- 1Department for Innovation in Biological, Agrofood and Forest Systems (DIBAF), University of Tuscia, Viterbo, Italy
- 2Department of Agricultural and Forest Sciences (DAFNE), University of Tuscia, Viterbo, Italy
- 3CREA Research Centre for Food and Nutrition, Rome, Italy
Strains belonging to Pantoea agglomerans species are known for their ability to produce metabolites that can act in synergy with auxins to induce the adventitious root (AR) formation. The latter is critically important in the agamic propagation of several woody species, including pear (Pyrus communis L.), playing a considerable role in the commercial nursery farms including those using micropropagation techniques. When grown on a medium amended with tryptophan, the plant-growth-promoting (PGP) strain P. agglomerans C1 produces a cocktail of auxin and auxin-like molecules that can be utilized as biostimulants to improve the rooting of vegetable (Solanum lycopersicum L.) and woody crop species (Prunus rootstock GF/677 and hazelnut). In this study, we evaluated the morphological and molecular responses induced by strain C1 exometabolites in microcuttings of P. communis L. cv Dar Gazi and the potential benefits arising from their application. Results showed that exometabolites by P. agglomerans C1 induced a direct and earlier emergence of roots from stem tissues and determined modifications of root morphological parameters and root architecture compared to plants treated with the synthetic hormone indole-3-butyric acid (IBA). Transcription analysis revealed differences in the temporal expression pattern of ARF17 gene when IBA and C1 exometabolites were used alone, while together they also determined changes in the expression pattern of other key auxin-regulated plant genes. These results suggest that the phenotypic and molecular changes triggered by P. agglomerans C1 are dependent on different stimulatory and inhibitory effects that auxin-like molecules and other metabolites secreted by this strain have on the gene regulatory network of the plant. This evidence supports the hypothesis that the strategies used to harness the metabolic potential of PGP bacteria are key factors in obtaining novel biostimulants for sustainable agriculture. Our results demonstrate that metabolites secreted by strain C1 can be successfully used to increase the efficiency of micropropagation of pear through tissue culture techniques.
Introduction
A growing body of research demonstrated that cells and metabolites from plant-growth-promoting (PGP) bacteria and fungi can be successfully used as biostimulants to promote plant growth, enhancing nutrient uptake and photosynthetic activity, as well as to increase crop quality and plant resistance to both biotic and abiotic stresses (Wu and Zou, 2009; Glick, 2014; Ruzzi and Aroca, 2015; Colla et al., 2017; Rouphael and Colla, 2018; Woo and Pepe, 2018). Due to the increasing demand of high-quality planting materials through agamic propagation and, in particular, tissue culture techniques (Twaij et al., 2020), there is rising interest in the use of microbial and nonmicrobial biostimulants in the improvement of micropropagation methods for industrial, ornamental, and food vegetable and fruit crops (Campobenedetto et al., 2020; Cardarelli et al., 2020; Cirillo et al., 2020; de Azevedo et al., 2020; Dong et al., 2020; Gulzar et al. 2020; Luziatelli et al. 2020b; Umanzor et al., 2020). In this respect, beneficial microorganisms able to synthesize or metabolize plant hormones are of particular importance because they can modulate the balance between plant growth and defense against stresses and pathogens, by changing the concentration of specific phytohormones (Tsukanova et al., 2017; Saia et al., 2019, 2020; Rouphael et al., 2020).
In the context of plant tissue culture, one of the crucial processes in the vegetative propagation of many woody species is the formation of adventitious rooting (AR) from the stem, which involves the changing of the destiny of stem cells near the wound region which leads to the organization of new radical meristem (Garrido et al., 2002). For many woody species, including pear (one of the world’s most important fruit crops; FAOSTAT, 20181), this process is critical even in in vitro culture systems, making difficult the production of many plants on an industrial propagation scale and maintenance of the genetic identity of elite genotypes. Among many endogenous and environmental factors, auxins, such as indole-3-acetic acid (IAA), play a pivotal role for priming, initiation, and establishment of adventitious roots (Wiesman et al., 1988; Li et al., 2009; Pacurar et al., 2014; Druege et al., 2019) and in the regulation of root system architecture in response to internal signals and environmental stimuli (Overvoorde et al., 2010; Semeradova et al., 2020; Waidmann et al., 2020).
Plants synthesize auxins in the shoot apical meristem of young leaves, and then these molecules are transported and concentrated at the base of cutting through basipetal polar transport (Ahkami et al., 2013). Generally, the concentration of endogenous auxins at the base of cutting does not sufficiently induce AR; exogenous auxin is thus added to the culture medium used for in vitro culture systems to reach the optimal gradient. Many synthetic auxins are then used to induce roots on the shoot, and the use of these compounds in commercial plant propagation is commonly implemented (Kurepin et al., 2011; Reed et al., 2012). Each auxin has a different ability to induce AR, which can be attributed to the differences in the auxin receptors involved in the rhizogenic process, as well as to the interaction with other molecules and environmental factors that can interfere with the process (de Klerk et al., 1999; Tereso et al., 2008; Iacona and Muleo, 2010; da Costa et al., 2013; Daud et al., 2013). It is also known that plants exhibit different dose-dependent responses to exogenous auxin gradients: low concentrations of IAA can stimulate primary root elongation, whereas high IAA levels have an opposite effect (Thimann, 1939; Enders and Strader, 2015).
Several bacterial species, including Pantoea agglomerans, can produce IAA and auxin-related compounds that have great physiological relevance in bacteria-plant interactions and determine modifications both in the root system architecture and in the structure of root tissues (Spaepen, 2015). Production of bacterial auxins varies among different strains and is influenced by strain growth conditions, as well as by precursor (tryptophan) availability (Duca et al., 2014). In addition, IAA and IAA-related compounds are frequently produced together with other plant growth regulators that can enhance the growth stimulatory effect of microbial phytohormones (Stringlis et al., 2018).
P. agglomerans is a rod-shaped, non-spore-forming, Gram-negative bacterium that is found in soil and is adapted to live in association with plants (Walterson and Stavrinides, 2015; Zhang and Qiu, 2015). Certain strains belonging to this species are agronomically relevant for their PGP traits and biocontrol activity (Dutkiewicz et al., 2016) and for their ability to produce IAA through a highly conserved indole-3-pyruvic acid (IPyA) pathway (Luziatelli et al., 2020b). P. agglomerans strain C1 was previously isolated from the phyllosphere of lettuce plants (Lactuca sativa L.) treated with plant-derived protein hydrolysates (Luziatelli et al., 2016). The strain was characterized for its ability to solubilize phosphate, produce IAA, and produce siderophores for inhibiting plant pathogens (Luziatelli et al., 2019a, 2020a). Recently, the whole genome of P. agglomerans C1 was sequenced, providing insights into heavy metal resistance and metabolic capacities of this strain (Luziatelli et al., 2019b, 2020a). In addition, it was demonstrated that inoculation with C1 cells improved the growth in pots of tomato (Solanum lycopersicum L.) and corn (Zea mays L.) fertilized with rock phosphates (Saia et al., 2020) and, also, that metabolites secreted by this strain can be utilized as biostimulants to improve the root surface area in cuttings of tomato (Luziatelli et al., 2020a), Prunus rootstocks GF/677, and Corylus avellana L. (Luziatelli et al., 2020b).
Considering the importance of AR induction in the agamic propagation and micropropagation of cultures for research and industrial nursery farming applications, further evaluation of the plant response to metabolites secreted by selected PGP rhizobacteria (PGPR) can provide useful insights into the rational development of new biostimulants that can contribute to achieving agriculture-related sustainable development goals. In this work, we compared the effect of indole-3-butyric acid (IBA; the most widely used synthetic auxin for cutting propagation), metabolites secreted by P. agglomerans C1, and a combination of both on root architectural traits and gene expression in cuttings of Pyrus communis L. cv Dar Gazi. In addition, we evaluated the possibility to develop an improved rooting procedure based on the use of P. agglomerans metabolites.
Materials and Methods
Bacterial Strain and Culture Conditions
P. agglomerans C1 was routinely cultured in Lennox LB broth (Acumedia, Baltimore, MD, USA; Miller, 1972). Shake cultures were performed in Erlenmeyer flasks at 30°C, in agitation (180 rpm). Production of IAA occurred only when tryptophan was added to the medium. Stock cultures were maintained at −80°C in LB medium amended with glycerol 20% (v/v). Bacterial metabolites used for in vitro rooting experiments were prepared according to a two-step culture procedure (Luziatelli et al., 2019b). Overnight pre-seed cultures were prepared by inoculating 50 ml of fresh LB medium with 0.5 ml of a glycerol stock and grown at 30°C with shaking (180 rpm). The culture was used to inoculate a 250 ml shake-flask containing 25 ml of LB supplemented with tryptophan (4 mM), with an initial optical density (OD600) of 0.1. Cells were grown under suboptimal temperature-agitation speed conditions (28°C and 150 rpm) to maintain auxin concentration between 200 and 350 μM IAA equivalent (IAAequ). After 24 h of growth, cells were separated from the exhausted medium by centrifugation (10 min at 10,000 rpm) and discharged. The supernatant was filter-sterilized (0.22 μm), the IAA concentration was determined as described below, and samples were stored at −20°C until use.
