- 1College of Resource Sciences and Technology, Sichuan Agricultural University, Chengdu, China
- 2Safety and Environmental Protection Quality Supervision and Testing Research Institute, CNPC Chuanqing Drilling Engineering Co. Ltd., Guanghan, China
- 3Departamento de Microbiologia, Escuela Nacional de Ciencias Biologicas, Instituto Politecnico Nacional, Mexico City, Mexico
Waste drill cuttings (WDCs), produced during gas and oil drilling consisting of 80% rock cuttings and 20% drilling muds, are an increasingly potent source of environmental pollution. We studied the efficiency of bioaugmentation and phytoremediation to remediate WDCs in an experiment where WDCs were incubated in a greenhouse for 120 days with and without black locust (Robinia pseudoacacia) plant and with or without bacterial and fungal consortium inoculant. The pollutant removal rates were highest in inoculated and planted treatment, followed by inoculated treatment and planted treatment. The small decrease in contaminant level in the control treatment suggested that indigenous microorganisms in WDCs had little pollutant degradation capability. In the inoculated and planted treatments, after 120 days, the germination rate of red clover seeds was on the same level as in the natural soil, showing a marked decrease in the ecotoxicity of WDC. Both the bacterial and fungal richness and bacterial diversity increased in all the treatments over time, whereas fungal diversity increased only in the not-inoculated treatments. The activity of laccase enzyme played a key role in the bioremediation process. The enzyme activities were mostly governed by inoculated consortium and soil bacterial community, and black locust affected the bioremediation mainly through its effect on N content that further affected bacterial and fungal communities.
Highlights
- Waste drill cuttings (WDCs) are a potent source of environmental pollution.
- Bioremediation of WDCs with a combination of bioaugmentation and phytoremediation is an attractive strategy.
- Lignin degradation enzyme activities were the primary factors correlated to the contaminant removal in WDC bioremediation.
- Enzyme activities were mostly governed by inoculated consortium and soil bacterial community.
- Black locust affected the bioremediation through N content that affected bacterial and fungal communities.
Introduction
Waste drill cutting (WDC) produced during gas and oil drilling consists of 80% rock cuttings and 20% drilling muds. Drilling muds are applied to lubricate and cool the drill bit, maintain hydrostatic equilibrium, and move drill cuttings to the surface during gas and oil drilling (Okoro, 2011; Fattah and Lashin, 2016). To achieve desirable rheological properties and density, drilling mud is amended with additives, for example, with a liquid (water or diesel, mineral or synthetic oil), a weighting agent (barium or calcium sulfate), amargosite, sulfonated phenol formaldehyde (SPF) resin, and sulfonated lignite (SL) (Fink, 2012). However, using oils, SPF resin and SL create potential environmental hazards, for example, since these additives are ecotoxic and increase the chemical oxygen demand (COD). Approximately 2.5 million tons of WDCs are produced annually in China (Sun et al., 2016), making WDC an increasingly potent source of environmental pollution.
Among the remediation methods, bioremediation is considered an efficient, low-cost technology to treat polluted soils and sediments (Mutairi et al., 2008; Cerqueira et al., 2014). Bioremediation methods may be divided into five types—phytoremediation, biostimulation, bioaugmentation, natural attenuation, and bioventing—out of which the first three are most commonly used. Bioaugmentation involves inoculating exogenous contaminant-degrading microbes. In biostimulation, nutrients are added to stimulate the indigenous community and to avoid metabolic limitations. Phytoremediation involves the utilization of plants to extract, accumulate, degrade, filter, stabilize, and volatilize contaminants. Biostimulation, bioaugmentation, and phytoremediation approaches can be used in combination. The inoculated bacteria compete with the bacteria already present, and a successful establishment of the inoculum is required for long-term efficiency (Gkorezis et al., 2016). Vegetated soils are capable of supporting high microbial numbers and diversity, thus combining bioaugmentation with plants that provide nutrients for bacterial growth may affect the establishment of the inoculum (Gkorezis et al., 2016). Understanding how bioremediation affects the populations of pollutant-degrading microbes, the diversity and activity of the microbial community and the adaptability of exogenous microbes into the contaminated environment are essential in ensuring effective bioremediation (Kaplan and Kitts, 2004; Kauppi et al., 2011; Liu et al., 2012; Taccari et al., 2012). Bioaugmentation and biostimulation have been used to remediate WDC and waste drilling fluids (Rojas-Avelizapa et al., 2007; Chen et al., 2015; Avdalovic et al., 2016; Zha et al., 2017). To our knowledge, combined phytoremediation and bioaugmentation in WDC bioremediation has not been studied to date, and the relative contribution of changes in microbial communities to WDC bioremediation efficiency has not received attention. The objectives of this research were (1) to evaluate the efficiency of combined bioaugmentation and phytoremediation in organic pollutant degradation using a fungal and bacterial consortium as microbial inoculant and Robinia pseudoacacia (black locust), (2) to determine changes in microbial communities during the WDC bioremediation, and (3) to estimate the relative contributions of treatments and microbial communities on WDC bioremediation efficiency.
Materials and Methods
Soil Collection and Pretreatment
Water-based WDCs, produced in drilling gas wells, were collected from a WDC centralized treatment point in Deyang, China (E, 31°16′; N, 104°11′). Natural soil (NS) was collected from an agricultural field in Chengdu, China (E, 31°6′; N, 102°59′). The WDCs were loose and of dark gray color. Before the experiment, NS and WDC were dried and passed through a 2-mm sieve. The physicochemical properties of NS and WDC are in Supplementary Table S1.
Experimental Setup
The fungal consortium consisted of Pseudallescheria ellipsoidea WNF-15 (accession number MG976626), Stachybotrys chartarum WNF-20 (MG976627), and Scopulariopsis brevicaulis WNF-22 (MG976628). The three strains, with the ability to degrade drilling mud additives such as sulfonated lignite and sulfonated phenolic resin, were isolated and identified in a previous study (unpublished). The strains were cultivated separately in PDA liquid medium at 25°C (Sambrock and Russel, 2001) for 5 days, then inoculated onto a distilled solid medium (80% wheat bran, 20% rice bran, pH 7.0) at the ratio of 3% (v/w), and incubated at 25°C in the dark for 5–7 days. After a large amount of spores formed, equal quantities of the strains were mixed as the fungal inoculum with 3.6 × 107 CFU g–1.
The bacterial consortium consisted of Sinobaca sp. SCAU3 (accession number KP241934), Belliella pelovolcani JH3 (KX230135), Halomonas sp. JH4 (KX230136), and Bacillus halodurans JU5 (KX230139). The four strains are capable of degrading diesel and decreasing COD in waste drilling mud (Leng, 2013). The strains were cultivated in LB broth at 28°C (Sambrock and Russel, 2001) for 36 h. Cells were collected by centrifugation and resuspended in sterile water to gain an optical density of 1.0 at 600 nm, mixed in equal volumes, and used as the bacterial inoculum with 4.8 × 107 CFU ml–1.
Experiments were carried out in 4-L plastic [polyvinyl chloride (PVC)] pots (top diameter 40.6 cm, bottom diameter 14.4 cm, height 44.5 cm) with 4.5 kg WDC per pot. The experimental treatments were (1) WDC: WDC without inoculum or plant; (2) WDC + M: WDC inoculated with bacterial consortium in suspension (0.5% v/w) and solid fungal consortium (0.5% w/w); (3) WDC + P: WDC planted with one 1-year-old black locust (R. pseudoacacia) sapling per pot; and (4) WDC + M + P: WDC inoculated with the bacterial consortium in suspension (0.5% v/w) and solid fungal consortium (0.5% w/w) and planted with black locust. Each treatment was carried out in triplicate.
The pots were incubated in a greenhouse under simulated natural illumination conditions, the temperature was 21°C at day and 15°C at night, with a 16-h photoperiod. Soil moisture of each treatment was kept at 60% maximum water holding capacity by adding distilled water. The experiment was started in December 1, 2017.
Soil samples were collected at 0, 15, 30, 60, 90, and 120 days. Two hundred grams of the fresh sample was air-dried and sieved (0.25 mm) for determining physicochemical properties, 50 g was stored at 4°C for enzyme assays within 1 week, and 10 g was stored at −70°C for the subsequent microbial community analyses.
Total Nitrogen Analysis
Total nitrogen (TN) was analyzed by the semi-micro Kjeldahl method (Bremner, 1996), where 1 g of soil sample was digested with 1.1 g of K2SO4:CuSO4:Se (100:10:1 mass ratio) and 5 ml of H2SO4. The digestion solution was distilled in a semi-micro Kjeldahl apparatus and titrated with 0.005 M H2SO4.
Enzyme Activity Assays
Soil (4.0 g soil wet weight) was mixed with 40 ml of double-distilled water. The solution was incubated for 60 min in a rotary shaker at 120 rpm and centrifuged at 11,000 × g for 10 min at 4°C. The supernatant was collected for the enzyme assays.
Laccase (Lac) activity was determined by monitoring the oxidation of 2,2′-azino-bis(3-ethylbenzothiazoline-6-sulfonic acid) (ABTS) at 25°C (Wolfenden and Wilson, 1982) in a 2-ml reaction mixture containing 0.1 ml soil extract, B&R buffer (0.1 M acetic acid, 0.1 M boric acid, 0.1 M phosphoric acid, pH 5.0), and 1 mM ABTS. The absorbance of the solution was determined with a spectrophotometer (model 752, CANY, China), and Lac activity was calculated from the increase in absorbance at 420 nm (ε420 = 36,000 M–1cm–1). Manganese peroxidase (MnP) activity was determined by monitoring the formation of Mn3+-malonate complex in 50 mM sodium malonate buffer (pH 4.5) with 0.5 mM MnSO4 at 270 nm (ε = 11,590 M–1 cm–1) (Wariishi et al., 1992). The reaction was initiated by adding H2O2 to the final concentration of 0.1 mM. Lignin peroxidase (LiP) activity was determined by monitoring the oxidation of veratryl alcohol to veratraldehyde at 310 nm (ε = 9,300 M–1 cm–1) in a 1-ml reaction mixture containing 2 mM veratryl alcohol, 50 mM tartaric acid (pH 3), and 0.4 mM H2O2 (Tanaka et al., 2009). Enzyme absorption spectra were determined at room temperature in a cuvette with a 1-cm light path using spectrophotometer (model 752, CANY, China). One unit of enzymatic activity (U) was defined as the amount of enzyme that transformed 1 μmol of substrate per minute.
Pollutant Content and Ecotoxicity Analysis
Total petroleum hydrocarbon (TPH) and total organic carbon (TOC) content removal rates and decreases in COD were employed as indicators of bioremediation. The residual TPH was measured using a gravimetric method (Mishra et al., 2001). COD was measured using the rapid digestion spectrophotometry method in a 5B-3C (V8) dry thermostat reactor (Ministry of Ecology and Environment the People’s Republic of China, 2008). TOC was measured using the Walkley–Black method (Sparks et al., 1996).
