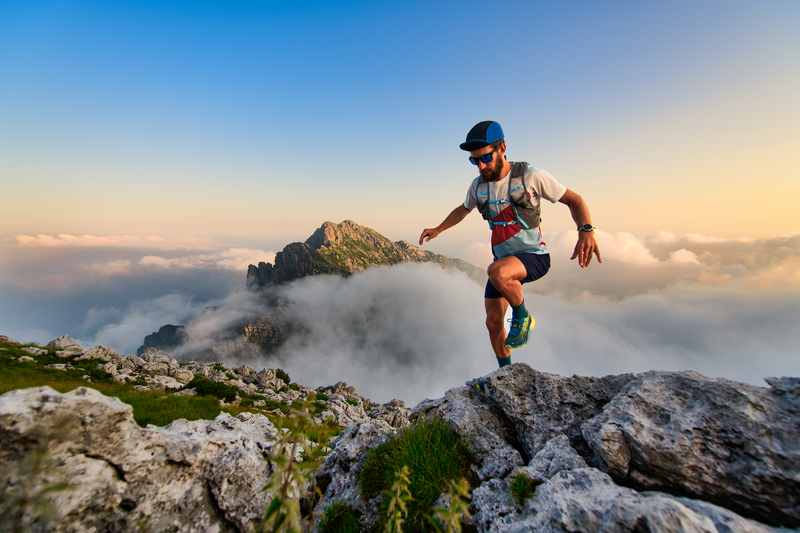
94% of researchers rate our articles as excellent or good
Learn more about the work of our research integrity team to safeguard the quality of each article we publish.
Find out more
ORIGINAL RESEARCH article
Front. Microbiol. , 30 September 2020
Sec. Terrestrial Microbiology
Volume 11 - 2020 | https://doi.org/10.3389/fmicb.2020.560861
We investigated the effects of trace metal additions on microbial nitrogen (N) and carbon (C) cycling using freshwater wetland sediment microcosms amended with micromolar concentrations of copper (Cu), molybdenum (Mo), iron (Fe), and all combinations thereof. In addition to monitoring inorganic N transformations (NO3–, NO2–, N2O, NH4+) and carbon mineralization (CO2, CH4), we tracked changes in functional gene abundance associated with denitrification (nirS, nirK, nosZ), dissimilatory nitrate reduction to ammonium (DNRA; nrfA), and methanogenesis (mcrA). With regards to N cycling, greater availability of Cu led to more complete denitrification (i.e., less N2O accumulation) and a higher abundance of the nirK and nosZ genes, which encode for Cu-dependent reductases. In contrast, we found sparse biochemical evidence of DNRA activity and no consistent effect of the trace metal additions on nrfA gene abundance. With regards to C mineralization, CO2 production was unaffected, but the amendments stimulated net CH4 production and Mo additions led to increased mcrA gene abundance. These findings demonstrate that trace metal effects on sediment microbial physiology can impact community-level function. We observed direct and indirect effects on both N and C biogeochemistry that resulted in increased production of greenhouse gasses, which may have been mediated through the documented changes in microbial community composition and shifts in functional group abundance. Overall, this work supports a more nuanced consideration of metal effects on environmental microbial communities that recognizes the key role that metal limitation plays in microbial physiology.
Wetland microbes are important for removing anthropogenic pollutants from surface waters, effectively preventing the contaminants from entering downstream coastal and marine ecosystems. Human-derived industrial nitrogen (N) input to the environment has been estimated to 150 Tg N y–1 (Schlesinger, 2009), and is of particular concern because it is linked to water quality degradation, eutrophication, and increased emissions of nitrous oxide (N2O), which is a strong greenhouse gas (GHG) that also contributes to ozone depletion (Ravishankara et al., 2009; IPCC, 2013). Biological production of N 2O is mainly through denitrification, nitrification, and nitrifier-dentrification; in addition, some denitrifying microbes can remove N2O by reducing it to inert dinitrogen (N2). Annually, ∼10% of the human-derived N input is returned to the atmosphere as N2 via denitrification from wetlands and terrestrial ecosystems globally (Schlesinger, 2009). Wetlands are equally important for their role in carbon (C) cycling. Despite their relatively small coverage (∼10% of global land area), these ecosystems store large amounts of organic C and emit considerable amounts of methane (CH4) at an estimated rate of 144 Tg CH4 y–1 (IPCC, 2014). These net CH4 emissions represent a balance between the microbial processes of methanogenesis and methanotrophy.
Microbial production and consumption of N2O and CH4 is catalyzed by oxidoreductases that utilize metal co-factors such as molybdenum (Mo), copper (Cu), and iron (Fe) (Glass and Orphan, 2012). For denitrification, the pathway includes the reduction of nitrate (NO3–) to nitrite (NO2–), catalyzed by a periplasmic or membrane bound Mo nitrate reductase (NAR) (Schwarz et al., 2009), and the further reduction of NO2– to nitric oxide (NO) by either a heme-Fe cytochrome cd1 or a Cu co-factor nitrite reductase (NIR), expressed by the genes nirS and nirK, respectively (Adman et al., 1995; Einsle et al., 1999). NO is further reduced to N2O by nitric oxide reductase (NOR), which contains a heme-Fe co-factor (Shiro et al., 2012). Finally, N2O is reduced by a Cu-containing nitrous oxide reductase (NOS) (Rosenzweig, 2000). Similar metal complexes are key in methane cycling. For example, Fe-, Ni-, and Zn-dependent ferredoxins and dehydrogenases catalyze the early steps of methanogenesis, and all methanogens utilize a common Ni methyl-coenzyme M reductase (MCR; mcrA) to catalyze the rate-limiting and final step of CH4 production (Glass and Orphan, 2012; Wongnate and Ragsdale, 2015). Cu also has an integral role in CH4 oxidation. For example, aerobic methanotrophs scavenge Cu with methanobactin, a high-affinity chaperone (Chang et al., 2018), for use in Cu-dependent CH4 monoxygenases. Some anaerobic methanotrophs, such as members of the NC10 phylum (e.g., Methylomirabilis oxyfera), utilize a Cu-dependent particulate methane monoxygenase (pMMO) and an Fe-rich cd1 nitrite reductase to couple CH4 oxidation to denitrification via nitrite-dependent methane oxidation (N-DAMO) (Deutzmann et al., 2014; Cheng et al., 2019). Because of these sorts of interconnected pathways and processes, the abundance and bioavailability of trace metals in the environment can impact N and C biogeochemistry and exert a control on associated GHG emissions.
Although extensive research has considered the relative contribution of key environmental factors (e.g., pH, NO3–, NO2–, O2, and C/N ratio) in regulating the microbial processes associated with N and C biogeochemistry, our understanding of the impact of metal availability is more limited. Metals in the environment have traditionally been seen as unwanted pollutants, and trace metal accumulation has been linked to toxicity and inhibition of ecosystem processes (Samanidou and Papadoyannis, 1992). For example, concentrations exceeding the mg L–1 range for Cu, Mo, Fe, Zn, and Pb severely inhibit denitrification in soils, sediments, surface water bodies, and waste waters (Labbé et al., 2003; Magalhães et al., 2007; Liu et al., 2016). However, given the dependence of many microbial enzymes on metal co-factors, it also is possible for the opposite effect to occur – wherein enzyme function is limited due to an inadequate supply of trace metals. This scenario has received limited attention in environmental studies, but is well documented in case studies with model organisms. In fact, in vitro studies have shown that lack of Cu, Mo, or Fe severely inhibits denitrification or methane cycling due to the formation of non-functional enzymes typically lacking the respective metal co-factor. For example, in the soil bacteria Paracoccus denitrificans and Pseudomonas stutzeri, Cu is required to express a functional NOS dimer and reduce N2O to N2 (Granger and Ward, 2003; Felgate et al., 2012; Black et al., 2016). In methanotrophs oxidizing CH4 to methanol (CH3OH), the switch between a Cu-dependent pMMO or Fe-dependent soluble MMO (sMMO) is regulated by the availability of each metal (Murrell et al., 2000; Bollinger, 2010).