Determination of Indole Auxins in Culture Filtrate
Auxin production was measured using Salkowski’s reagent, as described previously by Patten and Glick (2002). In brief, 1 ml of filter-sterilized (0.22 μm) supernatant was added to 2 ml of Salkowski reagent (0.5 M FeCl3, 35% v/v HClO4). The mixture was incubated for 15 min in the dark at room temperature; the development of a pink color, which was determined spectrophotometrically at 535 nm, indicated the production of indole auxins. A series of IAA standard solutions of known concentrations were prepared to set up the calibration curve.
Plant Material
Shoot cultures of pear cultivar Dar Gazi were initiated with 5–10-mm-long shoot tips, excised from an already-established in vitro culture, and maintained in the proliferation medium. Proliferation medium, hormonal composition, and growth chamber conditions were reported in Abdollahi et al. (2004). Rooting experiments were run using microcuttings, about 2 cm long, cut from shoot cluster, and transferred onto rooting medium. The latter was composed of half concentrations of MS salts (Murashige and Skoog, 1962), supplemented with 20 g L−1 sucrose and with an appropriate amount of IBA (1.0 mM) and/or auxin-like phytohormones from P. agglomerans C1.
The medium was sterilized at 121°C for 20 min after the addition of 6 g L−1 plant agar (Duchefa Biochemie BV, The Netherlands) and pH titration to 5.7 with NaOH. To test the AR induction potential of C1 strain’s auxin-like metabolites, the medium was supplemented with the amount of exometabolites necessary to obtain an IAAequ concentration of 1.0 (C1-sm) and 2.0 mM (2× C1-sm). In standard experiments, an IAAequ concentration of 1.0 mM was obtained by a 250-fold dilution of the filter-sterilized supernatant of a C1 culture grown at 28°C and an agitation speed of 150 rpm. Rooting tests were carried out by using the bacterial metabolites alone or in combination with IBA 1.0 mM. Control treatments in which the medium was amended with a 250-fold dilution of LB amended with tryptophan (4 mM), with tryptophan at a final concentration of 16 mM, or with no hormonal addition were also used. All the supplements were added to the medium after sterilization immediately before pouring the plates. As culture vessels, 250 ml glass jars were used, each containing 30 ml of rooting medium.
Experimental Design and Parameters
Experiments were performed in the growth chamber maintained at a temperature of 24 ± 1°C, by placing vessels under the darkness for a period of 4 days, after the beginning of experiments, and then moving them under the light condition of 40 μmol m−2 s−1. Five jars, each containing six explants, were used in each hormonal treatment. Measurements included the number of rooted explants, the total number of roots per shoot, and the dimension of ARs for each hormonal treatment and were detected for a 40-day period, from the beginning of experimentation. All experiments were repeated twice, and the results were the average values of both experiments.
Ex Vitro Acclimatization
For in vivo acclimatization, rooted plantlets were transferred into plug trays (2 × 2 × 3.5 cm), containing sterile BRIL typical TYP 3 (Germany) peat substrate, and placed in a climate-controlled growth chamber, at a temperature of 25 ± 4°C, at constant photoperiod at a light intensity of 40 μmol m−2 s−1, and at a virtually constant RH (80%). Plant survival was determined after 2 months.
RNA Extraction and Gene Expression Analyses
Total RNA was extracted starting from 450 mg of tissues collected: time point T0, before the treatment; time point T1, at the end of the dark treatment (4 days after the treatment) or, for C1-sm + IBA treatment, at the beginning of callus formation (6 days after the treatment); time point T2, after the development of the primordial roots. The apex was removed, and the tissue collected at the base of the microcutting was powdered under liquid nitrogen according to Pistelli et al. (2012). Samples were purified using a Plant RNA Purification Kit (Norgen Biotek, Thorold, ON, Canada) combined with an on-column DNase digestion with the RNase-Free DNase set (Qiagen, Hilden, Germany) to remove genomic DNA contamination. All kits were used following the manufacturer’s instructions. RNA purity was evaluated by agarose gel electrophoresis and quantified by using a QUBIT® 2.0 fluorometer (Thermo Fisher Scientific, Monza, Italy). First-strand cDNA was synthesized using the kit Tetro Reverse Transcriptase (BioLine, London, UK), following the manufacturer’s guidelines.
Real-time PCR analysis of genes was conducted using the thermal cycler LC480II® (Roche, Monza, Italy). Each reaction (10 μl) contained 6 μl of LightCycler 480 SYBR Green I Master (Roche, Monza Italy), 0.5 μM of each primer, 0.5 μl of cDNA, and 2.3 μl of PCR-grade water. The PCR was conducted using the following conditions: 95°C for 10 min and 45 cycles at 94°C for 20 s, 59°C for 30 s, and 72°C for 30 s, followed by a melting cycle from 65 to 95°C. Real-time quantitative PCR was performed using three biological replicates, with three technical replicates for each sample. A minus reverse transcriptase control was used to exclude genomic DNA contamination. Data were expressed with the 2ΔCp method (Kubista et al., 2006) using the geometric means of the actin gene as endogenous reference genes for the normalization of transcript abundance. After PCR amplification, melting analysis and gel electrophoresis visualization were run to confirm their identity and the absence of false-positive products.
Statistical Analysis
The significance of the effects of P. agglomerans C1 metabolites on the AR induction and root development characters was tested by analysis of variance (ANOVA), performed by the SigmaStat 3.1 package (Systat Software Inc., San Jose, CA, USA). Means were separated by the LSD test. Data percentages were transformed to arcsine degree values before the ANOVA, when necessary.
Results
Auxin Production
To evaluate synergistic effects between auxins and other metabolites secreted by P. agglomerans C1, cells were grown in medium amended with tryptophan at suboptimal temperature (28°C) and agitation speed (150 rpm). Under these conditions, culture filtrate of strain C1 contained 286 ± 57 μmol of IAAequ per liter and was used for in vitro experiments without extensive dilution. Under conditions of optimal temperature (30°C) and agitation speed (180 rpm), strain C1 produced on the same culture medium about 900 ± 50 μmol of IAAequ per liter.
In Vitro Rooting
Table 1 shows the effects of hormonal composition and concentration of compound applied into the rooting medium on stimulation of rooting from shoots of pear cv Dar Gazi. AR formation was strongly enhanced by the addition of metabolites secreted by P. agglomerans C1 (C1-sm) and to a lesser extent by IBA (Table 1). In the first experiment, two IAAequ concentrations of secreted metabolites were tested: 1 (C1-sm) and 2 μM (2× C1-sm). Exometabolites from strain C1 were tested alone or in combination with IBA (1 μM), and experiments were repeated twice. Subsequently, with the aim of confirming the results, other trials were run and only C1-sm was used, alone or in combination with IBA. In all experiments, the positive role in the induction of AR clearly resulted when compared with IBA, confirming the observations made in the first two trials.
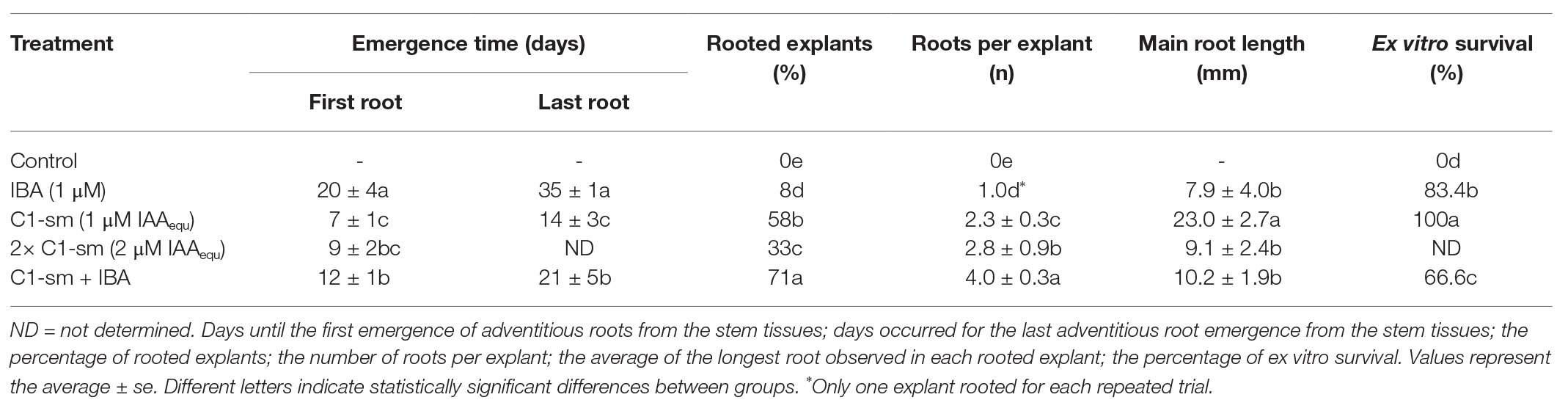
Table 1. Effect of IBA, C1-sm, and the combination of both on pear root production and ex vitro survival.