Germination tests were done using the method of Saterbak et al. (2009). Red clover (Trifolium pratense) seeds were sterilized in 0.5% sodium hypochlorite solution for 20 min, then rinsed with sterile distilled water three times, and dried with sterilized filter paper. Fifty seeds were placed onto a 20-g wet weight sample of WDC in a plastic plate. Germination percentage was calculated after incubation at 25°C in the dark for 5 days. COD analysis and germination test included natural soil samples for comparison.
DNA Extraction and Sequencing
Samples for microbial community analysis were collected at days 0, 60, and 120. At day 0, planted samples were mixed with the respective WDC and WDC + M samples. DNA was extracted from 0.5 g using Fast DNA SPIN Kit (MP Biomedicals, Illkirch, France) following the manufacturer’s instructions. V3–V4 region of the bacterial 16S rRNA gene was amplified using the primers 338F and 806R (Lane, 1991; McBain et al., 2003), and the fungal 18S rRNA gene fragment was amplified using the primers 817F and 1196R (Borneman and Hartin, 2000) as described by Chen et al. (2016). The resulting amplicons were sequenced using Illumina MiSeq reagent kits V3 (600 cycles, MS-102-3003) (PE300 for bacteria, PE250 for fungi) and platform at Personal Biotechnology Co., Ltd., China. Sequences were analyzed using MOTHUR (version 1.34.0) (Schloss et al., 2009). Sequence reads were assigned to each sample according to sample-specific barcodes. Sequences were regarded as low quality and removed if they did not meet the following criteria: exact match to barcode and primers, sequences longer than 200 nucleotides without ambiguous base pairs, and high average quality score (Q ≥ 20).
After quality filtering, the 16S rRNA and 18S rRNA gene amplicons were clustered into operational taxonomic units (OTUs) at 97% nucleotide similarity. Taxonomic characterization of the representative sequences of the OTUs was done using the SILVA database (SILVA Release 123). The Simpson and Shannon diversity indexes were calculated using the Mothur program (version 1.34.0) (Schloss et al., 2009). The heatmap was produced using HemI (Heatmap Illustrator, v. 1.0). The sequences have been submitted to the NCBI Sequence Read Archive under accession numbers PRJNA601856 and PRJNA609003.
Statistical Analysis
Differences in chemical characteristics and enzyme activities between treatments over time were tested using three-way mixed ANOVA with plant and inoculation as the between-subjects factors and time as the within-subject factor, followed by computing simple interactions, simple main effects, and multiple pairwise comparisons in R v.3.6.3 with package rstatix (Kassambara, 2020b; R Core Team, 2020). Differences in COD and seed germination rate between the natural soil and the treatments over time were tested using two-way mixed ANOVA with group as the between-subjects factor and time as the within-subject factor, followed by computing simple main effects in R package rstatix. The results were visualized using R package ggpubr (Kassambara, 2020a). Spearman correlations between the dominant taxa and TOC removal rate were tested using one-way ANOVA and Fisher’s least significant difference (P < 0.05) in IBM SPSS Statistics for Windows 20.0 (IBM Corp., Armonk, NY, United States). Differences in microbial community composition based on Bray–Curtis dissimilarities were tested using permutational multivariate analysis of variance (PERMANOVA) and visualized using non-metric multidimensional scaling (NMDS) in PRIMER v7 (Anderson et al., 2008; Clarke and Gorley, 2015). Taxa that characterized the differences between treatments were identified using the linear discriminant analysis (LDA) effect size (LEfSe) method (Segata et al., 2011). The relationships between TN, bacterial and fungal communities, enzyme activities, and organic fraction removal were analyzed using partial least squares path modeling (PLS-PM) according to Tenenhaus et al. (2005) and Ai et al. (2018). The estimates of path coefficients and the coefficients of determination (R2) in the path model were validated using the package plspm (1,000 bootstraps) in R v.3.3.3 (R Core Team, 2017).
Results
Pollutant Removal, Germination Rate, and Nitrogen Content
The TPH content, TOC content, and the COD decreased with time in all treatments (Figure 1, Supplementary Figures S1, S2, and Supplementary Table S2). At day 120, TPH content was lower in the inoculated treatments WDC + M and WDC + M + P than in the not-inoculated treatments WDC and WDC + P (P < 0.05) (Figure 1), suggesting that inoculation with fungal and bacterial consortium enhanced the removal of TPH. Similarly, at day 120, TOC contents and COD were lower in the inoculated treatments than in the not-inoculated treatments (P < 0.05) (Supplementary Figures S1, S2). In addition, at day 120, TOC content was slightly lower in the planted and inoculated treatment than in its not planted counterpart (P < 0.05) (Supplementary Figure S1), and COD was slightly lower in the planted treatments than in their not planted counterparts (P < 0.05) (Supplementary Figure S2). In WDC + M + P, COD was on the same level as in the natural soil (Supplementary Figure S3). Both the TOC and COD decreases suggested that combining black locust with fungal and bacterial consortium inoculation enhanced the degradation of pollutant matter in WDC.
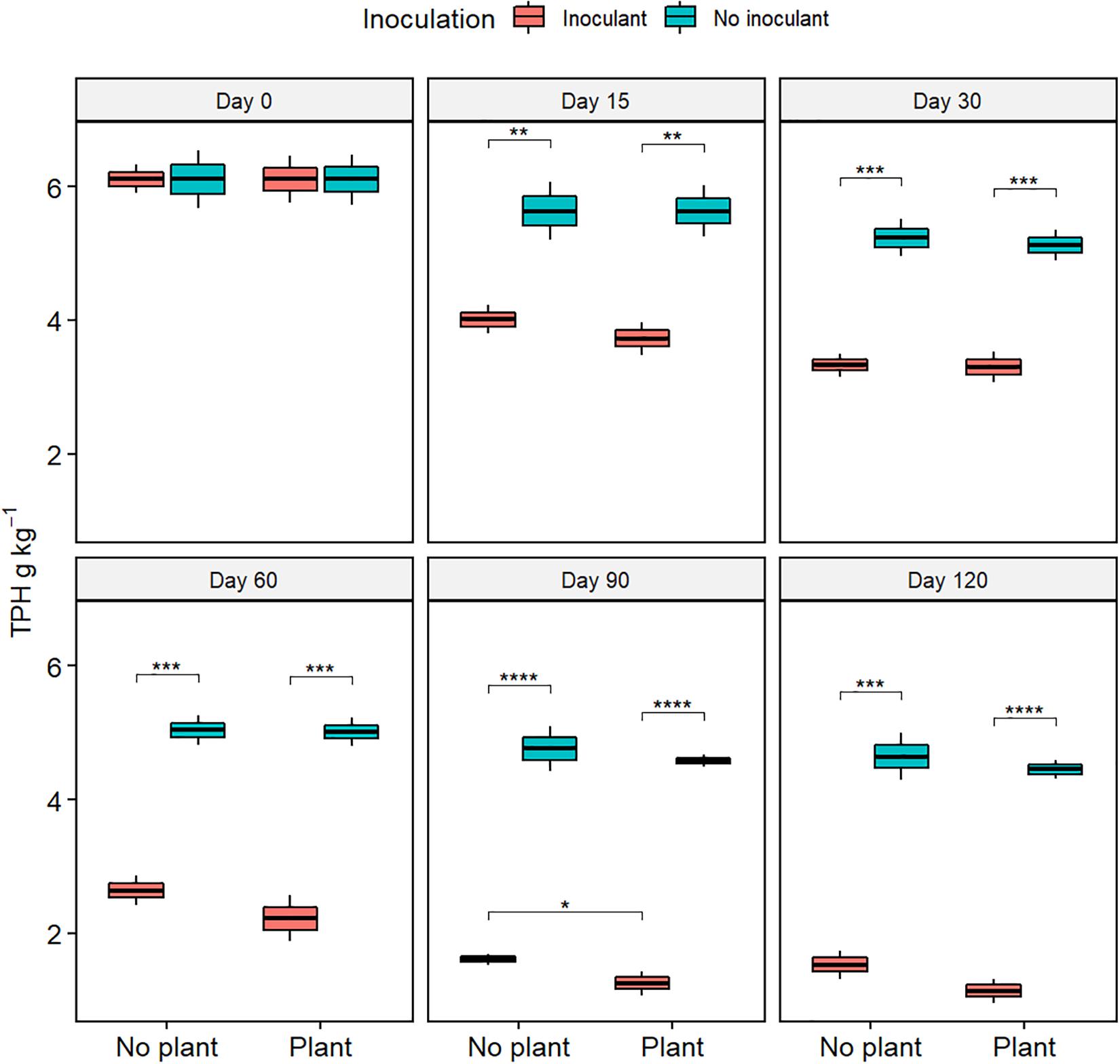
Figure 1. Total petroleum hydrocarbon (TPH) contents of soil extracts from waste drill cuttings (WDCs). WDCs were incubated in a greenhouse for 120 days with and without black locust (Robinia pseudoacacia) plant and with or without bacterial and fungal consortium inoculant. Statistically significant differences are indicated with asterisks: *P < 0.05; **P < 0.01; ***P < 0.001; ****P < 0.0001. The boxes show mean, lower and upper hinges indicate the first and third quartiles, and the whiskers indicate the ranges 1.5 times the interquartile range.
The germination rate of red clover seeds increased from the initial 0% in all treatments (Figure 2 and Supplementary Table S2), and the total nitrogen (TN) content increased with time in the planted treatments (Figure 3). At day 120, the germination rates in the inoculated treatments were on the same level as in the natural soil and in the not-inoculated treatments approximately half of that in natural soil (P ≤ 0.05) (Supplementary Figure S4), suggesting that the ecotoxicity of WDC had markedly decreased due to the inoculation. At day 120, TN content was highest in the inoculated and planted treatment (P ≤ 0.05) (Figure 3).
