- 1Department of Pharmacy, Faculty of Health Sciences, UiT The Arctic University of Norway, Tromsø, Norway
- 2Biosystems and Integrative Sciences Institute (BioISI), Faculdade de Ciências, Universidade de Lisboa, Lisbon, Portugal
- 3Departamento de Biologia Vegetal, Faculdade de Ciências, Universidade de Lisboa, Lisbon, Portugal
- 4Centre for Ecology, Evolution and Environmental Changes (cE3c), Faculdade de Ciências, Universidade de Lisboa, Lisbon, Portugal
Bacterial biofilms have an impact in medical and industrial environments because they often confer protection to bacteria against harmful agents, and constitute a source from which microorganisms can disperse. Conjugative plasmids can enhance bacterial ability to form biofilms because conjugative pili act as adhesion factors. However, plasmids may interact with each other, either facilitating or inhibiting plasmid transfer. Accordingly, we asked whether effects on plasmid transfer also impacts biofilm formation. We measured biofilm formation of Escherichia coli cells harboring two plasmid types, or when the two plasmids were present in the same population but carried in different cells. Using eleven natural isolated conjugative plasmids, we confirmed that some indeed promote biofilm formation and, importantly, that this ability is correlated with conjugative efficiency. Further we studied the effect of plasmid pairs on biofilm formation. We observed increased biofilm formation in approximately half of the combinations when both plasmids inhabited the same cell or when the plasmids were carried in different cells. Moreover, in approximately half of the combinations, independent of the co-inhabitation conditions, one of the plasmids alone determined the extent of biofilm formation – thus having a dominant effect over the other plasmid. The molecular mechanisms responsible for these interactions were not evaluated here and future research is required to elucidate them.
Introduction
Bacteria may live as unicellular planktonic organisms or as part of biofilms, which are complex bacterial communities embedded in a polymeric matrix. These complex structures have an important impact in medical and industrial environments because they often confer protection to bacteria against harmful agents, such as antimicrobials and host immune components, and constitute a source from which microorganisms can disperse (Costerton et al., 1999; Hall-Stoodley et al., 2004; Flemming et al., 2016). Clinically, biofilms are estimated to be involved in at least 65% of bacterial infections (Joo and Otto, 2012). Additionally, due to the close proximity between cells in biofilms, these structures can potentially favor horizontal gene transfer, mediated by mobile genetic elements such as plasmids, contributing to the spread of antibiotic resistance genes [reviewed in Molin and Tolker-Nielsen (2003), Sorensen et al. (2005), Stalder and Top (2016))]. Plasmids, concurrently, can also promote biofilm formation (Ghigo, 2001; Reisner et al., 2006).
Escherichia coli strains carrying plasmid F are good biofilm formers while plasmid-free isogenic strains are not (Ghigo, 2001). This effect is no longer observed when using a derivative of plasmid F not expressing sex pili, showing that these appendages can act as adhesion factors to increase biofilm formation (Ghigo, 2001). Plasmid-mediated biofilm promotion is more evident when plasmid-carrying strains invade a population of plasmid-free cells (Ghigo, 2001; Krol et al., 2013). The role of plasmids as biofilm developers was confirmed by screening 403 E. coli strains (Reisner et al., 2006) of which almost 50% developed better biofilms when co-cultured with plasmid-free cells than when cultured alone. This sub-collection of strains was shown to carry conjugative plasmids. Those plasmid-free cells that acquired plasmids during co-culture also became better biofilm producers.
While sex-pili promote cellular contact in early phases of biofilm formation (Ghigo, 2001), plasmids may enhance biofilm formation by other means (D’Alvise et al., 2010; Lim et al., 2010) allegedly acting in parallel or in subsequent phases. As an example, the conjugative machinery of plasmid F also stimulates E. coli to synthesize colanic acid and curli proteins, which play a role in biofilm maturation (May and Okabe, 2008). Other studies, focused on plasmid R1drd19, showed that the expression of several E. coli chromosomal genes changed due to the presence of this plasmid (Barrios et al., 2006; Yang et al., 2008). Such changes increase cell aggregation, promote quorum sensing AI-2 signaling and decrease motility, thus resulting in enhanced biofilm formation. These examples affecting the different biofilm phenotypes are due to molecular cross-talk between plasmid and chromosome, that was additionally shown to be specific of plasmid-host combinations. For instance, plasmid pKJK5 decreases Pseudomonas putida’s ability to form biofilms and increases that of Kluyvera sp. but does not affect E. coli (Roder et al., 2013).