AR required a lot of time to emerge from the stem of microcuttings; a strong difference was in fact observed when the IBA and microbial IAA were added into the rooting medium both alone and in combination (Table 1). The formation of roots occurred in the microcuttings treated with C1-sm in a shorter time (7 ± 1 days after the treatment) than in microcuttings treated with C1-sm + IBA (12 ± 1 days after the treatment) and IBA alone (20 ± 4 days after the treatment; Table 1). Root emergence was not a synchronous event and occurred in a time span between 14 ± 3 and 35 ± 1 days (Table 1). Microcuttings treated with metabolites secreted by P. agglomerans C1 rooted earlier and for a shorter period than those treated with IBA alone (Table 1).
The number of ARs per explant induced by the combination of C1-sm and IBA (4.0 ± 0.3) was, respectively, 1.7‐ and 4-fold higher than that obtained using C1-sm and IBA alone (Table 1). Root formation was not observed in control experiments with plant growth medium: (1) without growth regulators; (2) with 16 mM tryptophan; or (3) with 250-fold diluted LB medium +4 mM tryptophan (Table 1).
As reported in Table 1, the use of C1-sm, alone or in combination with IBA, also stimulated root elongation. In particular, the length of the main root was 2.9-fold higher when the medium was supplemented with C1 exometabolites in place of IBA (Table 1).
Interestingly, in the microcuttings where the ARs were induced by IBA or a combination of IBA and C1-sm, roots emerged from the undifferentiated callus tissue; on the contrary, in microcuttings treated with C1-sm, no callus formation was observed at the base of the shoot explants, without any apparent formation of callus tissues (Figure 1).
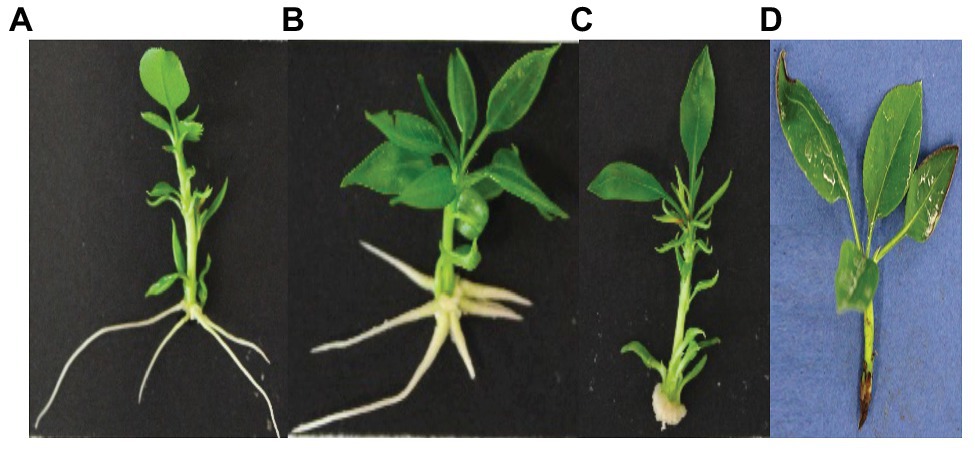
Figure 1. Adventitious root formation in microcuttings of Pyrus communis cv Dar Gazi cultured on MS medium added with C1-sm (1 μM IAAequ) (A), a combination of C1-sm (1 μM IAAequ) and IBA (1 μM) (B), and IBA (1 μM) (C) after 13 days of in vitro culture. Callus development was observed only when IBA was used alone or in combination with C1-sm (B,C). No root formation was observed in control treatments with microcuttings cultured on MS medium without auxins (D).
Expression Analysis of Selected Auxin Signaling-Responsive Genes
Auxin Response Factor PcARF6, PcARF8, and PcARF17 Genes
Differential expressions of PcARF6, PcARF8, and PcARF17 genes in pear microcuttings treated with IBA and C1-sm, alone or in combination with IBA, were investigated using real-time RT-PCR, and PcActin was used as a reference gene for normalization of target gene expression. The transcription analysis revealed that all these genes were downregulated by IBA (Figure 2). A similar effect was observed for PcARF6 and PcARF8 on microcuttings treated with C1-sm (Figure 2). In contrast, in cuttings treated with C1 exometabolites, the mRNA level of the PcARF17 gene decreased during the dark period (T1) and increased at the initiation of root primordia (time point T2; Figure 2). Interestingly, the expression profiles of the three genes were quite different when microcuttings were treated with a combination of C1-sm and IBA, with PcARF6 and PcARF17 expressions being upregulated at the initiation of root primordia (T2) and PcARF8 expression remaining constant across all time points (Figure 2).
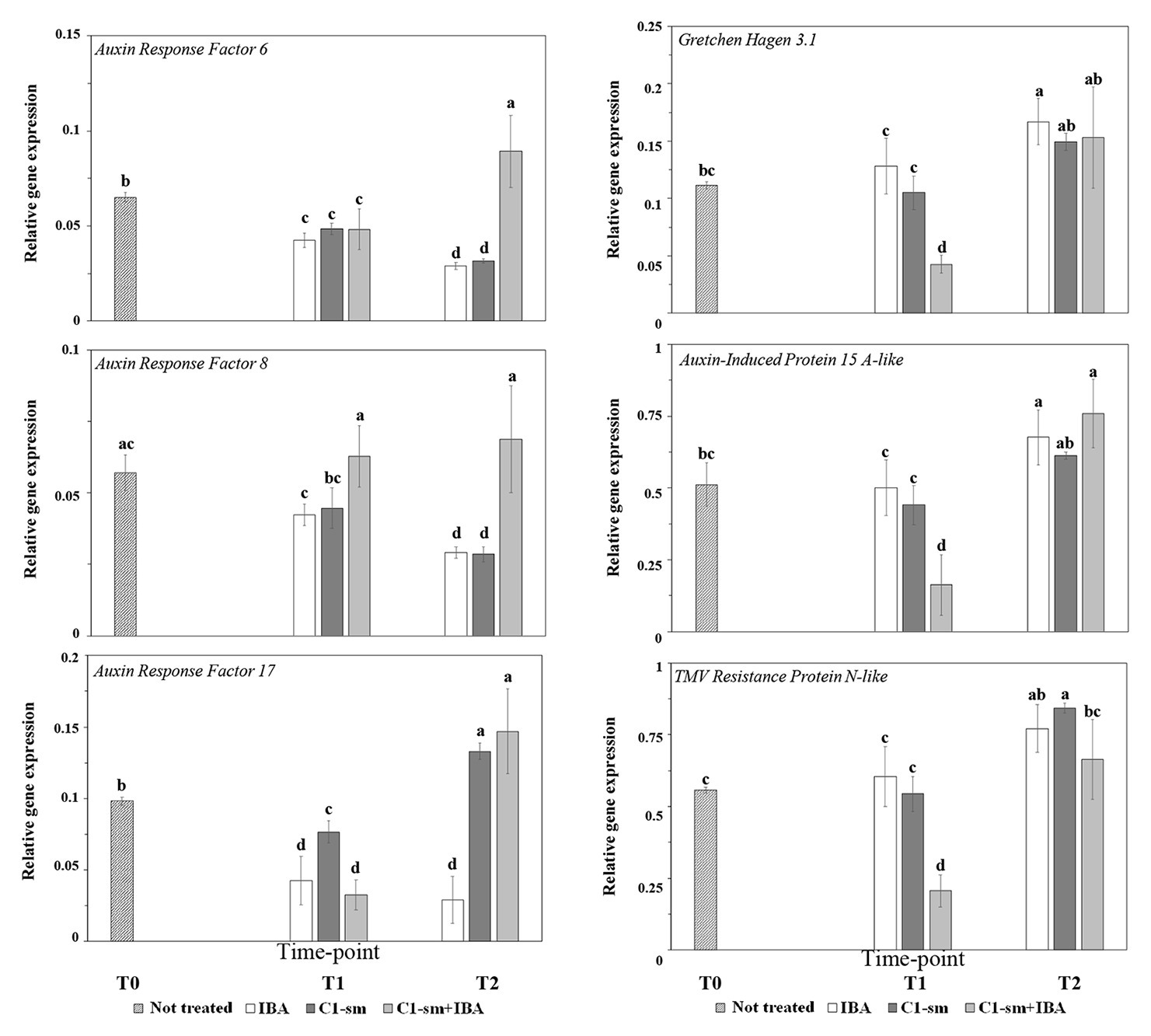
Figure 2. The expression pattern of selected Pyrus communis (Pc) genes responding to auxin signaling in microcuttings treated with IBA (1 μM), C1-sm (1 μM of IAAequ), and a combination of both, C1-sm (1 μM of IAAequ) + IBA (1 μM). The bar at time point T0 indicates expression in untreated control microcuttings, the bars at time point T1 indicate expression in microcuttings 4 days (IBA and C1-sm) or 6 days (C1-sm + IBA) after the treatment, the bars at time point T2 indicate expression at the initiation of root primordia. Relative expression of the target gene was normalized to the reference gene PcActin, as an internal control. Means are from three independent repeats; error bars show standard deviations. Different letters indicate statistically significant differences compared to control (T0) and between groups (p < 0.05).
