteriophage treatment and the lack of protocols for testing bacterial susceptibility in vitro (Kutateladze and Adamia, 2010). As with antibiotics, if incomplete bacterial elimination by bacteriophages occurs, this could result in the pathogen reemergence (Carlton et al., 2005; Bigwood et al., 2008). A probable explanation could be that bacteria might show a temporal resistance, or that the bacteriophage infection results in high levels of reduction but not a complete elimination of bacteria (Hoskisson and Smith, 2007; Tokman et al., 2016; Moye et al., 2018).
However, contrasting findings of the bacteriophages cocktails effectiveness (compared to “conventional” treatments such as antibodies) were also achieved, with limitations and advantages in the use of cocktails to treat bacterial infections being extensively reviewed (Altamirano and Barr, 2019; Furfaro et al., 2018; Principi et al., 2019). In study conducted by Jault et al. (2019), a cocktail contend 12 bacteriophages was compared to antibody treatment in patients with skin infections, in a randomized control trial. At the end of the study, the conventional treatment with a 1% sulfadiazine silver emulsion cream was still more effective than the cocktail. However, more randomized, placebo-controlled trials must be done in order to have some consensus in dealing with the use of bacteriophages to treat infections.
There are some limitations in the use of bacteriophages for treating human infections. Due to the ability of certain bacteriophages to integrate their genome into the host’s genome, care must be taken when selecting isolated bacteriophages. Some bacteriophages have potential for gene transfer; for instance, the bacterial acquisition of antibiotic resistance genes (ARGs) occurs by transduction, with bacteriophages acting as mobile genetic elements (MGE). Consequently, bacteriophages have been studied as possible vehicles of ARGs, not only as a source, but also as propagators in the environment (Gunathilaka et al., 2017). Bacteriophages containing ARGs are present in a wide range of environments; however, some environmental niches have a greater abundance, such as freshwater or marine environments (Lekunberri et al., 2017a,b; Calero-Cáceres and Luis, 2019). Bacteriophages can be also found in hospital wastewater, yet human-associated viromes rarely charge ARGs (Figure 1B; Enault et al., 2017; Lekunberri et al., 2017a,b).
Control Tools for Food and Environmental Bacterial Contamination
Foodborne pathogens are a major food safety threat, in 2010 an estimated 2 billion individuals contracted foodborne illnesses, resulting in 1 million deaths around the world (Kirk et al., 2015). Food safety is regarded by the World Health Organization [WHO] (2015) as a major obstacle in human development, especially in developing countries that lack infrastructure and proper environmental health practices to counter the issue. The application of bacteriophages has been proposed as an alternative tool to disinfect food and food-related environments (Pang et al., 2017). The advantage of this method is that bacteriophages kill their bacterial hosts without changing food organoleptic properties (Loc-Carrillo and Abedon, 2011; Perera et al., 2015). Also, bacteriophage low-cost large scale production, self-replicating nature, and low toxicity provide a cheap and safe disinfecting agent for low-income communities, being employed in the former Soviet Union for over 100 years (Skurnik et al., 2007; Abedon et al., 2011; Wójcik et al., 2020).
The United States Department of Agriculture (USDA) approved some products based on bacteriophages as food sanitizers, such as ListShield™, Listex P-100™, SalmoFresh™, and Salmonelex™ (Hagens and Loessner, 2010). The use of a bacteriophage cocktail to inactivate foodborne bacteria like S. enteritidis and S. typhimurium on the chicken breast has also been proposed (Duc et al., 2018). These bacteriophages have been isolated from environmental sources such as wastewater, sewage, water or food (Pereira et al., 2016). Bacteriophages may also be applied for biofilm control on the food industry, such structures form on surfaces that have been improperly sanitized (Jessen and Lammert, 2003). Outbreaks of bacterial pathogens associated to biofilms in food chain have been related to the presence of Listeria monocytogenes, Yersinia enterocolitica, Campylobacter jejuni, Salmonella spp., Staphylococcus spp. and E. coli O157:H7 (Aarnisalo et al., 2007). In this context, bacteriophages have been suggested as a green strategy for biofilm control, as they may provide a natural, highly specific, non-toxic, feasible approach for biofilm formation control (Grant et al., 2017). Biofilm control using bacteriophages has also been used to prevent dental caries, where the bacteriophages were first isolated from saliva samples and also in biofilm-mediated illness like endodontic disease, which is caused by dental pulp infection (Stevens et al., 2009; Dalmasso et al., 2015). However, it is important to highlight that each bacterial serovar could show different degrees of susceptibility to different bacteriophages (Grant et al., 2017). In addition, it is important to highlight that biofilm control by bacteriophages is mediated by the chemical composition, environmental factors, growth stage and bacteriophage concentration. Additionally, bacteriophage-biofilm interactions depend on the susceptibility of the biofilm cells and availability of receptor sites, where bacteriophage production of polysaccharide-degrading enzymes combined with effective cell lysis may rapidly destroy the biofilm (Simões et al., 2010).
Bacteriophages also show significant potential in the animal production chain such as fish, poultry, shrimps, oysters, sheep, pork and also as additives in food products such as poultry meat and eggs (Moye et al., 2018). They can prevent foodborne pathogens such as Campylobacter spp., E. coli, L. monocytogenes, Salmonella enterica, and Shigella spp., that are the top five foodborne public health threatening pathogens (Figueiredo and Almeida, 2017; Harada et al., 2018; Kim et al., 2020).
Bacteriophages have shown very effective to control L. monocytogenes by the commercial product based on bacteriophages LISTEXP™100 reported a better efficacy against L. monocytogenes than nisin and sodium lactate in ready-to-eat (RTE) sliced pork ham (Figueiredo and Almeida, 2017). Chibeu et al. (2013) used a Listeria-specific bacteriophage on the surface of deli meats; a single bacteriophage strain was effective in reducing the numbers of Listeria cells (ATCC 19115). The evaluation of LISTEX™P100 as a bacteria controller measured the bacteriophage inactivation using black tea extract and ferrous sulfate and isolation of regrowth bacteria and their control. The result was the reduction of 1.5–2.1 log10 CFU/cm2 on RTE meat samples by application of 100 μl LISTEXP™100 covering 10 cm2 area during 28 days, resulting on 107 PFU/cm2 final concentration. A cocktail of bacteriophages can be a more effective approach against a unique species of bacteria, ensuring that resistant bacteria are not selected. The application of the cocktail ListShield™ including six L. monocytogenes specific bacteriophages efficiently reduces this pathogen in cheese, smoked salmon, apple slices, and frozen entrees (reduction of 91, 82, 90, and 99%, respectively), without changing the food organoleptic properties (Perera et al., 2015). Similarly, reductions of up to 5 logs of L. monocytogenes were observed in various solid foods, such as smoked salmon, iceberg lettuce leaves, sliced cabbage, hot dogs, mixed seafood, turkey meat, and mozzarella cheese brine (Guenther et al., 2009). In fact, the use of a lytic bacteriophage on soft cheese was able to reduce 2 logs of the Listeria contamination while maintaining the natural microbial community of the cheese, reinforcing the host specificity of bacteriophages, and in this case the bacteriophage A511 (Guenther and Loessner, 2011).