Plasmids carry accessory genes that shape biofilm formation. As an illustration, plasmids belonging to the incompatibility subgroup IncX1, as plasmids pOLA52 and pMAS2027, commonly encode type 3 fimbriae (Burmolle et al., 2012), which mediate attachment to surfaces and promote biofilm formation (Burmolle et al., 2008; Ong et al., 2009). The effect of plasmid pOLA52 was to foster an increase in biofilm formation in Salmonella enterica serovar Typhimurium, Kluyvera sp. and Enterobacter aerogenes, whereas in Klebsiella pneumoniae, that also chromosomally encodes other type 3 fimbriae, the effect was lower (Burmolle et al., 2008). On the other hand, common antibiotic resistance genes frequently carried in plasmids can also affect biofilm development. Specifically, overexpression of efflux pumps leading to tetracycline resistance induces expression of surface structures that promote biofilm formation, while class A and D β-lactamases inhibit biofilm formation, possibly by preventing the correct assembly of such structures (Gallant et al., 2005; May et al., 2009). Intriguingly, conjugative pili may sometimes decrease the ability of conjugative plasmids to enhance biofilm formation (Ong et al., 2009), and even non-conjugative plasmids may enhance biofilm formation despite not expressing sex pili (Teodosio et al., 2012; Mathlouthi et al., 2018; Nakao et al., 2018).
Altogether, the role of plasmids in biofilm formation seems to be complex, not being dictated in a single direction nor by single factors, also obeying interactions with the host chromosome. Another layer to this already complex behavior is that multiple mobile genetic elements can be found in bacterial communities, even at the intracellular level, and interact among themselves. The existence of such interactions can shape individual effects, leading to a diversity of behaviors (Dionisio et al., 2019).
As far as it concerns the effect of specific and multiple plasmid interactions on biofilm formation, research is scarce. It has been shown that a conjugative and a non-conjugative plasmids interacted in a synergistic manner to promote biofilm formation (Dudley et al., 2006). Moreover, antagonistic interactions were reported when cells in a population carried different, but related, plasmids such that they expressed surface exclusion (a process by which plasmid-carrying cells become less capable to engage in conjugation with cells harboring a related plasmid) which prevented cell contacts and biofilm formation (Reisner et al., 2006).
In order to expand the limited research on this subject, we aim to evaluate how conjugative plasmids interactions affect biofilm formation. We show that the ability of conjugative plasmids to promote biofilm formation is correlated with their conjugative efficiency. Furthermore, in half of the strains with multiple plasmids analyzed, one of the plasmids exhibited a dominant effect on biofilm development.
Materials and Methods
Bacterial Strains, Plasmids and Media
We used the following bacterial strains: E. coli K12 MG1655 Δara, as plasmid-free, containing each of the 11 natural conjugative plasmids (summarized in Table 1), or containing the 33 possible combinations (due to incompatibility or selective markers) of two plasmids. For single-plasmid conjugation experiments, we used E. coli K12 MG1655 as the recipient strain. All these strains were produced in prior work (Gama et al., 2017). We conducted all experiments in Lysogeny Broth (LB) without antibiotics, unless otherwise stated.
Growth Rate Measurements
We cultivated the plasmid-free strain, 11 single-plasmid carrying strains and six strains carrying two plasmids (combinations of F, R16a, R388 and R6K) in LB overnight at 37°C with agitation. We diluted these cultures 100-fold in LB, and added 250 μL (∼2 × 107 CFU/mL) to a 96-well plate. We conducted the experiments with three biological replicates, each consisting of three technical replicates. We incubated the plates overnight at 37°C in an EPOCH 2 microplate reader (BioTek Instruments, Inc) with continuous shaking, taking OD600 measurements every 10 min. We used GrowthRates v3.0 (Hall et al., 2014) to estimate growth rates. We calculated growth rates for each biological replicate as the average of the technical replicates (after discarding those with a R2 correlation coefficient <0.95). Finally, we estimated the growth rate of each strain relative to the plasmid-free strain. Thus, we calculated the ratio between the growth rate of each biological replicate of each strain by the mean growth rate of the plasmid-free strain.
To estimate the population size of cultures for each of the strains mentioned above, we plated serial 10-fold dilutions of the respective overnight cultures in LB agar plates and incubated them overnight at 37°C, to estimate the CFU/mL. We performed this experiment with three biological replicates.
Biofilm Formation Assays
We measured biofilm formation in four conditions: (i) the plasmid-free strain cultivated alone (reference values); (ii) single-plasmid carrying strains co-cultivated with the plasmid-free strain; (iii) double-plasmid carrying strains co-cultivated with the plasmid-free strain; (iv) two different single-plasmid carrying strains co-cultivated together.
To assay biofilm formation, we followed the protocol by Christensen et al. (1985). Briefly, we cultivated the strains in 96-well plates (U-bottom, polystyrene, Greiner bio-one) containing 200 μL of LB per well. After overnight incubation at 37°C with agitation, we mixed 5 μL of the donor and 5 μL of the recipient strain in 190 μL of LB in a new 96-well plate. We then transferred 5 μL of each mix (∼2.5 × 105 CFU per strain) into 195 μL of LB in a new plate, which we incubated for 24 h at 37°C without agitation, inside plastic bags to prevent evaporation.
Biofilm Formation Quantification
We washed the plates three times to remove unattached cells and then stained them with crystal violet (0.1%) for 15 min [adapted from Christensen et al. (1985)]. We dissolved the crystal violet with ethanol (96%) and measured absorbance at 595 nm (Microplate Reader Tecan Spec 11.A Rainbow). We collected the values for the monoculture of the plasmid-free strain, for each plate and averaged them after removing outliers (see section “Statistics”). We normalized all well-measurements of the same plate by dividing each value by the average of the monoculture of the plasmid-free strain.
