- 1Division of Applied Phycology and Biotechnology, CSIR – Central Salt and Marine Chemicals Research Institute, Bhavnagar, India
- 2Academy of Scientific and Innovative Research (AcSIR), CSIR, Ghaziabad, India
Arachis hypogaea (Peanut) is one of the most important cash crops grown for food and oil production. Salinity is a major constraint for loss of peanut productivity, and halotolerant plant growth promoting bacteria not only enhance plant-growth but also provide tolerance against salt stress. The potential of halotolerant bacterium Stenotrophomonas maltophilia BJ01 isolated from saline-soil was explored to enhance the growth of peanut plants under salt stress conditions. Interaction of S. maltophilia BJ01 enhances the growth of the peanut plants and protects photosynthetic pigments under salt stress. Lower electrolyte leakage (about 20%), lipid peroxidation (2.1 μmol g–1 Fw), proline (2.9 μg mg–1 Fw) content and H2O2 (55 μmol g–1 Fw) content were observed in plants, co-cultivated with PGPR compared to untreated plants under stress condition. The growth hormone auxin (0.4 mg g–1 Fw) and total amino acid content (0.3 mg g–1 Fw) were enhanced in plants co-cultivated with PGPR under stress conditions. Overall, these results indicate the beneficial effect of S. maltophilia BJ01 on peanut plants under salt (100 mM NaCl) stress conditions. In conclusion, bacterium S. maltophilia BJ01 could be explored further as an efficient PGPR for growing legumes especially peanuts under salt stress conditions. However, a detailed agronomic study would be needed to ascertain its commercial role.
Introduction
Soil salinity adversely affects the system of the plants at the physiological, biochemical, and molecular levels (Roy et al., 2014). Salinity causes osmotic stress, nutrient imbalance/unavailability, reduction of photosynthesis, ion toxicity, generation of reactive oxygen species (ROS), and ethylene (stress hormone) (Alexander et al., 2019a). Around the world, approximately 77 million hectares (Mha) of agricultural land is affected by salt (Arora, 2017). In India, a total of 9.38 Mha is affected by salinity, while specifically the Gujarat state has a significant share of the salinity-affected area of about 2.23 Mha (Srivastava et al., 2019). Arid and semiarid regions of the world are more affected by salinity due to inadequate rain and agricultural practices (Glick et al., 2007). Among crop plants, cereals and legumes are the most sensitive to salt. In legumes, salt affects the nodulation process and finally the nitrogen fixation (Ramana et al., 2012). Even 100 mM of salt is enough to inhibit nodule formation (Dardanelli et al., 2009). Salt creates a hindrance in Ca absorption, which in turn affects the growth of roots and root hair, hence providing an additional mechanism to hinder nodule formation (Bouhmouch et al., 2005). Peanut (Arachis hypogaea L.) is an important oil crop that is used for food, fodder, and industrial raw material. India in particular has been reported to have the second largest peanut production after China (Fabra et al., 2010).
To tackle the effects of salinity on crops and enhance productivity, several methods are employed, including but not limited to good agricultural practices, genetic manipulation of crops to make them resistant to salt, improvement of the agricultural soil, and irrigation water use. Application of halophilic/halotolerant plant growth-promoting bacteria in stressed soil is the most useful and environmentally friendly approach to increase the productivity and health of crops as well as enhance the soil system in the long term (Alexander et al., 2019a; Fazeli-Nasab and Sayyed, 2019). Plant roots secrete various nutrient substances (∼40% of photosynthetic products) known as root exudates, that play a significant role in the attachment and growth of various endophytic and free-living bacteria (Wang et al., 2016). Some of these bacteria enhance the plant growth and health even under stress conditions. These bacteria are known as plant growth-promoting rhizobacteria (PGPR) (Cook et al., 1995; Alexander et al., 2019b). PGPR enhance the plant growth and development in several ways, including via nitrogen fixation, phosphate solubilization, production of phytohormones, ACC deaminase activity, production of exopolysaccharide (EPS), priming the plant immunity (induced systemic resistance; ISR), acting as a biocontrol agent, and increasing the plant antioxidant enzymes that are produced under stress conditions, such as the ascorbate peroxidase (APX), the catalase (CAT), and the glutathione reductase (GR) (Kloepper et al., 2004; Arevalo-Ferro et al., 2005; Yang et al., 2009: Upadhyay et al., 2012; Bal et al., 2013; Bhargava et al., 2017; Sarkar et al., 2018).
Stenotrophomonas is a gram-negative, yellow-pigmented bacillus, which is a member of the gamma-Proteobacteria class (Moore et al., 1997). It is either free-living or endophytic and is associated with many plant species (Egamberdieva et al., 2016). Different species of Stenotrophomonas have previously been reported for their ability to promote plant growth (Ryan et al., 2009; Berg et al., 2010; Alavi et al., 2013; Singh and Jha, 2017). Egamberdieva et al. (2011) and Singh et al. (2013) have isolated Stenotrophomonas stains from high salinity soils. Members of this species can survive in high salt concentrations because of the production of compatible solutes, especially glucosyl glycerol (GG) and trehalose, which also help the plant to survive in harsh environmental conditions (Alavi et al., 2013). Stenotrophomonas maltophilia BJ01 was isolated from the rhizosphere of Cyperus laevigatus L., which was grown at the coastal region of Dwarka, India and submitted to the Indian marine microbial culture collection of CSMCRI, Bhavnagar with culture collection number IMMCC255 S. maltophilia BJ01 grew in an environment that contained up to 4% NaCl (unpublished data) and it was shown to possess the nifH gene (Singh et al., 2013). Quorum quenching (QQ) and antibiofilm activity of the strain has been reported, hence further supporting its ability to promote plant growth and biocontrol against various plant pathogenic bacteria. It can therefore be employed as part of a strategy that enhances plant survival under harsh growth conditions (Singh et al., 2013). We have also previously reported the nitrogen fixing ability of S. maltophilia BJ01 and its potential to promote plant growth and specifically support peanut plant growth under conditions where N2 is lacking (Alexander et al., 2019b).
The nitrogen fixing ability of legumes is adversely affected by soil salinity that hinders nodule formation, low microbial diversity around root which maintains the holobiome of plant hence halotolerant rhizobacterial species which can naturally survive in saline soils can be useful in agriculture especially in saline soils (Hamaoui et al., 2001; Dardanelli et al., 2008; Egamberdieva, 2011; Patel et al., 2012; Etesami and Beattie, 2018). Recently there are a few studies which shows the positive effect of PGPR on legumes under salt stress. The salt tolerance capacity of soybean was elevated when plants co-cultivated with halotolerant bacteria under 200 mM NaCl stress (Khan et al., 2019). The bacteria Bacillus megaterium NRCB001, B. subtilis subsp. subtilis NRCB002 and B. subtilis NRCB003 isolated from rice rhizosphere showed the plant growth promoting potential under salt stress (130 mM NaCl) when co-cultivated with Medicago sativa (alfalfa) (Zhu et al., 2020). Bacillus megaterium AL-18, B. cereus AL-19 (PGPR isolated from Tamarix ramosissima) improved the growth of Phaseolus vulgaris under salt stress (Abdelmoteleb and Gonzalez-Mendoza, 2020). This study aims to assess the plant growth-promoting attributes of a halophytic bacterium, namely S. maltophilia BJ01, and how these affect the growth of peanut plants under salt stress conditions.
Materials and Methods
Plant Material and Bacterial Interaction
Seeds of Arachis hypogaea cv. GG 20 were collected from the Gujarat Seed Corporation, Sihor, Gujarat, India. The seeds were surface sterilized according to the previously optimized protocol (Alexander et al., 2019b). In brief, the seeds were washed in 70% ethanol for 2 min and submerged in 0.1% HgCl2 for 10 min followed by washing with double autoclaved Milli-Q water 4-5 times to remove any traces of HgCl2. Sterilized seeds were placed in small tissue culture bottles (50 mL) containing sterilized cotton soaked with 1/2 MS (Murashige & Skoog) media in the bottom and kept in the dark for 2-3 days for germination. After 7 days of germination, seedlings of equal size were transferred to the hydroponics culture with the help of floating thermocol disks in 500 mL beaker containing 1/2 MS media. The plantlets were allowed to acclimatize for seven days. The bacterial inoculum was prepared according to the previously reported protocol Alexander et al. (2019b). In brief, For the bacterial inoculum preparation, the bacterial strain was streaked on DYGS agar plate (dextrose 1.0 g L–1; malate 1.0 g L–1; peptone 1.5 g L–1; yeast extract 2.0 g L–1; MgSO4.7H2O 0.5 g L–1; L-glutamic acid 1.5 g L–1; pH 6.0) from glycerol stock stored in -80°C and incubated for 16 hrs at 30°C, followed by subculture in 5 mL DYGS broth media overnight at 30°C and 180 rpm in an incubator shaker. The overnight grown culture was reinoculated in 150 mL of DYGS medium, and the culture was centrifuged at 4000 × g for 10 min once the bacterial growth reached the OD600 nm = 0.6. The supernatant was discarded, and the pellet was re-suspended in 1/2 MS media before co-cultivation with the plant.
Following acclimatization, plants were divided into four groups: (1) without bacterial inoculum and salt stress; (2) with bacterial inoculum and without salt stress; (3) without bacterial inoculum and with 100 mM salt stress; (4) with bacterial inoculum and 100 mM salt stress. All 4 sets were supplemented with 300 mL of 1/2 MS media and were grown in a culture room at 25 ± 2°C under a 16-h/8-h light/dark cycle (light intensity 170 ± 25 μmol m–2 s–1) for another 14 days. The media and inoculum in each plant were changed every seven days, and changes in morphology were recorded. The day that the plants were inoculated with the bacterium was considered to be day zero. After 14 days of stress, the root length, the shoot length, the fresh weight and the dry weight of the plants were recorded and samples were harvested for further analysis.