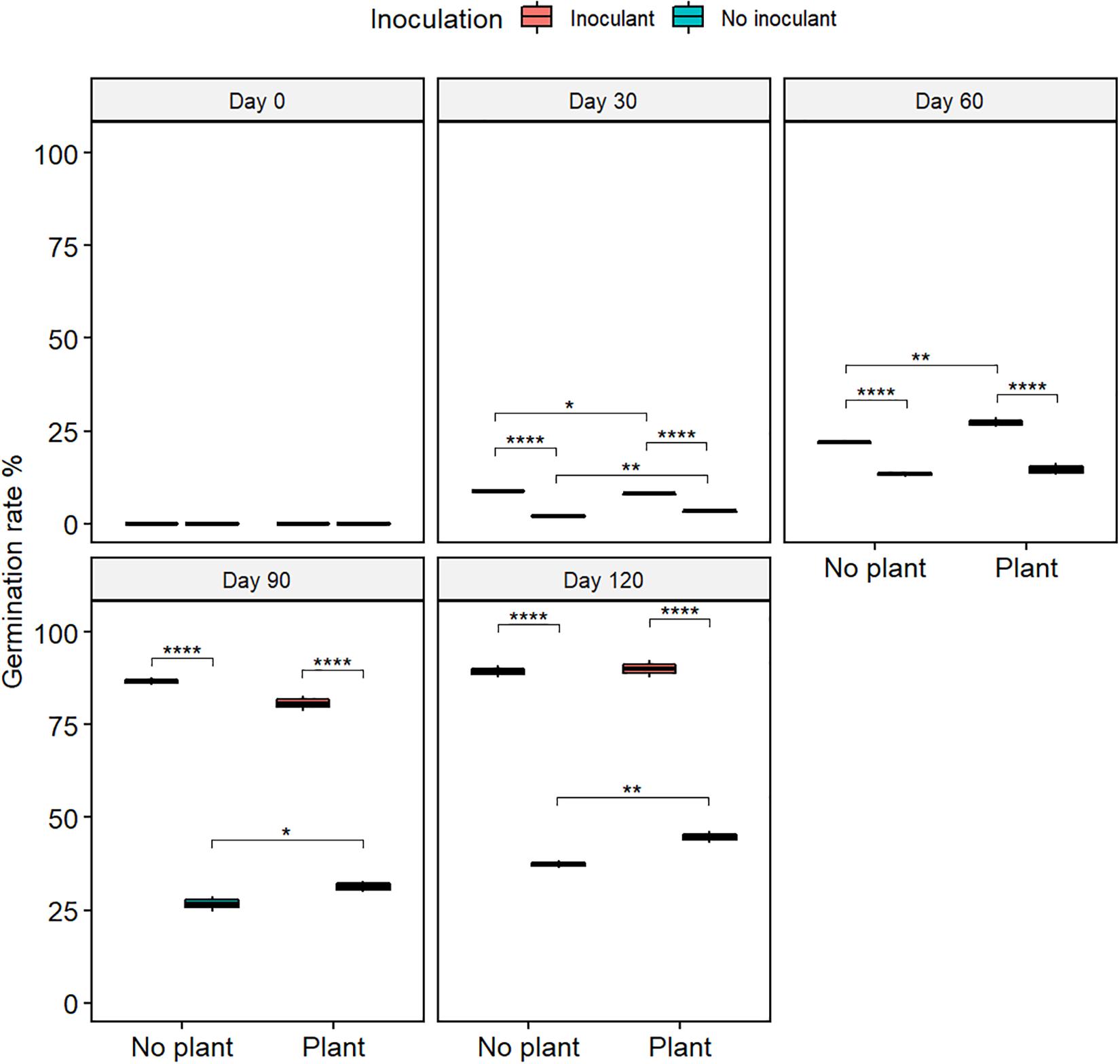
Figure 2. The germination rate of red clover seeds in waste drill cuttings (WDCs). WDCs were incubated in a greenhouse for 120 days with and without black locust (Robinia pseudoacacia) plant and with or without bacterial and fungal consortium inoculant. Statistically significant differences are indicated with asterisks: *P < 0.05; **P < 0.01; ****P < 0.0001. The boxes show mean, lower and upper hinges indicate the first and third quartiles, and the whiskers indicate the ranges 1.5 times the interquartile range.
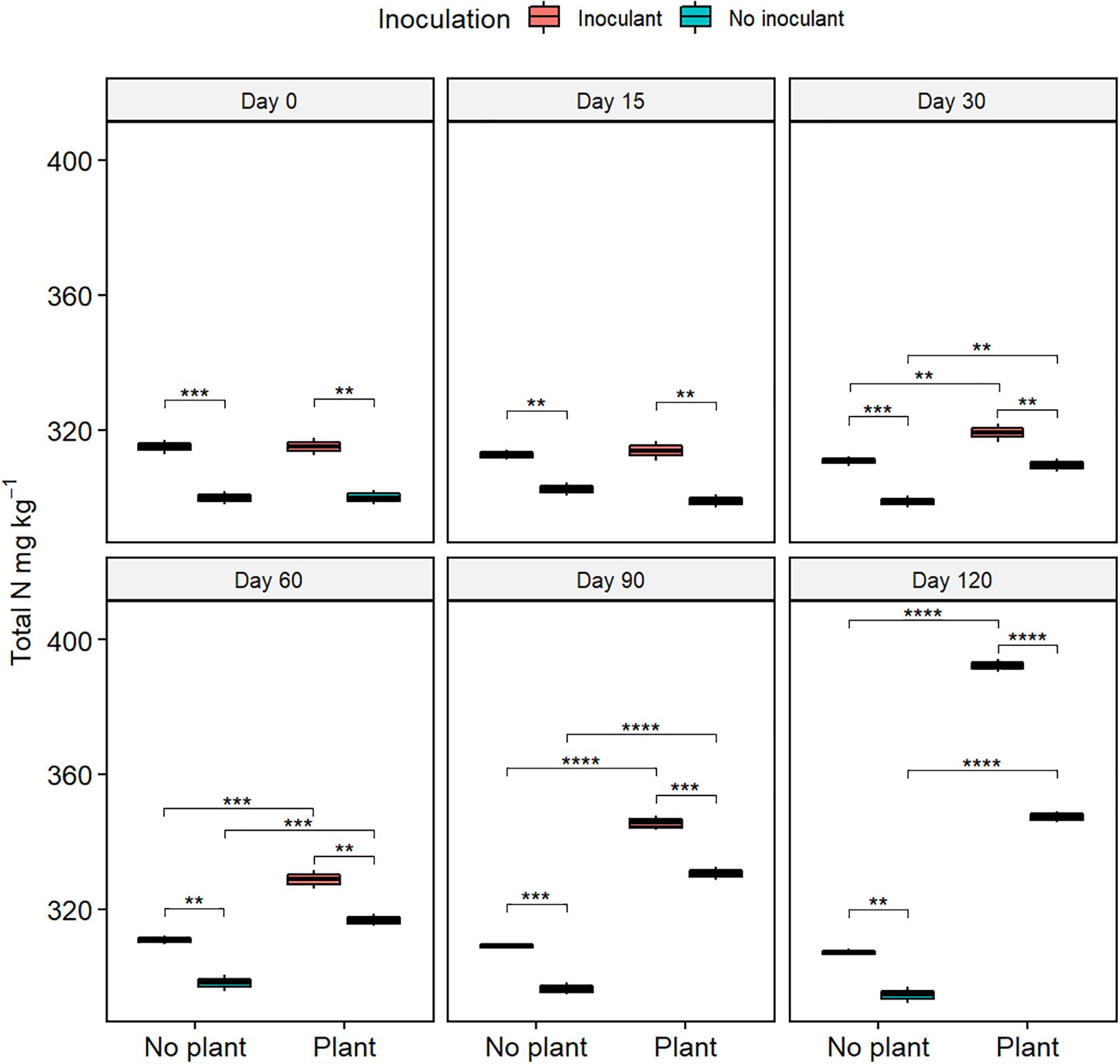
Figure 3. Total nitrogen (TN) content in waste drill cuttings (WDCs). WDCs were incubated in a greenhouse for 120 days with and without black locust (Robinia pseudoacacia) plant and with or without bacterial and fungal consortium inoculant. Statistically significant differences are indicated with asterisks: **P < 0.01; ***P < 0.001; ****P < 0.0001. The boxes show mean, lower and upper hinges indicate the first and third quartiles, and the whiskers indicate the ranges 1.5 times the interquartile range.
Enzyme Activity
Lac activity increased from the initial 0 in all treatments (Figure 4). At day 120, the Lac activity was highest in the inoculated treatments and lowest in WDC (P ≤ 0.05). MnP activity remained nearly unchanged in the not-inoculated treatments during the experiment (Supplementary Figure S5). In the inoculated treatments, MnP activity increased from day 15 to day 60, followed by a sharp decrease. Generally, MnP activity was highest in the WDC + M + P treatment. Changes in LiP activity showed no clear pattern (Supplementary Figure S6).
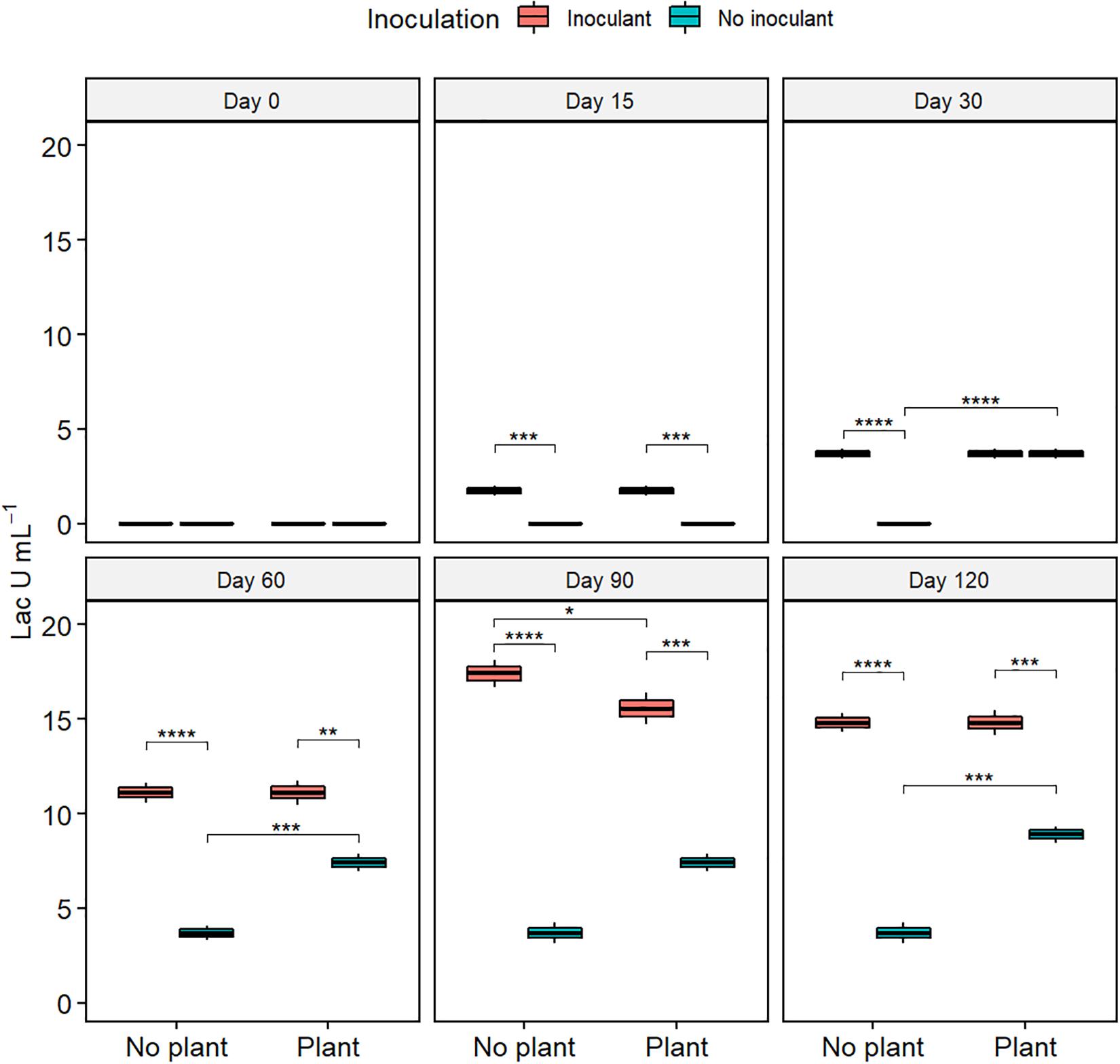
Figure 4. Activity of laccase (Lac) in waste drill cuttings (WDCs). WDCs were incubated in a greenhouse for 120 days with and without black locust (Robinia pseudoacacia) plant and with or without bacterial and fungal consortium inoculant. Statistically significant differences are indicated with asterisks: *P < 0.05; **P < 0.01; ***P < 0.001; ****P < 0.0001. The boxes show mean, lower and upper hinges indicate the first and third quartiles, and the whiskers indicate the ranges 1.5 times the interquartile range.