We performed at least six biological replicates for each experiment. As the number of replicates varies for different strains/conditions, we indicate the number of biological replicates used (after removal of outliers) in Figures 1, 3 and 4.
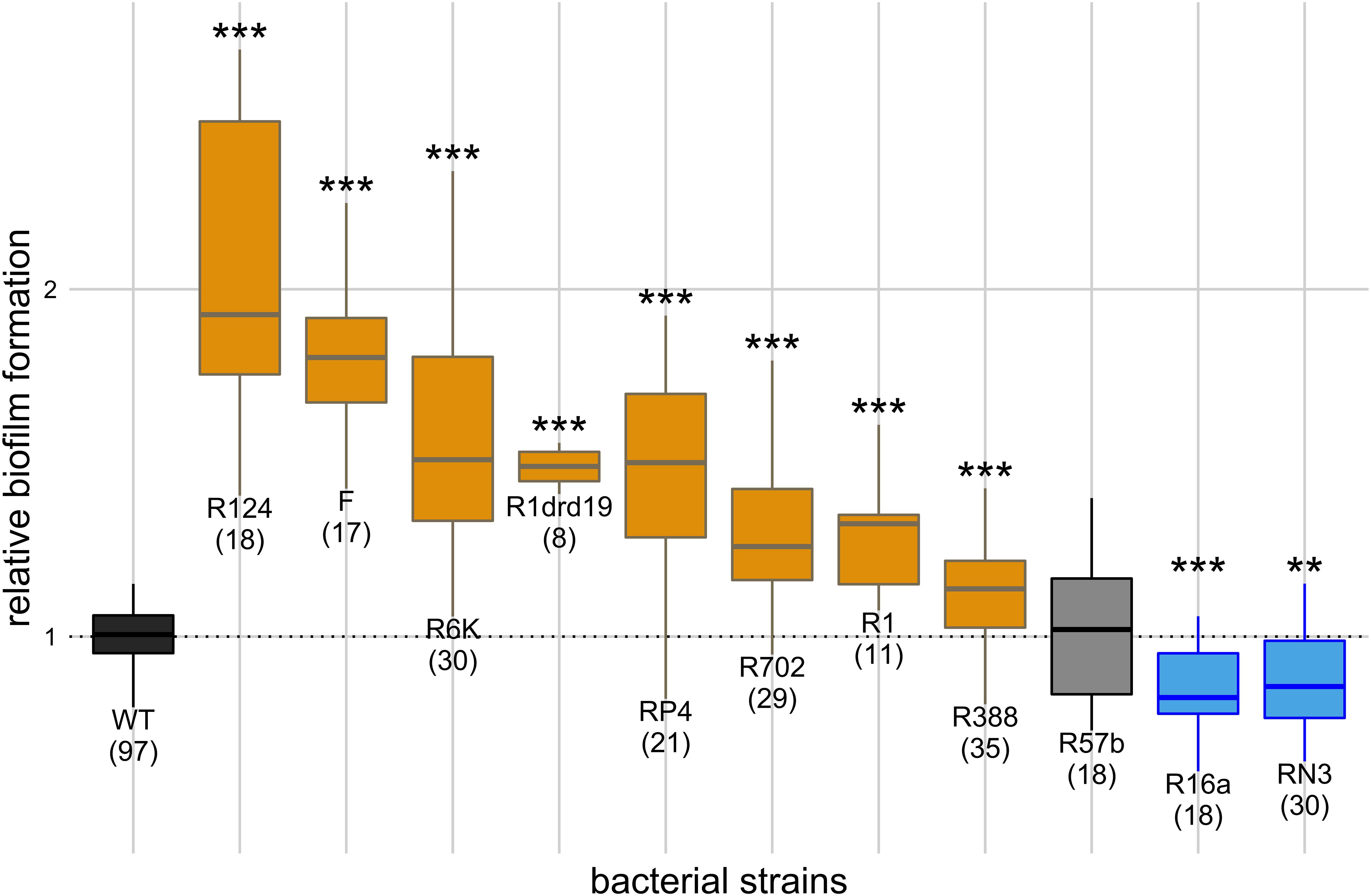
Figure 1. Effect of single plasmids on biofilm formation. Y axis represents biofilm formation relative to the plasmid-free strain (WT). Annotations under the boxes indicate the identity of the plasmids and, in parenthesis, the number of biological replicates. Results of Dunnett’s multiple comparison test against the plasmid-free strain are indicated above the boxes as: **p-value < 0.01; ***p-value < 0.001. Box color indicates the phenotype qualitatively, such that biofilm formation: increased significantly (orange), decreased significantly (blue), or did not change significantly (light gray). Dark gray indicates the plasmid-free reference strain (WT).