Chlorophyll Estimation
Total chlorophyll contents of leaf tissues were estimated according to the method given by Arnon (1949) in which leaf tissue (100 mg) was crushed with the help of mortar pestle in 80% acetone and incubated for 6 h in the dark. This was subsequently centrifuged at 10000 × g and the supernatant was pooled out. Absorbance was recorded at 663 and 645 nm. Total chlorophyll contents were calculated using the following equations:
Electrolyte Leakage
Leaves from the distal end of the primary branch were harvested and washed thoroughly with deionized water to remove surface adhered electrolytes. Samples were kept in 10 mL falcons (Eppendorf, United States) containing double distilled water and kept at room temperature on a rotary shaker for 24 h and electrical conductivity (EC) of this water (L1) was measured in μScm–1 using a conductivity meter (Seven Easy, Mettler Toledo, United States). These samples were autoclaved at 120°C for 20 min, cooled at room temperature (RT), and electrical conductivity (L2) was determined (Lutts et al., 1996). The electrolyte leakage was calculated by the following equation:
Membrane Stability Index
Leaves of the same age and size were harvested from the primary branch, they were washed adequately and they were kept in 10 mL vials that were placed on a shaker for 24 h. The EC was subsequently recorded. These samples were put in the water bath (Julabo) at 40°C for 30 min and cooled at RT, and the EC was measured (L1). The same samples were boiled off at 100°C for 20 min, and the EC (L2) of the cooled samples was recorded to calculate the MSI (Jha et al., 2013). The following equation was used for the calculation:
Proline Content
The proline estimation was done as per Bates et al. (1973). 100 mg of plant leaf samples were crushed in liquid nitrogen and extracted in chilled sulphosalicylic acid (SSA). An equal volume of extract and ninhydrin reagent were mixed and incubated at 100°C for 1 h. After cooling the samples in ice bath, toluene was added in the reaction mixture, followed by vortexing and centrifugation. The upper phase was collected, and absorbance was taken at 520 nm. The proline content was calculated by using the standard curve of the known amount of proline.
Total Amino Acid Content
Plant leaf samples (100 mg) were extracted with 80% chilled ethanol. This extract was treated with an equal volume of 0.2 M citrate buffer (pH 5) and 1% ninhydrin reagent. The tubes containing reaction mixture were heated at 95°C in a water bath for 15 min. After cooling, the samples were centrifuged, and the absorbance was read at 570 nm (Patel et al., 2016).
Auxin Content
For the auxin estimation, the extract of leaf samples was prepared in 95% chilled ethanol, and the reaction was carried out further only in ice. The extract was mixed with a double amount of Salkowski reagent and was kept in the dark for 20 min. The absorbance was recorded at 535 nm (Andreae and Van Ysselstein, 1960). The total auxin amount was calculated by a standard curve drawn with the known concentration of indole acetic acid (IAA).
Total H2O2 Contents
Extract of 100 mg leaf samples was prepared in 80% ice-cold acetone, and hydrogen peroxide was quantified by the modified method (Mukherjee and Choudhuri, 1983). The absorbance was measured at 415 nm. The total H2O2 content was calculated by a standard curve drawn with the known concentration of H2O2.
In vivo Localization of Hydrogen Peroxide and Superoxide Radicals
The hydrogen peroxide and the superoxide radicals in stressed and unstressed plant samples were determined in vivo using a histochemical stain of 3,3- diaminobenzidine (DAB) and nitro-blue tetrazolium (NBT) respectively (Singh et al., 2016). Solutions of DAB and NBT were prepared in 10 mM phosphate buffer (pH 7.8). Fresh leaves were immersed in the freshly prepared DAB or NBT, they were kept in the dark for 2 h, and they were illuminated in white light for DAB (8 h) and NBT (1 h). The blue and brown spots that appeared on the leaves indicated in vivo localization.
Lipid Peroxidation
Lipid peroxidation was estimated, according to Hodges et al., 1999 by quantifying the malondialdehyde (MDA) content. Leaf samples (100 mg) were homogenized in chilled 80% ethanol for extract preparation. The extract was divided into two sets; one set was mixed with an equal volume of thiobarbituric acid reagent (containing TBA; 1 mL of 0.5% w/v prepared in 20% w/v TCA); another set was mixed with an equal volume of TCA (20% w/v). Both sets were incubated at 95°C for 30 min, were cooled at RT, and were centrifuged at 10000 × g for 10 min. The optical density of the supernatant recorded at 440, 532, and 600 nm. MDA content was calculated according to the following equation:
Statistical Analysis
Each group contained five plants, and the experiment was performed three times. Statistical analysis was performed by GraphPad Prism software. All data were subjected to one-way analysis of variance (ANOVA) followed by post hoc Tukey’s test. All values are expressed as the mean ± SE. ‘∗’ denotes P < 0.05; ‘∗∗’ denotes P < 0.01 and ‘∗∗∗’ denotes P < 0.001.
Results and Discussion
Interaction of S. maltophilia BJ01 Enhances the Growth of the Plant Under Salt Stress
Previously we have reported the plant growth promoting potential of S. maltophilia BJ01 under N2 starvation conditions (Alexander et al., 2019b). Here we are evaluating the potential of this bacterial strain under 100 mM salt stress condition. After the interaction of the PGPR strain, BJ01 with the plant under control condition (without salt stress) and stress condition (100 mM NaCl) plant were evaluated for their growth pattern for 14 days. Higher plant growth was observed in the plant treated with the bacteria under salt stress (Figure 1.). The shoot length of the treated plants under salt stress was significantly different from their untreated control. The shoot length of the untreated plant was about 13.4 cm whereas the shoot length of the treated plant was about 16 cm (Figure 2A). There was no significant difference in the root of the untreated and treated plants in salt stress conditions (Figure 2B). Enhanced production of auxin could be possible reason for the shoot elongation. The improved fresh weight (Fw) was observed when plants are grown with the bacteria. Under salt stress conditions, the fresh weight of the untreated plant was 5 g and the fresh weight of the treated plant was about 7 g (Figure 2C). Similarly, improved dry weight (Dw) was observed when the plant treated with bacteria. About 0.7 g and about 0.8 g of dry weight were observed in untreated and treated plants under stress conditions, respectively (Figure 2D).
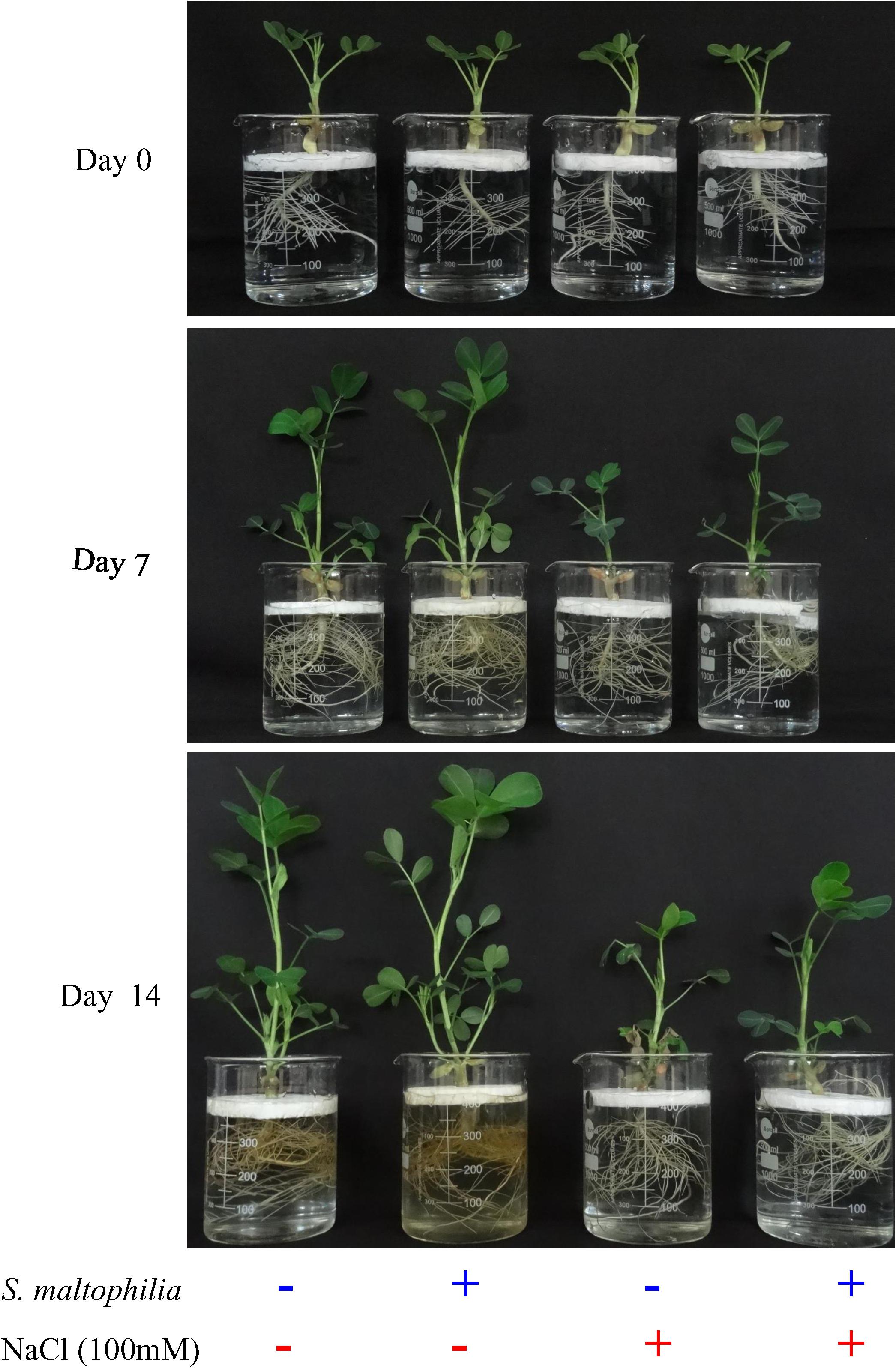
Figure 1. Morphological difference in inoculated and uninoculated plants. Plant grown without NaCl considered as control condition and plant under 100 mM salt considered as stressed conditions. “+” represents the presence of bacteria or NaCl, whereas “-” represents the absence of NaCl or bacteria.