Microbial Community
The 16S rRNA gene amplicon sequencing resulted in 1,663,757 reads that were classified into 3,062 OTUs. Chao1 richness was lower in the beginning than after 60 and 120 days (P ≤ 0.05) (Supplementary Table S3). Shannon diversity and Pielou’s evenness were lowest in the beginning, and in all treatments highest and approximately on the same level at day 120 (P ≤ 0.05) (Supplementary Table S3). At the phylum level, the relative abundances of OTUs assigned into Proteobacteria, Actinobacteria, Chloroflexi, Bacteroidetes, and Gemmatimonadetes were high in all treatments, and communities at day 120 were distinct from those at days 0 and 60 (Figure 5A and Supplementary Figure S7). Based on the most abundant taxa, the communities clustered mostly based on time and less based on treatments (Figure 6A).
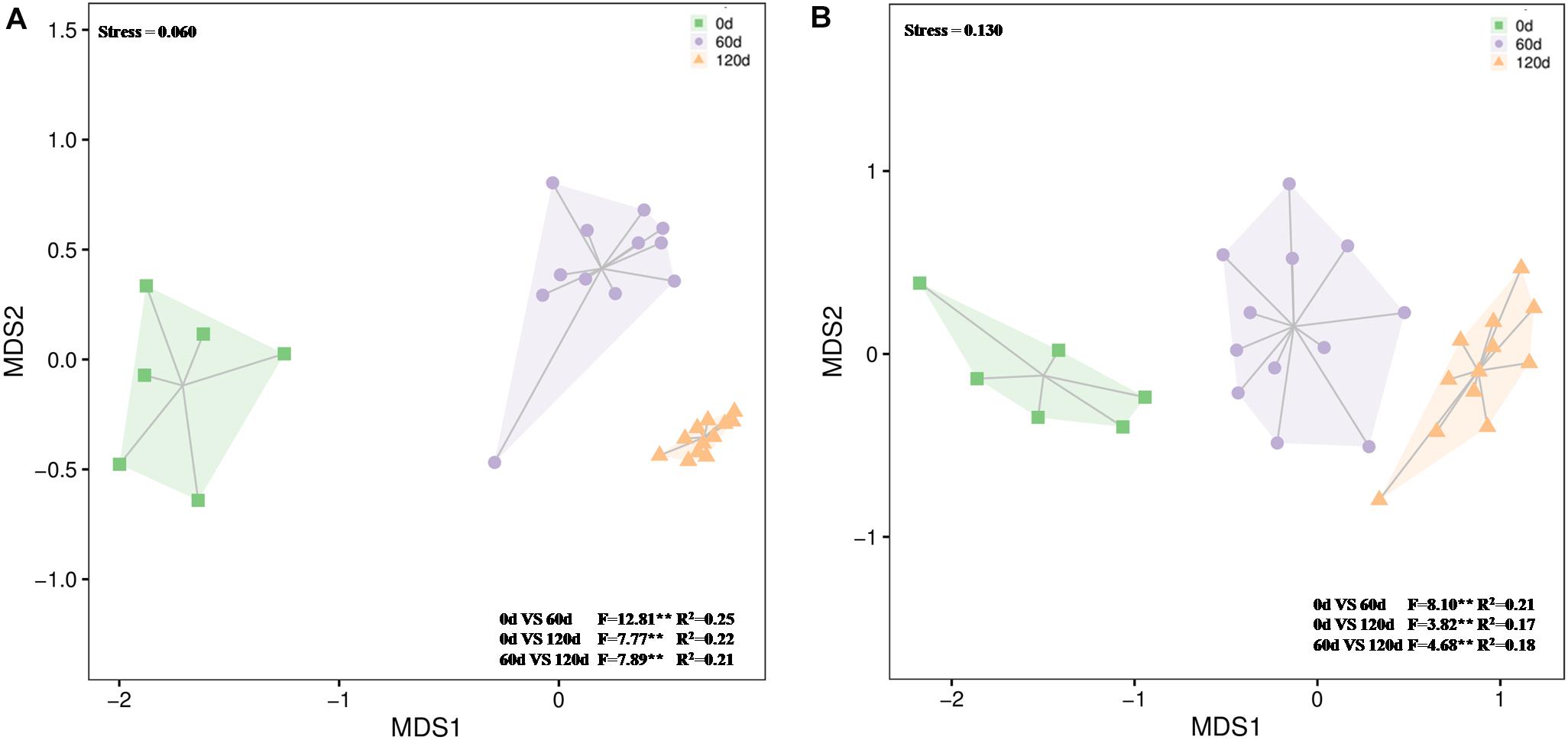
Figure 5. Differences in bacterial (A) and fungal (B) community composition over time in waste drill cuttings (WDCs). WDCs were incubated in a greenhouse for 120 days with and without black locust (Robinia pseudoacacia) plant and with or without bacterial and fungal consortium inoculant. The differences are based on Bray–Curtis dissimilarities and tested using permutational multivariate analysis of variance (PERMANOVA). **P < 0.01.
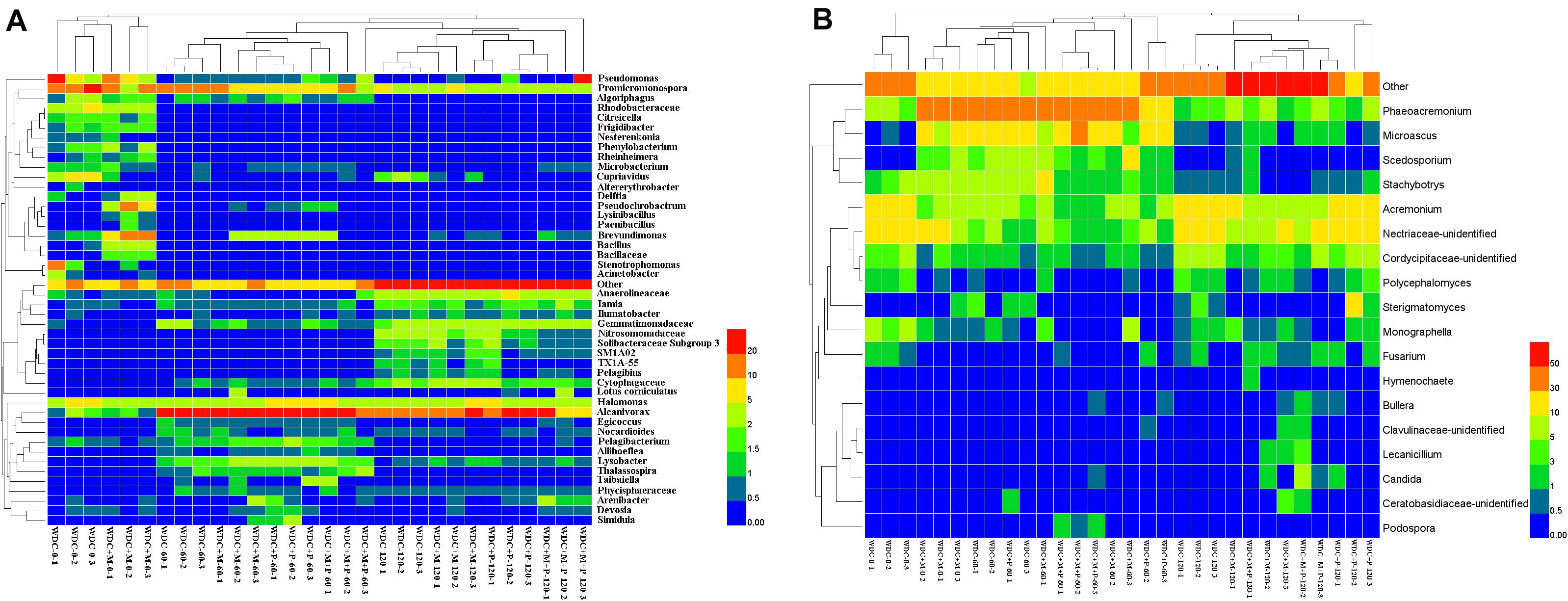
Figure 6. Dominant bacterial (A) and fungal (B) taxa in waste drill cuttings (WDCs). WDCs were incubated in a greenhouse for 120 days with and without black locust (Robinia pseudoacacia) plant and with or without bacterial and fungal consortium inoculant. WDC, no plant and no inoculant; WDC + M, no plant and inoculant; WDC + P, plant and no inoculant; WDC + M + P, plant and inoculant.
We identified the taxa characterizing the bacterial communities in different treatments using the LEfSe method. Most of the taxa with a large effect size (LDA score > 4.0) were associated with the initial stages (Supplementary Figure S7 and Supplementary Table S4). The relative abundance of Promicromonospora was high in WDC and WDC + M at day 0. The other taxa characterizing the initial stage WDC included Pseudomonas, Stenotrophomonas, Cupriavidus, and Rhodobacteraceae. Inoculant was seen as an increase in the relative abundance of Bacillus and changes in the relative abundances of other characterizing taxa, for example, Brevundimonas in the WDC + M treatment. The relative abundance of Alcanivorax was high in all treatments at days 60 and 120 and that of Halomonas relatively stable across all treatments over the whole experiment.
The 18S rRNA gene amplicon resulted in 1,820,720 reads that were classified into 458 OTUs. At day 120, Chao1 richness was higher in the not-inoculated treatments than in the WDC + M + P treatment, and Shannon diversity and Pielou’s evenness were highest (P ≤ 0.05) (Supplementary Table S3), indicating that over time, the inoculated fungal and bacterial consortium had affected the succession of the fungal community. At phylum level, the relative abundances of OTUs assigned to Ascomycota were high in all treatments, the relative abundances of Basidiomycota OTUs increased with time (Supplementary Figure S8), and communities at day 120 were distinct from those at days 0 and 60 (Figure 5B and Supplementary Figure S8). Based on the most abundant taxa, the community in the initial WDC was distinct from the rest, and communities at day 120 clustered together (Figure 6B).