Conjugation Efficiency
To measure the conjugative efficiency of the 11 individual plasmids, we set up 96-well plates as described above for biofilm assays, with two differences: (1) we co-cultivated each E. coli K12 MG1655 Δara carrying a single plasmid with plasmid-free E. coli K12 MG1655 (ara+), and (2) we incubated the plates for 2 h. After incubation, we diluted a 100 μL culture sample in 0.9% NaCl and plated serial dilutions on tetrazolium arabinose (TA) agar to quantify donor and recipient CFU/mL (red and white colonies, respectively) and on M9 minimal media supplemented with one antibiotic (Table 1) selective for the specific plasmid to quantify transconjugants. We incubated TA plates overnight and M9 plates for 48 h, at 37°C. We estimated conjugation efficiency as, where T, D, and R are the CFU/mL of transconjugants, donors and recipients, respectively. We performed three biological replicates for each experiment.
When co-cultivating two different single-plasmid carrying strains together, we repeated the biofilm assay to quantify the proportion of cells that carried two plasmids at the end of the experiment. After the 24 h incubation, we diluted a 100 μL culture sample in 0.9% NaCl and plated appropriate dilutions on LB agar to quantify total cells and on LB agar supplemented with all antibiotics selective for the two plasmids (Table 1). We incubated the plates overnight at 37°C. We performed this experiment for the six combinations of strains carrying plasmids F, R16a, R388, and R6K, with three biological replicates.
Homologues of Type 3 Fimbriae
We used the BLASTX algorithm to search in the nucleotide sequence of plasmid R6K1 for homology with the type 3 fimbriae of K. pneumoniae (accession no. M55912), considering an e-value < 10–5.
Statistics
We performed statistical tests in R version 3.5.1, available at http://www.rstudio.com/ (R Core Team, 2018).
We verified normality of the biofilm formation values obtained for each sample (Shapiro-Wilk test) after removing outliers (no values outside [Q1 − 1.5 × IQR, Q3 + 1.5 × IQR], where Q1 and Q3 are, respectively, the first and third quartiles and IQR is the interquartile range (Q3–Q1).
We estimated linear regression and correlations between biofilm formation and conjugation efficiency, growth rate and plasmid size, after excluding outliers according to the studentized residuals method (Venables and Ripley, 2002).
We conducted one-way ANOVAs controlled for heteroscedasticity, and when significant we further performed Tukey’s or Dunnett’s multiple comparison tests. Non-paired Welch t-tests were used to compare two samples.
Results
The Effect of Single Plasmids on Biofilm Formation
We measured how co-cultivation of the plasmid-free strain with each of the 11 single-plasmid carrying strains affected biofilm formation relatively to being cultivated alone. The effect of the 11 natural conjugative plasmids, belonging to six different incompatibility groups, on biofilm formation is shown in Figure 1. Raw OD595 reads for negative controls (uninoculated LB) varied from 0.05 to 0.07, while reads for inoculated experimental assays varied from 0.10 to 0.51 with raw reads for the plasmid-free strain varying from 0.10 to 0.35. Compared with the plasmid-free strain (one-way ANOVA, d.f. = 11, p-value = 1.49 × 10–39, followed by Dunnett’s multiple comparison test), eight plasmids increased the strain’s ability to form biofilms, two decreased it and one did not have a significant effect. Cells carrying plasmid R124 exhibited the highest biofilm formation, while those carrying plasmid R16a or RN3 exhibited less biofilm formation than the plasmid-free strain.
Since plasmids can enhance biofilm formation via conjugative pili, we checked for a correlation between the ability to form biofilms and conjugation efficiency. This correlation was significant (Pearson method, two-sided, d.f. = 8, p-value = 0.009, ρ = 0.77) after outlier (plasmid R6K) exclusion (Figure 2). The correlation was no longer significant if R6K was included (Pearson method, two-sided, d.f. = 9, p-value = 0.16, ρ = 0.45). The result shows that, in general, a high conjugation rate correlates with an increased ability to form biofilms.