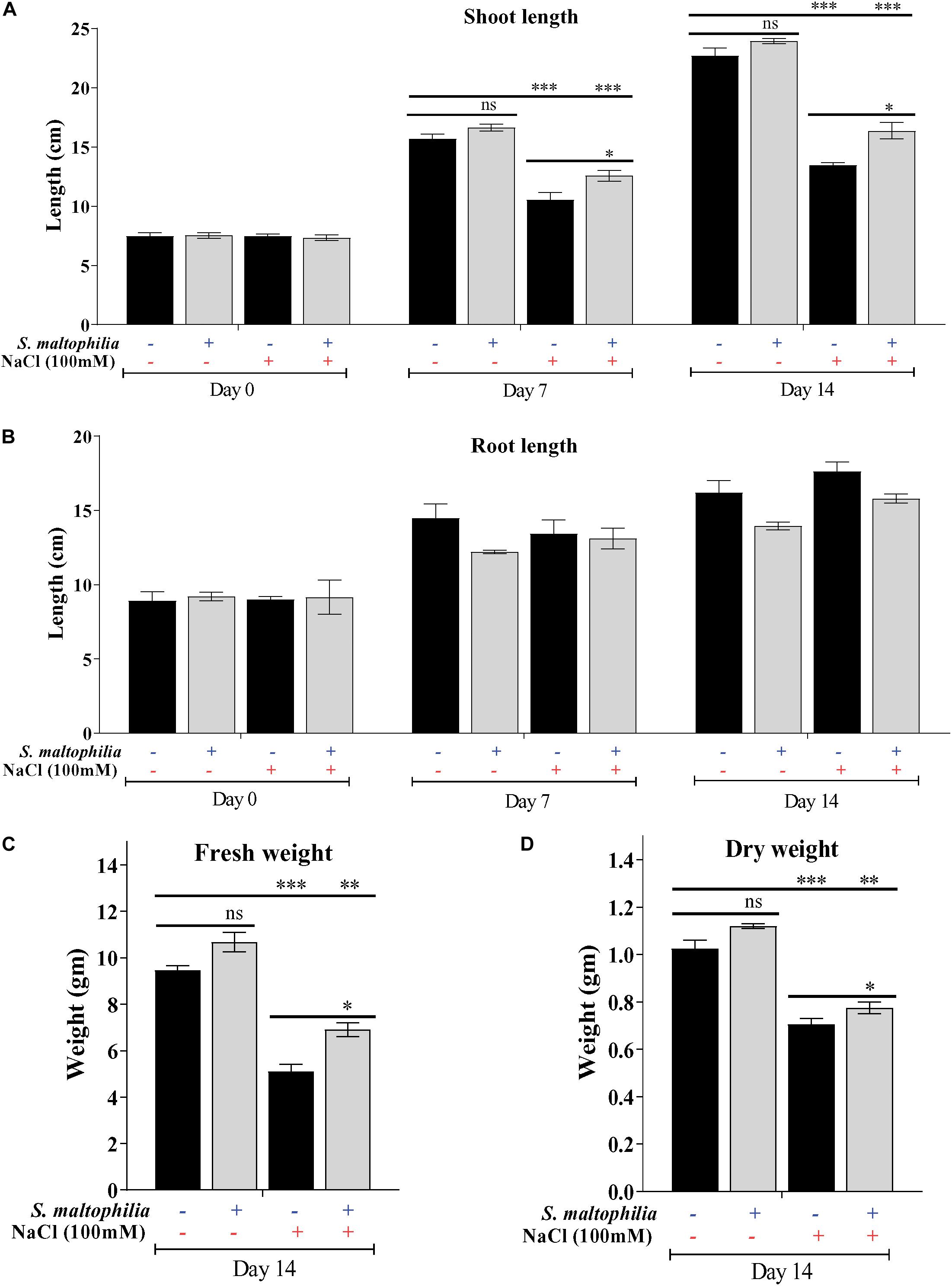
Figure 2. Difference in various growth parameters and comparative analysis. Shoot length (A), root length (B) fresh weight (C) dry weight (D) of control, and stressed plants. Plant grown without NaCl considered as control condition and plant under 100 mM salt considered as stressed conditions. “+” represents the presence of bacteria or NaCl whereas “-” represents the absence of NaCl or bacteria. Bars denote means ± SE. ‘*,’ ‘**,’ ‘***’ indicate significant differences at P < 0.05, P < 0.01 and P < 0.001, respectively and ‘ns’ represents no significant difference.
For survival under abiotic stress, plants generally compromise their growth, physiology, and development because the resources like nutrients and photosynthetic byproducts are used in defense (Egamberdieva et al., 2019). In this study, we observed that the untreated plant (without bacterial interaction) under salt stress plant growth was stunned, shoot length, fresh weight, dry weight reduced drastically. On another set where plants were treated with bacterial inoculum under salt stress showed improved growth (shoot length, fresh weight and dry weight). These observations showed the role of S. maltophilia BJ01 in growth and development under salt stress conditions. Similar results were also reported in which PGPR showed the improved growth in crop Solanum melongena L, Triticum aestivum, and Chenopodium quinoa under salt stress (Fu et al., 2010; Orhan, 2016; Yang et al., 2016). Bacterial inoculation reduces the salt stress and showed the improved plant growth and phosphate uptake in Phaseolus vulgaris (Abdelmoteleb and Gonzalez-Mendoza, 2020). The co-cultivation of plant growth-promoting rhizobacteria also showed the improved plant growth specially the dry weight of the Medicago sativa (alfalfa) under salinity stress (Zhu et al., 2020).
Photosynthetic Pigment of Arachis hypogaea Was Protected by S. maltophilia BJ01 Under Salt Stress
Salt stress affects the plant cells physiologically and due to osmotic pressure, cells get dehydrated which results in stomatal closure, reduced cell growth and reduced chlorophyll content in plants (Shannon and Grieve, 1998). The peanut plants were grown under salt stress (100 mM) for 14 days. The leaves turned pale and necrosis in leaves were observed which are the sign of chlorophyll degradation and senescence. When the plants are grown with the S. maltophilia BJ01 under salt stress, the plant have much healthy leaves and higher chlorophyll concentration. The chlorophyll a, chlorophyll b, and total chlorophyll contents were 0.2 mg g–1 Fw, 0.3 mg g–1 Fw, and 0.5 mg g–1 Fw respectively in the plant without bacteria (Figures 3A–C). The chlorophyll a, chlorophyll b and total chlorophyll contents were 0.4 mg g–1 Fw, 0.3 mg g–1 Fw, and 0.7 mg g–1 Fw respectively in a plant grown under salt stress with bacteria (Figures 3A–C). The positive effect of rhizospheric bacteria on chlorophyll content and photosynthetic ability of host plant under saline stress condition was also reported in Zea mays and Oryza sativa (Nadeem et al., 2007; Rojas-Tapias et al., 2012; Yoolong et al., 2019). The protection of the photosynthetic pigment under salt stress was also reported in common bean (Phaseolus vulgaris) by rhizospheric bacteria (Abdelmoteleb and Gonzalez-Mendoza, 2020).
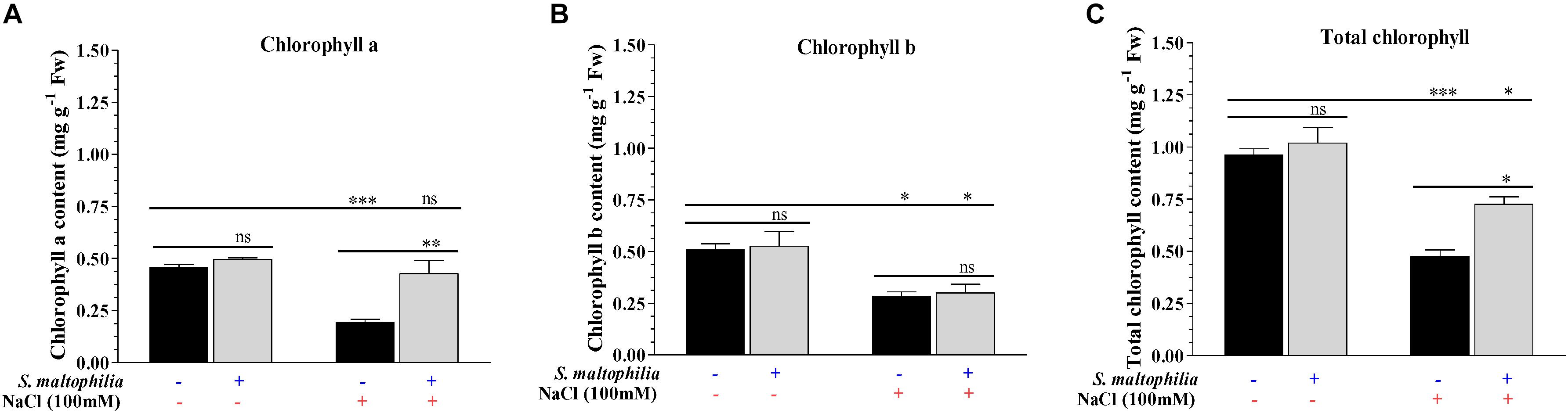
Figure 3. Estimation of photosynthetic pigments. Chlorophyll a contents (A), chlorophyll b contents (B) and total chlorophyll content (C) of inoculated and uninoculated plants. Plant grown without NaCl considered as control condition and plant under 100 mM salt considered as stressed conditions. “+” represents the presence of bacteria or NaCl whereas “-” represents the absence of NaCl or bacteria. Bars denote means ± SE. ‘*,’ ‘**,’ ‘***’ indicate significant differences at P < 0.05, P < 0.01, and P < 0.001, respectively and ‘ns’ represents no significant difference.