Gretchen Haven 3.1 (PcGH3.1) Gene
PcGH3.1 gene was upregulated at the initiation of root primordia (time point T2) in tissues treated with IBA (Figure 2). The expression profile of this gene was significantly different when tissues were treated with a combination of C1-sm and IBA, where a significant reduction of PcGH3.1 mRNA level was observed 6 days after the treatment (T1; Figure 2). In contrast, no significant difference was observed comparing the relative expression of this gene in microcuttings treated with IBA or C1-sm alone (Figure 2).
Auxin-Induced Protein 15A-Like (PcSAUR7) Gene
The expression profile of PcSAUR7 was similar to that observed for PcGH3.1, with a significant reduction of mRNA level at time point T1 on microcuttings treated with a combination of C1-sm and IBA and an increase in the mRNA level of the three genes between time points T1 and T2 (Figure 2).
TMV Resistance Protein N-Like (PcTMV) Gene
The expression profile of PcTMV on microcuttings treated with IBA and C1-sm alone and in combination followed the same expression trend observed for PcGH3.1 and PcSAUR7 under the same conditions (Figure 2).
Discussion
In previous work, we demonstrated that the production of IAA and IAA-related compounds by P. agglomerans strain C1 is tryptophan dependent and is affected by several parameters, including the medium composition, the carbon source, the physiological state of the cells, and the induction timing (Luziatelli et al., 2020b). Usually, standard experiments with PGP bacteria are designed to obtain the maximum auxin production, and to avoid inhibitory effects on the plant growth, culture filtrates containing a higher IAAequ titer are extensively diluted before use as plant biostimulants. Although, in general, bacterial strains exhibiting higher volumetric productivity of a specific metabolite are preferred for large-scale production, in this context, extensive dilution of the culture filtrate can be detrimental to evaluating the synergistic effects that metabolites secreted by IAA/auxin-producing bacteria can have on transport and turnover of exogenous and endogenous auxin in plants (Luziatelli et al., 2020b). In this study, we demonstrated that the auxin production by P. agglomerans strain C1 can be modulated (until one-third of the maximum) by lowering the growth temperature and the aeration of the liquid culture. The cultivation under suboptimal conditions alters the balance between auxin and other metabolites constitutively produced by strain C1 and allows the use of its spent growth medium at a higher strength.
In microcuttings of P. communis L. cv Dar Gazi cultured into rooting medium enriched with exometabolites from auxin-producing cultures of P. agglomerans C1, AR emergence occurred earlier than in cuttings that cultured into IBA-enriched rooting medium. In addition, in (C1-sm)-treated shoots (Figure 1), all roots were located at the side of the stem, indicating that the primordia of AR directly develop from the cells neighboring vascular tissues and the subsequent elongated root directly emerged from the epidermal tissue of side of the stem, just above the basal pruned zone (de Klerk et al., 1999; Lakehal and Bellini, 2019). The direct rooting formation resulted independently from the concentration of C1-sm used, and when the medium was enriched with IBA, either alone or in combination with C1-sm, another contrasting morphological scenario was evident. As a first step, a cell division forming callus tissue was observed, and then AR was observed, probably, after the reprogramming of the AR founder cell (Bustillo-Avendaño et al., 2018). Our data are also in agreement with the observations demonstrating that the auxin transcriptional networks involved in both direct and indirect de novo root regeneration (DNDR) and callus formation in the model plant Arabidopsis partially share the same genetic pathway (reviewed in Jing et al., 2020).
It is already known that in cuttings of woody species, wounding can stimulate callus formation and AR primordia can emerge from the cells of this undifferentiated tissue (Li et al., 2009; Xu, 2018). In microcuttings of the apple tree, the AR initiation occurred from cells of the cambial zone located between the two vascular tissues of the stem, without the formation of callus, and the root emerged outside the stem 15 days after the treatment with IBA (Naija et al., 2008). Furthermore, the time of root emergence outside the stem and the diverse root morphology in microcuttings treated with C1-sm and IBA reinforced the hypothesis that the AR phenotype is regulated differently by the two treatments.
To evaluate the synergistic effect of auxin and other metabolites produced by strain C1, experiments were carried out by using two different amounts of growth medium, and interestingly, better results in terms of percentage of rooted explants and the average length of the main root were obtained with C1-sm at the lower concentration (1 μM IAAequ; Table 1). It is noteworthy that, upon a combination of C1-sm and IBA, we observed a 40% reduction in the timing of AR emergence compared to IBA, as well as a ninefold increase in the percentage of rooted explants and a fourfold increase in the number of roots per explant, while the length of the main root increased compared to IBA, but not to the same extent when using C1-sm alone (Table 1). These results confirm our hypothesis that the balance between auxin and other biostimulant molecules produced by strain C1, as well as the appropriate gradient generated by the amount of exogenous auxin that is used, plays a critical role in determining the performance of the biostimulant during the rooting stage of micropropagation, improving the efficiency of the nursery industry. In this study, IBA was applied at a concentration (1 μM) less than fivefold lower than the one which is usually used in the pear rooting medium (Reed et al., 2012), so it is not surprising that the addition of auxins present in C1-sm has a positive effect on AR formation. At the same time, the reduction in the percentage of rooted explants and in the length of the main root, which was observed when IBA was added to C1-sm, indicates that, when strain C1 metabolites are present at a certain concentration, an excess of auxins can inhibit the formation and the elongation of the adventitious roots (Table 1). The differences in the root architecture that are shown in Figure 1 clearly indicate that the metabolites produced by P. agglomerans C1 not only accelerate the AR formation when auxins are present but also increase the number and length of AR primordia that develop from stems, modifying the response of the pear plant to exogenous auxins.
In recent work, Lotfi et al. (2019) demonstrated that trans-cinnamic acid, a phenylpropenoic compound produced by the microbial deamination of phenylalanine (Hazir et al., 2017), efficiently induces AR formation in shoots of pear cv Arbi. It is noteworthy that P. agglomerans C1 has genes encoding enzymes (e.g., a CAT-catalase, ec 1.11.1.6) that are involved in the biosynthesis of cinnamic-related compounds from intermediates of the tryptophan pathway, and we have evidence that strain C1 produces cinnamates as well as peptides and cyclopeptides that can crosstalk with auxin (Luziatelli et al., 2020b). These compounds may likely play a possible regulative role in plant gene expression through epigenetic mechanisms, such as DNA methylation, histone modification (acetylation, methylation, and phosphorylation), and miRNA activation, as it already emerged in humans (Zhu et al., 2016; Frolinger et al. 2018; Arora et al., 2019; Carlos-Reyes et al., 2019; Patnaik and Anupriya, 2019). These mechanisms likely activate many regulatory pathways generating a response, in parallel to the pathway activated by auxin compounds, and thus establish a synergistic action. It is known, in fact, that polyphenols, such as phloroglucinol, caffeic acid, chlorogenic acid, and ferulic acid, have a synergistic action, together with auxins, as mediators of root formation from cuttings (James and Thurbon, 1981; Zimmerman and Broome, 1981; Wilson and Van Staden 1990; Wang, 1991; Nandi et al., 1996; De Klerk et al., 2011).