The number of commercial solutions containing bacteriophages is increasing worldwide, being an emerging industry and field of research (Sulakvelidze, 2013; Vikram et al., 2020). Different examples of bacteriophage applications on food industry are already available: a three-bacteriophage cocktail (containing EC6, EC9, and EC11) was able to reduce E. coli contamination; E. coli ATCC 25922 and E. coli O127:H6 in Ultra High Temperature (UHT) milk at 25°C and under refrigeration temperatures (5–9°C) (McLean et al., 2013). The cocktail EcoShield™ was able to reduce 2 logs of E. coli O157:H7, 30 min after administration on leafy greens under packaging storage (Boyacioglu et al., 2013). Magnone et al. (2013) verified the disinfection of E. coli, Salmonella and Shigella from broccoli, cantaloupe and strawberries, with the use of commercial bacteriophage cocktails (EcoShield™, SalmoFresh™, and ShigActive™) being as effective or even more than chlorine wash. Salmonella is a major threat for the food industry and the most common zoonotic foodborne pathogen isolated from livestock (Jajere, 2019). The bacteriophage FO1-E2 was able to reduce the levels of Salmonella contamination on milk and mixed seafood for 24 h, remaining undetectable at 8 and 15°C (Guenther et al., 2012). Similarly, bacteriophage wksl3 was also able to decrease by 3 logs Salmonella contaminations on chicken skin (Kang et al., 2013). Likewise, some bacteriophage cocktails for Salmonella control are also available. The commercial formulation SalmoFresh™ was able to reduce 2–3 logs of Salmonella on lettuce and sprouts, showing greater reduction (2.7–3.8 logs) when associated with chlorinated water (Zhang et al., 2013). An outstanding biocontrol activity was seen with the use of a bacteriophage cocktail composed by LPSTLL, LPST94, and LPST153, being able to reduce >5.23 log viable Salmonella counts on biofilm grow in microtiter plates and steel chips, for 72 h. The same bacteriophages combination was also able to completely reduce the Salmonella inoculum on chicken breast and milk (Islam et al., 2019). Table 2 summarizes the bacteriophages commercially available and the conditions for its applicability on treatment against different bacterial agents.
Indicators of the Presence of Foodborne Pathogens
Bacteriophages have been suggested as an alarm system in food and environmental microbiology and epidemiology since they generally fit the indicator criteria of pollution. Bacteriophages can be used as fecal indicators or microbial water quality bioindicators as an early warning of contamination by sewage, and as an efficiency marker of water or wastewater treatment (Yahya et al., 2015). This can be attributed to the bacteriophage response to the presence of pollutants, they are characteristic to adsorb to solid particles in the environment, and also due to some limitations of traditional indicators for public health such as fecal coliforms, E. coli and enterococci (Armon and Kott, 1993; Ashbolt et al., 2001; Jofre et al., 2016; McMinn et al., 2017).
Somatic coliphages are more persistent than traditional indicators, being also more resistant to sludge treatments, particularly when adsorbed to surfaces (Martín-Díaz et al., 2020). Many authors highlight the use of bacteriophages as indicators not only for enteric pathogenic bacteria, but also for enteric viruses such as human noroviruses, adenoviruses, and rotaviruses (Guelin, 1948; Dutka, 1990; Cornax et al., 1991; Kott, 1992; Armon and Kott, 1996; Leclerc et al., 2000; Arredondo-Hernandez et al., 2017; McMinn et al., 2017). This characteristic is due to the wide stability of phages in waste, water, soils and residues, with F-specific phages and somatic coliphages being the most used for monitoring water quality (Grabow, 2001; Sinton et al., 2002).
One of the challenges with bacteriophage application is related to bacteriophage-host interaction, which could vary depending on exposition temperature, where greater bacterial reductions are associated with higher temperatures (Tomat et al., 2013). The use of bacteriophages on wastewater treatment systems is based on their lytic capacity, which is a useful tool for the removal of human and animal pathogenic bacteria from wastewater or applied as an indicator for the presence of bacteria in wastewater treatment systems (Stefanakis et al., 2019). MS2 bacteriophages have been proposed to be suitable as operational monitoring indicators as established by guidelines of Australia, due to resistance to variation of pH and temperature (Amarasiri et al., 2017). Other applications of bacteriophages in the improvement of environmental quality are based on their survival in the environment, and soil percolation to control pathogenic bacteria in underground water (Ye et al., 2019). However, there are still some challenges for the use of bacteriophages in wastewater treatment: a high bacteriophage dosing must be used for a successful application and the use of polyvalent bacteriophages with a wider host variety could result in the reduction of beneficial bacteria. The bacterial analysis of the system is a basic requirement for bacteriophage application, as the bacterial population can change in the wastewater treatment plant (Jassim et al., 2016).
Challenges, Concerns and Trends in the Use of Bacteriophages for Environmental Health Purposes
Although a worldwide acceptance of bacteriophages as environmental agents is not yet achieved, bacteriophage-based technologies in the environmental field are still being developed. Besides being employed as monitoring agents, or by directly controlling pathogens, bacteriophages have demonstrated promising results in agricultural microbiome modulation, increasing crop production by infecting crop detrimental bacteria in leaves and soil (Jones et al., 2012; Ye et al., 2019). Plant-soil microbiome modulation by bacteriophages was even related to an increase in ammonium concentration, likely through lysis of certain bacteria and overall community shifting (Braga et al., 2020). The use of bacteriophages on plant soil was referred to as a safer and more reliable antibacterial agent than antibiotics, in which the exaggerated use of these chemicals was related to the development of ARGs and inhibition of soil phosphatase activity (Liu et al., 2009; Zhang et al., 2017; Sun et al., 2019).
Similar to soil applications, bacteriophages appears to have a low environmental impact in fish farming plants compared to “traditional” methods such as antibodies, as it is necessary a continuous application since seawater is considered a reservoir of antibiotic resistance bacteria (Almeida et al., 2009; Alves et al., 2014; Hatosy and Martyiny, 2015). Even though bacteriophages can be considered as highly flexible and cheap tools, some drawbacks concerning the safety and overall effectiveness of the phage product may hinder their implementation as a widely accepted technology (Payne and Jansen, 2003). Bacteriophages can increase bacteria pathogenicity and fitness by transferring toxin and environmental resistance encoding genes to nearby bacteria, essentially creating genetic hazards in the area of application (Colomer-Lluch et al., 2011; Feiner et al., 2015). Besides bacteriophage-induced resistance, the bacteria may also become resistant to the virus activity through spontaneous mutations or through adaptive immunity via the CRISPR system (Labrie et al., 2010). Another possible major drawback in bacteriophage application is the potential disruption of the local microbiome, consequently favoring the development of harmful bacteria or health problems associated to a microbiome disbalance. Bacteriophage application has been tied to microbiome dysbiosis in humans, and can be related to the subsequent development of intestinal and mental diseases (Norman et al., 2015; Tetz et al., 2018). Microbiome disruption was also related to the development of diseases in both livestock and plants, therefore an improper bacteriophage-based product (i.e., bacteriophages that may infect healthy microbiome) may also potentially harm animal and plant farming production (Meaden and Koskella, 2013; Zeineldin et al., 2018; Lei, 2020).