S. maltophilia BJ01 Modulates the Plant Physiology After Interaction Under Salt Stress
The plant grown under salt stress with bacteria showed reduced electrolyte leakage and high membrane integrity compared to plants grown under salt stress without bacteria. About 42% of electrolyte leakage was found in the plant grown in salt stress without bacteria and about 19% electrolyte leakage was found in the plant grown in salt stress with bacteria (Figure 4A). The membrane stability of the plant under salt stress without bacteria was about 40% whereas plants grown under salt stress with bacteria were about 77% (Figure 4B). Under salinity stress plant cells has a higher concentration of Na+ and Cl– and low concentration of K+; this ionic imbalance destabilizes/damages the cell membranes (due to Ca2+ displacement) and causes the leakage of electrolytes from cell sap (Hussain et al., 2008). The interaction between bacteria and plants attenuate the deleterious effect on plant cells which occurs due to high salt concentration and helps cells to maintain its structure and survival. Similar results were also obtained in Cajanus cajan (L.), where electrolyte leakage is higher in salt condition and it reduced by the application of arbuscular mycorrhiza (Garg and Manchanda, 2009). Reduction of the electrolyte leakage in chickpea (Cicer arietinum L.) under salt stress by Azospirillum lipoferum FK1 reported by El-Esawi et al. (2019). These results are suggesting that the S. maltophilia BJ01 reducing the salt stress on the plant which results the improved membrane stability.
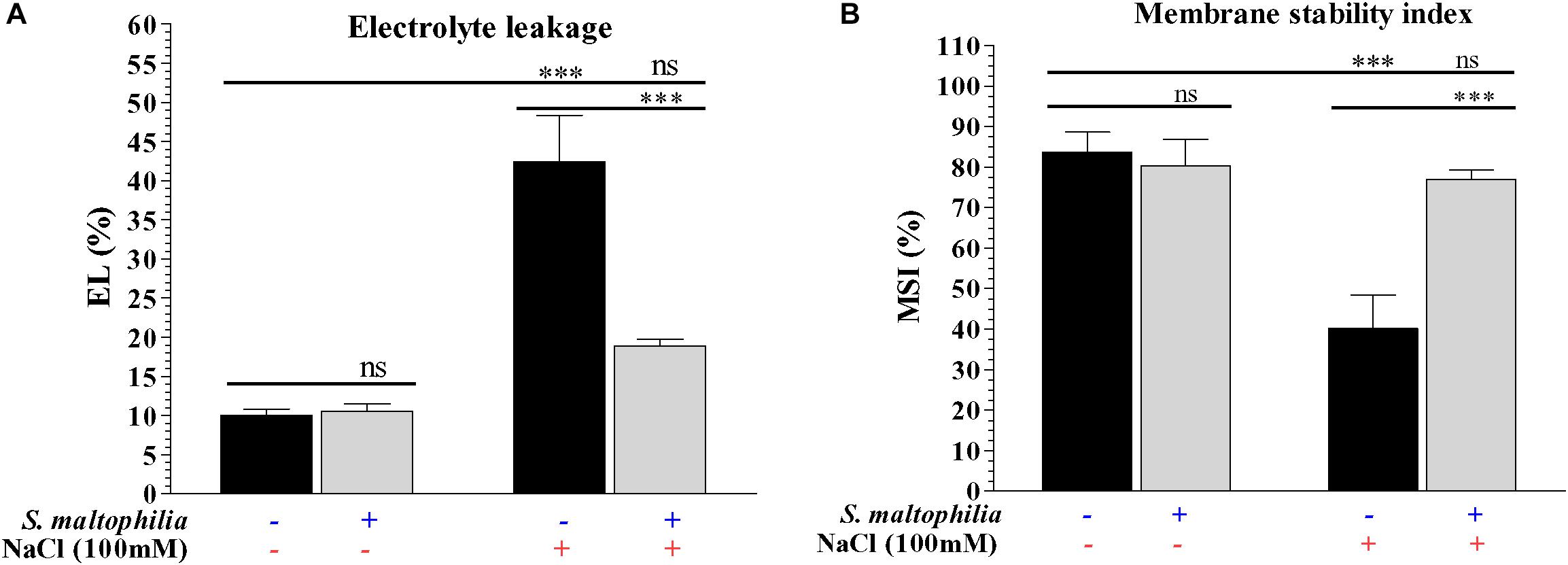
Figure 4. Measurement of physiological parameters. Membrane Stability Index (MSI) (A) and electrolyte leakage (EL) of control and stressed plants (B). Plant grown without NaCl considered as control condition and plant under 100 mM salt considered as stressed conditions. “+” represents the presence of bacteria or NaCl whereas “-” represents the absence of NaCl or bacteria. Bars denote means ± SE. ‘*,’ ‘**,’ ‘***’ indicate significant differences at P < 0.05, P < 0.01, and P < 0.001, respectively and ‘ns’ represents no significant difference.
Co-cultivation of S. maltophilia BJ01 Leads to the Better Biochemical Performance of Arachis hypogaea Under Salt Stress
The plant grown with the bacteria under salt stress showed lower proline content and higher total amino acid accumulation in the plant in comparison to the control counterpart. The proline content of the plant grown under salt stress without bacteria was about 3.2 μg mg–1 Fw and with bacteria was about 2.9 μg mg–1 Fw (Figure 5A). The total amino acid concentration was quantified in plants. About 0.23 mg g–1 Fw was found to present in plant grown in salt stress without bacteria and about 0.31 mg g–1 Fw was present in the plant grown with bacteria (Figure 5B). Maintenance of turgidity and viscosity in cells is a significant challenge for plants under salt stress. To cop up with this condition plants synthesize osmolytes/osmoprotectants which help plant to survive under harsh conditions and maintain water retention inside the cells (Zulfiqar et al., 2020). Amino acids like valine, isoleucine, proline, aspartic acid, etc., act as osmoprotectants and generate in high concentration by plants under salt condition (Burg and Ferraris, 2008). Proline is one of the amino acids which acts as osmoprotectant under various abiotic stress conditions and scavenger for hydroxyl free radicles (Claussen, 2005; Peng et al., 2008). The presence of lower proline content in the plant with the bacteria reflects the role of S. maltophilia BJ01 to helping the plant to overcome with the salt stress. A higher amount of total amino acids (TAA) content in plants having salt stress and bacterial inoculation shows the role of bacteria further strengthen the plant system under a saline environment. Han and Lee (2005) also found that the interaction of Glycine max with Bradyrhizobium japonicum under salt stress leads to lower production of the proline. To further strengthen our finding that the plant growth promoting rhizobacteria reduces the salt stress on the plants leading the lower production of proline was also reported after interaction of halotolerant rhizobacterium Pseudomonas koreensis MU2 with Soybean (Glycine max L.) under salt stress by Adhikari et al. (2020).
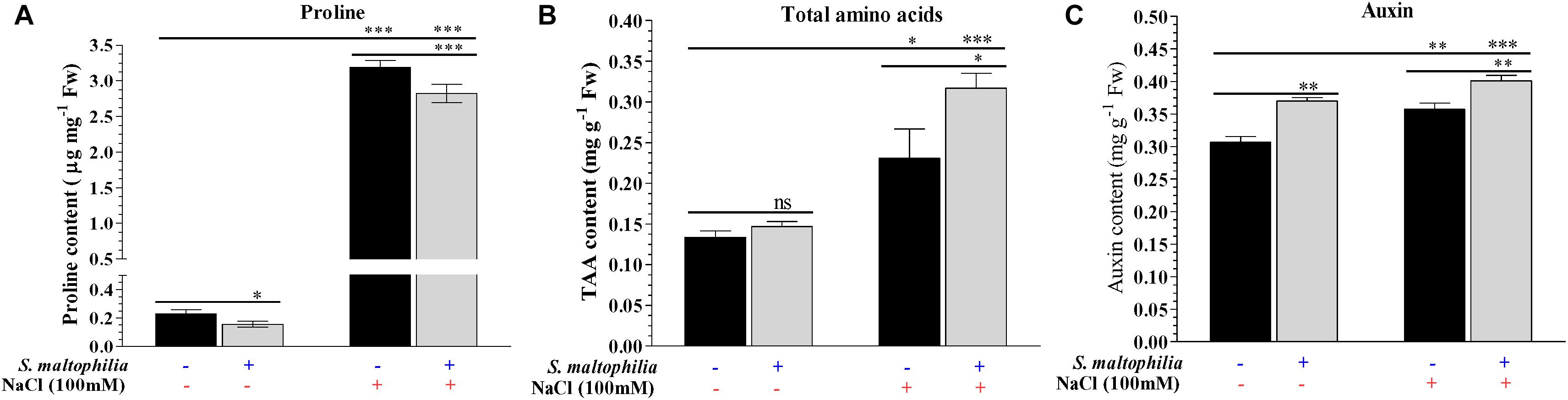
Figure 5. Biochemical changes in plants due to bacterial interaction. Quantification of proline (A), total amino acid (TAA) (B) and auxin (C) concentration of control and treated plants. Plant grown without NaCl considered as control condition and plant under 100 mM salt considered as stressed conditions. “+” represents the presence of bacteria or NaCl whereas “-” represents the absence of NaCl or bacteria. Bars denote means ± SE. ‘*,’ ‘**,’ ‘***’ indicate significant differences at P < 0.05, P < 0.01, and P < 0.001, respectively and ‘ns’ represents no significant difference.
The auxin production in the plant grown under salt stress without bacteria was 0.35 mg g–1 Fw, whereas in the plant treated with bacteria was 0.40 mg g–1 Fw (Figure 5C). Auxins are phytohormone which play a crucial role in the growth, development under stress conditions for plants (Egamberdieva et al., 2017). Indole-3-acetic acid (IAA) is the most common version of auxin found in plants and its concentration decreases in salt stress results in decreased growth of plants (Albacete et al., 2008). In our study, we found that the concentration of auxin decreases in salt stress without bacteria however, in plants with bacterial inoculation concentration of auxin increases. Increment in auxin concentration revealed that the bacterial interaction enhances the auxin synthesis in plants which helps plant for survival and growth under salt stress. This result is in accordance with Tiwari et al. (2011) and Noori et al. (2018).