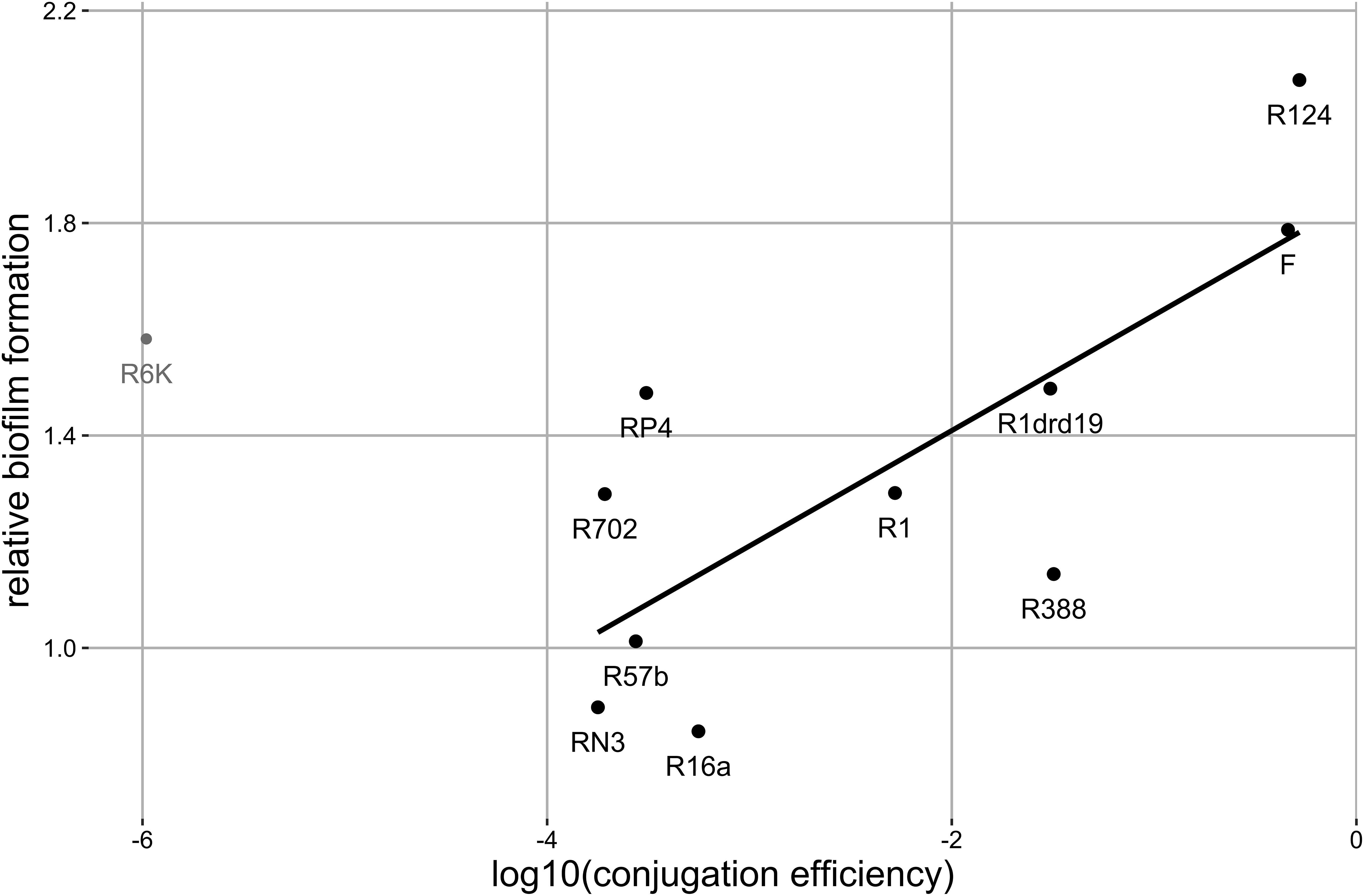
Figure 2. Correlation between biofilm formation and conjugation efficiency. Each data point represents the mean value for each plasmid (number of replicates as in Figure 1). The black line represents the regression line (y = 0.22x + 1.84, R2 = 0.59, σ2 = 0.07) for black points, where y is the biofilm production relative to the plasmid-free strain and x is the log10 of the conjugation rate. Gray point represents the excluded outlier. Significant correlation: Pearson method, two-sided, d.f. = 8, ρ = 0.77, p-value = 0.009.
Plasmid R6K stood as an outlier in the above correlation, increasing biofilm formation despite its low conjugative efficiency. Plasmids belonging to the incompatibility subgroup IncX1 commonly encode type 3 fimbriae, presumably acquired from K. pneumoniae, which enhances biofilm formation (Burmolle et al., 2012). Plasmid R6K is related to these plasmids since it belongs to the incompatibility subgroup IncX2. Therefore, we searched for genes coding for type 3 fimbriae in the sequence of R6K. Regardless, we did not identify any homologues.
We also investigated whether growth rate changes due to plasmid carriage affected biofilm formation. Compared to the plasmid-free strain (one-way ANOVA, d.f. = 11, p-value = 0.00015, followed by Dunnett’s multiple comparison test), four of the eleven plasmids affected host growth rates significantly (Supplementary Table S1). Strains carrying plasmids R57b, R124, R388, and R6K displayed significantly reduced growth rates, with effects ranging from 12.44 to 26.44%. Despite the difference in growth rates, all strains attained overnight population sizes (i.e., carrying capacity) of ∼2 × 109 cells/mL. The correlation between biofilm formation and relative growth rates was not significant neither with inclusion (Pearson method, two sided, d.f. = 9, p-value = 0.74, ρ = −0.11) nor exclusion of the two outliers F and R124 (Pearson method, two sided, d.f. = 7, p-value = 0.41, ρ = −0.32). It has been hypothesized that increasing plasmid sizes could be associated with higher fitness costs (Zünd and Lebek, 1980; Cheah et al., 1987; Vogwill and MacLean, 2015), however plasmid sizes could as well increase the probability of carriage of genes promoting biofilm formation. We observed no significant correlation (Pearson method, two sided, d.f. = 8, p-value = 0.053, ρ = 0.62) between relative growth rates and plasmid size (Supplementary Table S1). Likewise, the correlation between biofilm formation and plasmid size was not significant (Pearson method, two sided, d.f. = 8, p-value = 0.89, ρ = 0.05), even after excluding outliers (Pearson method, two sided, d.f. = 5, p-value = 0.33, ρ = 0.43).