In sight that bacteriophages may persist in food production plants due the virus high stability, potentially creating a genetic hazard in such facilities, the adoption of strategies for the use and manipulation of bacteriophages are required to counter bacteria resistance and achieve successful pathogen control (Hungaro et al., 2013; Chaturongakul and Ounjai, 2014; Fister et al., 2016, 2019). In this regard, practices that reduce the probability of bacteriophage resistance occurrence must be preferred, such as a two-stage self-cycling or a cellstat process (García et al., 2019). Bacteriophage cocktails have been also been employed as a way to counter bacterial bacteriophage resistance, in this strategy the bacteria would be unable to adapt (or have their viability greatly reduced) to the different infective dynamics of each virus, however, knowledge about the cocktail pharmacodynamic is required to achieve a multi-targeting system against the same bacterial strain (Abedon et al., 2021).
Aside from ARGs screening and mapped host targeting, the phage product must be suitable to the external factors present in the area of application, being resistant to the pH, temperature, UV radiation, salinity and ionic profile of the environment (Jończyk et al., 2011; Zaczek-Moczydłowska et al., 2020). In addition, the criteria for bacteriophage use in food and the environment, such as minimum exposure time, minimum effective dosage and characterization of animal local application must be established to achieve the expected therapy result while avoiding potentials drawbacks such as the presence of inhibitory compounds like antibodies, whey proteins or bacteriocins (Abedon, 2012; Vongkamjan et al., 2013; Ly-Chatain, 2014).
Special regards covering the bacteriophage properties are also advisable for an optimal and highly scalable confection of the final viral product, being of special relevance in extensive environmental applications. Bacteriophage production is directly related to the characteristics of the bacterial host (e.g., metabolic activity, growth rate, stage in cell life cycle, and abundance of bacteriophage receptors on cell surface), and the bacteriophage attributes (e.g., lysis time, burst size, and adsorption rate) (Agboluaje and Sauvageau, 2018). In addition, the initial multiplicity of infection (MOI), pH, aeration rate, presence of ions or cofactors, agitation and medium composition may also influence the outcome of infections, thus affecting bacteriophage production (Agboluaje and Sauvageau, 2018). Therefore, a full characterization of the virus and host synergy is highly advisable for easy escalation of the phage product (García et al., 2019).
With advances in molecular biology the engineering of bacteriophage particles allows a selected virus (favorited due desirable characteristics to the target therapy, such as host range and replicative potential) to be further enhanced through genetic modifications, removing undesirable viral properties that could hinder the application of the bacteriophage product as a safe and reliable object (Górski et al., 2018). Genetic engineering of phage products was able to remove toxin encoding genes and increment the virus stability in low pH environments, enhancing the functionality and removing safety hazards of the final viral product without requiring the selection of new bacteriophage strains (Nobrega et al., 2016; Park et al., 2017).
Although bacteriophages present certain safety drawbacks, largely due to negligence of mapping the product properties, bacteriophages are still considered safer than chemical treatments in environmental and food processing plants treatments applications (Meaden and Koskella, 2013; Zaczek et al., 2014). Bacteriophages stand as cheap and highly flexible structures, being able to be selected and edited for different approaches (Farr et al., 2014; Sunderland et al., 2017). Most of the research on bacteriophages has highlighted the potential for in vitro applications, and the number of scientific publications has increased in the last decades due to the potential use of bacteriophages in a broad spectrum of applications. In health sciences, bacteriophages are a promising approach in the fight against antibiotic-resistant bacteria, and, in the food chain, they could be a safe alternative for the control of foodborne pathogens. However, to guarantee effectiveness, a detailed understanding of the interaction between bacteriophages and the hosts is needed, considering restrictive criteria for their use to minimize their negative impact on food and food-related environments.
Author Contributions
PR directs the first version of the manuscript. GF and DR-L revised the first version of the manuscript and wrote the final version of the manuscript. The rest of the authors gave fundamental contributions to the first version of the manuscript. All authors contributed to the article and approved the submitted version.
Conflict of Interest
The authors declare that the research was conducted in the absence of any commercial or financial relationships that could be construed as a potential conflict of interest.
Publisher’s Note
All claims expressed in this article are solely those of the authors and do not necessarily represent those of their affiliated organizations, or those of the publisher, the editors and the reviewers. Any product that may be evaluated in this article, or claim that may be made by its manufacturer, is not guaranteed or endorsed by the publisher.
References
Aarnisalo, K., Lundén, J., Korkeala, H., and Wirtanen, G. (2007). Susceptibility of Listeria monocytogenes strains to disinfectants and chlorinated alkaline cleaners at cold temperatures. LWT Food Sci. Technol. 40, 1041–1048. doi: 10.1016/j.lwt.2006.07.009
Abedon, S. T. (2012). Bacterial ‘immunity’ against bacteriophages. Bacteriophage 2, 50–54. doi: 10.4161/bact.18609
Abedon, S. T. (2019). Use of phage therapy to treat long-standing, persistent, or chronic bacterial infections. Adv. Drug Deliv. Rev. 145, 18–39. doi: 10.1016/j.addr.2018.06.018
Abedon, S. T., Danis-Wlodarczyk, K. M., and Wozniak, D. J. (2021). Phage cocktail development for bacteriophage therapy: toward improving spectrum of activity breadth and depth. Pharmaceuticals 14:1019. doi: 10.3390/ph14101019
Abedon, S. T., Kuhl, S. J., Blasdel, B. G., and Kutter, E. M. (2011). Phage treatment of human infections. Bacteriophage 1, 66–85. doi: 10.4161/bact.1.2.15845
Agboluaje, M., and Sauvageau, D. (2018). Bacteriophage production in bioreactors. Methods Mol. Biol. 1693, 173–193. doi: 10.1007/978-1-4939-7395-8_15
Almeida, A., Cunha, Â, Gomes, N. C., Alves, E., Costa, L., and Faustino, M. A. (2009). Phage therapy and photodynamic therapy: low environmental impact approaches to inactivate microorganisms in fish farming plants. Mar. Drugs 7, 268–313. doi: 10.3390/md7030268
Altamirano, F. L. G., and Barr, J. J. (2019). Phage therapy in the postantibiotic era. Clin. Microbiol. Rev. 32:e066-18. doi: 10.1128/CMR.00066-18
Alves, M. S., Pereira, A., Araújo, S. M., Castro, B. B., Correia, A. C. M., and Henriques, I. (2014). Seawater is a reservoir of multi-resistant Escherichia coli, including strains hosting plasmid-mediated quinolones resistance and extended-spectrum beta-lactamases genes. Front. Microbiol. 5:426. doi: 10.3389/fmicb.2014.00426
Amarasiri, M., Kitajima, M., Nguyen, T. H., and Okabe, S. (2017). Bacteriophage removal efficiency as a validation and operational monitoring tool for virus reduction in wastewater reclamation: review. Water Res. 121, 258–269. doi: 10.1016/j.watres.2017.05.035
Armon, R., and Kott, Y. (1993). A simple, rapid and sensitive presence/absence detection test for bacteriophage in drinking water. J. Appl. Bacteriol. 74, 490–496. doi: 10.1111/j.1365-2672.1993.tb05159.x
Armon, R., and Kott, Y. (1996). Bacteriophages as indicators of pollution. Crit. Rev. Environ. Sci. Technol. 26, 299–335. doi: 10.1080/10643389609388494
Arredondo-Hernandez, L. J. R., Diaz-Avalos, C., Lopez-Vidal, Y., Castillo-Rojas, G., and Mazari-Hiriart, M. (2017). FRNA bacteriophages as viral indicators of faecal contamination in Mexican tropical aquatic systems. PLoS One 12:e0170399. doi: 10.1371/journal.pone.0170399
Ashbolt, N. J., Grabow, W. O. K., and Snozzi, M. (2001). “Indicators of microbial water quality,” in Water Quality: Guidelines, Standards and Health, eds L. Fewtrell and J. Bartram (London: IWA Publishing), 289–315.