Reactive Oxygen Species (ROS) Buildup in Arachis hypogea Protected by S. maltophilia BJ01 Interaction Under Salt Stress
Reduced production of hydrogen peroxide was observed in the plant grown with the bacterial in comparison to the plant grown without bacteria in the salt stress condition. About 75 μmol g–1 Fw H2O2 was found in the plant without bacteria under salt stress whereas 55 μmol g–1 Fw was measured in the plant with bacteria under stress condition (Figure 6A). These results were also supported by the in vivo localization of these ROS (superoxide and H2O2) in plant leaves (Figures 7A,B). In stress condition plants overproduce reactive oxygen species (ROS) which act as a signaling molecule for downstream regulation of defense mechanism; this situation called oxidative stress (Demidchik, 2015). Among the ROS, hydrogen peroxide (H2O2) is considered as the most stable molecule (Foyer and Noctor, 2009) and generate high concentration under salt stress (Singh et al., 2016). Lower H2O2 concentration in plants with bacterial inoculation in salt stress shows the beneficial effect of S. maltophilia BJ01 on peanut under stress conditions which reduces the oxidative stress on the plant system. Similar results were also obtained in strawberry plants by rhizobacterial treatment (Arıkan et al., 2020).
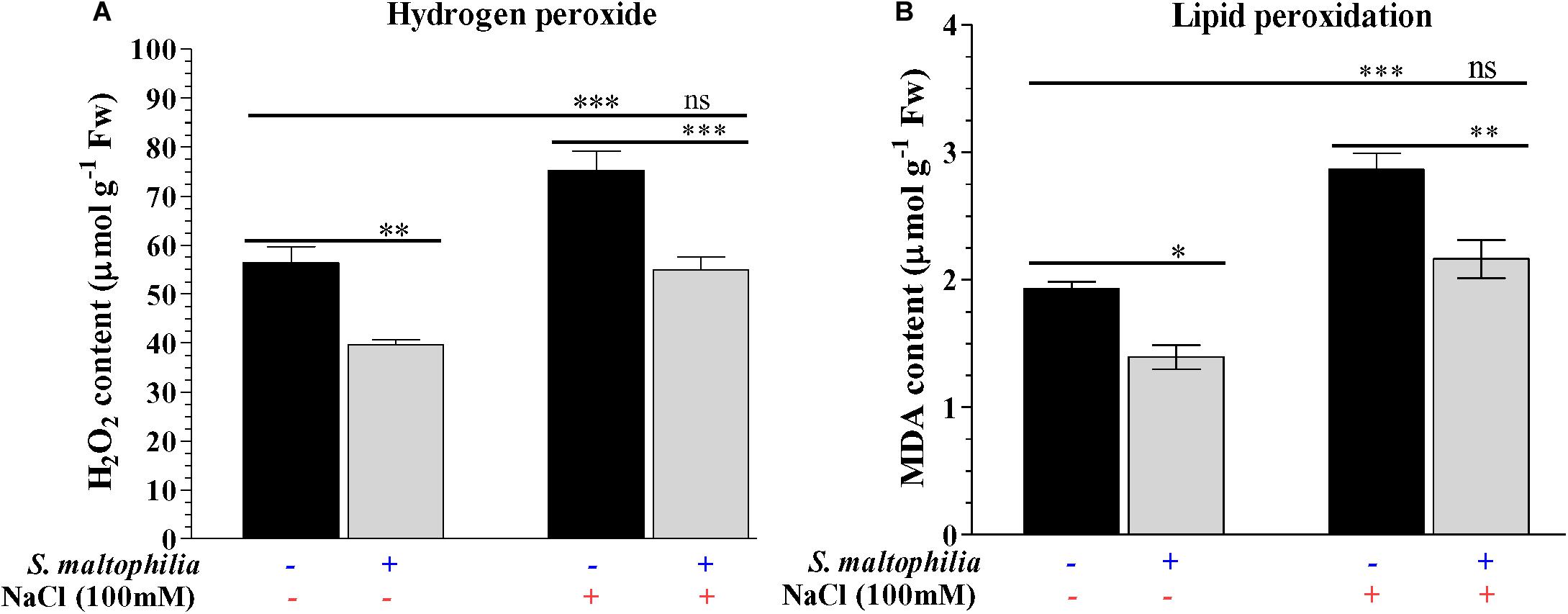
Figure 6. Estimation of reactive oxygen species and lipid peroxidation of the plant. Quantification of hydrogen peroxide (H2O2) (A) and MDA contents (B). Plant grown without NaCl considered as control condition and plant under 100 mM salt considered as stressed conditions. “+” represents the presence of bacteria or NaCl, whereas “-” represents the absence of NaCl or bacteria. Bars denote means ± SE. ‘*,’ ‘**,’ ‘***’ indicate significant differences at P < 0.05, P < 0.01, and P < 0.001, respectively and ‘ns’ represents no significant difference.
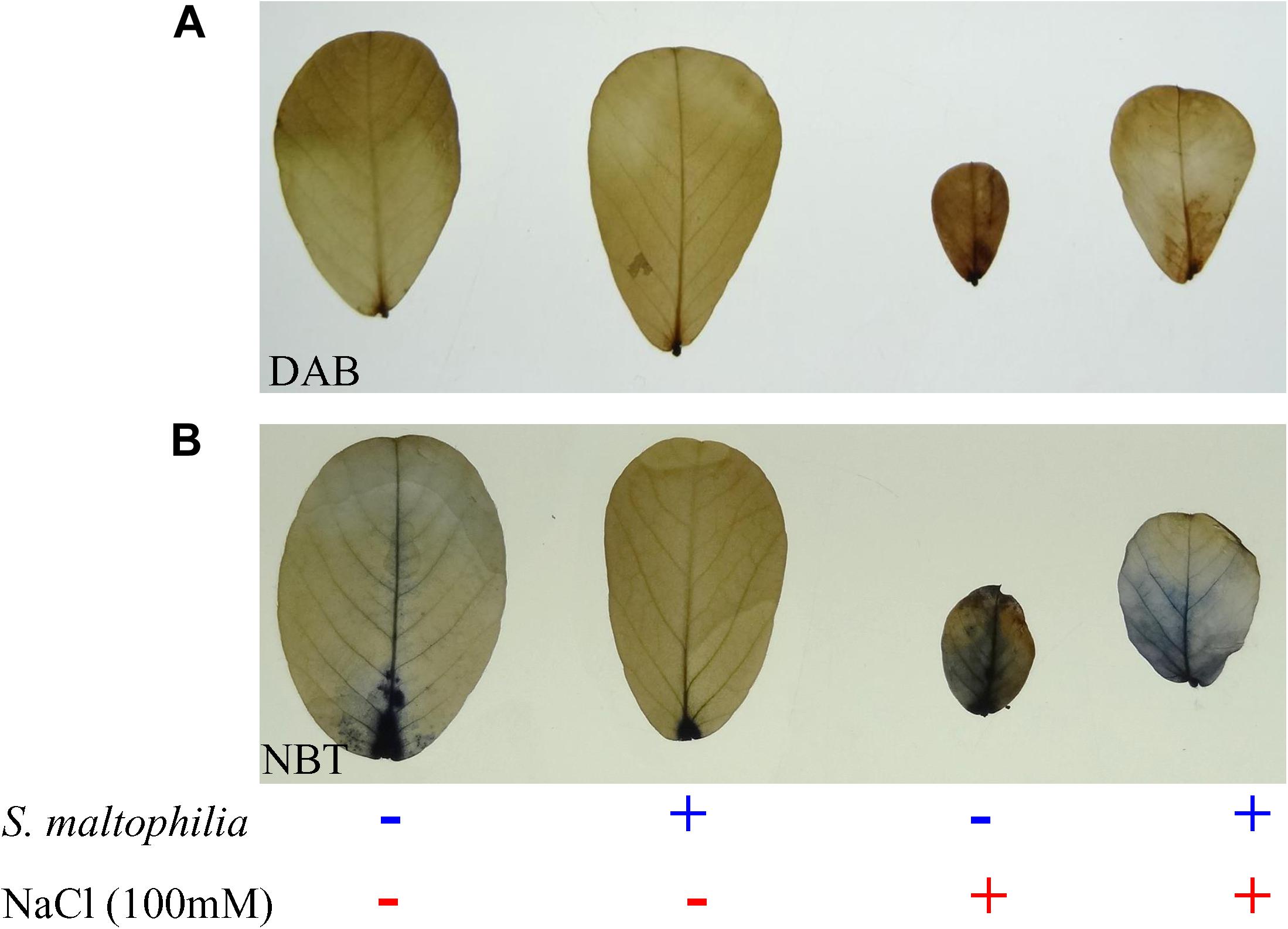
Figure 7. In vivo localization of reactive oxygen species in plant leaves. Staining of peroxide and superoxide free radicals via DAB (A) and NBT (B). Plant grown without NaCl considered as control condition and plant under 100 mM salt considered as stressed conditions. “+” represents the presence of bacteria or NaCl, whereas “-” represents the absence of NaCl or bacteria. Bars denote means ± SE. ‘*,’ ‘**,’ ‘***’ indicate significant differences at P < 0.05, P < 0.01, and P < 0.001, respectively and ‘ns’ represents no significant difference.