Despite great progress in understanding metal ecotoxicity and metalloenzyme biochemistry, our knowledge of how trace metal availability affects microbial activity in the environment is generally limited to selected processes such as ammonium (NH4+), NO3–, and CO2 assimilation in aquatic ecosystems (Twining et al., 2007; Glass et al., 2012; Moore et al., 2013; Romero et al., 2013; Schoffman et al., 2016). A broader understanding of how metal availability regulates microbial processes, especially in soils and sediments, is necessary if we are to fully comprehend environmental controls on ecosystem N and C cycling. In this study, we investigated the effects of trace metal additions on the microbial biogeochemistry of freshwater wetland sediments focusing primarily on NO3–/NO2– reduction and GHG kinetics, and used quantitative polymerase chain reaction (qPCR) to assess changes in the abundance of key microbial functional groups associated with these processes. We hypothesized that greater bioavailability of Mo, Fe, and Cu in the sediments would increase denitrification and that greater Cu availability would reduce N2O emissions (i.e., by stimulating NOS functioning). We also predicted that CO2 emissions would increase, driven by higher denitrification rates, and CH4 production would decrease because stimulated denitrifiers would outcompete methanogens for C substrates under anoxic conditions.
Wetland soil was collected from a tidal-fluvial bar deposit in Pamunkey River (Virginia, United States; N 37.557451, W −76.972521) in October 2016. Five samples from the 5–15 cm depth interval (∼350 g each) were collected ∼2 m apart using a PVC core (diameter, 10 cm). Porewater was collected from a small pit, which was emptied and allowed to refill naturally with porewater prior to collection. Samples were kept on ice in a cooler during transport to the lab and stored overnight (4°C) until experimental setup and characterization using standard methods (SSSA, 1996). Total metal content was analyzed according to United States Environmental Protection Agency (EPA) standard methodology at the Soil Testing, Insect ID and Plant Diagnostic Lab, Cooperative Extension of the University of the New Hampshire (Durham, NH, United States), and porewater Fe(II) concentrations were measured spectrophotometrically following Viollier et al. (2000). Sediment and porewater properties are summarized in Table 1. Previous studies at this site had identified these sediments to be conducive of denitrification, dissimilatory nitrate reduction to ammonium (DNRA), and methanogenesis (Berrier, 2019; Dang et al., 2019).
After removal of visible root and stone fragments, the five soil samples were combined in equal parts to form a composite sample for subsequent experiments. The soil (1 kg) was amended with 1.5 L of filtered porewater (Cellulose, 10-μm pore-size, Millipore, Burlington, MA, United States) and homogenized using a commercial blender (30 s at max speed) to a final soil-to-liquid ratio of 1:3.5 (dry w/v). Soil slurry aliquots (50 mL) were then transferred to sterile 125-mL glass bottles (Wheaton, Millville, NJ, United States). The bottles were crimped with rubber septa (#224100-180, Weaton, Millville, NJ, United States) and incubated for 3 days in the dark (25°C) to allow residual O2 to be consumed. Each bottle then received 250 μL of 2 M KNO3, yielding a final nominal NO3– concentration of 10 mM, ensuring non-limiting N conditions corresponding to typical NO3– concentrations used in DEA protocols (SSSA, 1994; Murray and Knowles, 1999). Next, metal additions were accomplished by dispensing small aliquots (<1 ml total) from aqueous stock solutions of ammonium heptamolybdate [(NH4)6Mo7O24], iron sulfate (FeSO4), and copper sulfate (CuSO4) to generate final slurry concentrations of 28 μM Mo, 74 μM Fe, and 26 μM Cu. Metal additions were based on a preliminary assessment of N2O emissions derived by a model denitrifying bacterium, P. denitrificans, incubated with different Mo, Fe, and Cu levels (Supplementary Table S1). Seven experimental treatments were established to test the effect of each metal individually (Mo, Fe, or Cu) and in all possible combinations (Mo + Fe, Mo + Cu, Fe + Cu, and Mo + Fe + Cu). In addition, control microcosms were prepared, to which no metals were added. Four replicate microcosms were prepared for each control and treatment. Each bottle was then vortexed briefly (30 s, 6000 rpm), flushed with N2 (60 min), and incubated without shaking in the dark (25°C). Gas (5 mL) and slurry supernatant (1.5 mL) samples were collected using a sterile syringe after 0, 6, 12, 24, 48, 72, and 96 h. Withdrawn gas volumes were replaced with equal N2 gas volumes. Following the 96-h sampling, bottles were opened in an O2-free chamber, and 0.3 g dry weight of soil was removed and immediately frozen (−20°C) for molecular analyses.
Slurry samples were centrifuged (10,000 g, 5 min) and the resulting supernatant was filtered (0.22-μm pore size), and stored frozen (−20°C) until the concentrations of NO3–, NO2–, sulfate (SO42–), and NH4+ was determined by ion-chromatography (ICS – 5000+, Dionex, Sunnydale, CA, United States) utilizing Eluent Generator Cartridges; 23–45 mM KOH and 20 mM methanesulfonic acid, for anion and cation analysis respectively. Ions were separated through Dionex IonPac AG18/AS18 (2 × 250 mm) and IonPac CG12/CS12 (A – 5 μm, 3 × 150 mm) columns with suppression, AERS 500 and CERS 500 for anion and cation analysis, respectively, and detected by a Dionex CD conductivity sensor.
Gas samples were stored in 3 mL Exetainer vials (Labco, United Kingdom) that previously were flushed with N2 and vacuumed with a gas-tight syringe by removing four volumes, i.e., resulting in a vial pressure of ca. 6 kPa. Concentrations of N2O, CO2, and CH4 were determined via gas chromatography (Shimadzu GC-14A using Porapak-N and HayeSep-D and Molecular Sieve MS13 columns and equipped with ECD (N2O), TCD (CO2), and FID (CH4) detectors (Shimadzu, Columbia, MD, United States) as described in Morrissey and Franklin (2015). For each gas, total gas production was determined as the sum of the gas accumulated in the headspace and the gas dissolved in the liquid slurry (e.g., Robertson et al., 1999) using the Ideal gas law, Henry’s gas solubility law, and Bunsen coefficients (at 25°C) of 0.545, 0.032, and 0.772 for N2O, CH4, and CO2, respectively (Felgate et al., 2012; Sander, 2015). To compare overall GHG emissions across treatments, we estimated the combined global warming potential (GWP) of CO2, N2O, and CH4 in terms of CO2-equivalents (g CO2-eq) using the 100 years GWP factors of 298 for N2O and 28 for CH4 as calculated on a mass to mass basis (IPCC, 2013).
DNA was extracted from frozen soil samples (∼0.3 g dry weight) using the DNEasy Kit from Qiagen (Germantown, MD, United States) following the manufacturer’s instructions The retrieved DNA was visualized on a 1.2% agarose gel and quantified using a Nanodrop spectrometer (Thermo Fisher Scientific, Wilmington, DE, United States). Quantitative PCR (qPCR) was used to determine the abundance of microbial groups typically found in wetland sediments: total bacteria (eub), nitrite reducers (denitrification: nirK and nirS; DNRA: nrfA), nitrous oxide reducers (nosZ-clade I and II), and methanogens (mcrA). SensiFASTTM SYBR® No-ROX Kit Polymerase 2× mix (Bioline, United Kingdom) was used for all reactions except nosZ-clade II, which used SYBR green Go-Taq® qPCR Master Mix (Promega, Madison, WI, United States). Primers were purchased from IDT (Integrative DNA Technologies, Skokie, IL, United States) and the DNA for the standard curves was extracted from isolates obtained from ATCC (American Type Culture Collection, Manassas, VA, United States). Data were collected using a Bio-Rad CFX-384 Real Time System (Bio-Rad, Hercules, CA, United States) and analyzed using CFX Manager software (Ver. 3.1), except nosZ-clade II quantification was conducted using QuantStudio 6 Flex (Thermo Fisher Scientific, Wilmington, DE, United States). Primers, qPCR reaction conditions, and efficiencies are summarized in Table 2.