Balter, M. (2000). Evolution on Life’s fringes. Science 289, 1866–1867. doi: 10.1126/science.289.5486.1866
Bigot, B., Lee, W., Mcintyre, L., Wilson, T., Hudson, J. A., Billington, C., et al. (2011). Control of Listeria monocytogenes growth in a ready-to-eat poultry product using a bacteriophage. Food Microbiol. 28, 1448–1452. doi: 10.1016/j.fm.2011.07.001
Bigwood, T., Hudson, J. A., Billington, C., Carey-Smith, G. V., and Heinemann, J. A. (2008). Phage inactivation of foodborne pathogens on cooked and raw meat. Food Microbiol. 25, 400–406. doi: 10.1016/j.fm.2007.11.003
Bobay, L. M., Rocha, E. P. C., and Touchon, M. (2013). The adaptation of temperate bacteriophages to their host genomes. Mol. Biol. Evol. 30, 737–751. doi: 10.1093/molbev/mss279
Boehme, J. (1993). Viruses, bacterioplankton, and Phytoplankton in the Southeastern Gulf of Mexico: distribution and contribution to Oceanic DNA pools. Mar. Ecol. Prog. Ser. 97, 1–10. doi: 10.3354/meps097001
Boyacioglu, O., Sharma, M., Sulakvelidze, A., and Goktepe, I. (2013). Biocontrol of Escherichia coli O157. Bacteriophage 3:e24620. doi: 10.4161/bact.24620
Boyd, E. F. (2012). “Bacteriophage-encoded bacterial virulence factors and phage-pathogenicity island interactions,” in Advances in Virus Research, 1st Edn, eds M. Łobocka and W. T. Szybalski (Amsterdam: Elsevier), 91–118. doi: 10.1016/B978-0-12-394621-8.00014-5
Braga, L. P. P., Spor, A., Kot, W., Breuil, M.-C., Hansen, L. H., Setubal, J. C., et al. (2020). Impact of phages on soil bacterial communities and nitrogen availability under different assembly scenarios. Microbiome 8, 45–72. doi: 10.1186/s40168-020-00822-z
Brüssow, H., Canchaya, C., and Hardt, W. (2004). Phages and the evolution of bacterial pathogens: from genomic rearrangements to lysogenic conversion. Microbiol. Mol. Biol. Rev. 68, 560–602. doi: 10.1128/MMBR.68.3.560-602.2004
Calero-Cáceres, W., and Luis, J. (2019). Antibiotic resistance genes in bacteriophages from diverse marine habitats. Sci. Total Environ. 654, 452–455. doi: 10.1016/j.scitotenv.2018.11.166
Carlton, R. M., Noordman, W. H., Biswas, B., de Meester, E. D., and Loessner, M. J. (2005). Bacteriophage P100 for control of Listeria monocytogenes in foods: genome sequence, bioinformatic analyses, oral toxicity study, and application. Regul. Toxicol. Pharmacol. 43, 301–312. doi: 10.1016/j.yrtph.2005.08.005
Chaturongakul, S., and Ounjai, P. (2014). Phage-host interplay: examples from tailed phages and gram-negative bacterial pathogens. Front. Microbiol. 5:442. doi: 10.3389/fmicb.2014.00442
Chibeu, A., Agius, L., Gao, A., Sabour, P. M., Kropinski, A. M., Balamurugan, S., et al. (2013). Efficacy of bacteriophage LISTEX P100 combined with chemical antimicrobials in reducing Listeria monocytogenes in cooked turkey and roast beef. Int. J. Food Microbiol. 167, 208–214. doi: 10.1016/j.ijfoodmicro.2013.08.018
Clavijo, V., Baquero, D., Hernandez, S., Farfan, J. C., Arias, J., Arévalo, A., et al. (2019). Phage cocktail SalmoFREE reduces Salmonella on a commercial broiler farm. Poult. Sci. 98, 5054–5063. doi: 10.3382/ps/pez251
Clokie, M. R. J., Millard, A. D., Letarov, A. V., and Heaphy, S. (2011). Phages in nature. Bacteriophage 1, 31–45. doi: 10.4161/bact.1.1.14942
Colomer-Lluch, M., Jofre, J., and Muniesa, M. (2011). Antibiotic resistance genes in the bacteriophage DNA fraction of environmental samples. PLoS One 6:e17549. doi: 10.1371/journal.pone.0017549
Cornax, R., Morilgligo, M. A., Balebona, M. C., Castro, D., and Borrego, J. J. (1991). Significance of several bacteriophage groups as indicators of sewage pollution in marine waters. Water Res. 25, 673–678. doi: 10.1016/0043-1354(91)90042-O
Dalmasso, M., De Haas, E., Neve, H., Strain, R., Cousin, F. J., Stockdale, S. R., et al. (2015). Isolation of a novel phage with activity against Streptococcus mutans biofilms. PLoS One 10:e0138651. doi: 10.1371/journal.pone.0138651
Delbruck, M. (1942). “Bacterial viruses (Bacteriophages),” in Advances in Enzymology - and Related Areas of Molecular Biology, Vol. 2, eds F. F. Nord and C. H. Werkman (Hoboken, NJ: Wiley), 1–30. doi: 10.1002/9780470122471.ch1
D’Herelle, F. (1926). The bacteriophage and its behaviour. Nature 118, 183–185. doi: 10.1038/118183a0
Duc, H. M., Son, H. M., Honjoh, K., and Miyamoto, T. (2018). Isolation and application of bacteriophages to reduce Salmonella contamination in raw chicken meat. LWT Food Sci. Technol. 91, 353–360. doi: 10.1016/j.lwt.2018.01.072
Dutka, B. J. (1990). The presence of bacterial virus in groundwater and treated drinking water microbiological tests. Environ. Pollut. 63, 293–298. doi: 10.1016/0269-7491(90)90136-Z
Edgar, R., Friedman, N., Molshanski-Mor, S., and Qimron, U. (2012). Reversing bacterial resistance to antibiotics by phage-mediated f dominant sensitive genes. Appl. Environ. Microbiol. 78, 744–751. doi: 10.1128/AEM.05741-11
Enault, F., Briet, A., Bouteille, L., Roux, S., Sullivan, M. B., Petit, M-A, et al. (2017). Phages rarely encode antibiotic resistance genes: a cautionary tale for virome analyses. ISME J. 11, 237–247. doi: 10.1038/ismej.2016.90
Fadlallah, A., Chelala, E., and Legeais, J. M. (2015). Corneal infection therapy with topical bacteriophage administration. Open Ophthalmol. J. 9, 167–168. doi: 10.2174/1874364101509010167
Farr, R., Choi, D. S., and Lee, S.-W. (2014). Phage-based nanomaterials for biomedical applications. Acta Biomater. 10, 1741–1750. doi: 10.1016/j.actbio.2013.06.037
Feiner, R., Argov, T., Rabinovich, L., Sigal, N., Borovok, I., and Herskovits, A. A. A. (2015). new perspective on lysogeny: prophages as active regulatory switches of bacteria. Nat. Rev. Microbiol. 13, 641–650. doi: 10.1038/nrmicro3527