The lower production of malondialdehyde (MDA) in the plant grown with the bacteria was observed in the comparison of the plant without bacteria under salt stress. The MDA content 2.8 μmol g–1 Fw was measured in a plant grown without bacteria, whereas 2.1 μmol g–1 Fw was measured in a plant grown with the bacteria under salt stress (Figure 6B). Membrane lipids are highly reactive toward the ROS which results in lipid peroxidation and generates MDA, which is an indicator of membrane disintegration (Hodges et al., 1999; Catalá, 2006; Farmer and Mueller, 2013). More membrane damage causes more production of MDA molecules which in our case reduced by bacterial treatment in a plant under stress condition. Singh and Jha (2016) obtain similar results in wheat inoculated with halotolerant bacteria under stress conditions.
Morpho-Physio-Biochemical Response of Plant Grown With or Without Bacteria Under Control and Stress Conditions
Principal component analysis (PCA) was carried out to extricate the response of the peanut plants under different growth conditions. The bi-plot analysis reveals the differential response of the plant under control and stress conditions when co-cultured with bacteria and without bacteria (Figure 8A). Differential responses to the variables was also observed in the integrated heat-map in different conditions of the plant growth (Figure 8B). The multivariance analysis strongly suggests that the bacterial interaction highly influence the morphology, physiology and biochemistry of the plant.
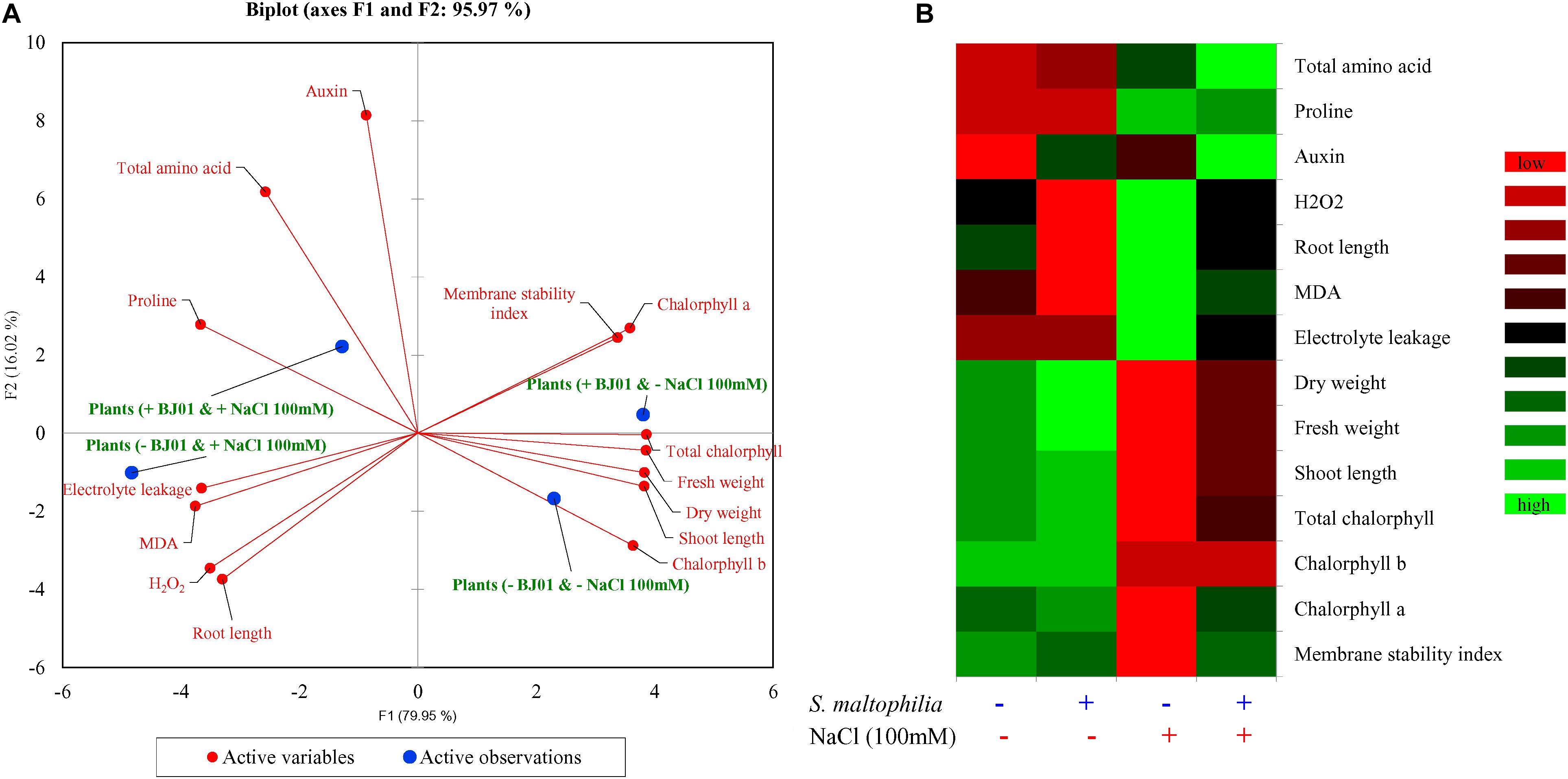
Figure 8. Multivariate data analyses of plant grown with or without bacteria under control and stress conditions. Principal component analysis (A) and integrated heat map (B). Plant grown without NaCl considered as control condition and plant under 100 mM salt considered as stressed conditions. “+” represents the presence of bacteria or NaCl, whereas “-” represents the absence of NaCl or bacteria.
Conclusion
In this study, the beneficial effects of Stenotrophomonas maltophilia BJ01 on Arachis hypogaea GG20 plants under 100 mM salt concentration were evaluated. Here we found that the plant growth promoting rhizobacteria isolated from halotolerant grass species can help the peanut plant to withstand the deleterious effect of salinity by supporting the plant at the morphological, physiological and biochemical level. Inhabitance in harsh conditions and nitrogen fixing ability of this bacterial strain help plants under direct salt stress. To meet up the demand for food for the growing population of the world under various abiotic stress, we need a more sustainable and environmentally friendly method. Thus, this study opens the door for the agricultural application of PGPR to overcome biotic and abiotic stress instead of chemical application. Further studies of genomic, proteomic, and metabolomics of holobiome (plant and associated microbiome) can be a beneficial intervention in this field to understand plant microbe interaction and uncover the mysteries of plant immunity and its survival.
Data Availability Statement
The raw data supporting the conclusions of this article will be made available by the authors, without undue reservation.
Author Contributions
AM conceived and designed the experiments. AA performed the experiments. AA, VS, and AM analyzed the data and wrote the manuscript.
Funding
This work was supported by the SERB-DST (EMR/2016/000538) and CSIR-Young Scientist (YSP-02/2016-17) projects.
Conflict of Interest
The authors declare that the research was conducted in the absence of any commercial or financial relationships that could be construed as a potential conflict of interest.
Acknowledgments
Prof. Bhavanath Jha is thankfully acknowledged for securing initial funds to support this research. Dr. Marianna Almpani, Harvard Medical School, Boston, United States, duly acknowledged for the language editing and proofreading. CSIR-CSMCRI Communication No.: PRIS-85/2020.
References
Abdelmoteleb, A., and Gonzalez-Mendoza, D. (2020). Isolation and identification of phosphate solubilizing Bacillus spp. from Tamarix ramosissima rhizosphere and their effect on growth of phaseolus vulgaris under salinity stress. Geomicrobiol. J. 11, 1–8. doi: 10.1080/01490451.2020.1795321
Adhikari, A., Khan, M. A., Lee, K. E., Kang, S. M., Dhungana, S. K., Bhusal, N., et al. (2020). The halotolerant rhizobacterium-Pseudomonas koreensis MU2 enhances inorganic silicon and phosphorus use efficiency and augments salt stress tolerance in soybean (Glycine max L.). Microorganisms 8:1256. doi: 10.3390/microorganisms8091256
Alavi, P., Starcher, M., Zachow, C., Müller, H., and Berg, G. (2013). Root-microbe systems: the effect and mode of interaction of stress protecting agent (SPA) Stenotrophomonas rhizophila DSM14405T. Front. Plant Sci. 4:141. doi: 10.3389/fpls.2013.00141
Albacete, A., Ghanem, M. E., Martínez-Andújar, C., Acosta, M., Sánchez-Bravo, J., Martínez, V., et al. (2008). Hormonal changes in relation to biomass partitioning and shoot growth impairment in salinized tomato (Solanum lycopersicum L.) plants. J. Exp. Bot. 59, 4119–4131. doi: 10.1093/jxb/ern251
Alexander, A., Mishra, A., and Jha, B. (2019a). “Halotolerant rhizobacteria: a promising probiotic for saline soil-based agriculture,” in Saline Soil-based Agriculture by Halotolerant Microorganisms, eds H. Etesami, V. Kumar, and M. Kumar (Singapore: Springer), 53–73. doi: 10.1007/978-981-13-8335-9_3
Alexander, A., Singh, V. K., Mishra, A., and Jha, B. (2019b). Plant growth promoting rhizobacterium Stenotrophomonas maltophilia BJ01 augments endurance against N2 starvation by modulating physiology and biochemical activities of Arachis hypogea. PLoS One 14:e0222405. doi: 10.1371/journal.pone.0222405
Andreae, W. A., and Van Ysselstein, M. W. (1960). Studies on 3-indoleacetic acid metabolism. VI. 3-Indoleacetic acid uptake and metabolism by pea roots and epicotyls. Plant Physiol. 35:225. doi: 10.1104/pp.35.2.225
Arevalo-Ferro, C., Reil, G., Görg, A., Eberl, L., and Riedel, K. (2005). Biofilm formation of Pseudomonas putida IsoF: the role of quorum sensing as assessed by proteomics. Syst. Appl. Microbiol. 28, 87–114. doi: 10.1016/j.syapm.2004.10.005
Arıkan, Ş, İpek, M., Eşitken, A., Pırlak, L., Dönmez, M. F., and Turan, M. (2020). Plant growth promoting rhizobacteria mitigate deleterious combined effects of salinity and lime in soil in strawberry plants. J. Plant Nutr. 43, 2028–2039. doi: 10.1080/01904167.2020.1766073
Arnon, D. I. (1949). Copper enzymes in isolated chloroplasts. Polyphenoloxidase in Beta vulgaris. Plant Physiol. 24:1. doi: 10.1104/pp.24.1.1
Arora, S. (2017). “Diagnostic properties and constraints of salt-affected soils,” in Bioremediation of Salt Affected Soils: An Indian Perspective, eds Y. P. Singh, A. K. Singh, and S. Arora (Cham: Springer), 41–52. doi: 10.1007/978-3-319-48257-6_2
Bal, H. B., Das, S., Dangar, T. K., and Adhya, T. K. (2013). ACC deaminase and IAA producing growth promoting bacteria from the rhizosphere soil of tropical rice plants. J. Basic Microbiol. 53, 972–984. doi: 10.1002/jobm.201200445