Data did not comply with the normality and variance homogeneity assumptions for ANOVA [RStudio (base); Shapiro’s test, Bartlett’s test]; therefore, non-parametric Kruskal-Wallis tests with Bonferroni correction were applied to assess differences in the mean of ranks among the treatments [RStudio (agricolae)], without any data transformation. For all statistical tests, p ≤ 0.05 was considered significant. Summary statistics were calculated with RStudio (dyplr) (Boston, MA, United States) and plotted with SigmaPlot 14 (Systat Software Inc., San Jose, CA, United States). Central tendency and measures of dispersion are shown as mean ± standard error (SE) with n = 4, unless otherwise specified.
Kinetics of NO3– and NO2– transformations in microcosm pore-water were similar across all treatments. Nitrate reduction commenced at the same time for all the treatments (12–24 h, Figure 1), and the NO3– pool (10–11 mM) was generally consumed within the first 48 h of the incubation. Nitrite was produced concurrently with NO3– depletion and, on average, 0.25 ± 0.02 mM NO2– remained across all treatments at the end of the experiment (Figure 1). An increasing trend in pore-water NH4+ concentration was observed for all treatments during the incubation, and treatments receiving additional Mo + Fe (p < 0.01) and Mo + Fe + Cu (p < 0.01) had significantly higher NH4+ than the control at the end of the experiment (Figure 1). On average, 2.7 ± 0.1% and 4.6 ± 0.7% of NO3– was recovered as NO2– or NH4+, respectively, at the end of the incubation.
Figure 1. Time course of pore-water concentrations (mM) of nitrate (NO3–; A–D), nitrite (NO2–; E–H), and ammonium (NH4+; I–L) in microcosms amended with molybdate (Mo), iron (Fe), copper (Cu), and combinations thereof. Data are shown as mean ± standard error (n = 4).
Concurrent with NO3– consumption in the slurries, N2O production increased rapidly between 24 and 48 h, especially in treatments without added Cu (Figure 2A). The control and treatments with Mo, Fe, and Mo + Fe accumulated significantly more N2O (approximately 79, 55, 62, and 94% of added NO3–, respectively), than the treatments with Cu, Fe + Cu, Mo + Cu, and Mo + Fe + Cu (approximately 13, 14, 4, and 11% of the added NO3–, respectively; Figure 2D). Total N2O concentrations reached a plateau between 48 and 96 h, most likely due to exhaustion of available C and NO3–. CH4 accumulated quickly in the microcosms (6–12 h) and continued to be produced for the duration of the incubation, reaching a final concentration of ∼0.8 mmoles (Figure 2B). By the end of the incubation, all metal additions caused an increase in CH4 production relative to the control, though the differences were only significant between the control and the Cu, Mo + Cu, and Mo + Fe + Cu treatments (Figure 2E). CO2 production also increased throughout the incubation period, most rapidly between 12 and 24 h, and reached ∼2 mmoles CO2 at the end of the incubation (Figure 2C). As with CH4, the only significant treatment differences for CO2 production were between the control and the Cu, Mo + Cu, Mo + Fe + Cu treatments (Figure 2F).
Figure 2. Time course (A–C) and final cumulative gas concentrations (96 h; D–F) of nitrous oxide (N2O; upper panels), methane (CH4; middle panels), and carbon dioxide (CO2; lower panels) in mmoles per microcosms amended with molybdate (Mo), iron (Fe), copper (Cu), and combinations thereof. Data are shown as mean ± standard error (n = 4). Letters within each bar graph (D–F) indicate significant differences as determined by Kruskal–Wallis and Bonferroni post hoc testing.
Bacterial abundance in the control treatment was 5.4 × 108 (±4.5 × 107) 16S rDNA copies g–1 sediment dry weight, which did not differ significantly from the abundance estimates obtained for the Cu, Mo, Mo + Fe, or Mo + Fe + Cu treatments. Abundance in the three remaining treatments (Fe, Fe + Cu, and Mo + Cu) was slightly higher (1.4-fold) and significantly different from the control (p = 0.005; Supplementary Figure S1). Trace metal addition affected the abundance of nirK (p < 0.001), nirS (p < 0.001), nosZ-I (p = 0.001) and nosZ-II (p = 0.013) denitrifying microbial groups (Figure 3). Reducers of NO2– utilizing Cu-NIR (nirK) were more abundant in all treatments when compared to the control, though the increase due to Fe addition was not significant. Microbial groups having a cytochrome cd1-NIR (nirS) were relatively more abundant in the Mo and Fe + Cu treatments. The nosZ clade I and II microbial groups responsible for the reduction of N2O to N2 were in general more abundant in the treatments that received Cu. We observed no effect of trace metals on DNRA NO2– reducers (nrfA, p = 0.24; Figure 3) but found that methanogens were more abundant in the treatments containing additional Mo (Mo, Mo + Cu, Mo + Fe, and Mo + Fe + Cu; mcrA (p = 0.001); Figure 3) when compared to the control.
Figure 3. Gene copies per g sediment (dry weight) at the end of incubation (96 h) in microcosms amended with molybdate (Mo), iron (Fe), copper (Cu), and combinations thereof. Genes were targeted representing nitrite reducers (nirS, nirK, and nrfA), nitrous oxide reducers (nosZ-I and II), and methanogens (mcrA). Data are shown as mean ± standard error (n = 4). Letters within each bar graph indicate significant differences as determined by Kruskal–Wallis and Bonferroni post hoc testing.
We observed that trace metal (Mo, Fe, Cu, and their combinations) addition to wetland sediments regulated GHG emissions (Figure 2) and altered the abundance of microbial functional groups typically associated with N removal and C cycling (Figure 3). Previous studies have examined the effects of trace metal availability on these processes, often reporting inhibitory effects (Giller et al., 1998; Magalhães et al., 2007, 2011; Deng et al., 2018; Keller and Wade, 2018) however, the range of metal concentrations previously tested far exceeds the levels applied in the current study. The key interpretation of the current experiment is that when available at trace levels, metals, specifically Cu, enhanced the reduction of N2O to N2 and increased CH4 and CO2 emissions from wetland sediments. When N2O, CH4, and CO2 were combined and expressed as g CO2-eq per microcosm, all treatments receiving Cu addition (Cu, Mo + Cu, Fe + Cu, and Mo + Fe + Cu) had significantly lower CO2-eq emissions (p < 0.001; Figure 4), indicating a major role of Cu in anaerobic respiration (denitrification and methanogenesis) as a regulator of nutrient cycling and GHG emissions.
Figure 4. Global warming potential in g of CO2 equivalents (g CO2-eq) of cumulative gas concentrations (96 h) of nitrous oxide, methane, and carbon dioxide in anoxic slurries amended with molybdate (Mo), iron (Fe), copper (Cu), and combinations thereof. Data are shown as mean ± standard error (n = 4). Letters indicate significant differences as determined by Kruskal–Wallis and Bonferroni post hoc testing.
In our experiments, none of the trace metal additions had an effect on NO3– removal (Figure 1), which is somewhat surprising since all bacterial nitrate reductases contain a Mo cofactor at their active sites (Moreno-Vivian et al., 1999). This may indicate that Mo was not limiting to the denitrifiers in this set of soils. Also, we observed a small delay in NO3– reduction (∼24 h) in all treatments that could be attributed to the lag time required to induce the denitrifying pathway within a population less accustomed to substantial concentrations of this alternative electron acceptor (Dodla et al., 2008). Concurrent with the loss of NO3–, we observed minor transient accumulation of NO2–; this pattern has been observed frequently (Cooper and Smith, 1963; Taghizadeh-Toosi et al., 2020; Wang et al., 2020) and is thought to be a result of stochastic transcriptional regulation of the nir operon (Hassan et al., 2016). Following NO2– reduction, NO is produced. Though we did not analyze NO concentrations, it is unlikely that NO accumulated in our microcosms but was instead quickly reduced to N2O. Since NO has potent cytotoxicity at μM levels (Chaudhari et al., 2017; Hartop et al., 2017), any accumulation would have had a negative effect on other aspects of microbial metabolism, particularly CO2 production, which we did not observe.