Fungal taxa characteristic for different treatment–time combinations were identified in WDC and WDC + M at each time point and in WDC + P at day 120 (Supplementary Figure S8 and Supplementary Table S5). Unidentified Nectriaceae was most abundant in WDC at day 0 (Figure 6B). The relative abundances of Acremonium and unidentified Nectriaceae in WDC and those of Phaeoacremonium in WDC + M were high all through the experiment (Supplementary Table S5). Compared to WDC, inoculation approximately doubled the relative abundance of Stachybotrys and changed the relative abundances of other taxa, for example, Microascus in the WDC + M treatment at day 0.
Spearman correlations between abundant taxa and TOC removal rate were calculated to estimate the roles of the taxa in the bioremediation process. Bacillus and Stachybotrys that were in the inoculant correlated with TOC removal rate negatively and positively, respectively (P ≤ 0.05) (Table 1). In addition, four bacterial and two fungal taxa correlated positively and one fungus taxon negatively with TOC removal rate.
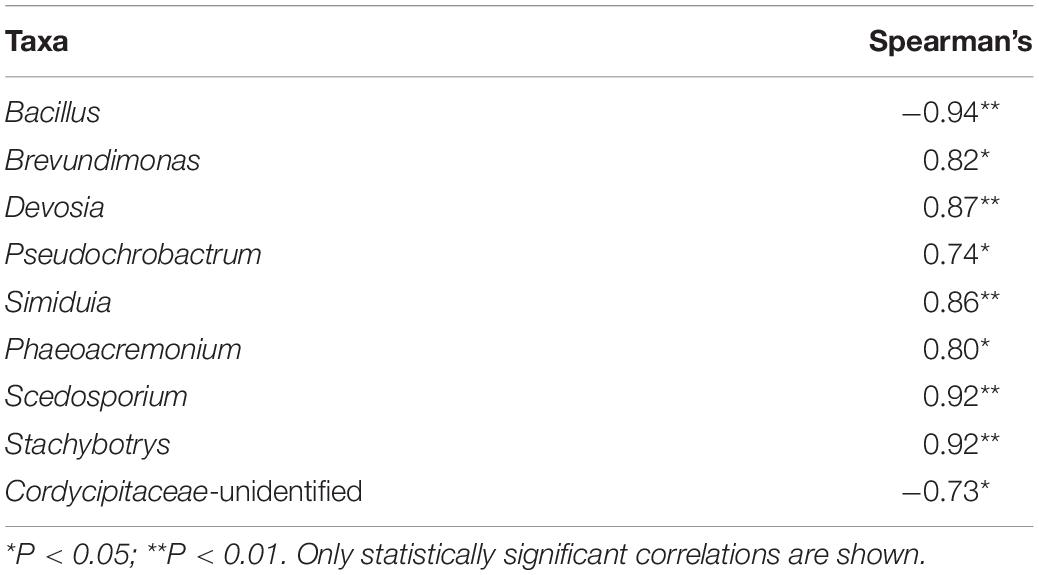
Table 1. Spearman correlation of total organic carbon removal rate and dominant taxa in remediating waste drill cuttings (WDCs).
Partial Least Squares Path Modeling Analysis
The relationships among treatment factors and measured variables were studied using PLS-PM. TPH, TOC, and COD, the decreases of which were employed as indicators of bioremediation, were combined into organic matter content in the model (Figure 7 and Supplementary Table S6). The combined three enzyme activities had a significant direct effect on the organic matter content. The enzyme activities were directly affected by bacterial community, microbial inoculant, fungal community, and black locust. TN had an indirect effect on enzyme activities through its direct effects on the bacterial and fungal communities. The microbial inoculant had negative direct effects on the bacterial and fungal communities. The black locust had a negative direct effect on the bacterial community but a positive direct effect on the fungal community. Both the microbial inoculant and black locust had a positive direct effect on the TN.
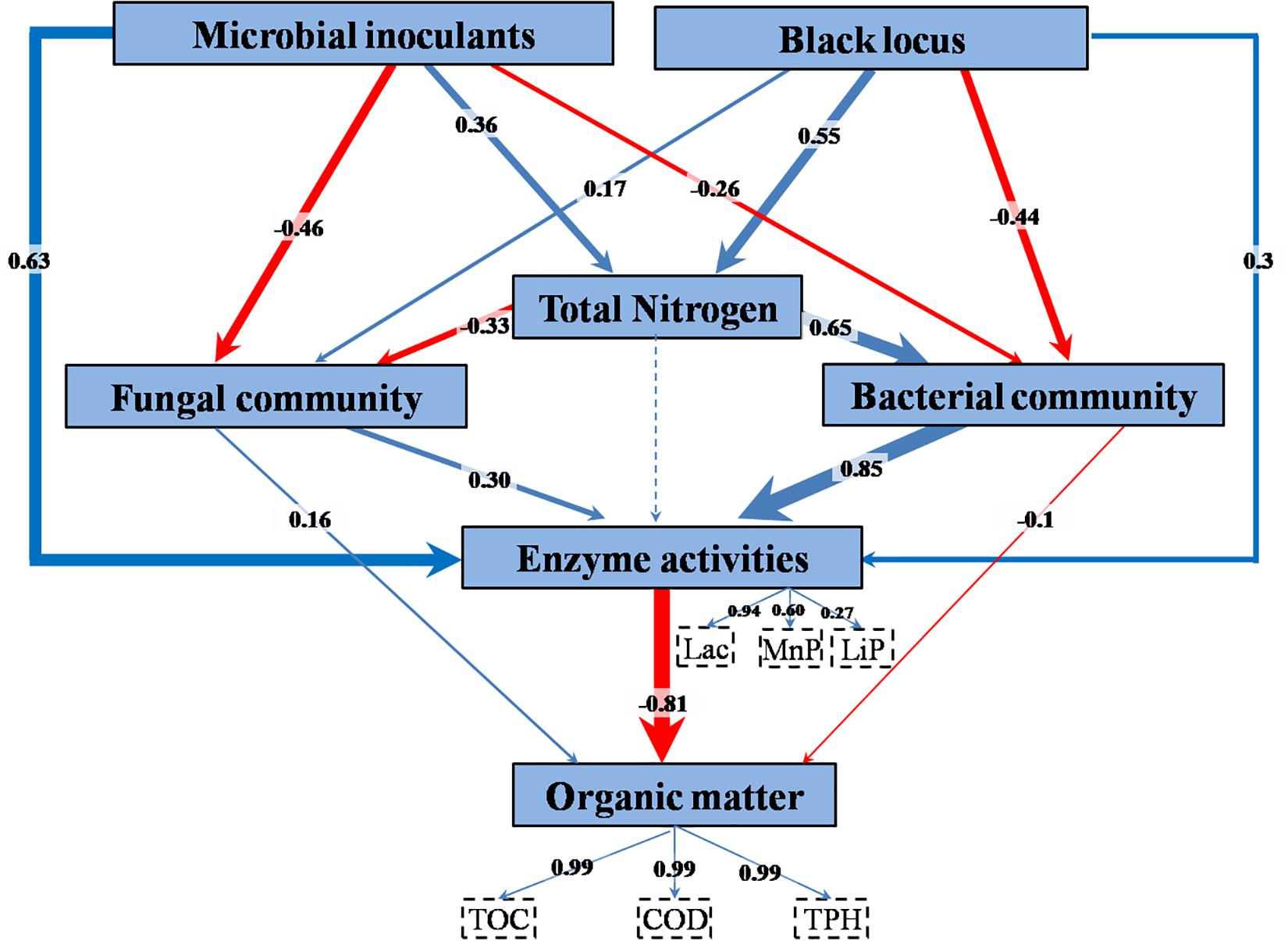
Figure 7. Partial least squares path model of remediating waste drill cuttings. A box represents an observed variable or a latent variable (i.e., a construct). The loadings for enzyme activities and organic removal rates that create the latent variables are shown in the dashed rectangles. Path coefficients are computed after 1,000 bootstraps and embodied in the width of the arrow, with blue and red indicating positive and negative effects, respectively. Dashed arrow shows a coefficient that did not differ significantly from 0 (P > 0.05). TN, total nitrogen content; Lac, laccase; MnP, manganese peroxidase; LiP, lignin peroxidase; TOC, total organic carbon content; COD, chemical oxygen demand; TPH, total petroleum hydrocarbon content.
Discussion
We studied the efficiency of bioaugmentation and phytoremediation to remediate WDCs in an experiment where WDCs were treated with a fungal and bacterial consortium as microbial inoculant and R. pseudoacacia (black locust), either individually or in a combination. TPH and TOC contents in the WDC and COD of WDC extract were measured as indicators of the bioremediation process.
The synergistic combinations of bioaugmentation and phytoremediation may promote contaminant removal (Escalante-Espinosa et al., 2005; Huang et al., 2005; Lin et al., 2008; Dashti et al., 2009; Gurska et al., 2009). In our study, the TPH and TOC contents and COD decreased slightly over time in the control treatment, suggesting that indigenous microorganisms in WDCs had little pollutant degradation capability, yet some of the decreases may have been due to sorption of oil and chemicals to the PVC pots employed in the experiment. The TPH content and COD decreased most in the inoculated treatments, and TOC removal rate was lowest in the control, suggesting that inoculation with the bacterial and fungal consortium and the black locust growth had enhanced the degradation of pollutants in WDC. Inoculant increased the contaminant removal more than the black locust, yet the inoculant and black locust combination was the most efficient remediation treatment possibly due to the root exudates in rhizosphere, which are known to stimulate contaminant-degrading microorganisms and to enhance biodegradation ability (Grayston et al., 1997; Walker et al., 2003; Kechavarzi et al., 2007; Neumann, 2007). The plant root system is not expected to spread in the soil as quickly as the microbial inoculum, which may explain why phytoremediation alone would need a longer treatment time than bioaugmentation, especially when the plant growth is affected by pollutants (Zhang et al., 2020).