Intra and Intercellular Plasmid Interactions
We measured biofilm formation of 33 strains carrying different combinations of two plasmids (from the 11 plasmids) to understand the effect of plasmids’ intracellular interactions on this phenotype. In this setup, the plasmid-free strain was co-cultivated with each of the 33 double-plasmid carrying strains. When compared with the plasmid-free strain alone (one-way ANOVA, d.f. = 33, p-value = 1.55 × 10–31, Dunnett’s multiple comparison test), 17 of the strains carrying two plasmids displayed increased biofilm formation and two strains displayed decreased biofilm formation (Figure 3). R16a, one of the two plasmids that, alone, decreases biofilm formation, was present in the two combinations exhibiting decreased biofilm formation.
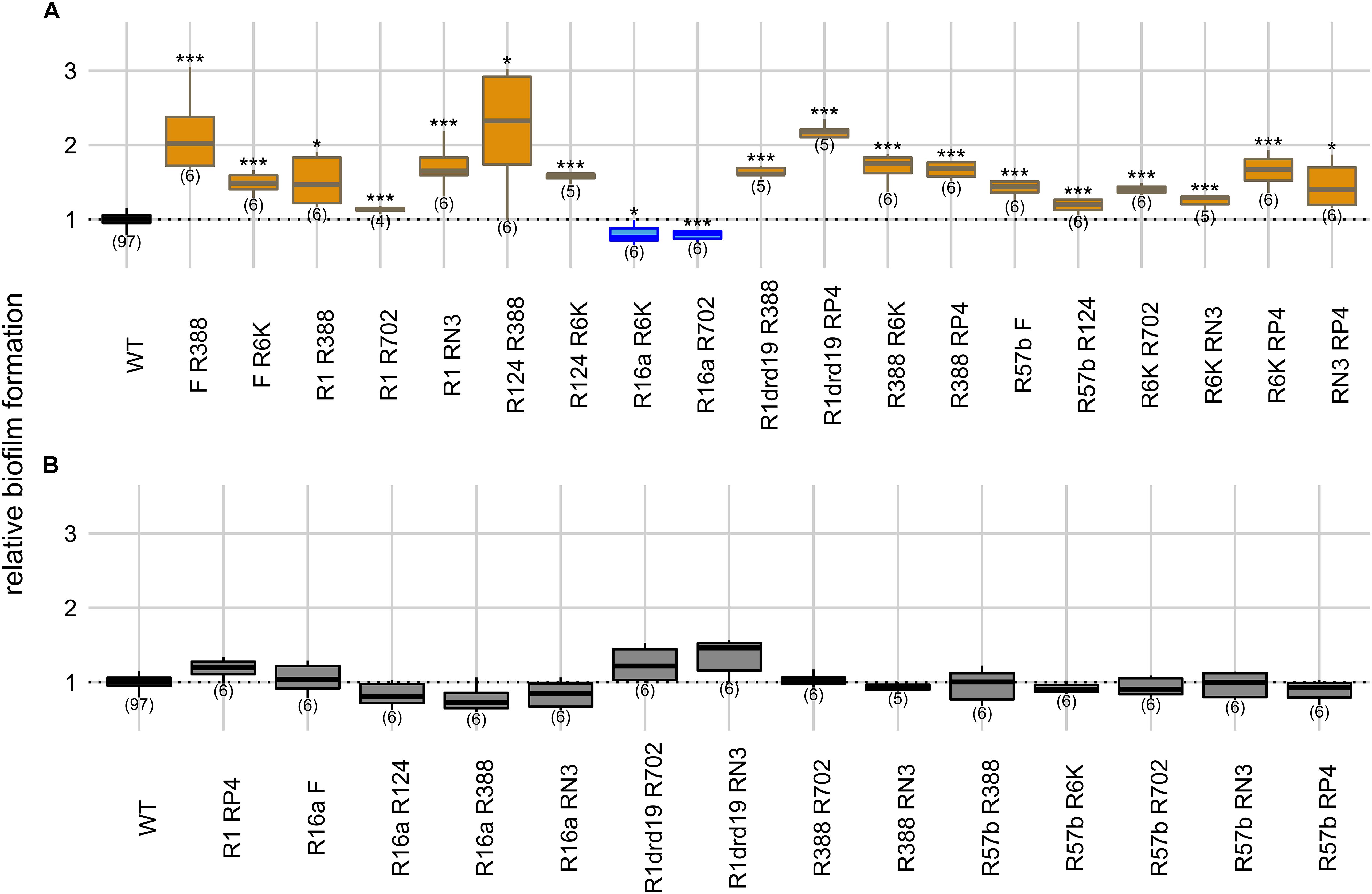
Figure 3. Intracellular effects of two plasmids on biofilm formation. Panels indicate significant (A) and non-significant effects (B) on biofilm formation relatively to the plasmid-free strain. Y axis represents biofilm formation relative to the plasmid-free strain (WT). Plasmid combinations are indicated in the x axis and the number of biological replicates below the boxes (in parenthesis). Results of Dunnett’s multiple comparison test against the plasmid-free strain are indicated as: *p-value < 0.05; **p-value < 0.01; ***p-value < 0.001. Box color, as in Figure 1, indicates the phenotype qualitatively, such that biofilm formation: increased significantly (orange), decreased significantly (blue), or did not change significantly (light gray in panel B). Dark gray indicates the plasmid-free reference strain (WT).