Regardless of what other metals were included in the microcosms, we observed a dramatic effect of Cu addition, leading to enhanced reduction of N2O to N2 (Figure 2) and an increased abundance of nitrite (nirK) and nitrous oxide (nosZ clade I and II) reductase genes (Figure 3), which both code for Cu-containing enzymes. Overall, gene abundance for nosZ clade II was ∼10-fold greater than for clade I, which is consistent with recent reports that clade II is the dominant form in many soil ecosystems (Jones et al., 2013, 2014; Orellana et al., 2014; Ligi et al., 2015; Graves et al., 2016). Clade II is also affiliated with a broader diversity of organisms than Clade I including several non-denitrifiers (Graf et al., 2014; Hallin et al., 2018), so it is important to recognize that our Clade II estimates may reflect changes in community composition that do not necessarily directly relate to N2O reduction. Subsequent analysis using primers that target subclades within Clade II (sensu Chee-Sanford et al., 2020) or metagenomic sequencing could help resolve this and provide a better understanding of the ecophysiology of various nosZ populations. The overall strong effect of Cu on N2O reduction was prevalent because the last step of denitrification is catalyzed by a Cu-containing reductase and no alternative pathways for N2O transformation have yet been discovered (Thomson et al., 2012). In other laboratory experiments, Cu has similarly been found to be a strong driver of denitrifying metabolism. For example, Cu-deficient cells can have more transcripts of nosZ and Cu-scavenging genes to compensate for the loss of N2O reduction due to limited Cu availability (Felgate et al., 2012). Also, strains deficient in Cu-transporters and chaperones may be unable to reduce N2O to N2, forming a nonfunctional NOR, even though nosZ is expressed (Sullivan et al., 2013). In our microcosms, denitrifying bacteria were able to rapidly take advantage of excess NO3– due to copious bioavailable Cu for synthesis of NOS. Even though concentrations of NO3– and Cu are usually lower in environmental scenarios, similar trade-offs may exist, which could affect ecosystem emissions of N2O and require further investigation.
Over the course of the study, small accumulations of pore-water NH occurred (Figure 1) depending on the type of metal added, and DNRA could be another possible factor affecting the fate of added NO3–. The accumulation of NH4+ was greatest in treatments that contained both Mo and Fe (i.e., Mo + Fe and Mo + Fe + Cu), which may indicate a synergistic response of the enzymes that reduce NO3– (all nitrate reductases are Mo-dependent) and the DNRA-specific nitrite reductase (nrfA), which includes a multi-heme complex. Overall, the accumulated NH4+ (<1 mM) was much lower than the amount of NO3– removed (∼10 mM), and the abundance of the DNRA marker gene nrfA was low (Figure 3), so it is likely that negligible DNRA occurred in our microcosms. Indeed, anoxic organic matter mineralization (as indicated by consistent CO2 production) could contribute to the accumulation of NH4+ and the observed differences due to metal addition could be indirect responses. DNRA and N mineralization commonly co-occur in wetland sediments (White and Reddy, 2009), but DNRA seems to be important mainly in environments with high C availability and limited NO3– availability (Hill, 2019). More conclusive results on DNRA activity in the present wetland setting require additional analyses, e.g., using 15NO3– to trace the production of 15NH4+ from DNRA. Additional analyses using alternate nrfA primers could also be informative. In particular, the qPCR primers we used have poor coverage of NrfA Clade I and thus limited ability to detect members of the family Geobacteraceae, which have recently been found to be potentially significant for DNRA across a range of different soil habitats (Nelson et al., 2016). Given the recognized importance of Geobacteraceae in metal cycling (Röling, 2014), further consideration of this group using novel primers such as those recently developed by Cannon et al. (2019) would better assess the genetic potential for DNRA and effects of trace metal availability.
Wetland soils are large C sinks because anoxic conditions constrain organic matter mineralization and oxidation. In freshwater wetlands, the lack of electron acceptors such as O2, NO3–, and SO42–, may lead to fermentative and reductive conditions where organic C will be reduced to CH4 (Kim et al., 2015; Cheng et al., 2019). In this study, the pattern of CO2 production primarily reflected the magnitude of denitrification, as discussed earlier. We assumed that the fraction of total CO2 as bicarbonate (HCO–) was likely constant, because at the end of the incubation pH increased approximately 1 pH-unit due to denitrification and no substantial differences were found in pH among the treatments (Supplementary Table S2). Equally, we did not quantify and identify forms of dissolved organic carbon as this was out of the scope of this study. We hypothesized that denitrifier activity would outcompete methanogen activity similar to the findings of Klüber and Conrad (1998); rather, CH4 accumulated throughout the incubations (Figure 2B). This result was unexpected because denitrification should suppress methanogenesis as a result of the higher Gibb’s free energy (ΔG0). This set of sediments originates from a freshwater wetland that is known to produce significant amounts of CH4 and host diverse methanogenic communities (Morrissey and Franklin, 2015; Berrier, 2019; Dang et al., 2019). It appears that the inhibitory effect of NO3– addition was transient or partial. It is possible that the rapid NO3– consumption and subsequent removal of denitrification intermediates alleviated inhibitory effects on methanogenesis. Since both processes were occurring, it is evident that denitrifiers and methanogens were competing for available C.
Overall, the higher abundance of methanogens (mcrA) and noticeable accumulation of CH4 in all metal treatments versus the control indicates an important role of trace metal availability in CH4 cycling in our system. As expected, Mo addition enhanced the abundance of mcrA genes (Mo, Mo + Cu, Mo + Fe, and Mo + Fe + Cu treatments; Figure 3). This is because a Mo co-factor is typically required for formylmethanofuran dehydrogenase (fmd) that catalyzes the reduction of CO2 to formyl-methanofuran in the first step of methanogenesis by reduction of CO2 with electrons from H2 (Glass and Orphan, 2012). The subsequent step in methanogenesis is catalyzed by MCR, which is a Ni-containing enzyme. Because we did not add Ni in any of our treatments, we assumed that MCR would not change (or would decrease due to competition with denitrification). Instead, Mo addition and the increasing partial pressure of CO2 in the bottles during the incubation seem to have triggered a shift toward a Mo-based methanogenesis pathway. In general, CH4 production in Pamunkey River sediments have been found to proceed by hydrogenotrophic, acetoclastic, and syntrophic pathways (Berrier, 2019; Dang et al., 2019).
Though no Cu-dependent enzymes have yet been identified in the methanogenesis pathways (Glass and Orphan, 2012), the effect of Cu concentration on CH4 emissions from wetlands is an area of active research. Prior work by Keller and Wade (2018) found a strong suppression of CH4 emissions following Cu amendments to peatland soils, and argued broadly that Cu may inhibit methanogenesis in wetland environments. This contradicts our finding that Cu addition elicited more CH4 than the control (Figure 2), as well as similar results from Thomas and Pearce (2004). Interestingly, the discrepancy across these three studies cannot be explained by simply looking at the concentration of Cu added; our Cu amendments were approximately 10-fold lower than those made by Keller and Wade (2018), whereas the amendments made by Thomas and Pearce (2004) were 10-fold higher. Understanding the effects of Cu on net CH4 fluxes requires further study and should consider potential mitigating factors such as soil texture and cation exchange capacity (Thomas and Pearce, 2004), the impact of Cu amendments on dissolved organic carbon availability (Jiao et al., 2005), and metal effects on methane oxidation (Mohanty et al., 2000).
Previous studies have examined the effects of trace metal abundance (or addition) on N and C cycling in sediments, peat, and agricultural soils. Typically, those studies report an inhibitory effect; however, the ranges of trace metal concentrations in those studies far exceed the levels of the current study. For instance, Keller and Wade (2018) amended peat slurries with ∼200 μM trace element solution and found a significant decrease in CH4 emission, most likely due to Cu-induced toxicity. Similarly, Magalhães et al. (2007) observed 85% inhibition of denitrification accompanied by accumulation of substantial N2O and NO2– at 79 μg Cu g–1 sediment (equivalent to 1200 μM Cu) in estuaries. In a follow-up study, lower denitrification rates due to Cu (∼900 μM) were accompanied by a decline in the abundance and β-diversity associated with the nirK, nirS, and nosZ microbial groups (Magalhães et al., 2011), while keeping in mind that the coverage of those primers has improved greatly since then. Elsewhere, the particularly low concentrations of dissolved Cu, Fe, and Mo (∼5, 1, and 0.2 μg L–1, respectively) and the copious dissolved organic C (∼80 mg L–1) (Raudina et al., 2017) in peatlands triggered substantial emissions of CH4 and N2O (Basiliko and Yavitt, 2001; Basiliko et al., 2013; Voigt et al., 2017). A more recent study that tested the effects of Cu pollution (addition of 100 μg L–1, ∼1.6 μM) on urban freshwater wetland sediments found significantly reduced CH4 emissions, but no change in N2O emissions (Doroski et al., 2019). In the latter case, the lack of a N2O response could be due to low NO3– availability (18 μM) relative to C.