Figueiredo, A. C. L., and Almeida, R. C. C. (2017). Antibacterial efficacy of nisin, bacteriophage P100 and sodium lactate against Listeria monocytogenes in ready-to-eat sliced pork ham. Braz. J. Microbiol. 48, 724–729. doi: 10.1016/j.bjm.2017.02.010
Filée, J., Forterre, P., and Laurent, J. (2003). The role played by viruses in the evolution of their hosts: a view based on informational protein phylogenies. Res. Microbiol. 154, 237–243. doi: 10.1016/S0923-2508(03)00066-4
Filée, J., Forterre, P., Sen-lin, T., and Laurent, J. (2002). Evolution of DNA polymerase families: evidences for multiple gene. J. Mol. Evol. 54, 763–773. doi: 10.1007/s00239-001-0078-x
Fister, S., Fuchs, S., Stessl, B., Schodera, D., Wagner, M., and Rossmanitha, P. (2016). Screening and characterisation of bacteriophage P100 insensitive Listeria monocytogenes isolates in Austrian dairy plants. Food Control. 59, 108–117. doi: 10.1016/j.foodcont.2015.05.026
Fister, S., Mester, P., Witte, A. K., Sommer, J., Schodera, D., Rossmanith, P., et al. (2019). Part of the problem or the solution? Indiscriminate use of bacteriophages in the food industry can reduce their potential and impair growth-based detection methods. Trends Food Sci. Technol. 90, 170–174. doi: 10.1016/j.tifs.2019.02.031
Forterre, P. (1999). Displacement of cellular proteins by functional analogues from plasmids or viruses could explain puzzling phylogenies of many DNA informational proteins. Mol. Microbiol. 33, 457–465. doi: 10.1046/j.1365-2958.1999.01497.x
Friedman, A., and Lai, X. (2018). Combination therapy for cancer with oncolytic virus and checkpoint inhibitor: a mathematical model. PLoS One 13:e0192449. doi: 10.1371/journal.pone.0192449
Fuhrman, J. A. (1999). Marine viruses and their biogeochemical and ecological effects. Nature 399, 541–548. doi: 10.1038/21119
Fuller, N. J., Wilson, W. H., Joint, I. R., and Mann, N. H. (1998). Occurrence of a sequence in marine cyanophages similar to that of T4 g20 and its application to PCR-based detection and quantification techniques. Appl. Environ. Microbiol. 64, 2051–2060. doi: 10.1128/AEM.64.6.2051-2060.1998
Furfaro, L. L., Payne, M. S., and Chang, B. J. (2018). Bacteriophage therapy: clinical trials and regulatory hurdles. Front. Cell. Infect. Microbiol. 8:376. doi: 10.3389/fcimb.2018.00376
García, R., Latz, S., Romero, J., Higuera, G., García, K., and Bastías, R. (2019). Bacteriophage production models: an overview. Front. Microbiol. 10:1187. doi: 10.3389/fmicb.2019.01187
Górski, A., Międzybrodzki, R., Łobocka, M., Głowacka-rutkowska, A., Bednarek, A., Borysowski, J., et al. (2018). Phage therapy: what have we learned? Viruses 10:288. doi: 10.3390/v10060288
Grabow, W. O. K. (2001). Bacteriophages: update on application as models for viruses in water. Water 27, 251–268. doi: 10.4314/wsa.v27i2.4999
Grant, A. Q., Parveen, S., Schwarz, J., Hashem, F., and Vimini, B. (2017). Reduction of Salmonella in ground chicken using a bacteriophage. Poult. Sci. 96, 2845–2852. doi: 10.3382/ps/pex062
Guenther, S., Herzig, O., Fieseler, L., Klumpp, J., and Loessner, M. J. (2012). Biocontrol of Salmonella Typhimurium in RTE foods with the virulent bacteriophage FO1-E2. Int. J. Food Microbiol. 154, 66–72. doi: 10.1016/j.ijfoodmicro.2011.12.023
Guenther, S., Huwyler, D., Richard, S., and Loessner, M. J. (2009). Virulent bacteriophage for efficient biocontrol of Listeria monocytogenes in ready-to-eat foods. Appl. Environ. Microbiol. 75, 93–100. doi: 10.1128/AEM.01711-08
Guenther, S., and Loessner, M. J. (2011). Bacteriophage biocontrol of Listeria monocytogenes on soft ripened white mold and red-smear cheeses. Bacteriophage 1, 94–100. doi: 10.4161/bact.1.2.15662
Gunathilaka, G. U., Tahlan, V., Mafiz, A. I., Polur, M., and Zhang, Y. (2017). International journal of antimicrobial agents phages in urban wastewater have the potential to disseminate antibiotic resistance. Int. J. Antimicrob. Agents 50, 678–683. doi: 10.1016/j.ijantimicag.2017.08.013
Hagens, S., and Loessner, M. J. (2010). Bacteriophage for biocontrol of foodborne pathogens: calculations and considerations. Curr. Pharm. Biotechnol. 11, 58–68. doi: 10.2174/138920110790725429
Harada, L. K., Silva, E. C., Campos, W. F., Del Fiol, F. S., Vila, M., Dąbrowska, K., et al. (2018). Biotechnological applications of bacteriophages: state of the art. Microbiol. Res. 212–213, 38–58. doi: 10.1016/j.micres.2018.04.007
Hatosy, S. M., and Martyiny, A. C. (2015). The ocean as a global reservoir of antibiotic resistance genes. Appl. Environ. Microbiol. 81, 7593–7599. doi: 10.1128/AEM.00736-15
Higgins, J. P., Higgins, S. E., Guenther, K. L., Huff, W., Donoghue, A. M., Donoghue, D. J., et al. (2005). Use of a specific bacteriophage treatment to reduce Salmonella in poultry products. Poult. Sci. 84, 1141–1145. doi: 10.1093/ps/84.7.1141
Hoskisson, P. A., and Smith, M. C. M. (2007). Hypervariation and phase variation in the bacteriophage ‘resistome’. Curr. Opin. Microbiol. 10, 396–400. doi: 10.1016/j.mib.2007.04.003
Hungaro, H. M., Mendonêa, R. C. S., Gouvça, D. M., Vanetti, M. C. D., and Pintoc, C. L. O. (2013). Use of bacteriophages to reduce Salmonella in chicken skin in comparison with chemical agents. Food Res. Int. 52, 75–81. doi: 10.1016/j.foodres.2013.02.032
Islam, M. S., Zhou, Y., Liang, L., Nime, I., Liu, K., Yan, T., et al. (2019). Application of a phage cocktail for control of Salmonella in foods and reducing biofilms. Viruses 11:841. doi: 10.3390/v11090841
Jajere, S. M. (2019). A review of Salmonella enterica with particular focus on the pathogenicity and virulence factors, host specificity and antimicrobial resistance including multidrug resistance. Vet. World 12, 2231–2916. doi: 10.14202/vetworld.2019.504-521