To gain insights into the molecular mechanisms elicited by strain C1 exometabolites, we analyzed the expression profile of three auxin response factor (ARF) genes, which play a key role in the AR initiation process and in auxin homeostasis. Results reported in Figure 2 indicated that these genes were differentially modulated in microcutting tissues treated with C1-sm or IBA. The temporal expression patterns of PcARF6 and PcARF8 were similar, while significant differences were observed in the temporal transcription pattern and in the steady-state transcript level of PcARF17 (Figure 2). The latter gene was overexpressed in microcuttings treated with C1-sm during the rooting phases examined in this work (Figure 2). Auxin homeostasis plays a key role in the AR formation: the level of free auxins, in the model plant Arabidopsis thaliana, controls the expression of the ARFs, AtARF6, AtARF8, and AtARF17, that activate a signaling cascade to lead to the AR initiation in the target cells until the emergence of root primordium (Gutierrez et al., 2012; Guan et al., 2015). These genes are also under the control of light and, when cuttings of A. thaliana are transferred from darkness to light, ARF6 and ARF8 are upregulated, while ARF17 is downregulated (Fattorini et al., 2018). In our experimental conditions, in microcuttings treated with IBA or C1-sm, the orthologous PcARF6 and PcARF8 genes were downregulated, and at each time point, no significant difference in transcript levels of these genes was observed (Figure 2). In contrast, when IBA was used in combination with C1-sm, PcARF6 was upregulated at sampling point T2, while the mRNA level of PcARF8 was stable across the three time points (Figure 2). Taken together, these results indicate that the addition of C1-sm to IBA determines a differential transcriptional or posttranscriptional regulation of PcARF6 and PcARF8 genes that is probably related to the different morphological and temporal responses elicited by IBA and C1-sm + IBA (Table 1; Figure 1). The temporal expression profiles of these ARF genes in cv Dar Gazi differ from those reported in the literature, but they appear to be consistent with data obtained in vitro with apple microcuttings, in which expressions of ARF6 and ARF8 were not observed during AR formation (Li et al., 2016). The same authors also demonstrated that, in apple microcuttings, a treatment with IBA determined an upregulation of ARF9 gene and a downregulation of ARF1 and ARF11. It is worth mentioning that, although no significant difference was found in the temporal expression patterns of PcARF6 and PcARF8 in microcuttings treated with IBA (1 μM) or C1-sm (1 μM IAAequ), the different timing of root primordium initiation at equal auxin molarity (7 and 20 days, respectively; Table 1) suggests that the auxin signal transduction is more effective and faster when target cells were treated with strain C1 exometabolites. This hypothesis is supported by the observation that, regardless of the speed of the process, at the AR emergence (time point T2), a significant reduction in the steady-state transcript levels of PcARF6 and PcARF8 was observed in both IBA‐ and C1-sm-treated microcuttings. It is reasonable to assume that the downregulation of PcARF6 and PcARF8 observed at time point T2 is related to the low concentration of auxin (1 μM) that was used in this study. According to the auxin signaling model proposed by Guilfoyle and Hagen (2007), the expression of these genes should be downregulated when auxin concentration is below a threshold level because ARF6 and ARF8 proteins act as transcriptional activators of GH3 genes, which encode auxin adenylating enzymes. The same model can also explain results obtained analyzing the expression of the PcARF17 gene at time point T1, which indicated downregulation of this gene independently of treatment (Figure 2). In A. thaliana, it has been demonstrated that ARF17 is a negative regulator of AR formation and is an antagonist of AtARF6 and AtARF8 genes (Gutierrez et al., 2009; Villacorta-Martín et al., 2015). So it can be suggested that by changing the concentration of ARF17 protein and modifying the balance among ARF transcriptional activators and repressors, the AR formation promoted by ARF6 and ARF8 can be promoted. Interestingly, at time point T1, the steady-state transcript level of PcARF17 significantly increased when replacing IBA with C1-sm, and independently from IBA, this gene was upregulated in the presence of strain C1 exometabolites (Figure 2). In accordance with the observations reported in the literature (Gutierrez et al., 2012; Gleeson et al., 2014; Ruedell et al., 2015), it can be postulated that the upregulation of PcAFR17 can explain, at least in part, the altered adventitious root phenotype of C1-sm-treated microcuttings.
C1-sm may contain metabolites that likely behave as co-inducers and/or inhibitors of AR formation. The higher expressions at time point T2 of PcAFR17 and all PcARF genes when C1-sm was used alone or in combination with IBA, respectively (Figure 2), may imply that C1-sm contains molecules which interfere with auxin homeostasis and, in synergy with auxin, accelerate the AR formation process. In this context, the regulatory effect that the metabolites secreted by P. agglomerans C1 have on the transcriptional and posttranscriptional control of the PcAFR17 gene plays a central role in the modification of the root traits and temporal patterns that occur in Dar Gazi.
Interestingly, independent of treatment, the temporal expression patterns of PcGH3.1, PcSAUR7, and PcTMV were similar (Figure 2). All three genes were upregulated at time point T2 when IBA or C1-sm was used alone and were downregulated at time point T1 when IBA was used in combination with C1-sm (Figure 2). These results are in accordance with data obtained on apple microcuttings, which indicate that the expressions of these genes are stimulated by a reduction in the concentration of free auxin (Li et al., 2019). At the same time, the downregulation of the three genes observed at time point T1 after treatment with C1-sm + IBA can be due to the auxin initial concentration that is doubled compared to the conditions in which IBA and C1-sm are used alone.
Genes Gretchen Hagen 3 (GH3) is a family of genes involved in processes of the conjugation of auxins and regulates free auxin content (Ludwig-Müller, 2011; Westfall et al., 2016). The GH3.1 gene encodes an auxin-conjugating enzyme involved in modulating the level of free auxin (Druege et al., 2016).
The trend of PcGH3.1 gene expression that was observed in the different treatments at time point T2 (Figure 2) confirms the hypothesis by Druege et al. (2016). According to them, as soon as the rooting induction is completed, the level of free auxin must decrease in order to avoid an uncontrolled induction of root primordia and inhibit the development of AR previously developed. The minor expression of PcGH3.1 that is observed at time point T1 in tissues treated with C1-sm + IBA may imply that auxin remains in a free form for a longer time when exometabolites from strain C1 are present and thus induces more efficiently the AR formation.
The stability of the auxin signal depends on the type of auxin used in the culture medium. It is known, in fact, that IAA degrades very quickly when it undergoes high temperatures, air, and light (Yamakawa et al., 1979), while IBA, which is a synthetic hormone, is more stable (Robbins et al., 1988). It may thus be hypothesized that auxins present in C1-sm may degrade some days after treatment and, therefore, do not generate unbalances in plant hormone homeostasis when C1-sm and IBA are added together. However, it should be emphasized that in this study, we reduced the hormone concentration, which is usually 5 μM (Jan et al., 2015), up to 1 μM, in order to reduce the risk of uncontrolled accumulation of auxin.
The auxin-induced protein 15A-like encoding gene from P. communis is homologous to the “small auxin upregulated RNA” SAUR7 gene from A. thaliana. SAUR7 encodes a protein involved in an early response of the auxin stimulus and can be used as a marker gene to evaluate the efficiency of auxin treatment (Goda et al., 2004). Instead, the pear gene PcTMV, orthologous to the AtTMV gene, encodes a resistance protein, TIR-NBS-LRR, belonging to the family of response proteins to pathogens R and can be used as a sensor of the response to exogenous auxin and high-light stress (Huang et al., 2019). In microcuttings treated with C1-sm, the steady-state transcript level of both genes in the different rooting phases was similar to that observed in IBA-treated microcuttings, confirming that P. agglomerans C1 metabolites impact the timing of the auxin turnover rather than the accumulation of auxin.
In conclusion, the results obtained in this study show that the metabolites secreted by P. agglomerans C1 contain molecules that act in synergy with auxins and maintain an optimal gradient of this hormone, which positively affect the temporal pattern of de novo root formation, as well as root morphology and efficiency. Identification and characterization of these molecules will be useful to investigate the underlying mechanisms leading to the modification of AR development.
Data Availability Statement
The genome sequence of P. agglomerans C1 is available under NCBI with genome assembly number ASM975988v1.
Author Contributions
FL, LG, AF, RM, and MR contributed to the conception and design of the study. FL, LG, AF, CS, RM, and MR contributed to defining the methodology. FL, LG, AF, RM, and MR contributed to data validation. FL, AF, LG, GM, CS, FM, RM, and MR contributed to the investigation. FL, RM, and MR wrote the first draft of the manuscript. FL, FM, RM, and MR wrote and edited the final version of the manuscript. All authors have read and agreed to the published version of the manuscript. All authors contributed to the article and approved the submitted version.
Funding
LG was supported by a Ph.D. grant of the Minister for Education, University and Research (MIUR), Department of Excellence project SAFE-Med (Law 232/2016). The funder was not involved in the study design, collection, analysis, interpretation of data, the writing of this article, or the decision to submit it for publication.