The abundance of bioavailable metals in the environment, typically in their ion forms, is often several orders of magnitude lower than the routinely reported total metal content. Further, the ion form of trace metals could be unavailable to microorganisms due to physicochemical interactions (release and sorption kinetics with soil particles and organic matter) and plant uptake (Giller et al., 1998). At neutral and alkaline pH levels, metals tend to be effectively immobilized as inorganic compounds (metal-oxides, -hydroxides, and -carbonates). Additionally, organic complexes such as humic ligands are known to bind metal cations thus lowering their availability to microbes and plants. Furthermore, hydrogen sulfide (H2S), naturally produced due to dissimilatory SO42– reduction in salt marshes, may limit the availability of trace metals through the production of insoluble metal-sulfide complexes with potential implications for N cycling and N2O emissions (Gauci et al., 2004; Butterbach-Bahl et al., 2013). Sulfate-reducing bacteria may outcompete methanogens and thus suppress CH4 emissions in salt marshes. In the current study, the added SO42– as CuSO4 and FeSO4 could potentially have been reduced to H2S; however, the initial and final porewater SO42– concentrations remained at comparable levels (average change over time across treatments was only 2.8%, Supplementary Table S2) and no sulfide odor was detected when microcosms were opened.
Out of the three metals tested here, Fe2+ addition does not appear to influence N and C cycling in this set of sediments. Fe3+ is an abundant element in our biosphere including wetlands, however not directly biologically available, as it has to be reduced to Fe2+. Fe2+ was added in our microcosms due to its role in the reduction of NO2– to NO with cytochrome cd1 NIR. Apparently, this set of sediments has the capacity to supply enough Fe2+. Another, Fe-dependent N-cycling process besides denitrification and DNRA is anaerobic ammonium oxidation (anammox), which relies heavily on cytochromes and oxyreductases containing Fe (Ferousi et al., 2017), and its relative prevalence could be determined by isotopic assessments (Brunner et al., 2013).
Ex situ experimentation offers key advantages in disentangling factors in a controlled environment. It is acknowledged that longer incubation times could shift the microbial community toward a fitter structure for trace metal or high levels of NO3– utilization. The aim of this study was to investigate the prospective of trace metal additions stimulating mixed-community microbial systems, as seen previously on model organisms. Equally, longer incubations, frequent sampling and non-limiting substrates could help us illustrate the potential of trace metals to enhance microbial functioning and microbial groups. Follow-up studies should consider shorter incubation times and lower substrate concentrations to understand the temporal shift in microbial function and diversity upon trace metal additions.
The present results suggest that trace levels of Cu exert a more important effect on N2 and N2O emissions from the environment than previously thought. These findings are important not only for understanding fundamental controls on N2O production, but also for making predictions about potential future emissions given increasing nutrient and metal loads associated with urban pollution. Likewise, the effect of trace metal availability on denitrification enzymology also is of considerable interest in the context of agriculture and food production, and it has been suggested that metal additions could be a possible strategy to mitigate high soil N2O emissions associated with crop production (Richardson et al., 2009; Shen et al., 2019).
Our results demonstrate that trace metal availability affects microbial processes, resulting in greater GHG emissions and C mineralization. We found that even short-term (96 h) manipulation of trace metal availability led to changes in community structure (functional group abundance) and potential increases in GHG emissions. In particular, our results suggest that trace metal bioavailability may, directly or indirectly, regulate or co-limit denitrification kinetics and favor more active microbial communities that are able to quickly acquire the bioavailable metals and incorporate them in functional oxidoreductases. Disentangling the potential controls of various metals on denitrification and other N-cycling processes such as DNRA and anaerobic ammonium oxidation (anammox) and co-varying effects on C cycling, requires additional experimentation across a range of metal concentrations, substrates [e.g., lower (NO3–)] and biochemical processes in different soil types and ecosystems. Future studies should also investigate metagenomic and metatranscriptomic profiles to understand and evaluate the ecological importance of trace metal limitation. This would allow a more comprehensive examination of potential impacts beyond the small group of functional genes that we considered, and would avoid issues such as primer bias, poor coverage, and inefficient amplification often associated with qPCR of phylogenetically diverse groups. Such studies should be accompanied by high-throughput metabolite or process rate analysis either in the lab or in the field.
All datasets presented in this study are included in the article/Supplementary Material.
GG designed the experiments, performed lab analysis, statistically tested and interpreted the data, and wrote and reviewed the manuscript. KRH performed lab analysis, and wrote and reviewed the manuscript. BLB, BS, and LE performed lab analysis and reviewed the manuscript. RBF performed lab analysis, interpreted data, and wrote and reviewed the manuscript. All authors contributed to the article and approved the submitted version.
This work was supported by a grant from the National Science Foundation (DEB 1355059) and is contribution (#92) from the VCU Rice Rivers Center.
The authors declare that the research was conducted in the absence of any commercial or financial relationships that could be construed as a potential conflict of interest.
We thank Dr. Ioannis Ipsilantis for his comments on an earlier version of this manuscript. We also thank Colin McKenny for assistance in field and lab, Gabriella Balasa and Renia Passie for assistance with qPCR, Olivia De Meo for setting up the GC and HPLC procedures, and Dr. Scott Neubauer for GC and HPLC equipment access. This manuscript has been released as a pre-print at bioRxiv (#515809) (Giannopoulos et al., 2019).
The Supplementary Material for this article can be found online at: https://www.frontiersin.org/articles/10.3389/fmicb.2020.560861/full#supplementary-material
FIGURE S1 | Average eub gene copies g–1 sediment (dry weight) at the end of incubation (96 h) in microcosms amended with molybdate (Mo), iron (Fe), copper (Cu), and combinations thereof. Letters above each bar graph indicate significant differences as determined by Kruskal–Wallis and Bonferroni post hoc testing.
TABLE S1 | Growth (OD600) and headspace N2O (ppm) at 24 h for Paracoccus denitrificans incubated at different metal levels, under denitrifying conditions, in denitrifying mineral medium containing 20 mM NO3–. Cu2+, total Mo and total Fe were determined by ICP-OES at Environmental Sciences Analytical Labs, School of Environmental Sciences, University of East Anglia, United Kingdom (Unpublished data, Ph.D. Thesis, Giannopoulos, G. 2015).
TABLE S2 | Initial (0 h) and final (96 h) SO42– concentrations and pH (96 h) of microcosm pore-water in the various treatments (n = 4, mean ± SE).
Adman, E. T., Godden, J. W., and Turley, S. (1995). The structure of Copper-Nitrite Reductase from Achromobacter cycloclastes at five pH values, with NO bound and with Type II copper depleted. J. Biol. Chem. 270, 27458–27474. doi: 10.1074/jbc.270.46.27458
Basiliko, N., Henry, K., Gupta, V., Moore, T. R., Driscoll, B. T., and Dunfield, P. F. (2013). Controls on bacterial and archaeal community structure and greenhouse gas production in natural, mined, and restored Canadian peatlands. Front. Microbiol. 4:215. doi: 10.3389/fmicb.2013.00215
Basiliko, N., and Yavitt, J. B. (2001). Influence of Ni, Co, Fe, and Na additions on methane production in Sphagnum-dominated Northern American peatlands. Biogeochemistry 52, 133–153. doi: 10.1023/a:1006461803585
Berrier, D. J. (2019). Response and Recovery of Syntrophic and Methanogenic Activity to Saltwater Intrusion in a Tidal Freshwater Marsh Soil. Richmond, VA: Virginia Commonwealth University. M.Sc. Thesis.