We measured growth rates of the strains carrying six combinations of two plasmids (F, R16a, R388, and R6K) relatively to the plasmid-free strain (Supplementary Table S1). Four of these strains displayed significantly different growth rates (one-way ANOVA, d.f. = 6, p-value = 0.007, Dunnett’s multiple comparison test), but all six attained population sizes of ∼2 × 109 cells/mL as described for plasmid-free and single-plasmid carrying strains. The combinations F/R388, F/R6K, R16a/R6K, and R16a/R388 reduced host’s growth rate significantly but affected biofilm formation differently. Among the two combinations not significantly affecting growth rates, R388/R6K increased biofilm formation, while F/16a did not affect it significantly. Therefore, the relative growth rate of the strains does not seem to be a good predictor of the effect on biofilm formation, supporting the previous results of no significant correlation between the two variables.
Plasmids can be present simultaneously in the same bacterial population but carried in different host cells. Therefore, we also measured the ability conferred by two plasmids to form biofilms when carried in two different strains. In this setup two single-plasmid carrying strains were co-cultivated. When compared with the plasmid-free strain alone (one-way ANOVA, d.f. = 33, p-value = 1.76 × 10–45, Dunnett’s multiple comparison test), the ability to form biofilms increased in 12 cases, and decreased in four (Figure 4). R16a and RN3, the two plasmids that, alone, decrease biofilm formation, are involved in three of the four cases.
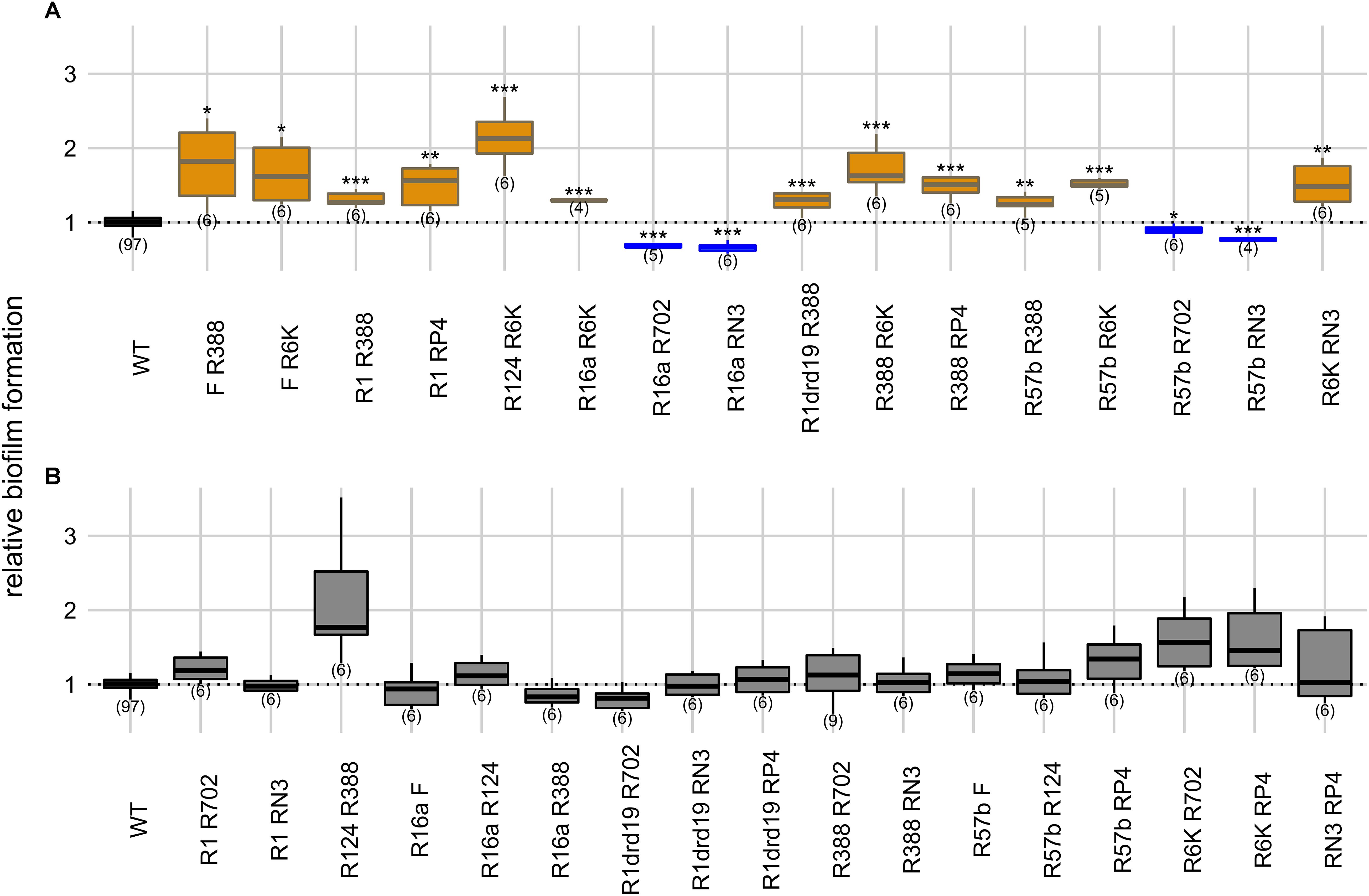
Figure 4. Intercellular effects of two plasmids on biofilm formation. Panels indicate significant (A) and non-significant effects (B) on biofilm formation relatively to the plasmid-free strain. Y axis represents biofilm formation relative to the plasmid-free strain (WT). Plasmid combinations are indicated in the x axis and the number of biological replicates below the boxes (in parenthesis). Results of Dunnett’s multiple comparison test against the plasmid-free strain are indicated as: *p-value < 0.05; **p-value < 0.01; ***p-value < 0.001. Box color, as in Figures 1 and 3, indicates the phenotype qualitatively, such that biofilm formation: increased significantly (orange), decreased significantly (blue), or did not change significantly (light gray in panel B). Dark gray indicates the plasmid-free reference strain.
In this “intercellular” experimental condition, cells harboring one of the plasmids serve both as donors and as recipients of plasmids carried by the other cells. This should result in more cellular interactions since both cells can express sex pili and can promote more stable mating pairs during the conjugative process (Dionisio et al., 2019). However, we observed no statistical difference (Fisher’s exact test, two-sided, p-value = 0.39) between intra- and intercellular conditions regarding the number of cases of increase, decrease or no effect on biofilm formation. One potentially confounding factor is that a high proportion of the populations may end up carrying both plasmids in the “intercellular” experiment. Therefore, we