In heavily hydrocarbon-contaminated environments, for example, in petroleum-contaminated soil, the C/N ratio imbalance due to high carbon and low nitrogen contents can affect microbial activity and bioremediation efficiency. Hence, the amount of N supplied is of vital importance for bioremediation (Rojas-Avelizapa et al., 2007; Alavi et al., 2014; Taiwo et al., 2015). In agreement, the N content and TOC removal rate correlated positively in our study, indicating that the lower C/N ratio had increased the efficiency of remediation. Direct fertilization could rapidly increase the amount of N in soil, and in heavily contaminated environments, the need for swift pollutant removal is paramount; biostimulation has been used successfully, for example, in remediating Exxon Valdez oil spill (Lindstrom et al., 1991; Venosa et al., 2010). However, it may bring about additional environmental problems for eutrophication of water bodies. In contrast, taking advantage of biological nitrogen fixation (BNF) is efficient and inexpensive and a sustainable way to increase the amount of N in soil. In our study, the black locust, a legume tree that forms symbiotically nitrogen-fixing nodules with rhizobia bacteria (Gilman and Watson, 1994; Bolat et al., 2015), increased soil N content in the black locust alone and black locust in combination with the inoculant treatments. The abundance of free-living nitrogen-fixing microorganisms was lower in black locust alone than in black locust in combination with the inoculant. In bioremediation, N content increase can be due to the activity of free-living nitrogen-fixing bacteria (Al-Mailem et al., 2019). In our study, possibly the inoculation had stimulated BNF by free-living microorganisms. The relative abundances of Halomonas and Bacillus that were in the inoculum, and those of Pseudomonas and Lysobacter, all of which include free-living nitrogen fixers (Seldin et al., 1983; Llamas et al., 2006; Yan et al., 2008), were higher with than without inoculum or plant, suggesting that these taxa may have influenced N content during the remediation process.
The lignin-degrading enzymes, including Lac, MnP, and LiP, play an important role in the bioremediation of pesticides, polycyclic aromatic hydrocarbons, and other xenobiotics (Karigar and Rao, 2011). Lac is abundant in soil and functions outside of the cells to facilitate bacterial and fungal degradation of pesticides, Polycyclic aromatic hydrocarbons (PAHs), and lignin (Yanto et al., 2017; Dandare et al., 2019; Muhammad et al., 2019). In our study, Lac activity correlated positively with the TOC removal rate and was highest in the inoculated treatments, suggesting that the activity was either brought on or stimulated by the inoculated bacterial and fungal consortium.
Both the bacterial and fungal richness and bacterial diversity and evenness indices increased in all the treatments over time, whereas the fungal diversity and evenness increased only in the not-inoculated treatments. As the contaminant removal was highest in the inoculated treatments, the less even fungal communities in the inoculated treatments might have been due to increased growth of contaminant-degrading fungi or contaminant-sensitive fungi. The dominant taxa that characterized the differences between bioremediation treatments and control included bacterial genera Pseudochrobactrum, Brevundimonas, and Bacillus and fungal genera Scedosporium, Stachybotrys, Microascus, and Acremonium. Pseudochrobactrum can degrade phenols (Mao et al., 2015), halogenated aromatics (Liu et al., 2016), and lignin (Shannon et al., 2017), and Brevundimonas has the ability to degrade phenols (Zhang et al., 2018). The dominant fungal genera have the ability to degrade lignin, cellulose, and PAHs (Santos et al., 2006; López-González et al., 2015). In our study, Brevundimonas, Phaeoacremonium, Scedosporium, and Stachybotrys, members of the inoculated consortium, correlated positively with TOC removal rate, suggesting that these four genera played major roles in WDC remediation. Out of the other detected inoculant consortium members, Bacillus peaked at day 0 and correlated negatively with TOC removal, suggesting that the adaptive ability of the inoculated Bacillus strain was poor in WDC. However, since relative abundances are not informative of absolute abundances (McLaren et al., 2019), determining the contribution of the inoculated strains would require quantitative analyses, for example, qPCR. Due to the differences in degradation efficiency between strains, determining the absolute contribution of the strains seems unattainable in an in vivo experiment.
The results above suggested that the inoculated consortium and the planting of black locust increased N content, enzyme activities, and macromolecular organic pollutant (SPF and SL) removal rate and affected microbial community. In a contaminated environment, the major factors in biological degradation of organic pollutants are the intracellular and extracellular enzymes produced by microbes (Tünde and Tien, 2000; Karigar and Rao, 2011). Phytoremediation, biostimulation, and bioaugmentation commonly increased the efficiency of bioremediation by increasing the activities of degradation enzymes (Mauricio-Gutiérrez et al., 2014; Chandanshive et al., 2018; Song et al., 2019). In agreement, in our study, the PLS-PM analysis showed that among the complex interrelationships between the factors, enzyme activities, especially that of Lac, played a key role in the bioremediation process. The enzyme activities were mostly governed by inoculated consortium and bacterial community, and black locust affected the bioremediation mainly through its effect on N content that further affected bacterial and fungal communities.
Conclusion
Compared with natural attenuation in the control treatment, bioaugmentation and phytoremediation individually and especially in combination enhanced contaminant removal from WDCs. The microbial inoculant affected the soil fungal and bacterial communities directly and planting of black locust indirectly via soil N content, yet most of their effect on the bioremediation process was indirect through enzyme activities.
Data Availability Statement
The sequences have been submitted to the NCBI Sequence Read Archive under accession numbers PRJNA601856 and PRJNA609003.
Author Contributions
HL, QC, and LD designed the experiments. LC and MH collected samples. HL, YL, and LZ carried out the experiments. KZ and XY analyzed the experimental results. YG analyzed the sequencing data and developed analysis tools. XZ assisted with Illumina sequencing. HL, EW, QC, and PP wrote the manuscript. All authors contributed to the article and approved the submitted version.
Funding
This work was financed by the 211 Discipline Construction Plans of Sichuan Agricultural University (Grant No. 03571873), the program of Safety Environmental Protection Quality Supervision and Testing Research Institute, CNPC Chuanqing Drilling Engineering Co., Ltd. (Grant No. CQ2019B-42-1-7), and the program of environmental protection technology integration and key equipment for shale gas and coalbed methane development (Grant No. 2016ZX05040006).
Conflict of Interest
HL, LC, and MH were employed by the company Safety and Environmental Protection Quality Supervision and Testing Research Institute, CNPC Chuanqing Drilling Engineering Co. Ltd.
The remaining authors declare that the research was conducted in the absence of any commercial or financial relationships that could be construed as a potential conflict of interest.
Supplementary Material
The Supplementary Material for this article can be found online at: https://www.frontiersin.org/articles/10.3389/fmicb.2020.536787/full#supplementary-material
Supplementary Figure 1 | Total organic carbon (TOC) contents of soil extracts from waste drill cuttings (WDCs). WDCs were incubated in a greenhouse for 120 days with and without black locust (Robinia pseudoacacia) plant and with or without bacterial and fungal consortium inoculant. Statistically significant differences are indicated with asterisks: ∗P < 0.05; ∗∗P < 0.01; ∗∗∗P < 0.001; ****P < 0.0001. The boxes show mean, lower and upper hinges indicate the first and third quartiles, and the whiskers indicate the ranges 1.5 times the interquartile range.
Supplementary Figure 2 | Chemical oxygen demand (COD) of soil extracts from waste drill cuttings (WDCs). WDCs were incubated in a greenhouse for 120 days with and without black locust (Robinia pseudoacacia) plant and with or without bacterial and fungal consortium inoculant. Statistically significant differences are indicated with asterisks: ∗P < 0.05; ∗∗P < 0.01; ∗∗∗P < 0.001; ****P < 0.0001. The boxes show mean, lower and upper hinges indicate the first and third quartiles, and the whiskers indicate the ranges 1.5 times the interquartile range.
Supplementary Figure 3 | Chemical oxygen demand (COD) of soil extracts from waste drill cuttings (WDCs) versus that in natural soil. WDCs were incubated in a greenhouse for 120 days with and without black locust (Robinia pseudoacacia) plant and with or without bacterial and fungal consortium inoculant. WDC, no plant and no inoculant; WDC + M, no plant and inoculant; WDC + P, plant and no inoculant; WDC + P + M, plant and inoculant. Statistically significant differences are indicated with asterisks: ∗P < 0.05; ∗∗P < 0.01; ∗∗∗P < 0.001; ****P < 0.0001. The boxes show mean, lower and upper hinges indicate the first and third quartiles, and the whiskers indicate the ranges 1.5 times the interquartile range.
Supplementary Figure 4 | The germination rate of red clover seeds in waste drill cuttings (WDCs) versus that in natural soil. WDCs were incubated in a greenhouse for 120 days with and without black locust (Robinia pseudoacacia) plant and with or without bacterial and fungal consortium inoculant. WDC, no plant and no inoculant; WDC + M, no plant and inoculant; WDC + P, plant and no inoculant; WDC + P + M, plant and inoculant. Statistically significant differences are indicated with asterisks: ∗P < 0.05; ∗∗P < 0.01; ∗∗∗P < 0.001; ****P < 0.0001. The boxes show mean, lower and upper hinges indicate the first and third quartiles, and the whiskers indicate the ranges 1.5 times the interquartile range.
Supplementary Figure 5 | Activity of manganese peroxidase (MnP) in waste drill cuttings (WDCs). WDCs were incubated in a greenhouse for 120 days with and without black locust (Robinia pseudoacacia) plant and with or without bacterial and fungal consortium inoculant. Statistically significant differences are indicated with asterisks: ∗P < 0.05; ∗∗P < 0.01; ∗∗∗P < 0.001; ****P < 0.0001. The boxes show mean, lower and upper hinges indicate the first and third quartiles, and the whiskers indicate the ranges 1.5 times the interquartile range.
Supplementary Figure 6 | Activity of lignin peroxidase (LiP) in waste drill cuttings (WDCs). WDCs were incubated in a greenhouse for 120 days with and without black locust (Robinia pseudoacacia) plant and with or without bacterial and fungal consortium inoculant. Statistically significant differences are indicated with asterisks: ∗P < 0.05; ∗∗P < 0.01; ∗∗∗P < 0.001; ****P < 0.0001. The boxes show mean, lower and upper hinges indicate the first and third quartiles, and the whiskers indicate the ranges 1.5 times the interquartile range.
Supplementary Figure 7 | Composition of bacterial community in waste drill cuttings (WDCs). WDCs were incubated in a greenhouse for 120 days with and without black locust (Robinia pseudoacacia) plant and with or without bacterial and fungal consortium inoculant. The relative abundances at phylum level on the left. Taxa characterizing the differences between treatments on the right, identified using the linear discriminant analysis (LDA) effect size (LEfSe) method. WDC, no plant and no inoculant; WDC + M, no plant and inoculant; WDC + P, plant and no inoculant; WDC + M + P, plant and inoculant.
Supplementary Figure 8 | Composition of fungal community in waste drill cuttings (WDCs). WDCs were incubated in a greenhouse for 120 days with and without black locust (Robinia pseudoacacia) plant and with or without bacterial and fungal consortium inoculant. The relative abundances at phylum level on the left. Taxa characterizing the differences between treatments on the right, identified using the linear discriminant analysis (LDA) effect size (LEfSe) method. WDC, no plant and no inoculant; WDC + M, no plant and inoculant; WDC + P, plant and no inoculant; WDC + M + P, plant and inoculant.
Supplementary Table 1 | Physicochemical properties of natural soil (NS) and waste drill cuttings prior the experiment [waste drill cutting (WDC)].
Supplementary Table 2 | Statistical significances of the differences between inoculation and plant treatments over time and their interactions in three-way mixed ANOVA. ∗ indicates P < 0.05.