Black, A., Hsu, P. C., Hamonts, K. E., Clough, T. J., and Condron, L. M. (2016). Influence of copper on expression of nirS, norB and nosZ and the transcription and activity of NIR, NOR and N2OR in the denitrifying soil bacteria Pseudomonas stutzeri. Microb. Biotechnol. 9, 381–388. doi: 10.1111/1751-7915.12352
Brunner, B., Contreras, S., Lehmann, M. F., Matantseva, O., Rollog, M., Kalvelage, T., et al. (2013). Nitrogen isotope effects induced by anammox bacteria. Proc. Natl. Acad. Sci. U.S.A. 110, 18994–18999. doi: 10.1073/pnas.1310488110
Butterbach-Bahl, K., Baggs, E. M., Dannenmann, M., Kiese, R., and Zechmeister-Boltenstern, S. (2013). Nitrous oxide emissions from soils: how well do we understand the processes and their controls? Philos. Trans. R. Soc. Lond. B Biol. Sci. 368:20130122. doi: 10.1098/rstb.2013.0122
Cannon, J., Sanford, R. A., Connor, L., Yang, W. H., and Chee-Sanford, J. (2019). Optimization of PCR primers to detect phylogenetically diverse nrfA genes associated with nitrite ammonification. J. Microbiol. Methods 160, 49–59. doi: 10.1016/j.mimet.2019.03.020
Chang, J., Gu, W., Park, D., Semrau, J. D., DiSpirito, A. A., and Yoon, S. (2018). Methanobactin from Methylosinus trichosporium OB3b inhibits N2O reduction in denitrifiers. ISME J. 12, 2086–2089. doi: 10.1038/s41396-017-0022-8
Chaudhari, S. S., Kim, M., Lei, S., Razvi, F., Alqarzaee, A. A., Hutfless, E. H., et al. (2017). Nitrite derived from endogenous bacterial Nitric Oxide Synthase activity promotes aerobic respiration. mBio 8, e00887–17.
Chee-Sanford, J. C., Connor, L., Krichels, A., Yang, W. H., and Sanford, R. A. (2020). Hierarchical detection of diverse Clade II (atypical) nosZ genes using new primer sets for classical- and multiplex PCR array applications. J. Microbiol. Methods 172:105908. doi: 10.1016/j.mimet.2020.105908
Cheng, C., Shen, X., Xie, H., Hu, Z., Pavlostathis, S. G., and Zhang, J. (2019). Coupled methane and nitrous oxide biotransformation in freshwater wetland sediment microcosms. Sci. Total Environ. 648, 916–922. doi: 10.1016/j.scitotenv.2018.08.185
Cooper, G. S., and Smith, R. L. (1963). Sequence of products formed during denitrification in some diverse Western soils. Soil Sci. Soc. Am. J. 27, 659–662. doi: 10.2136/sssaj1963.03615995002700060027x
Dang, C., Morrissey, E. M., Neubauer, S. C., and Franklin, R. B. (2019). Novel microbial community composition and carbon biogeochemistry emerge over time following saltwater intrusion in wetlands. Glob. Change Biol. 25, 549–561. doi: 10.1111/gcb.14486
Deng, D., Hu, M., Li, L., and Huang, Y. (2018). Denitrifying microbial communities in heavy metal contaminated paddy soils near electronic-waste processing centers. Water Air Soil Pollut. 229:318. doi: 10.1007/s11270-018-3974-z
Deutzmann, J. S., Stief, P., Brandes, J., and Schink, B. (2014). Anaerobic methane oxidation coupled to denitrification is the dominant methane sink in a deep lake. Proc. Natl. Acad. Sci. U.S.A. 111, 18273–18278. doi: 10.1073/pnas.1411617111
Dodla, S. K., Wang, J. J., DeLaune, R. D., and Cook, R. L. (2008). Denitrification potential and its relation to organic carbon quality in three coastal wetland soils. Sci. Total Environ. 407, 471–480. doi: 10.1016/j.scitotenv.2008.08.022
Doroski, A. A., Helton, A. M., and Vadas, T. M. (2019). Greenhouse gas fluxes from coastal wetlands at the intersection of urban pollution and saltwater intrusion: a soil core experiment. Soil Biol. Biochem. 131, 44–53. doi: 10.1016/j.soilbio.2018.12.023
Einsle, O., Messerschmidt, A., Stach, P., Bourenkov, G. P., Bartunik, H. D., Huber, R., et al. (1999). Structure of cytochrome c nitrite reductase. Nature 400:476. doi: 10.1038/22802
Felgate, H., Giannopoulos, G., Sullivan, M. J., Gates, A. J., Clarke, T. A., Baggs, E., et al. (2012). The impact of copper, nitrate and carbon status on the emission of nitrous oxide by two species of bacteria with biochemically distinct denitrification pathways. Environ. Microbiol. 14, 1788–1800. doi: 10.1111/j.1462-2920.2012.02789.x
Ferousi, C., Lindhoud, S., Baymann, F., Kartal, B., Jetten, M. S. M., and Reimann, J. (2017). Iron assimilation and utilization in anaerobic ammonium oxidizing bacteria. Curr. Opin. Chem. Biol. 37, 129–136. doi: 10.1016/j.cbpa.2017.03.009
Fierer, N., Jackson, J. A., Vilgalys, R., and Jackson, R. B. (2005). Assessment of soil microbial community ctructure by use of taxon-specific quantitative PCR assays. Appl. Environ. Microbiol. 71, 4117–4120. doi: 10.1128/aem.71.7.4117-4120.2005
Gauci, V., Matthews, E., Dise, N., Walter, B., Koch, D., Granberg, G., et al. (2004). Sulfur pollution suppression of the wetland methane source in the 20th and 21st centuries. Proc. Natl. Acad. Sci. U.S.A. 101, 12583–12587. doi: 10.1073/pnas.0404412101
Giannopoulos, G., Hartop, K. R., Brown, B. L., and Franklin, R. (2019). Response relationships between CO2, CH4 and N2O emissions and microbial functional groups in wetland sediments after trace metal addition. bioRxiv[Preprint]. doi: 10.1101/515809
Giller, K. E., Witter, E., and McGrath, S. P. (1998). Toxicity of heavy metals to microorganisms and microbial processes in agricultural soils: a review. Soil Biol. Biochem. 30, 1389–1414. doi: 10.1016/s0038-0717(97)00270-8
Glass, J., and Orphan, V. J. (2012). Trace metal requirements for microbial enzymes involved in the production and consumption of methane and nitrous oxide. Front. Microbiol. 3:61. doi: 10.3389/fmicb.2012.00061
Glass, J. B., Axler, R. P., Chandra, S., and Goldman, C. R. (2012). Molybdenum limitation of microbial nitrogen assimilation in aquatic ecosystems and pure cultures. Front. Microbiol. 3:331. doi: 10.3389/fmicb.2012.00331
Graf, D. R. H., Jones, C. M., and Hallin, S. (2014). Intergenomic comparisons highlight modularity of the denitrification pathway and underpin the importance of community structure for N2O emissions. PLoS One 9:e114118. doi: 10.1371/journal.pone.0114118
Granger, J., and Ward, B. B. (2003). Accumulation of nitrogen oxides in copper-limited cultures of denitrifying bacteria. Limnol. Oceanogr. 48, 313–318. doi: 10.4319/lo.2003.48.1.0313
Graves, C. J., Makrides, E. J., Schmidt, V. T., Giblin, A. E., Cardon, Z. G., and Rand, D. M. (2016). Functional responses of salt marsh microbial communities to long-term nutrient enrichment. Appl. Environ. Microbiol. 82, 2862–2871. doi: 10.1128/aem.03990-15
Hallin, S., Philippot, L., Löffler, F. E., Sanford, R. A., and Jones, C. M. (2018). Genomics and ecology of novel N2O-reducing microorganisms. Trends Microbiol. 26, 43–55. doi: 10.1016/j.tim.2017.07.003
Hartop, K. R., Sullivan, M. J., Giannopoulos, G., Gates, A. J., Bond, P. L., Yuan, Z., et al. (2017). The metabolic impact of extracellular nitrite on aerobic metabolism of Paracoccus denitrificans. Water Res. 113, 207–214. doi: 10.1016/j.watres.2017.02.011
Hassan, J., Qu, Z., Bergaust, L. L., and Bakken, L. R. (2016). Transient accumulation of NO and N2O during denitrification explained by assuming cell diversification by stochastic transcription of denitrification genes. PLoS Comput. Biol. 12:e1004621. doi: 10.1371/journal.pcbi.1004621
Henry, S., Bru, D., Stres, B., Hallet, S., and Philippot, L. (2006). Quantitative detection of the nosZ gene, encoding nitrous oxide reductase, and comparison of the abundances of 16S rRNA, narG, nirK, and nosZ genes in soils. Appl. Environ. Microbiol. 72, 5181–5189. doi: 10.1128/aem.00231-06
Hill, A. R. (2019). Groundwater nitrate removal in riparian buffer zones: a review of research progress in the past 20 years. Biogeochemistry 143, 347–369. doi: 10.1007/s10533-019-00566-5
IPCC (2013). “Climate Change 2013,” in The Physical Science Basis. Contribution of Working Group I to the Fifth Assessment, eds T. F. Stocker, D. Qin, G.-K. Plattner, M. Tignor, S. K. Allen, J. Boschung, et al. (New York, NY: Intergovernmental Panel on Climate Change).