Bates, L. S., Waldren, R. P., and Teare, I. D. (1973). Rapid determination of free proline for water-stress studies. Plant Soil 39, 205–207. doi: 10.1007/BF00018060
Berg, G., Egamberdieva, D., Lugtenberg, B., and Hagemann, M. (2010). “Symbiotic plant–microbe interactions: stress protection, plant growth promotion, and biocontrol by Stenotrophomonas,” in Symbioses and Stress, eds M. Grube and J. Seckbach (Dordrecht: Springer), 445–460. doi: 10.1007/978-90-481-9449-0_22
Bhargava, P., Singh, A. K., and Goel, R. (2017). “Microbes: bioresource in agriculture and environmental sustainability,” in Plant-Microbe Interactions in Agro-Ecological Perspectives, eds H. B. Singh, D. P. Singh, and R. Prabha (Singapore: Springer), 361–376. doi: 10.1007/978-981-10-5813-4_18
Bouhmouch, I., Souad-Mouhsine, B., Brhada, F., and Aurag, J. (2005). Influence of host cultivars and Rhizobium species on the growth and symbiotic performance of Phaseolus vulgaris under salt stress. J. Plant Physiol. 162, 1103–1113. doi: 10.1016/j.jplph.2004.12.003
Burg, M. B., and Ferraris, J. D. (2008). Intracellular organic osmolytes: function and regulation. J. Biol. Chem. 283, 7309–7313. doi: 10.1074/jbc.R700042200
Catalá, A. (2006). An overview of lipid peroxidation with emphasis in outer segments of photoreceptors and the chemiluminescence assay. Int. J. Biochem. Cell Biol. 38, 1482–1495. doi: 10.1016/j.biocel.2006.02.010
Claussen, W. (2005). Proline as a measure of stress in tomato plants. Plant Sci. 168, 241–248. doi: 10.1016/j.plantsci.2004.07.039
Cook, R. J., Thomashow, L. S., Weller, D. M., Fujimoto, D., Mazzola, M., Bangera, G., et al. (1995). Molecular mechanisms of defense by rhizobacteria against root disease. Proc. Natl. Acad. Sci. U.S.A. 92, 4197–4201. doi: 10.1073/pnas.92.10.4197
Dardanelli, M. S., de Cordoba, F. J. F., Espuny, M. R., Carvajal, M. A. R., Díaz, M. E. S., Serrano, A. M. G., et al. (2008). Effect of Azospirillum brasilense coinoculated with Rhizobium on Phaseolus vulgaris flavonoids and Nod factor production under salt stress. Soil Biol. Biochem. 40, 2713–2721. doi: 10.1016/j.soilbio.2008.06.016
Dardanelli, M. S., González, P. S., Medeot, D. B., Paulucci, N. S., Bueno, M. Á, and Garcia, M. B. (2009). Effects of peanut rhizobia on the growth and symbiotic performance of Arachis hypogaea under abiotic stress. Symbiosis 47, 175–180. doi: 10.1007/BF03179977
Demidchik, V. (2015). Mechanisms of oxidative stress in plants: from classical chemistry to cell biology. Environ. Exp. Bot. 109, 212–228. doi: 10.1016/j.envexpbot.2014.06.021
Egamberdieva, D. (2011). Survival of Pseudomonas extremorientalis TSAU20 and P. chlororaphis TSAU13 in the rhizosphere of common bean (Phaseolus vulgaris) under saline conditions. Plant Soil Environ. 57, 122–127. doi: 10.17221/316/2010-PSE
Egamberdieva, D., Jabborova, D., and Berg, G. (2016). Synergistic interactions between Bradyrhizobium japonicum and the endophyte Stenotrophomonas rhizophila and their effects on growth, and nodulation of soybean under salt stress. Plant Soil 405, 35–45. doi: 10.1007/s11104-015-2661-8
Egamberdieva, D., Kucharova, Z., Davranov, K., Berg, G., Makarova, N., Azarova, T., et al. (2011). Bacteria able to control foot and root rot and to promote growth of cucumber in salinated soils. Biol. Fertil. Soils 47, 197–205. doi: 10.1007/s00374-010-0523-3
Egamberdieva, D., Wirth, S., Bellingrath-Kimura, S. D., Mishra, J., and Arora, N. K. (2019). Salt-tolerant plant growth promoting rhizobacteria for enhancing crop productivity of saline soils. Front. Microbiol. 10:2791. doi: 10.3389/fmicb.2019.02791
Egamberdieva, D., Wirth, S. J., Alqarawi, A. A., Abd_Allah, E. F., and Hashem, A. (2017). Phytohormones and beneficial microbes: essential components for plants to balance stress and fitness. Front. Microbiol. 8:2104. doi: 10.3389/fmicb.2017.02104
El-Esawi, M. A., Al-Ghamdi, A. A., Ali, H. M., and Alayafi, A. A. (2019). Azospirillum lipoferum FK1 confers improved salt tolerance in chickpea (Cicer arietinum L.) by modulating osmolytes, antioxidant machinery and stress-related genes expression. Environ. Exp. Bot. 159, 55–65. doi: 10.3390/genes10020163
Etesami, H., and Beattie, G. A. (2018). Mining halophytes for plant growth-promoting halotolerant bacteria to enhance the salinity tolerance of non-halophytic crops. Front. Microbiol. 9:148. doi: 10.3389/fmicb.2018.00148
Fabra, A., Castro, S., Taurian, T., Angelini, J., Ibañez, F., Dardanelli, M., et al. (2010). Interaction among Arachis hypogaea L.(peanut) and beneficial soil microorganisms: how much is it known? Crit. Rev. Microbiol. 36, 179–194. doi: 10.3109/10408410903584863
Farmer, E. E., and Mueller, M. J. (2013). ROS-mediated lipid peroxidation and RES-activated signaling. Annu. Rev. Plant Biol. 64, 429–450. doi: 10.1146/annurev-arplant-050312-120132
Fazeli-Nasab, B., and Sayyed, R. Z. (2019). “Plant growth-promoting rhizobacteria and salinity stress: a journey into the soil,” in Plant Growth Promoting Rhizobacteria for Sustainable Stress Management, eds N. K. Arora, R. Z. Sayyed, and M. S. Reddy (Singapore: Springer), 21–34. doi: 10.1007/978-981-13-6536-2_2
Foyer, C. H., and Noctor, G. (2009). Redox regulation in photosynthetic organisms: signaling, acclimation, and practical implications. Antioxid. Redox Signal. 11, 861–905. doi: 10.1089/ars.2008.2177
Fu, Q., Liu, C., Ding, N., Lin, Y., and Guo, B. (2010). Ameliorative effects of inoculation with the plant growth-promoting rhizobacterium Pseudomonas sp. DW1 on growth of eggplant (Solanum melongena L.) seedlings under salt stress. Agric. Water Manag. 97, 1994–2000. doi: 10.1016/j.agwat.2010.02.003
Garg, N., and Manchanda, G. (2009). Role of arbuscular mycorrhizae in the alleviation of ionic, osmotic and oxidative stresses induced by salinity in Cajanus cajan (L.) Millsp. (pigeon pea). J. Agron. Crop Sci. 195, 110–123. doi: 10.1111/j.1439-037x.2008.00349.x
Glick, B. R., Todorovic, B., Czarny, J., Cheng, Z., Duan, J., and McConkey, B. (2007). Promotion of plant growth by bacterial ACC deaminase. Crit. Rev. Plant Sci. 26, 227–242. doi: 10.1080/07352680701572966
Hamaoui, B., Abbadi, J., Burdman, S., Rashid, A., Sarig, S., and Okon, Y. (2001). Effects of inoculation with Azospirillum brasilense on chickpeas (Cicer arietinum) and faba beans (Vicia faba) under different growth conditions. Agronomie 21, 553–560. doi: 10.1051/agro:2001144
Han, H. S., and Lee, K. D. (2005). Physiological responses of soybean-inoculation of Bradyrhizobium japonicum with PGPR in saline soil conditions. Res. J. Agric. Biol. Sci. Res. J. Agric. Biol. Sci. 1, 216–221.
Hodges, D. M., DeLong, J. M., Forney, C. F., and Prange, R. K. (1999). Improving the thiobarbituric acid-reactive-substances assay for estimating lipid peroxidation in plant tissues containing anthocyanin and other interfering compounds. Planta 207, 604–611. doi: 10.1007/s004250050524
Hussain, T. M., Chandrasekhar, T., Hazara, M., Sultan, Z., Saleh, B. K., and Gopal, G. R. (2008). Recent advances in salt stress biology–a review. Biotechnol. Mol. Biol. Rev. 3, 008–013.