Supplementary Table 3 | Richness and diversity of the bacterial and fungal communities in remediating waste drill cuttings (WDCs). WDCs were incubated in a greenhouse for 120 days with and without black locust (Robinia pseudoacacia) plant and with or without bacterial and fungal consortium inoculant.
Supplementary Table 4 | Relative abundances of dominant bacterial taxa in waste drill cuttings (WDCs). WDCs were incubated in a greenhouse for 120 days with and without black locust (Robinia pseudoacacia) plant and with or without bacterial and fungal consortium inoculant.
Supplementary Table 5 | Relative abundance of dominant fungal taxa in waste drill cuttings (WDCs). WDCs were incubated in a greenhouse for 120 days with and without black locust (Robinia pseudoacacia) plant and with or without bacterial and fungal consortium inoculant.
Supplementary Table 6 | The direct and indirect relationships between variables in the partial least squares path model (PLS-PM) of remediating waste drill cuttings. The path coefficients are calculated by PLS-PM after 1,000 bootstraps.
References
Ai, C., Zhang, S., Zhang, X., Guo, D., Zhou, W., and Huang, S. (2018). Distinct responses of soil bacterial and fungal communities to changes in fertilization regime and crop rotation. Geoderma 319, 156–166. doi: 10.1016/j.geoderma.2018.01.010
Alavi, N., Mesdaghinia, A. R., Naddafi, K., Mohebali, G., Daraei, H., Maleki, A., et al. (2014). Biodegradation of petroleum hydrocarbons in a soil polluted sample by oil-based drill cuttings. Soil Sediment Contamin. Int. J. 23, 586–597. doi: 10.1080/15320383.2014.847900
Al-Mailem, D. M., Kansour, M. K., and Radwan, S. S. (2019). Cross-bioaugmentation among four remote soil samples contaminated with oil exerted just inconsistent effects on oil-bioremediation[J]. Front. Microbiol. 2019:2827. doi: 10.3389/fmicb.2019.02827
Anderson, M. J., Gorley, R. N., and Clarke, K. R. (2008). PERMANOVA+ for PRIMER: Guide to Software and Statistical Methods. Plymouth: PRIMER-E Ltd.
Avdalovic, J., Duric, A., Miletic, S., Ilic, M., Milic, J., and Vrvic, M. M. (2016). Treatment of a mud pit by bioremediation. Waste Manag. Res. 34, 734–739. doi: 10.1177/0734242x16652961
Bolat, I., Kara, O., Sensoy, H., and Yüksel, K. (2015). Influences of Black Locust (Robinia pseudoacacia L.) afforestation on soil microbial biomass and activity. Iforest Biogeosci. For. 9, 171–177. doi: 10.3832/ifor1410-007
Borneman, J., and Hartin, R. E. J. (2000). PCR primers that amplify fungal rRNA genes from environmental samples. Appl. Environ. Microbiol. 66, 4356–4360. doi: 10.1128/aem.66.10.4356-4360.2000
Bremner, J. M. (1996). “Nitrogen—total,” in Methods of Soil Analysis. Part 3: Chemical Methods SSSA Book Series 5, ed. D. L. Sparks (Madison, WI: SSSA and ASA), 1085–1121.
Cerqueira, V. S., Peralba, M. C. R., Camargo, F. A. O., and Bento, F. M. (2014). Comparison of bioremediation strategies for soil impacted with petrochemical oily sludge. Int. Biodeterioration Biodegradation 95, 338–345. doi: 10.1016/j.ibiod.2014.08.015
Chandanshive, V. V., Kadam, S. K., Khandare, R. V., Kurade, M. B., Jeon, B. H., Jadhav, J. P., et al. (2018). In situ phytoremediation of dyes from textile wastewater using garden ornamental plants, effect on soil quality and plant growth. Chemosphere 210, 968–976. doi: 10.1016/j.chemosphere.2018.07.064
Chen, C., Zhang, J., Lu, M., Qin, C., Chen, Y., Yang, L., et al. (2016). Microbial communities of an arable soil treated for 8 years with organic and inorganic fertilizers. Biol. Fertil. Soils 52, 455–467. doi: 10.1007/s00374-016-1089-5
Chen, L. R., Huang, M., Jiang, X. B., Li, H., Chen, Q., Zhang, M., et al. (2015). Pilot tests of microbe-soil combined treatment of waste drilling sludge. Nat. Gas Ind. B 2, 270–276. doi: 10.1016/j.ngib.2015.07.021
Dandare, S. U., Young, J. M., Kelleher, B. P., and Allen, C. C. R. (2019). The distribution of novel bacterial laccases in alpine paleosols is directly related to soil stratigraphy. Sci. Total Environ. 671, 19–27. doi: 10.1016/j.scitotenv.2019.03.250
Dashti, N., Khanafer, M., Elnemr, I., Sorkhoh, N., Ali, N., and Radwan, S. (2009). The potential of oil-utilizing bacterial consortia associated with legume root nodules for cleaning oily soils. Chemosphere 74, 1354–1359. doi: 10.1016/j.chemosphere.2008.11.028
Escalante-Espinosa, E., Gallegos-Martınez, M. E., Favela-Torres, E., and Gutierrez Rojas, M. (2005). Improvement of the hydrocarbon phytoremediation rate by Cyperus laxus Lam. Inoculated with a microbial consortium in a model system. Chemosphere 59, 405–413. doi: 10.1016/j.chemosphere.2004.10.034
Fattah, K. A., and Lashin, A. (2016). Investigation of mud density and weighting materials effect on drilling fluid filter cake properties and formation damage. J. Afr. Earth Sci. 117, 345–357. doi: 10.1016/j.jafrearsci.2016.02.003
Fink, J. K. (2012). Petroleum Engineer’s Guide to Oil Field Chemicals and Fluids. Oxford: Elsevier, 1–59.
Gilman, F. E., and Watson, G. D. (1994). Robinia Pseudoacacia: Black Locust. United States Forest Service Fact Sheet ST-570. Available online at: http://hort.ifas.ufl.edu/database/documents/pdf/tree_fact_sheets/robpsea.pdf
Gkorezis, P., Daghio, M., Franzetti, A., Van, H. J. D., Sillen, W., and Vangronsveld, J. (2016). The interaction between plants and bacteria in the remediation of petroleum hydrocarbons: an environmental perspective. Front. Microbiol. 7:1836. doi: 10.3389/fmicb.2016.01836
Grayston, S. J., Vaughan, D., and Jones, D. (1997). Rhizosphere carbon flow in trees, in comparison with annual plants: the importance of root exudation and its impact on microbial activity and nutrient availability. Appl. Soil Ecol. 5, 29–56. doi: 10.1016/s0929-1393(96)00126-6
Gurska, J., Wang, W., Gerhardt, K. E., Khalid, A. M., Isherwood, D. M., Huang, X. D., et al. (2009). Three year field test of a plant growth promoting rhizobacteria enhanced phytoremediation system at a land farm for treatment of hydrocarbon waste. Environ. Sci. Technol. 43, 4472–4479. doi: 10.1021/es801540h
Huang, X. D., El-Alawi, Y., Gurska, J., Glick, B. R., and Greenberg, B. M. (2005). A multi-process phytoremediation system for decontamination of persistent total petroleum hydrocarbons (TPHs) from soils. Microchem. J. 81, 139–147. doi: 10.1016/j.microc.2005.01.009
Kaplan, C. W., and Kitts, C. L. (2004). Bacterial succession in a petroleum land treatment unit. Appl. Environ. Microbiol. 70, 1777–1786. doi: 10.1128/aem.70.3.1777-1786.2004
Karigar, C. S., and Rao, S. S. (2011). Role of microbial enzymes in the bioremediation of pollutants: a review. Enzyme Res. 7:805187.
Kassambara, A. (2020b). rstatix: Pipe-Friendly Framework for Basic Statistical Tests. R package version 0.6.0.
Kauppi, S., Sinkkonen, A., and Romantschuk, M. (2011). Enhancing bioremediation of diesel-fuel-contaminated soil in a boreal climate: comparison of biostimulation and bioaugmentation. Int. Biodeterioration Biodegradation 65, 359–368. doi: 10.1016/j.ibiod.2010.10.011
Kechavarzi, C., Pettersson, K., Leeds-Harrison, P., Ritchie, L., and Ledin, S. (2007). Root establishment of perennial ryegrass (L.perenne) in diesel contaminated subsurface soil layers. Environ. Pollut. 145, 68–74. doi: 10.1016/j.envpol.2006.03.039
Lane, D. J. (1991). “16S/23S rRNA sequencing,” in Nucleic Acid Techniques in Bacterial Systematic, eds E. Stackebrandt and M. Goodfellow (New York: John Wiley and Sons), 115–175.
Leng, X. J. (2013). Isolation and Characterization of Composite Pollution Degrading Bacteria from Polymer-Sulphated Drilling Slurry. Sichuan: University of Sichuan Agricultural, 45–58.
Lin, X., Li, X., Li, P., Li, F., Zhang, L., and Zhou, Q. (2008). Evaluation of plant–microorganism synergy for the remediation of diesel fuel contaminated soil. Bull. Environ. Contamin. Toxicol. 81, 19–24. doi: 10.1007/s00128-008-9438-1
Lindstrom, J. E., Prince, R. C., Clark, J. C., Grossman, M. J., Yeager, T. R., Braddock, J. F., et al. (1991). Microbial populations and hydrocarbon biodegradation potentials in fertilized shoreline sediments affected by the T/V Exxon Valdez oil spill. Appl. Environ. Microbiol. 57, 2514–2522. doi: 10.1128/aem.57.9.2514-2522.1991
Liu, P. W. G., Wang, S. Y., Huang, S. G., and Wang, M. Z. (2012). Effects of soil organic matter and ageing on remediation of diesel oil-contaminated soil and on the microbial ecology. Environ. Technol. 33, 2661–2672. doi: 10.1080/09593330.2012.673017
Liu, X. M., Gou, Z. J., Hu, Q., Wang, J. H., Chen, K., Xu, C. F., et al. (2016). Hydrolytic dehalogenation of the chlorothalonil isomer o-tetrachlorophthalonitrile by Pseudochrobactrum sp. bsq-1. Int. Biodeterioration Biodegradation 107, 42–47. doi: 10.1016/j.ibiod.2015.11.006
Llamas, I., Moral, A. D., Martínez-Checa, F., Arco, Y., Arias, S., and Quesada, E. (2006). Halomonas maura is a physiologically versatile bacterium of both ecological and biotechnological interest. Antonie Van Leeuwenhoek 89, 395–403. doi: 10.1007/s10482-005-9043-9
López-González, J. A., Vargas-García, M. D. C., López, M. J., Suárez-Estrella, F., Jurado, M. D. M., and Moreno, J. (2015). Biodiversity and succession of mycobiota associated to agricultural lignocellulosic waste-based composting. Bioresour. Technol. 187, 305–313. doi: 10.1016/j.biortech.2015.03.124
Mao, Z., Yu, C., and Xin, L. (2015). Enhancement of phenol biodegradation by Pseudochrobactrum sp. through ultraviolet-induced mutation. Int. J. Mol. Sci. 16, 7320–7333. doi: 10.3390/ijms16047320
Mauricio-Gutiérrez, A., Jiménez, S. T., Tapia, H. A., Cavazos, A. J., and Pérez, A. B. (2014). Biodegradation of hydrocarbons exploiting spent substrate from pleurotus ostreatus in agricultural soils. Afr. J. Biotechnol. 13, 3385–3393. doi: 10.5897/ajb2014.13964
McBain, A. J., Bartolo, R. G., Catrenich, C. E., Charbonneau, D., Ledder, R. G., Rickard, A. H., et al. (2003). Microbial characterization of biofilms in domestic drains and the establishment of stable biofilm microcosms. Appl. Environ. Microbiol. 69, 177–185. doi: 10.1128/aem.69.1.177-185.2003
McLaren, M. R., Willis, A. D., and Callahan, B. J. (2019). Consistent and correctable bias in metagenomic sequencing experiments. eLife 8:e46923. doi: 10.7554/eLife.46923
Ministry of Ecology and Environment the People’s Republic of China (2008). Water Quality-Determination of the Chemical Oxygen Demand-Fast Digestion-Spectrophotometric Method. HJ/T 399-2007. Beijing: Ministry Of Ecology And Environment The People’s Republic Of China.