Janczuk, M., Niedzió, J., and Szot-Karpi, K. (2016). Bacteriophages in electrochemistry: a review. J. Electroanal. Chem. 779, 207–219. doi: 10.1016/j.jelechem.2016.05.019
Jassim, S. A. A., Limoges, R. G., and El-Cheikh, H. (2016). Bacteriophage biocontrol in wastewater treatment. World J. Microbiol. Biotechnol. 32, 1–10. doi: 10.1007/s11274-016-2028-1
Jault, P., Leclerc, T., Jennes, S., Pirnay, J. P., Que, Y., Resch, G., et al. (2019). Efficacy and tolerability of a cocktail of bacteriophages to treat burn wounds infected by Pseudomonas aeruginosa (PhagoBurn): a randomised, controlled, double-blind phase 1/2 trial. Lancet Infect. Dis. 19, 35–45. doi: 10.1016/S1473-3099(18)30482-1
Jessen, B., and Lammert, L. (2003). Biofilm and disinfection in meat processing plants. Int. Biodeter. Biodegrad. 51, 265–269. doi: 10.1016/S0964-8305(03)00046-5
Jofre, J., Lucena, F., Blanch, A. R., and Muniesa, M. (2016). Coliphages as model organisms in the characterization and management of water resources. Water 8:199. doi: 10.3390/w8050199
Jończyk, E., Kłak, M., Międzybrodzki, R., and Górski, A. (2011). The influence of external factors on bacteriophages—review. Folia Microbiol. 56, 191–200. doi: 10.1007/s12223-011-0039-8
Jones, J. B., Vallad, G. E., Iriarte, F. B., Obradović, A., Wernsing, M. H., Jackson, L. E., et al. (2012). Considerations for using bacteriophages for plant disease control. Bacteriophage 2, 689–709. doi: 10.4161/bact.23857
Kang, H., Kim, J., Jung, T., and Woo, G.-J. (2013). wksl3, a new biocontrol agent for Salmonella enterica Serovars Enteritidis and Typhimurium in foods: characterization, application, sequence analysis, and oral acute toxicity study. Appl. Environ. Microbiol. 79, 1956–1968. doi: 10.1128/AEM.02793-12
Kim, H. J., Giri, S. S., Kim, S. G., Kim, S. W., Kwon, J., Lee, S. B., et al. (2020). Isolation and characterization of two bacteriophages and their preventive effects against pathogenic vibrio coralliilyticus causing mortality of pacific oyster (Crassostrea Gigas) larvae. Microorganisms 8:926. doi: 10.3390/microorganisms8060926
King, A. M. Q., Elliot, L., Michael, J. A., and Eric, B. C. (2012). Virus Taxonomy. Amsterdam: Elsevier.
Kirk, M. D., Pires, S. M., Black, R. E., Caipo, M., Crump, J. A., Devleesschauwer, B., et al. (2015). World health organization estimates of the global and regional disease burden of 22 foodborne bacterial, protozoal, and viral diseases. PLoS Med. 12:e1001940. doi: 10.1371/journal.pmed.1001940
Kott, Y. (1992). Wastewater upgrading as measured by coliphage. Environ. Toxicol. Water Qual. Ann. Int. J. 7, 61–69. doi: 10.1002/tox.2530070106
Kutateladze, M., and Adamia, R. (2008). Phage therapy experience at the Eliava Institute. Med. Mal. Infect. 38, 426–430. doi: 10.1016/j.medmal.2008.06.023
Kutateladze, M., and Adamia, R. (2010). Bacteriophages as potential new therapeutics to replace or supplement antibiotics. Trends Biotechnol. 28, 591–595. doi: 10.1016/j.tibtech.2010.08.001
Labrie, S. J., Samson, J. E., and Moineau, S. (2010). Bacteriophage resistance mechanisms. Nat. Rev. Microbiol. 8, 317–327. doi: 10.1038/nrmicro2315
Leclerc, H., Edberg, S., and Pierzo, V. (2000). Bacteriophages as indicators of enteric viruses and public health risk in groundwaters. J. Appl. Microbiol. 88, 5–21. doi: 10.1046/j.1365-2672.2000.00949.x
Lekunberri, I., Subirats, J., and Borrego, C. M. (2017a). Exploring the contribution of bacteriophages to antibiotic resistance. Environ. Pollut. 220, 981–984. doi: 10.1016/j.envpol.2016.11.059
Lekunberri, I., Villagrasa, M., Luis, J., and Borrego, C. M. (2017b). Contribution of bacteriophage and plasmid DNA to the mobilization of antibiotic resistance genes in a river receiving treated wastewater discharges. Sci. Total Environ. 601–602, 206–209. doi: 10.1016/j.scitotenv.2017.05.174
Leverentz, B., Conway, W. S., Alavidze, Z., Janisiewicz, W. J., Fuchs, Y., Camp, M. J., et al. (2001). Examination of bacteriophage as a biocontrol method for Salmonella on fresh-cut fruit: a model study. J. Food Prot. 64, 1116–1121. doi: 10.4315/0362-028X-64.8.1116
Liu, F., Ying, G.-G., Tao, R., Zhao, J.-L., Yang, J.-F., and Zhao, L.-F. (2009). Effects of six selected antibiotics on plant growth and soil microbial and enzymatic activities. Environ. Pollut. 157, 1636–1642. doi: 10.1016/j.envpol.2008.12.021
Loc-Carrillo, C., and Abedon, S. T. (2011). Pros and cons of phage therapy. Bacteriophage 2, 111–114. doi: 10.4161/bact.1.2.14590
Lu, T. K., and Collins, J. J. (2009). Engineered bacteriophage targeting gene networks as adjuvants for antibiotic therapy. Proc. Natl. Acad. Sci. U.S.A. 106, 4629–4634. doi: 10.1073/pnas.0800442106
Ly-Chatain, M. H. (2014). The factors affecting effectiveness of treatment in phages therapy. Front. Microbiol. 5:51. doi: 10.3389/fmicb.2014.00051
Magnone, J. P., Marek, P. J., and Sulakvelidze, A. (2013). Additive approach for inactivation of Escherichia coli O157:H7, Salmonella, and Shigella spp. on contaminated fresh fruits and vegetables using bacteriophage cocktail and produce wash. J. Food Prot. 76, 1336–1341. doi: 10.4315/0362-028X.JFP-12-517
Marston, M. F., and Sallee, J. L. (2003). Genetic diversity and temporal variation in the cyanophage community infecting marine Synechococcus species in Rhode Island’s coastal waters. Appl. Environ. Microbiol. 69, 4639–4647. doi: 10.1128/AEM.69.8.4639-4647.2003
Martín-Díaz, J., Lucena, F., Blanch, A. R., and Jofre, J. (2020). Review: indicator bacteriophages in sludge, biosolids, sediments and soils. Environ. Res. 182:109133. doi: 10.1016/j.envres.2020.109133