Conflict of Interest
The authors declare that the research was conducted in the absence of any commercial or financial relationships that could be construed as a potential conflict of interest.
Footnotes
References
Abdollahi, H., Rugini, E., Ruzzi, M., and Muleo, R. (2004). In vitro system for studying the interaction between Erwinia amylovora and genotypes of pear. Plant Cell Tissue Organ Cult. 79, 203–212. doi: 10.1007/s11240-004-0661-0
Ahkami, A. H., Melzer, M., Ghaffari, M. R., Pollmann, S., Ghorbani, J., Shahinnia, F. M., et al. (2013). Distribution of indole-3-acetic acid in Petunia hybrida shoot tip cuttings and relationship between auxin transport, carbohydrate metabolism and adventitious root formation. Planta 238, 499–517. doi: 10.1007/s00425-013-1907-z
Arora, I., Sharma, M., and Tollefsbol, T. O. (2019). Combinatorial epigenetics impact of polyphenols and phytochemicals in cancer prevention and therapy. Int. J. Mol. Sci. 20:4567. doi: 10.3390/ijms20184567
Bustillo-Avendaño, E., Ibáñez, S., Sanz, O., Barros, J. A. S., Gude, I., Perianez-Rodriguez, J., et al. (2018). Regulation of hormonal control, cell reprogramming, and patterning during de novo root organogenesis. Plant Physiol. 176, 1709–1727. doi: 10.1104/pp.17.00980
Campobenedetto, C., Grange, E., Mannino, G., van Arkel, J., Beekwilder, J., Karlova, R., et al. (2020). A biostimulant seed treatment improved heat stress tolerance during cucumber seed germination by acting on the antioxidant system and glyoxylate cycle. Front. Plant Sci. 11:836. doi: 10.3389/fpls.2020.00836
Cardarelli, M., Rouphael, Y., Coppa, E., Hoagland, L., and Colla, G. (2020). Using microgranular-based biostimulant in vegetable transplant production to enhance growth and nitrogen uptake. Agronomy 10:842. doi: 10.3390/agronomy10060842
Carlos-Reyes, Á., López-González, J. S., Meneses-Flores, M., Gallardo-Rincón, D., Ruíz-García, E., Marchat, L. A., et al. (2019). Dietary compounds as epigenetic modulating agents in Cancer. Front. Genet. 10:79. doi: 10.3389/fgene.2019.00079
Cirillo, C., Rouphael, Y., El Nakhel, C., Pannico, A., and de Pascale, S. (2020). Controlled-release fertilizer type and granulated soil activator combination modulate growth and ornamental quality of two bedding plants. Acta Hortic. 1271, 371–378. doi: 10.17660/ActaHortic.2020.1271.51
Colla, G., Hoagland, L., Ruzzi, M., Cardarelli, M., Bonini, P., Canaguier, R., et al. (2017). Biostimulant action of protein hydrolysates: unraveling their effects on plant physiology and microbiome. Front. Plant Sci. 8:2202. doi: 10.3389/fpls.2017.02202
da Costa, C. T., de Almeida, M. R., Ruedell, C. M., Schwambach, J., Maraschin, F. S., and Fett-Neto, A. G. (2013). When stress and development go hand in hand: main hormonal controls of adventitious rooting in cuttings. Front. Plant Sci. 4:133. doi: 10.3389/fpls.2013.00133
Daud, N., Faizal, A., and Geelen, D. (2013). Adventitious rooting of Jatropha curcas L. is stimulated by phloroglucinol and by red LED light. In Vitro Cell. Dev. Biol. Plant 49, 183–190. doi: 10.1007/s11627-012-9486-4
de Azevedo, H. P. A., de Carvalho, A. M., Vidal, D. A., Dos Santos, H. O., and Doria, J. (2020). Rooting biostimulants for Coffea arabica L. cuttings. Coffee Sci. 15:e151635. doi: 10.25186/cs.V15i.1635
De Klerk, G. J., Guan, H., Huisman, P., and Marinova, S. (2011). Effects of phenolic compounds on adventitious root formation and oxidative decarboxylation of applied indoleacetic acid in Malus ‘Jork 9’. Plant Growth Regul. 63, 175–185. doi: 10.1007/s10725-010-9555-9
de Klerk, G. J., van der Krieken, W., and de Jong, J. C. (1999). Review–the formation of adventitious roots: new concepts, new possibilities. In Vitro Cell. Dev. Biol. Plant 35, 189–199. doi: 10.1007/s11627-999-0076-z
Dong, C., Wang, G., Du, M., Niu, C., Zhang, P., Zhang, X., et al. (2020). Biostimulants promote plant vigor of tomato and strawberry after transplanting. Sci. Hortic. 267:109355. doi: 10.1016/j.scienta.2020.109355
Druege, U., Franken, P., and Hajirezaei, M. R. (2016). Plant hormone homeostasis, signaling, and function during adventitious root formation in cuttings. Front. Plant Sci. 7:381. doi: 10.3389/fpls.2016.00381
Druege, U., Hilo, A., Pérez-Pérez, J. M., Klopotek, Y., Acosta, M., Shahinnia, F., et al. (2019). Molecular and physiological control of adventitious rooting in cuttings: phytohormone action meets resource allocation. Ann. Bot. 123, 929–949. doi: 10.1093/aob/mcy234
Duca, D., Lorv, J., Patten, C. L., Rose, D., and Glick, B. R. (2014). Indole-3-acetic acid in plant-microbe interactions. Antonie Van Leeuwenhoek 106, 85–125. doi: 10.1007/s10482-013-0095-y
Dutkiewicz, J., Mackiewicz, B., Lemieszek, M. K., Golec, M., and Milanowski, J. (2016). Pantoea agglomerans: a mysterious bacterium of evil and good. Part IV. Beneficial effects. Ann. Agric. Environ. Med. 23, 206–222. doi: 10.5604/12321966.1203879
Enders, T. A., and Strader, L. C. (2015). Auxin activity: past, present, and future. Am. J. Bot. 102, 180–196. doi: 10.3732/ajb.1400285
Fattorini, L., Hause, B., Gutierrez, L., Veloccia, A., Della Rovere, F., Piacentini, D., et al. (2018). Jasmonate promotes auxin-induced adventitious rooting in dark-grown Arabidopsis thaliana seedlings and stem thin cell layers by a cross-talk with ethylene signaling and a modulation of xylogenesis. BMC Plant Biol. 18:182. doi: 10.1186/s12870-018-1392-4
Frolinger, T., Herman, F., Sharma, A., Sims, S., Wang, J., and Pasinetti, G. M. (2018). Epigenetic modifications by polyphenolic compounds alter gene expression in the hippocampus. Biol. Open 7:10. doi: 10.1242/bio.035196
Garrido, G., Guerreo, J. R., Cano, E. A., Acosta, M., and Sanchez-Bravo, J. (2002). Origin and basipetal transport for IAA responsible for rooting of carnation cuttings. Physiol. Plant. 114, 303–312. doi: 10.1034/j.1399-3054.2002.1140217.x
Gleeson, M., Constantin, M., Carroll, B. J., and Mitter, N. (2014). MicroRNAs as regulators of adventitious root development. J. Plant Biochem. Biotechnol. 23, 339–347. doi: 10.1007/s13562-014-0269-3
Glick, B. R. (2014). Bacteria with ACC deaminase can promote plant growth and help to feed the world. Microbiol. Res. 169, 30–39. doi: 10.1016/j.micres.2013.09.009
Goda, H., Sawa, S., Asami, T., Fujioka, S., Shimada, Y., and Yoshida, S. (2004). Comprehensive comparison of auxin-regulated and brassinosteroid-regulated genes in Arabidopsis. Plant Physiol. 134, 1555–1573. doi: 10.1104/pp.103.034736
Guan, L., Murphy, A., Peer, W. A., Gan, L., Li, Y., and Ming, Z. (2015). Physiological and molecular regulation of adventitious root formation. Crit. Rev. Plant Sci. 34, 506–521. doi: 10.1080/07352689.2015.1090831
Guilfoyle, T. J., and Hagen, G. (2007). Auxin response factors. Curr. Opin. Plant Biol. 10, 453–460. doi: 10.1016/j.pbi.2007.08.014
Gulzar, B., Mujib, A., and Qadir Malik, M. (2020). “Plant tissue culture: agriculture and industrial applications” in Transgenic technology based value addition in plant biotechnology. eds. U. Kiran, M. Z. Abdin, and Kamaluddin (Cambridge, MA: Academic Press-Elsevier Inc), 25–49.