Mishra, S., Jyot, J., Kuhad, R. C., and Lal, B. (2001). In situ bioremediation potential of an oily sludge-degrading bacterial consortium. Curr. Microbiol. 43, 328–335. doi: 10.1007/s002840010311
Muhammad, B., Hafiz, M. N. I., and Damiá, B. (2019). Persistence of pesticides-based contaminants in the environment and their effective degradation using laccase-assisted biocatalytic systems. Sci. Total Environ. 695:133896. doi: 10.1016/j.scitotenv.2019.133896
Mutairi, N., Bufarsan, A., and Rukaibik, F. (2008). Ecorisk evaluation and treatability potential of soils contaminated with petroleum hydrocarbon-based fuels. Chemosphere 74, 142–148. doi: 10.1016/j.chemosphere.2008.08.020
Neumann, G. (2007). Root Exudates and Nutrient Cycling// Nutrient Cycling in Terrestrial Ecosystems. Berlin: Springer, 123–157.
Okoro, C. (2011). Aerobic degradation of synthetic based drilling mud base fluids by Gulf of Guinea sediments under natural environmental conditions. Life Sci. J. 8, 569–576.
R Core Team (2017). R: A Language and Environment for Statistical Computing. Vienna: R Foundation for Statistical Computing.
R Core Team (2020). R: A Language and Environment for Statistical Computing. Vienna: R Foundation for Statistical Computing.
Rojas-Avelizapa, N. G., Roldán, C. T., Zegarra, M. H., MuOz, C. A. M., and Fernández, L. L. C. (2007). A field trial for an ex-situ bioremediation of a drilling mud-polluted site. Chemosphere 66, 1595–1600. doi: 10.1016/j.chemosphere.2006.08.011
Sambrock, J., and Russel, D. (2001). Molecular cloning: a laboratory manual, 3rd ed. Immunology 49, 895–909.
Santos, C., Sílvia, F., Valentim, H., and Phillips, A. (2006). Phenotypic characterisation of Phaeoacremonium and Phaeomoniella strains isolated from grapevines: enzyme production and virulence of extra-cellular filtrate on grapevine calluses. Sci. Hortic. 107, 123–130. doi: 10.1016/j.scienta.2005.04.014
Saterbak, A., Toy, R. J., Wong, D. C. L., McMain, B. J., Williams, M. P., Dorn, P. B., et al. (2009). Ecotoxicological and analytical assessment of hydrocarbon-contaminated soils and application to ecological risk assessment. Environ. Toxicol. Chem. 18, 1591–1607. doi: 10.1002/etc.5620180735
Schloss, P. D., Westcott, S. L., Ryabin, T., Hall, J. R., Hartmann, M., Hollister, E. B., et al. (2009). Introducing mothur: opensource, platform-independent, community-supported software for describing and comparing microbial communities. Appl. Environ. Microbiol. 75, 7537–7541. doi: 10.1128/aem.01541-09
Segata, N., Izard, J., Waldron, L., and Dirk, G. (2011). Metagenomic biomarker discovery and explanation. Genome Biol. 12:R60. doi: 10.1186/gb-2011-12-6-r60
Seldin, L., Elsas, J. D. V., and Penido, E. G. C. (1983). Bacillus nitrogen fixers from Brazilian soils. Plant Soil 70, 243–255. doi: 10.1007/bf02374784
Shannon, J. C., Yu, C. W., Josh, T. C., Steven, W. S., Blake, A. S., Michael, P. T., et al. (2017). Development and characterization of a thermophilic, lignin degrading microbiota. Process Biochem. 63, 193–203. doi: 10.1016/j.procbio.2017.08.018
Song, N., Xu, C. H., Yan, Z. S., Yang, T., Wang, C. H., and Jiang, H. L. (2019). Improved lignin degradation through distinct microbial community in subsurface sediments of one eutrophic lake. Renew. Energy 138, 861–869. doi: 10.1016/j.renene.2019.01.121
Sparks, D. L., Helmke, P. A., Loeppert, R. H., Soltanpour, P. N., Tabatabai, M. A., Johnston, C. T., et al. (1996). Methods of Soil Analysis, Part 3, Chemical Methods, SSSA Bookseries No 5. Madison: SSSA.
Sun, L., Yao, C. S., Zhang, T., Gong, J., and Branch, Z. D. (2016). High efficient and harmless drilling waste mud treatment process. Petrochem. Ind. Technol. 2:93.
Taccari, M., Milanovic, V., Comitini, F., Casucci, C., and Ciani, M. (2012). Effects of biostimulation and bioaugmentation on diesel removal and bacterial community. Int. Biodeterioration Biodegradation 66, 39–46. doi: 10.1016/j.ibiod.2011.09.012
Taiwo, A. M., Gbadebo, A. M., Oyedepo, J. A., Ojekunle, Z. O., and Taiwo, O. T. (2015). Bioremediation of industrially contaminated soil using compost and plant technology. J. Hazard. Mater. 304, 166–177. doi: 10.1016/j.jhazmat.2015.10.061
Tanaka, H., Koike, K., and Itakura, S. (2009). Degradation of wood and enzyme production by ceriporiopsis subvermispora. Enzyme Microb. Technol. 45, 384–390. doi: 10.1016/j.enzmictec.2009.06.003
Tenenhaus, M., Vinzi, V. E., Chatelin, Y. M., and Lauro, C. (2005). PLS path modeling. Comput. Stat. Data Anal. 48, 159–205.
Tünde, M., and Tien, M. (2000). Oxidation mechanism of ligninolytic enzymes involved in the degradation of environmental pollutants. Int. Biodeterioration Biodegradation 46, 51–59. doi: 10.1016/s0964-8305(00)00071-8
Venosa, A. D., Campo, P., and Suidan, M. T. (2010). Biodegradability of lingering crude oil 19 years after the exxon valdez oil spill. Environ. Sci. Technol. 44, 7613–7621. doi: 10.1021/es101042h
Walker, T. S., Bais, H. P., Grotewold, E., and Vivanco, J. M. (2003). Root exudation and rhizosphere biology. Plant Physiol. 132:44. doi: 10.1104/pp.102.019661
Wariishi, H., Valli, K., and Gold, M. H. (1992). Manganese(ii) oxidation by manganese peroxidase from the basidiomycete Phanerochaete chrysosporium. kinetic mechanism and role of chelators. J. Biol. Chem. 267, 23688–23695.
Wolfenden, B. S., and Wilson, R. L. (1982). Radical cations as reference chromogens in studies of one-electron transfer reactions: pulse radiolysis studies of 2,29-azinobis-(3-ethylbenzthiazoline-6-sulfonate). J. Chem. Soc. Perkin Trans. 2, 805–812. doi: 10.1039/p29820000805
Yan, Y., Yang, J., Dou, Y., Chen, M., and Al, S. P. E. (2008). Nitrogen fixation island and rhizosphere competence traits in the genome of root-associated Pseudomonas stutzeri a1501. Proc. Natl. Acad. Sci. U.S.A. 105, 7564–7569. doi: 10.1073/pnas.0801093105
Yanto, D. H. Y., Hidayat, A., and Tachibana, S. (2017). Periodical biostimulation with nutrient addition and bioaugmentation using mixed fungal cultures to maintain enzymatic oxidation during extended bioremediation of oily soil microcosms. Int. Biodeterioration Biodegradation 116, 112–123. doi: 10.1016/j.ibiod.2016.10.023
Zha, X., Liao, X., Zhao, X., Liu, F., He, A. Q., and Xiong, W. X. (2017). Turning waste drilling fluids into a new, sustainable soil resources for landscaping. Ecol. Eng. 121, 130–136. doi: 10.1016/j.ecoleng.2017.06.026
Zhang, H. Y., Yuan, X. Z., Xiong, T., Wang, H., and Jiang, L. B. (2020). Bioremediation of co-contaminated soil with heavy metals and pesticides: Influence factors, mechanisms and evaluation methods. Chem. Eng. J. 398:125657. doi: 10.1016/j.cej.2020.125657
Keywords: waste drill cutting, bioaugmentation plus phytoremediation, enzyme activity, microbial communities, partial least squares path modeling
Citation: Liu H, Chen L, Wang ET, Liu Y, Zhang L, Zhao K, Gu Y, Yu X, Ma M, Penttinen P, Zhang X, Huang M, Deng L and Chen Q (2020) Combined Microbial Consortium Inoculation and Black Locust Planting Is Effective in the Bioremediation of Waste Drill Cuttings. Front. Microbiol. 11:536787. doi: 10.3389/fmicb.2020.536787
Received: 21 February 2020; Accepted: 04 September 2020;
Published: 30 September 2020.
Edited by:
Pier-Luc Tremblay, Wuhan University of Technology, ChinaReviewed by:
Miguel Castañeda, Meritorious Autonomous University of Puebla, MexicoLloyd Douglas Potts, University of Aberdeen, United Kingdom
Copyright © 2020 Liu, Chen, Wang, Liu, Zhang, Zhao, Gu, Yu, Ma, Penttinen, Zhang, Huang, Deng and Chen. This is an open-access article distributed under the terms of the Creative Commons Attribution License (CC BY). The use, distribution or reproduction in other forums is permitted, provided the original author(s) and the copyright owner(s) are credited and that the original publication in this journal is cited, in accordance with accepted academic practice. No use, distribution or reproduction is permitted which does not comply with these terms.
*Correspondence: Liangji Deng, auh6@sicau.edu.cn; Qiang Chen, cqiang@sicau.edu.cn