Jha, B., Mishra, A., Jha, A., and Joshi, M. (2013). Developing transgenic Jatropha using the SbNHX1 gene from an extreme halophyte for cultivation in saline wasteland. PLoS One 8:e0071136. doi: 10.1371/journal.pone.0071136
Khan, M. A., Asaf, S., Khan, A. L., Adhikari, A., Jan, R., Ali, S., et al. (2019). Halotolerant rhizobacterial strains mitigate the adverse effects of NaCl stress in soybean seedlings. BioMed Res. Int. 2019:9530963. doi: 10.1155/2019/9530963
Kloepper, J. W., Ryu, C. M., and Zhang, S. (2004). Induced systemic resistance and promotion of plant growth by Bacillus spp. Phytopathology 94, 1259–1266. doi: 10.1094/PHYTO.2004.94.11.1259
Lutts, S., Kinet, J. M., and Bouharmont, J. (1996). NaCl-induced senescence in leaves of rice (Oryza sativa L.) cultivars differing in salinity resistance. Ann. Bot. 78, 389–398. doi: 10.1006/anbo.1996.0134
Moore, E., Krüger, A., Hauben, L., Seal, S., De Baere, R., De Wachter, K., et al. (1997). 16S rRNA gene sequence analyses and inter- and intrageneric relationship of Xanthomonas species and Stenotrophomonas maltophilia. FEMS Microbiol. Lett. 151, 145–153. doi: 10.1111/j.1574-6968.1997.tb12563.x
Mukherjee, S. P., and Choudhuri, M. A. (1983). Implications of water stress-induced changes in the levels of endogenous ascorbic acid and hydrogen peroxide in Vigna seedlings. Physiol. Plant. 58, 166–170. doi: 10.1111/j.1399-3054.1983.tb04162.x
Nadeem, S. M., Zahir, Z. A., Naveed, M., and Arshad, M. (2007). Preliminary investigations on inducing salt tolerance in maize through inoculation with rhizobacteria containing ACC deaminase activity. Can. J. Microbiol. 53, 1141–1149. doi: 10.1139/W07-081
Noori, F., Etesami, H., Zarini, H. N., Khoshkholgh-Sima, N. A., Salekdeh, G. H., and Alishahi, F. (2018). Mining alfalfa (Medicago sativa L.) nodules for salinity tolerant non-rhizobial bacteria to improve growth of alfalfa under salinity stress. Ecotoxicol. Environ. Saf. 162, 129–138. doi: 10.1016/j.ecoenv.2018.06.092
Orhan, F. (2016). Alleviation of salt stress by halotolerant and halophilic plant growth-promoting bacteria in wheat (Triticum aestivum). Braz. J. Microbiol. 47, 621–627. doi: 10.1016/j.bjm.2016.04.001
Patel, D., Jha, C. K., Tank, N., and Saraf, M. (2012). Growth enhancement of chickpea in saline soils using plant growth-promoting rhizobacteria. J. Plant Growth Regul. 31, 53–62. doi: 10.1007/s00344-011-9219-7
Patel, M. K., Mishra, A., and Jha, B. (2016). Non-targeted metabolite profiling and scavenging activity unveil the nutraceutical potential of psyllium (Plantago ovata Forsk). Front. Plant Sci. 7:431. doi: 10.3389/fpls.2016.00431
Peng, Y. L., Gao, Z. W., Gao, Y., Liu, G. F., Sheng, L. X., and Wang, D. L. (2008). Eco-physiological characteristics of Alfalfa seedlings in response to various mixed salt-alkaline stresses. J. Integr. Plant Biol. 50, 29–39. doi: 10.1111/j.1744-7909.2007.00607.x
Ramana, G. V., Padhy, S. P., and Chaitanya, K. V. (2012). Differential responses of four soybean (Glycinemax. L) cultivars to salinity stress. Legume Res. Int. J. 35, 185–193.
Rojas-Tapias, D., Moreno-Galván, A., Pardo-Díaz, S., Obando, M., Rivera, D., and Bonilla, R. (2012). Effect of inoculation with plant growth-promoting bacteria (PGPB) on amelioration of saline stress in maize (Zea mays). Appl. Soil Ecol. 61, 264–272. doi: 10.1016/j.apsoil.2012.01.006
Roy, S. J., Negrão, S., and Tester, M. (2014). Salt resistant crop plants. Curr. Opin. Biotechnol. 26, 115–124. doi: 10.1016/j.copbio.2013.12.004
Ryan, R. P., Monchy, S., Cardinale, M., Taghavi, S., Crossman, L., Avison, M. B., et al. (2009). Versatility and adaptation of bacteria from the genus Stenotrophomonas. Nat. Rev. Microbiol. 7, 514–525. doi: 10.1038/nrmicro2163
Sarkar, A., Ghosh, P. K., Pramanik, K., Mitra, S., Soren, T., Pandey, S., et al. (2018). A halotolerant Enterobacter sp. displaying ACC deaminase activity promotes rice seedling growth under salt stress. Res. Microbiol. 169, 20–32. doi: 10.1016/j.resmic.2017.08.005
Shannon, M. C., and Grieve, C. M. (1998). Tolerance of vegetable crops to salinity. Sci. Hortic. 78, 5–38. doi: 10.1016/S0304-4238(98)00189-7
Singh, R. P., and Jha, P. N. (2016). A halotolerant bacterium Bacillus licheniformis HSW-16 augments induced systemic tolerance to salt stress in wheat plant (Triticum aestivum). Front. Plant Sci. 7:1890. doi: 10.3389/fpls.2016.01890
Singh, R. P., and Jha, P. N. (2017). The PGPR Stenotrophomonas maltophilia SBP-9 augments resistance against biotic and abiotic stress in wheat plants. Front. Microbiol. 8:1945. doi: 10.3389/fmicb.2017.01945
Singh, V. K., Kavita, K., Prabhakaran, R., and Jha, B. (2013). Cis-9-octadecenoic acid from the rhizospheric bacterium Stenotrophomonas maltophilia BJ01 shows quorum quenching and anti-biofilm activities. Biofouling 29, 855–867. doi: 10.1080/08927014.2013.807914
Singh, V. K., Mishra, A., Haque, I., and Jha, B. (2016). A novel transcription factor-like gene SbSDR1 acts as a molecular switch and confers salt and osmotic endurance to transgenic tobacco. Sci. Rep. 6:31686. doi: 10.1038/srep31686
Srivastava, P., Wu, Q. S., and Giri, B. (2019). “Salinity: an overview,” in Microorganisms in Saline Environments: Strategies and Functions, eds B. Giri and A. Varma (Cham: Springer), 3–18.
Tiwari, S., Singh, P., Tiwari, R., Meena, K. K., Yandigeri, M., Singh, D. P., et al. (2011). Salt-tolerant rhizobacteria-mediated induced tolerance in wheat (Triticum aestivum) and chemical diversity in rhizosphere enhance plant growth. Biol. Fertil. Soils 47:907. doi: 10.1007/s00374-011-0598-5
Upadhyay, S. K., Singh, J. S., Saxena, A. K., and Singh, D. P. (2012). Impact of PGPR inoculation on growth and antioxidant status of wheat under saline conditions. Plant Biol. 14, 605–611. doi: 10.1111/j.1438-8677.2011.00533.x
Wang, J., Zhang, Y., Li, Y., Wang, X., Liu, Z., Nan, W., et al. (2016). Involvement of polar auxin transport in the inhibition of Arabidopsis seedling growth induced by Stenotrophomonas maltophilia. Biol. Plant. 60, 299–310. doi: 10.1007/s10535-016-0585-7
Yang, A., Akhtar, S. S., Iqbal, S., Amjad, M., Naveed, M., Zahir, Z. A., et al. (2016). Enhancing salt tolerance in quinoa by halotolerant bacterial inoculation. Funct. Plant Biol. 43, 632–642. doi: 10.1071/FP15265
Yang, J., Kloepper, J. W., and Ryu, C. M. (2009). Rhizosphere bacteria help plants tolerate abiotic stress. Trends Plant Sci. 14, 1–4. doi: 10.1016/j.tplants.2008.10.004
Yoolong, S., Kruasuwan, W., Pham, H. T. T., Jaemsaeng, R., Jantasuriyarat, C., and Thamchaipenet, A. (2019). Modulation of salt tolerance in Thai jasmine rice (Oryza sativa L. cv. KDML105) by Streptomyces venezuelae ATCC 10712 expressing ACC deaminase. Sci. Rep. 9, 1–10. doi: 10.1038/s41598-018-37987-5
Zhu, Z., Zhang, H., Leng, J., Niu, H., Chen, X., Liu, D., et al. (2020). Isolation and characterization of plant growth-promoting rhizobacteria and their effects on the growth of Medicago sativa L. under salinity conditions. Antonie van Leeuwenhoek 113, 1263–1278. doi: 10.1007/s10482-020-01434-1
Keywords: peanut, saline agriculture, halotolerant bacteria, salt stress, Stenotrophomonas, PGPR - plant growth-promoting rhizobacteria, Arachis hypogaea, plant microbe interaction
Citation: Alexander A, Singh VK and Mishra A (2020) Halotolerant PGPR Stenotrophomonas maltophilia BJ01 Induces Salt Tolerance by Modulating Physiology and Biochemical Activities of Arachis hypogaea. Front. Microbiol. 11:568289. doi: 10.3389/fmicb.2020.568289
Received: 09 June 2020; Accepted: 22 September 2020;
Published: 14 October 2020.
Edited by:
Dilfuza Egamberdieva, Leibniz Centre for Agricultural Landscape Research (ZALF), GermanyReviewed by:
Anukool Vaishnav, GLA University, IndiaSuriyan Cha-um, National Science and Technology Development Agency (NSTDA), Thailand
Copyright © 2020 Alexander, Singh and Mishra. This is an open-access article distributed under the terms of the Creative Commons Attribution License (CC BY). The use, distribution or reproduction in other forums is permitted, provided the original author(s) and the copyright owner(s) are credited and that the original publication in this journal is cited, in accordance with accepted academic practice. No use, distribution or reproduction is permitted which does not comply with these terms.
*Correspondence: Avinash Mishra, YXZpbmFzaEBjc21jcmkucmVzLmlu; YXZpbmFzaG1pc2hyYTExQHJlZGlmZm1haWwuY29t
†Present address: Vijay K. Singh, Department of Microbiology and Immunobiology, Harvard Medical School, Boston, MA, United States; Department of Surgery, Massachusetts General Hospital, Boston, MA, United States