Gutierrez, L., Bussell, J. D., Pacurar, D. I., Schwambach, J., Pacurar, M., and Bellini, C. (2009). Phenotypic plasticity of adventitious rooting in Arabidopsis is controlled by complex regulation of auxin response factor transcripts and microRNA abundance. Plant Cell 21, 3119–3132. doi: 10.1105/tpc.108.064758
Gutierrez, L., Mongelard, G., Floková, K., Pacurar, D. I., Novák, O., Staswick, P., et al. (2012). Auxin controls Arabidopsis adventitious root initiation by regulating jasmonic acid homeostasis. Plant Cell 24, 2515–2527. doi: 10.1105/tpc.112.099119
Hazir, S., Shapiro-Ilan, D. I., Bock, C. H., and Laite, L. G. (2017). Trans-cinnamic acid and Xenorhabdus szentirmaii metabolites synergize the potency of some commercial fungicides. J. Invertebr. Pathol. 145, 1–8. doi: 10.1016/j.jip.2017.03.007
Huang, J., Zhao, X., and Chory, J. (2019). The Arabidopsis transcriptome responds specifically and dynamically to high light stress. Cell Rep. 29, 4186.e3–4199.e3. doi: 10.1016/j.celrep.2019.11.051
Iacona, C., and Muleo, R. (2010). Light quality affects in vitro adventitious rooting and ex vitro performance of cherry rootstock colt. Sci. Hortic. 125, 630–636. doi: 10.1016/j.scienta.2010.05.018
James, D. J., and Thurbon, I. J. (1981). Shoot and root initiation in vitro in the apple rootstock M9 and the promotive effects of phloroglucinol. J. Hortic. Sci. 56, 15–20. doi: 10.1080/00221589.1981.11514960
Jan, I., Sajid, M., Rab, A., Iqbal, A., Khan, O., Jamal, Y., et al. (2015). Effect of various concentrations of indole butyric acid (IBA) on olive cuttings. Mitt. Klosterneuburg 65, 49–55.
Jing, T., Ardiansyah, R., Xu, Q., Xing, Q., and Müller-Xing, R. (2020). Reprogramming of cell fate during root regeneration by transcriptional and epigenetic networks. Front. Plant Sci. 11:317. doi: 10.3389/fpls.2020.00317
Kubista, M., Andrade, J. M., Bengtsson, M., Forootan, A., Jonák, J., Lind, K., et al. (2006). The real-time polymerase chain reaction. Mol. Asp. Med. 27, 95–125. doi: 10.1016/j.mam.2005.12.007
Kurepin, L., Haslam, T., Lopez-Villalobos, A., Oinam, G., and Yeung, E. (2011). Adventitious root formation in ornamental plants: II. The role of plant growth regulators. Propag. Ornam. Plants 11, 161–171.
Lakehal, A., and Bellini, C. (2019). Control of adventitious root formation: insights into synergistic and antagonistic hormonal interactions. Physiol. Plant. 165, 90–100. doi: 10.1111/ppl.12823
Li, K., Liu, Z., Xing, L., Wei, Y., Mao, J., Meng, Y., et al. (2019). miRNAs associated with auxin signaling, stress response, and cellular activities mediate adventitious root formation in apple rootstocks. Plant Physiol. Biochem. 139, 66–81. doi: 10.1016/j.plaphy.2019.03.006
Li, S., Xue, L. G., Xu, S. J., Feng, H. Y., and An, L. Z. (2009). Mediators, genes and signaling in adventitious rooting. Bot. Rev. 75, 230–247. doi: 10.1007/s12229-009-9029-9
Li, S. B., Xie, Z. Z., Hu, C. G., and Zhang, J. Z. (2016). A review of auxin response factors (ARFs) in plants. Front. Plant Sci. 7:47. doi: 10.3389/fpls.2016.00047
Lotfi, M., Mars, M., and Werbrouck, S. (2019). Optimizing pear micropropagation and rooting with light emitting diodes and trans-cinnamic acid. Plant Growth Regul. 88, 173–180. doi: 10.1007/s10725-019-00498-y
Ludwig-Müller, J. (2011). Auxin conjugates: their role for plant development and in the evolution of land plants. J. Exp. Bot. 62, 1757–1773. doi: 10.1093/jxb/erq412
Luziatelli, F., Brunetti, L., Ficca, A. G., and Ruzzi, M. (2019b). Maximizing the efficiency of vanillin production by biocatalyst enhancement and process optimization. Front. Bioeng. Biotechnol. 7:279. doi: 10.3389/fbioe.2019.00279
Luziatelli, F., Ficca, A. G., Bonini, P., Muleo, R., Gatti, L., Meneghini, M., et al. (2020b). A genetic and metabolomic perspective on the production of indole-3-acetic acid by Pantoea agglomerans and use of their metabolites as biostimulants in plant nurseries. Front. Microbiol. 11:1575. doi: 10.3389/fmicb.2020.01475
Luziatelli, F., Ficca, A. G., Cardarelli, M. T., Melini, F., Cavalieri, A., and Ruzzi, M. (2020a). Genome sequencing of Pantoea agglomerans C1 provides insights into molecular and genetic mechanisms of plant growth-promotion and tolerance to heavy metals. Microorganisms 8:153. doi: 10.3390/microorganisms8020153
Luziatelli, F., Ficca, A. G., Colla, G., Baldassarre Švecová, E., and Ruzzi, M. (2019a). Foliar application of vegetal derived bioactive compounds stimulates the growth of beneficialbacteria and enhances microbiome biodiversity in lettuce. Front. Plant Sci. 10:60. doi: 10.3389/fpls.2019.00060
Luziatelli, F., Ficca, A. G., Colla, G., Švecová, E., and Ruzzi, M. (2016). Effects of a protein hydrolysate-based biostimulant and two micronutrient based fertilizers on plant growth and epiphytic bacterial population of lettuce. Acta Hortic. 1148, 43–48. doi: 10.17660/ActaHortic.2016
Luziatelli, F., Ficca, A. G., Melini, F., and Ruzzi, M. (2019b). Genome sequence of the plant growth-promoting rhizobacterium (PGPR) Pantoea agglomerans C1. Microbiol. Resour. Announc. 8:153. doi: 10.1128/mra.00828-19
Miller, J. H. (1972). Experiments in molecular genetics. Cold Spring Harbor, NY: Cold Spring Harbor Laboratory.
Murashige, T., and Skoog, F. (1962). A revised medium for rapid growth and bioassays with tobacco tissue cultures. Physiol. Plant. 15, 473–497.
Naija, S., Elloumi, N., Jbir, N., Ammar, S., and Kevers, C. (2008). Anatomical and biochemical changes during adventitious rooting of apple rootstocks MM 106 cultured in vitro. CR Biol. 331, 518–525. doi: 10.1016/j.crvi.2008.04.002
Nandi, S. K., Palni, L. M. S., and Rikhari, H. C. (1996). Chemical induction of adventitious root formation in Taxus baccata cuttings. Plant Growth Regul. 19, 117–122. doi: 10.1007/BF00024577
Overvoorde, P., Fukaki, H., and Beeckman, T. (2010). Auxin control of root development. Cold Spring Harb. Perspect. Biol. 2:a001537. doi: 10.1101/cshperspect.a001537
Pacurar, D. I., Perrone, I., and Bellini, C. (2014). Auxin is a central player in the hormone cross-talks that control adventitious rooting. Physiol. Plant. 151, 83–96. doi: 10.1111/ppl.12171
Patnaik, S., and Anupriya, A. (2019). Drugs targeting epigenetic modifications and plausible therapeutic strategies against colorectal cancer. Front. Pharmacol. 10:588. doi: 10.3389/fphar.2019.00588
Patten, C. L., and Glick, B. R. (2002). Role of Pseudomonas putida indoleacetic acid in development of the host plant root system. Appl. Environ. Microbiol. 68, 3795–3801. doi: 10.1128/aem.68.8.3795-3801.2002
Pistelli, L., Iacona, C., Miano, D., Cirilli, M., Colao, M. C., Mensuali-Sodi, A., et al. (2012). Novel Prunus rootstock somaclonal variants with divergent ability to tolerate waterlogging. Tree Physiol. 32, 355–368. doi: 10.1093/treephys/tpr135
Reed, B. M., De Noma, J., Wada, S., and Postman, J. (2012). “Micropropagation of pear (Pyrus sp.)” in Protocols for micropropagation of selected economically important horticultural plants. Vol. 994. eds. M. Lambardi, E. Ozudogru, and S. Jain (Totowa, NJ: Humana Press).