IPCC (2014). “Climate Change 2014,” in Synthesis Report. Contribution of Working Groups I, II and III to the Fifth Assessment Report of the Intergovernmental Panel on Climate Change, eds Core Writing Team Pachauri, R. K., and Meyer, L. A. (Geneva: IPPC).
Jiao, Y., Huang, Y., Zong, L., Zheng, X., and Sass, R. L. (2005). Effects of copper concentration on methane emission from rice soils. Chemosphere 58, 185–193. doi: 10.1016/j.chemosphere.2004.03.005
Jones, C. M., Graf, D. R. H., Bru, D., Philippot, L., and Hallin, S. (2013). The unaccounted yet abundant nitrous oxide-reducing microbial community: a potential nitrous oxide sink. ISME J. 7, 417–426. doi: 10.1038/ismej.2012.125
Jones, C. M., Spor, A., Brennan, F. P., Breuil, M.-C., Bru, D., Lemanceau, P., et al. (2014). Recently identified microbial guild mediates soil N2O sink capacity. Nat. Clim. Change 4, 801–805. doi: 10.1038/nclimate2301
Keller, J. K., and Wade, J. (2018). No evidence for trace metal limitation on anaerobic carbon mineralization in three peatland soils. Geoderma 314, 95–101. doi: 10.1016/j.geoderma.2017.11.001
Kim, S. Y., Veraart, A. J., Meima-Franke, M., and Bodelier, P. L. E. (2015). Combined effects of carbon, nitrogen and phosphorus on CH4 production and denitrification in wetland sediments. Geoderma 25, 354–361. doi: 10.1016/j.geoderma.2015.03.015
Klüber, H. D., and Conrad, R. (1998). Effects of nitrate, nitrite, NO and N2O on methanogenesis and other redox processes in anoxic rice field soil. FEMS Microbiol. Ecol. 25, 301–318. doi: 10.1111/j.1574-6941.1998.tb00482.x
Labbé, N., Parent, S., and Villemur, R. (2003). Addition of trace metals increases denitrification rate in closed marine systems. Water Res. 37, 914–920. doi: 10.1016/s0043-1354(02)00383-4
Ligi, T., Truu, M., Oopkaup, K., Nõlvak, H., Mander, Ü, Mitsch, W. J., et al. (2015). The genetic potential of N2 emission via denitrification and ANAMMOX from the soils and sediments of a created riverine treatment wetland complex. Ecol. Eng. 80, 181–190. doi: 10.1016/j.ecoleng.2014.09.072
Liu, Y., Liu, Y., Zhou, H., Li, L., Zheng, J., Zhang, X., et al. (2016). Abundance, composition and activity of denitrifier communities in metal polluted paddy soils. Sci. Rep. 6:19086. doi: 10.1038/srep19086
Magalhães, C., Costa, J., Teixeira, C., and Bordalo, A. A. (2007). Impact of trace metals on denitrification in estuarine sediments of the Douro River estuary. Portugal. Mar. Chem. 107, 332–341. doi: 10.1016/j.marchem.2007.02.005
Magalhães, C. M., Machado, A., Matos, P., and Bordalo, A. A. (2011). Impact of copper on the diversity, abundance and transcription of nitrite and nitrous oxide reductase genes in an urban European estuary. FEMS Microbiol. Ecol. 77, 274–284. doi: 10.1111/j.1574-6941.2011.01107.x
Mohanty, S. R., Bharati, K., Deepa, N., Rao, V. R., and Adhya, T. K. (2000). Influence of heavy metals on methane oxidation in tropical rice soils. Ecotoxicol. Environ. Safety 47, 277–284. doi: 10.1006/eesa.2000.1963
Moore, C. M., Mills, M. M., Arrigo, K. R., Berman-Frank, I., Bopp, L., Boyd, P. W., et al. (2013). Processes and patterns of oceanic nutrient limitation. Nat. Geosci. 6, 701–710. doi: 10.1038/ngeo1765
Moreno-Vivian, C., Cabello, P., Martinez-Luque, M., Blasco, R., and Castillo, F. (1999). Prokaryotic nitrate reduction: molecular properties and functional distinction among bacterial nitrate reductases. J. Bacteriol. 181, 6573–6584. doi: 10.1128/jb.181.21.6573-6584.1999
Morrissey, E. M., and Franklin, R. B. (2015). Evolutionary history influences the salinity preference of bacterial taxa in wetland soils. Front. Microbiol. 6:1013. doi: 10.3389/fmicb.2015.01013
Murray, R. E., and Knowles, R. (1999). Chloramphenicol inhibition of denitrifying enzyme activity in two agricultural soils. Appl. Environ. Microbiol. 65, 3487–3492. doi: 10.1128/aem.65.8.3487-3492.1999
Murrell, J. C., McDonald, I. R., and Gilbert, B. (2000). Regulation of expression of methane monooxygenases by copper ions. Trends Microbiol. 8, 221–225. doi: 10.1016/S0966-842X(00)01739-X
Nelson, M. B., Martiny, A. C., and Martiny, J. B. H. (2016). Global biogeography of microbial nitrogen-cycling traits in soil. Proc. Natl. Acad. Sci. U.S.A. 113, 8033–8040. doi: 10.1073/pnas.1601070113
Orellana, L. H., Rodriguez-R, L. M., Higgins, S., Chee-Sanford, J. C., Sanford, R. A., Ritalahti, K. M., et al. (2014). Detecting nitrous oxide reductase (nosZ) genes in soil metagenomes: method development and implications for the nitrogen cycle. mBio 5:e01193-14.
Raudina, T. V., Loiko, S. V., Lim, A. G., Krickov, I. V., Shirokova, L. S., Istigechev, G. I., et al. (2017). Dissolved organic carbon and major and trace elements in peat porewater of sporadic, discontinuous, and continuous permafrost zones of western Siberia. Biogeosciences 14, 3561–3584. doi: 10.5194/bg-14-3561-2017
Ravishankara, A. R., Daniel, J. S., and Portmann, R. W. (2009). Nitrous Oxide (N2O): the dominant ozone-depleting substance emitted in the 21st Century. Science 326:123. doi: 10.1126/science.1176985
Richardson, D., Felgate, H., Watmough, N., Thomson, A., and Baggs, E. (2009). Mitigating release of the potent greenhouse gas N2O from the nitrogen cycle - could enzymic regulation hold the key? Trends Biotechnol. 27, 388–397. doi: 10.1016/j.tibtech.2009.03.009
Robertson, G. P., Coleman, D. C., Bledsoe, C. S., and Sollins, P. (1999). Standard Soil Methods for Long-Term Ecological Research. New York, NY: Oxford university Press.