McLean, S. K., Dunn, L. A., and Palombo, E. A. (2013). Phage inhibition of Escherichia coli in ultrahigh-temperature-treated and raw milk. Foodb. Pathog. Dis. 10, 956–962. doi: 10.1089/fpd.2012.1473
McMinn, B. R., Ashbolt, N. J., and Korajkic, A. (2017). Bacteriophages as indicators of faecal pollution and enteric virus removal. Lett. Appl. Microbiol. 65, 11–26. doi: 10.1111/lam.12736
Meaden, S., and Koskella, B. (2013). Exploring the risks of phage application in the environment. Front. Microbiol. 4:358. doi: 10.3389/fmicb.2013.00358
Modi, R., Hirvi, Y., and Hill, A. (2001). Effect of phage on survival of Salmonella enteritidis during manufacture and storage of cheddar cheese made from raw and pasteurized milk. J. Food Prot. 64, 927–933. doi: 10.4315/0362-028X-64.7.927
Moye, Z. D., Woolston, J., and Sulakvelidze, A. (2018). Bacteriophage applications for food production and processing. Viruses 10:205. doi: 10.3390/v10040205
Nobrega, F. L., Costa, A. R., Santos, J. F., Siliakus, M. F., Van Lent, J. W. M., Kengen, S. W. M., et al. (2016). Genetically manipulated phages with improved pH resistance for oral administration in veterinary medicine. Sci. Rep. 6:39235. doi: 10.1038/srep39235
Norman, J. M., Handley, S. A., Baldridge, M. T., Droit, L., Liu, C. Y., Keller, B. C., et al. (2015). Disease-specific alterations in the enteric virome in inflammatory bowel disease. Cell 160, 447–460. doi: 10.1016/j.cell.2015.01.002
Pang, H., Lambertini, E., Buchanan, R. L., Schaffner, D. W., and Pradhan, A. K. (2017). Quantitative microbial risk assessment for Escherichia coli O157: H7 in fresh-cut lettuce. J. Food Prot. 80, 302–311. doi: 10.4315/0362-028X.JFP-16-246
Park, J. Y., Moon, B. Y., Park, J. W., Thornton, J. A., Park, Y. H., and Seo, K. S. (2017). Genetic engineering of a temperate phage-based delivery system for CRISPR/Cas9 antimicrobials against Staphylococcus aureus. Sci. Rep. 7:44929. doi: 10.1038/srep44929
Paul, J. H., Sullivan, M. B., Segall, A. M., and Rohwer, F. (2002). Marine phage genomics. Comp. Biochem. Physiol. Part B 133, 463–476. doi: 10.1016/S1096-4959(02)00168-9
Payne, R. J. H., and Jansen, V. A. A. (2003). Pharmacokinetic principles of bacteriophage therapy. Clin. Pharm. 42, 315–325. doi: 10.2165/00003088-200342040-00002
Penadés, J. R., Chen, J., Quiles-Puchalt, N., Carpena, N., and Novick, R. P. (2015). Bacteriophage-mediated spread of bacterial virulence genes. Curr. Opin. Microbiol. 23, 171–178. doi: 10.1016/j.mib.2014.11.019
Pereira, C., Moreirinha, C., Lewicka, M., Almeida, P., Clemente, C., Cunha, A., et al. (2016). Bacteriophages with potential to inactivate Salmonella Typhimurium: use of single phage suspensions and phage cocktails. Virus Res. 220, 179–192. doi: 10.1016/j.virusres.2016.04.020
Perera, M. N., Abuladze, T., Li, M., Woolston, J., and Sulakvelidze, A. (2015). Bacteriophage cocktail significantly reduces or eliminates Listeria monocytogenes contamination on lettuce, apples, cheese, smoked salmon and frozen foods. Food Microbiol. 52, 42–48. doi: 10.1016/j.fm.2015.06.006
Pratama, A. A., and Van Elsas, J. D. (2017). A novel inducible prophage from the mycosphere inhabitant Paraburkholderia terrae BS437. Sci. Rep. 7:9156. doi: 10.1038/s41598-017-09317-8
Principi, N., Silvestri, E., and Esposito, S. (2019). Advantages and limitations of bacteriophages for the treatment of bacterial infections. Front. Pharmacol. 10:513. doi: 10.3389/fphar.2019.00513
Simões, M., Simões, L. C., and Vieira, M. J. (2010). A review of current and emergent biofilm control strategies. LWT Food Sci. Technol. 43, 573–583. doi: 10.1016/j.lwt.2009.12.008
Sinton, L. W., Hall, C. H., Lynch, P. A., and Davies-Colley, R. J. (2002). Sunlight inactivation of 615 fecal indicator bacteria and bacteriophages from waste stabilization pond effluent in fresh and 616 saline waters. Appl. Environ. Microbiol. 68, 1122–1131. doi: 10.1128/AEM.68.3.1122-1131.2002
Skurnik, M., Pajunen, M., and Kiljunen, S. (2007). Biotechnological challenges of phage therapy. Biotechnol. Lett. 29, 995–1003. doi: 10.1007/s10529-007-9346-1
Stefanakis, A. I., Bardiau, M., Trajano, D., Couceiro, F., Williams, J. B., Taylora, H., et al. (2019). Presence of bacteria and bacteriophages in full-scale trickling fi lters and an aerated constructed wetland. Sci. Total Environ. 659, 1135–1145. doi: 10.1016/j.scitotenv.2018.12.415
Stevens, R. H., Porras, O. D., and Delisle, A. L. (2009). Bacteriophages induced from lysogenic root canal isolates of Enterococcus faecalis. Oral Microbiol. Immunol. 24, 278–284. doi: 10.1111/j.1399-302X.2009.00506.x
Sulakvelidze, A. (2013). Using lytic bacteriophages to eliminate or significantly reduce contamination of food by foodborne bacterial pathogens. J. Sci. Food Agric. 93, 3137–3146. doi: 10.1002/jsfa.6222
Sullivan, M. B., Coleman, M. L., Quinlivan, V., Rosenkrantz, J. E., DeFrancesco, A. S., Tan, J., et al. (2008). Portal protein diversity and phage ecology. Environ. Microbiol. 10, 2810–2823. doi: 10.1111/j.1462-2920.2008.01702.x
Sun, M., Ye, M., Zhang, Z., Zhang, S., Zhao, Y., Deng, S., et al. (2019). Biochar combined with polyvalent phage therapy to mitigate antibiotic resistance pathogenic bacteria vertical transfer risk in an undisturbed soil column system. J. Hazard. Mater. 365, 1–8. doi: 10.1016/j.jhazmat.2018.10.093
Sunderland, K. S., Yang, M., and Mao, C. (2017). Phage-enabled nanomedicine: from probes to therapeutics in precision medicine. Angew. Chem. Intern. Edn. 56, 1964–1992. doi: 10.1002/anie.201606181
Tetz, G., Brown, S. M., Hao, Y., and Tetz, V. (2018). Parkinson’s disease and bacteriophages as its overlooked contributors. Sci. Rep. 8:45. doi: 10.1038/s41598-018-29173-4