Robbins, A., Campidonica, M., and Burger, D. W. (1988). Chemical and biological stability of Indole-3-butyric acid (IBA) after long-term storage at selected temperatures and light regimes. J. Environ. Hortic. 6, 39–41. doi: 10.24266/0738-2898-6.2.39
Rouphael, Y., and Colla, G. (2018). Synergistic biostimulatory action: designing the next generation of plant biostimulants for sustainable agriculture. Front. Plant Sci. 9:1655. doi: 10.3389/fpls.2018.01655
Rouphael, Y., Lucini, L., Miras-Moreno, B., Colla, G., Bonini, P., and Cardarelli, M. (2020). Metabolomic responses of maize shoots and roots elicited by combinatorial seed treatments with microbial and non-microbial biostimulants. Front. Microbiol. 11:664. doi: 10.3389/fmicb.2020.00664
Ruedell, C. M., Rodrigues de Almeida, M., and Fett-Neto, A. G. (2015). Concerted transcription of auxin and carbohydrate homeostasis‐ related genes underlies improved adventitious rooting of microcuttings derived from far-red treated Eucalyptus globulus Labill mother plants. Plant Physiol. Biochem. 97, 11–19. doi: 10.1016/j.plaphy.2015.09.005
Ruzzi, M., and Aroca, R. (2015). Plant growth-promoting rhizobacteria act as biostimulants in horticulture. Sci. Hortic. 196, 124–134. doi: 10.1016/j.scienta.2015.08.042
Saia, S., Aissa, E., Luziatelli, F., Ruzzi, M., Colla, G., Fiacca, A. G., et al. (2020). Growth-promoting bacteria and arbuscular mycorrhizal fungi differentially benefit tomato and corn depending upon the supplied form of phosphorus. Mycorrhiza 30, 133–147. doi: 10.1007/s00248-009-9531-y
Saia, S., Colla, G., Raimondi, G., Di Stasio, E., Cardarelli, M., Bonini, P., et al. (2019). An endophytic fungi-based biostimulant modulated lettuce yield, physiological and functional quality responses to both moderate and severe water limitation. Sci. Hortic. 256:108595. doi: 10.1016/j.scienta.2019.108595
Semeradova, H., Montesinos, J. C., and Benkova, E. (2020). All roads lead to auxin: post-translational regulation of auxin transport by multiple hormonal pathways. Plant Comm. 1:100048. doi: 10.1016/j.xplc.2020.100048
Spaepen, S. (2015). “Plant hormones produced by microbes” in Principles of plant-microbe interactions. ed. B. Lugtenberg (Cham: Springer International Publishing), 247–256.
Stringlis, I. A., Zhang, H., Pieterse, C. M. J., Bolton, M. D., and de Jonge, R. (2018). Microbial small molecules ‐ weapons of plant subversion. Nat. Prod. Rep. 35, 410–433. doi: 10.1039/c7np00062f
Tereso, S., Miguel, C. M., Mascarenhas, M., Roque, A., Trindade, H., Maroco, J., et al. (2008). Improved in vitro rooting of Prunus dulcis Mill. cultivars. Biol. Plant. 52, 437–444. doi: 10.1007/s10535-008-0088-2
Thimann, K. V. (1939). Auxins and the inhibition of plant growth. Biol. Rev. 14, 314–337. doi: 10.1111/j.1469-185X.1939.tb00937.x
Tsukanova, K. A., Chebotar, V., Meyer, J. J. M., and Bibikova, T. N. (2017). Effect of plant growth-promoting Rhizobacteria on plant hormone homeostasis. S. Afr. J. Bot. 113, 91–102. doi: 10.1016/j.sajb.2017.07.007
Twaij, B. M., Jazar, Z. H., and Hasan, M. N. (2020). Trends in the use of tissue culture, applications and future aspects. Int. J. Plant Biol. 11:1. doi: 10.4081/pb.2020.8385
Umanzor, S., Jang, S., Antosca, R., Antosca, R., Yarish, C., et al. (2020). Optimizing the application of selected biostimulants to enhance the growth of Eucheumatopsis isiformis, a carrageenophyte with commercial value, as grown in land-based nursery systems. J. Appl. Phycol. 32, 1917–1922. doi: 10.1007/s10811-020-02091-7
Villacorta-Martín, C., Sánchez-García, A. B., Villanova, J., Cano, A., van de Rhee, M., de Haan, J., et al. (2015). Gene expression profiling during adventitious root formation in carnation stem cuttings. BMC Genomics 16:789. doi: 10.1186/s12864-015-2003-5
Waidmann, S., Sarkel, E., and Kleine-Vehn, J. (2020). Same same, but different: growth responses of primary and lateral roots. J. Exp. Bot. 71, 2397–2411. doi: 10.1093/jxb/eraa027
Walterson, A. M., and Stavrinides, J. (2015). Pantoea: insights into a highly versatile and diverse genus within the Enterobacteriaceae. FEMS Microbiol. Rev. 39, 968–984. doi: 10.1093/femsre/fuv027
Wang, Q. (1991). Factors affecting rooting of microcuttings of the pear rootstock BP10030. Sci. Hortic. 45, 209–213. doi: 10.1016/0304-4238(91)90065-7
Westfall, C. S., Sherp, A. M., Zubieta, C., Alvarez, S., Schraft, E., Marcellin, R., et al. (2016). Arabidopsis thaliana GH3.5 acyl acid amido synthetase mediates metabolic crosstalk in auxin and salicylic acid homeostasis. Proc. Natl. Acad. Sci. U. S. A. 113, 11917–11922. doi: 10.1073/pnas.1612635113
Wiesman, Z., Riov, J., and Epstein, E. (1988). Comparison of movement and metabolism of indole-3-acetic acid and indole-3-butyric acid in mungbean cuttings. Physiol. Plant. 74, 556–560. doi: 10.1111/j.1399-3054.1988.tb02018.x
Wilson, P. J., and Van Staden, J. (1990). Rhizocaline, rooting co-factors, and the concept of promoters and inhibitors of adventitious rooting ‐ a review. Ann. Bot. 66, 479–490. doi: 10.1093/oxfordjournals.aob.a088051
Woo, S., and Pepe, O. (2018). Microbial consortia: promising probiotics as plant biostimulants for sustainable agriculture. Front. Plant Sci. 9:1801. doi: 10.3389/fpls.2018.01801
Wu, Q. S., and Zou, Y. N. (2009). Mycorrhiza has a direct effect on reactive oxygen metabolism of drought-stressed citrus. Plant Soil Environ. 55, 436–442. doi: 10.17221/61/2009-pse
Xu, L. (2018). De novo root regeneration from leaf explants: wounding, auxin, and cell fate transition. Curr. Opin. Plant Biol. 41, 39–45. doi: 10.1016/j.pbi.2017.08.004
Yamakawa, T., Kurahashi, O., Ishida, K., Kato, S., Kodama, T., and Minoda, Y. (1979). Stability of indole-3-acetic acid to autoclaving, aeration and light illumination. Agric. Biol. Chem. 43, 879–880. doi: 10.1080/00021369.1979.10863551
Zhang, Y., and Qiu, S. (2015). Examining phylogenetic relationships of Erwinia and Pantoea species using whole genome sequence data. Antonie Van Leeuwenhoek 108, 1037–1046. doi: 10.1007/s10482-015-0556-6
Zhu, B., Shang, B., Li, Y., and Zhen, Y. (2016). Inhibition of histone deacetylases by trans-cinnamic acid and its antitumor effect against colon cancer xenografts in athymic mice. Mol. Med. Rep. 13, 4159–4166. doi: 10.3892/mmr.2016.5041
Keywords: plant-growth-promoting rhizobacteria, auxin, biostimulants, plant tissue culture, auxin-regulated genes, adventitious root, micropropagation
Citation: Luziatelli F, Gatti L, Ficca AG, Medori G, Silvestri C, Melini F, Muleo R and Ruzzi M (2020) Metabolites Secreted by a Plant-Growth-Promoting Pantoea agglomerans Strain Improved Rooting of Pyrus communis L. cv Dar Gazi Cuttings. Front. Microbiol. 11:539359. doi: 10.3389/fmicb.2020.539359
Edited by:
Ying Ma, University of Coimbra, PortugalReviewed by:
Brian H. Kvitko, University of Georgia, United StatesAmanda May Vivian Brown, Texas Tech University, United States
Copyright © 2020 Luziatelli, Gatti, Ficca, Medori, Silvestri, Melini, Muleo and Ruzzi. This is an open-access article distributed under the terms of the Creative Commons Attribution License (CC BY). The use, distribution or reproduction in other forums is permitted, provided the original author(s) and the copyright owner(s) are credited and that the original publication in this journal is cited, in accordance with accepted academic practice. No use, distribution or reproduction is permitted which does not comply with these terms.
*Correspondence: Rosario Muleo, bXVsZW9AdW5pdHVzLml0; Maurizio Ruzzi, cnV6emlAdW5pdHVzLml0
†These authors have contributed equally to this work