Röling, W. F. M. (2014). “The Family Geobacteraceae,” in The Prokaryotes: Deltaproteobacteria and Epsilonproteobacteria, eds E. Rosenberg, E. F. DeLong, S. Lory, E. Stackebrandt, and F. Thompson (Berlin: Springer), 157–172. doi: 10.1007/978-3-642-39044-9_381
Romero, I. C., Klein, N. J., Sañudo-Wilhelmy, S. A., and Capone, D. G. (2013). Potential trace metal co-limitation controls on N2 fixation and NO uptake in lakes with varying trophic status. Front. Microbiol. 4:54. doi: 10.3389/fmicb.2013.00054
Samanidou, V., and Papadoyannis, I. (1992). Study of heavy metal pollution in the waters of Axios and Aliakmon rivers in northen Greece. J. Environ. Sci. Health A 27, 587–601. doi: 10.1080/10934529209375750
Sander, R. (2015). Compilation of Henry’s law constants (version 4.0) for water as solvent. Atmos. Chem. Phys. 15, 4399–4981. doi: 10.5194/acp-15-4399-2015
Schlesinger, W. H. (2009). On the fate of anthropogenic nitrogen. Proc. Natl. Acad. Sci. U.S.A. 106, 203–208. doi: 10.1073/pnas.0810193105
Schoffman, H., Lis, H., Shaked, Y., and Keren, N. (2016). Iron-nutrient interactions within phytoplankton. Front. Plant Sci. 7:1223. doi: 10.3389/fpls.2016.01223
Schwarz, G., Mendel, R. R., and Ribbe, M. W. (2009). Molybdenum cofactors, enzymes and pathways. Nature 460, 839–847. doi: 10.1038/nature08302
Semedo, M., Song, B., Sparrer, T., and Phillips, R. L. (2018). Antibiotic effects on microbial communities responsible for denitrification and N2O production in grassland soils. Front. Microbiol. 9:2121. doi: 10.3389/fmicb.2018.02121
Shen, W., Xue, H., Gao, N., Shiratori, Y., Kamiya, T., Fujiwara, T., et al. (2019). Effects of copper on nitrous oxide (N2O) reduction in denitrifiers and N2O emissions from agricultural soils. Biol. Fert. Soils 56, 39–51. doi: 10.1007/s00374-019-01399-y
Shiro, Y., Sugimoto, H., Tosha, T., Nagano, S., and Hino, T. (2012). Structural basis for nitrous oxide generation by bacterial nitric oxide reductases. Philos. Trans. R. Soc. Lond. B Biol. Sci. 367, 1195–1203. doi: 10.1098/rstb.2011.0310
Smith, C. J., Nedwell, D. B., Dong, L. F., and Osborn, A. M. (2007). Diversity and abundance of nitrate reductase genes (narG and napA), nitrite reductase genes (nirS and nrfA), and their transcripts in estuarine sediments. Appl. Environ. Microbiol. 73, 3612–3622. doi: 10.1128/aem.02894-06
SSSA (1994). Methods of Soil Analysis: Part 2 Microbiological and Biochemical Properties, 5.2. Madison, WI: Soil Science Society of America, Inc.
SSSA (1996). Methods of Soil Analysis: Part 3 Chemical Methods, 5.3. Madison, WI: Soil Science Society of America, Inc.
Steinberg, L. M., and Regan, J. M. (2009). mcrA-targeted real-time quantitative PCR method to examine methanogen communities. Appl. Environ. Microbiol. 75, 4435–4442. doi: 10.1128/aem.02858-08
Sullivan, M. J., Gates, A. J., Appia-Ayme, C., Rowley, G., and Richardson, D. J. (2013). Copper control of bacterial nitrous oxide emission and its impact on vitamin B12-dependent metabolism. Proc. Natl. Acad. Sci. U.S.A. 110, 19926–19931. doi: 10.1073/pnas.1314529110
Taghizadeh-Toosi, A., Clough, T., Petersen, S. O., and Elsgaard, L. (2020). Nitrous oxide dynamics in agricultural peat soil in response to availability of nitrate, nitrite, and iron sulfides. Geomicrobiol. J. 37, 76–85. doi: 10.1080/01490451.2019.1666192
Takeuchi, J. (2006). Habitat segregation of a functional gene encoding nitrate ammonification in estuarine sediments. Geomicrobiol. J. 23, 75–87. doi: 10.1080/01490450500533866
Thomas, P. A., and Pearce, D. M. E. (2004). Role of cation exchange in preventing the decay of anoxic deep bog peat. Soil Biol. Biochem. 36, 23–32. doi: 10.1016/j.soilbio.2003.07.003
Thomson, A. J., Giannopoulos, G., Pretty, J., Baggs, E. M., and Richardson, D. J. (2012). Biological sources and sinks of nitrous oxide and strategies to mitigate emissions. Philos. Trans. R. Soc. B Biol. Sci. 367, 1157–1168. doi: 10.1098/rstb.2011.0415
Throback, I. N., Enwall, K., Jarvis, A., and Hallin, S. (2004). Reassessing PCR primers targeting nirS, nirK and nosZ genes for community surveys of denitrifying bacteria with DGGE. FEMS Microbiol. Ecol. 49, 401–417. doi: 10.1016/j.femsec.2004.04.011
Twining, B. S., Mylon, S. E., and Benoit, G. (2007). Potential role of copper availability in nitrous oxide accumulation in a temperate lake. Limnol. Oceanogr. 52, 1354–1366. doi: 10.4319/lo.2007.52.4.1354
Viollier, E., Inglett, P. W., Hunter, K., Roychoudhury, A. N., and van Cappellen, P. (2000). The ferrozine method revisited: Fe(II)/Fe(III) determination in natural waters. Appl. Geochem. 15, 785–790. doi: 10.1016/s0883-2927(99)00097-9
Voigt, C., Marushchak, M. E., Lamprecht, R. E., Jackowicz-Korczyński, M., Lindgren, A., Mastepanov, M., et al. (2017). Increased nitrous oxide emissions from Arctic peatlands after permafrost thaw. Proc. Natl. Acad. Sci. U.S.A. 114, 6238–6243. doi: 10.1073/pnas.1702902114
Wang, M., Hu, R., Ruser, R., Schmidt, C., and Kappler, A. (2020). Role of chemodenitrification for N2O emissions from nitrate reduction in rice paddy soils. ACS Earth Space Chem. 4, 122–132. doi: 10.1021/acsearthspacechem.9b00296
White, J. R., and Reddy, K. R. (2009). “Biogeochemical Dynamics I: Nitrogen Cycling in Wetlands,” in The Wetlands Handbook, eds E. Maltby and T. Barker (Oxford: Blackwell Publishing Ltd), 800.
Keywords: denitrification, DNRA, carbon mineralization, wetland microbes, trace metals
Citation: Giannopoulos G, Hartop KR, Brown BL, Song B, Elsgaard L and Franklin RB (2020) Trace Metal Availability Affects Greenhouse Gas Emissions and Microbial Functional Group Abundance in Freshwater Wetland Sediments. Front. Microbiol. 11:560861. doi: 10.3389/fmicb.2020.560861
Received: 10 May 2020; Accepted: 24 August 2020;
Published: 30 September 2020.
Edited by:
Jeanette M. Norton, Utah State University, United StatesReviewed by:
Lijun Hou, East China Normal University, ChinaCopyright © 2020 Giannopoulos, Hartop, Brown, Song, Elsgaard and Franklin. This is an open-access article distributed under the terms of the Creative Commons Attribution License (CC BY). The use, distribution or reproduction in other forums is permitted, provided the original author(s) and the copyright owner(s) are credited and that the original publication in this journal is cited, in accordance with accepted academic practice. No use, distribution or reproduction is permitted which does not comply with these terms.
*Correspondence: Georgios Giannopoulos, R2VvcmdlLlouR2lhbm5vcG91bG9zQGdtYWlsLmNvbQ==
†Present address: Georgios Giannopoulos, School of Agriculture, Aristotle University of Thessaloniki, Thessaloniki, Greece; Katherine R. Hartop, Royal Society of Chemistry, Cambridge, United Kingdom
Disclaimer: All claims expressed in this article are solely those of the authors and do not necessarily represent those of their affiliated organizations, or those of the publisher, the editors and the reviewers. Any product that may be evaluated in this article or claim that may be made by its manufacturer is not guaranteed or endorsed by the publisher.
Research integrity at Frontiers
Learn more about the work of our research integrity team to safeguard the quality of each article we publish.