Tokman, J. I., Kent, D. J., Wiedmann, M., and Denes, T. (2016). Temperature significantly affects the plaquing and adsorption efficiencies of listeria phages. Front. Microbiol. 7:631. doi: 10.3389/fmicb.2016.00631
Tomat, D., Migliore, L., Aquili, V., Quiberoni, A., and Balagué, C. (2013). Phage biocontrol of enteropathogenic and shiga toxin-producing Escherichia coli in meat products. Front. Cell Infect. Microbiol. 3:20. doi: 10.3389/fcimb.2013.00020
Tree, J. J., Granneman, S., Mcateer, S. P., Tollervey, D., and Gally, D. L. (2014). Identification of bacteriophage-encoded anti-sRNAs in pathogenic Escherichia coli. Mol. Cell 55, 199–213. doi: 10.1016/j.molcel.2014.05.006
Twort, F. W. (1915). An investigation of the nature of ultra-microscopic viruses. Lancet 186, 1241–1243. doi: 10.1016/S0140-6736(01)20383-3
Vandamme, E. J., and Mortelmans, K. (2019). A century of bacteriophage research and applications: impacts on biotechnology, health, ecology and the economy. J. Chem. Technol. Biotechnol. 94, 323–342. doi: 10.1002/jctb.5810
Vikram, A., Woolston, J., and Sulakvelidze, A. (2020). Phage biocontrol applications in food production and processing. Curr. Issues Mol. Biol. 40, 267–302. doi: 10.21775/9781913652517.08
Vongkamjan, K., Roof, S., Stasiewicz, M. J., and Wiedmann, M. (2013). Persistent Listeria monocytogenes subtypes isolated from a smoked fish processing facility included both phage susceptible and resistant isolates. Food Microbiol. 35, 38–48. doi: 10.1016/j.fm.2013.02.012
Walker, S. J., Archer, P., and Banks, J. G. (1990). Growth of Listeria monocytogenes at refrigeration temperatures. J. Appl. Bacteriol. 68, 157–162. doi: 10.1111/j.1365-2672.1990.tb02561.x
Whichard, J. M., Sriranganathan, N., and Pierson, F. W. (2003). Suppression of Salmonella growth by wild-type and large-plaque variants of bacteriophage felix O1 in liquid. J. Food Prot. 66, 220–225. doi: 10.4315/0362-028X-66.2.220
Wójcik, E. A., Stańczyk, M., Wojtasik, A., Kowalska, J. D., Nowakowska, M., Łukasiak, M., et al. (2020). Comprehensive evaluation of the safety and efficacy of BAFASAL® bacteriophage preparation for the reduction of Salmonella in the food chain. Viruses 12:742. doi: 10.3390/v12070742
World Health Organization [WHO] (2015). WHO Estimates of the Global Burden of Foodborne Diseases: Foodborne Diseases Burden Epidemiology Reference Group 2007-2015. Available online at: https://www.who.int/publications/i/item/9789241565165 (accessed October, 2021).
World Health Organization [WHO] (2017). Diarrhoeal Disease. Available online at: https://www.who.int/en/news-room/fact-sheets/detail/diarrhoeal-disease (accessed October, 2021).
Yahya, M., Hmaied, F., Jebri, S., Jofre, J., and Hamdi, M. (2015). Bacteriophages as indicators of human and animal faecal contamination in raw and treated wastewaters from Tunisia. J. Appl. Microbiol. 118, 1217–1225. doi: 10.1111/jam.12774
Ye, M., Sun, M., Huang, D., Zhang, Z., Zhang, H., Zhang, S., et al. (2019). A review of bacteriophage therapy for pathogenic bacteria inactivation in the soil environment. Environ. Intern. 129, 488–496. doi: 10.1016/j.envint.2019.05.062
Yeh, Y., Purushothaman, P., Gupta, N., Ragnone, M., Verma, S. C., de Mello, A. S., et al. (2017). Bacteriophage application on red meats and poultry: effects on Salmonella population in final ground products. Meat Sci. 127, 30–34. doi: 10.1016/j.meatsci.2017.01.001
Zaczek, M., Weber-Dąbrowska, B., and Górski, A. (2014). Phages in the global fruit and vegetable industry. J. Appl. Microbiol. 118, 537–556. doi: 10.1111/jam.12700
Zaczek-Moczydłowska, M. A., Young, G. K., Trudgett, J., Plahe, C., Fleming, C. C., Campbell, K., et al. (2020). Phage cocktail containing Podoviridae and Myoviridae bacteriophages inhibits the growth of Pectobacterium spp. under in vitro and in vivo conditions. PLoS One 15:230842. doi: 10.1371/journal.pone.0230842
Zeineldin, M., Aldridge, B., and Lowe, J. (2018). Dysbiosis of the fecal microbiota in feedlot cattle with hemorrhagic diarrhea. Microb. Pathog. 115, 123–130. doi: 10.1016/j.micpath.2017.12.059
Zhang, H., Li, X., Yang, Q., Sun, L., Yang, X., Zhou, M., et al. (2017). Plant growth, antibiotic uptake, and prevalence of antibiotic resistance in an endophytic system of pakchoi under antibiotic exposure. Intern. J. Environ. Res. Public Health 14:1336. doi: 10.3390/ijerph14111336
Keywords: bacteriophages, food safety, biocontrol, foodborne pathogens, antimicrobial resistance
Citation: Rogovski P, Cadamuro RD, da Silva R, de Souza EB, Bonatto C, Viancelli A, Michelon W, Elmahdy EM, Treichel H, Rodríguez-Lázaro D and Fongaro G (2021) Uses of Bacteriophages as Bacterial Control Tools and Environmental Safety Indicators. Front. Microbiol. 12:793135. doi: 10.3389/fmicb.2021.793135
Received: 11 October 2021; Accepted: 11 November 2021;
Published: 30 November 2021.
Edited by:
Laurent Dufossé, Université de la Réunion, FranceReviewed by:
Diogo Silva, University of Brighton, United KingdomHak-Kim Chan, The University of Sydney, Australia
Copyright © 2021 Rogovski, Cadamuro, da Silva, de Souza, Bonatto, Viancelli, Michelon, Elmahdy, Treichel, Rodríguez-Lázaro and Fongaro. This is an open-access article distributed under the terms of the Creative Commons Attribution License (CC BY). The use, distribution or reproduction in other forums is permitted, provided the original author(s) and the copyright owner(s) are credited and that the original publication in this journal is cited, in accordance with accepted academic practice. No use, distribution or reproduction is permitted which does not comply with these terms.
*Correspondence: David Rodríguez-Lázaro, ZHJsYXphcm9AdWJ1LmVz; Gislaine Fongaro, Z2lzbGFpbmUuZm9uZ2Fyb0B1ZnNjLmJy