measured the proportion of cells carrying both plasmids for the six combinations of the four plasmids F, R16a, R388, and R6K. After 24 h of incubation, the populations where plasmid F (one of the plasmids with the highest conjugative efficiency) was present, 30–59% of cells carried both plasmids, while the populations with the other three plasmid combinations displayed 9–17% of cells carrying both plasmids (Supplementary Table S2). In comparison, the “intracellular” experiment started with 50% of the population already harboring both plasmids. Therefore, the impact of cells with both plasmids on biofilm formation appears to be low.
We then assessed the effect of the two conditions combination-wise, by comparing (Welch’s t-test) intracellular versus intercellular effects for each combination of plasmids (Supplementary Table S3). In 17 of the 33 combinations, biofilm formation did not differ significantly between conditions (Welch’s t-test, two-sided, p-values > 0.05). In nine combinations, biofilm formation was greater in intracellular conditions, while in the remaining seven combinations biofilm formation was greater in intercellular conditions. Overall, these results suggest that biofilm formation is not consistently favored by one of the two types of cellular interactions and depends on specific plasmid combinations.
One of the Plasmids Frequently Exerts a Dominant Effect on Biofilm Formation
In the previous section we showed that the effect of multiple plasmids on biofilm formation tends to be combination-specific, and therefore depends on how the plasmids interact. We checked whether one of the plasmids has a determinant effect (over the other) on biofilm production. For that, we simultaneously compared biofilm formation of the population carrying two plasmids (either one strain carrying two plasmids – intracellular condition –, or two strains each carrying one plasmid – intercellular condition) and each of the two strains carrying a single plasmid (one-way ANOVA followed by Tukey’s multiple comparison test). The amount of biofilm (B) produced is represented as BAB for the population carrying two plasmids and as BA and BB, respectively, for each of the strains carrying a single plasmid, A or B. The analysis can produce several outcomes:
(I) Dominance – when: BAB ≈ BA AND BAB ≠ BB
(II) Increased – when: BAB > BA AND BAB > BB
(III) Decreased – when: BAB < BA AND BAB < BB
(IV) Intermediate – when: BB < BAB < BA, OR when: BAB ≈ BA AND BAB ≈ BB AND BA ≠ BB
(V) Undetermined – when: BAB ≈ BA AND BAB ≈ BB AND BA ≈ BB
Where ≈ indicates non-significant differences and, ≠ indicates significant differences. It should be noted that there are two possible cases of Dominance: the biofilm produced by the strain carrying two plasmids does not differ from that produced by the single plasmid-carrying strain that produced more biofilm (Dominance by the Highest) or less biofilm (Dominance by the Lowest).
The results are summarized in Supplementary Table S4. Dominance prevailed in both intracellular and intercellular conditions, representing more than half (36/66 = 54.5%) of the outcomes: 16 cases by the Highest and 20 cases by the Lowest. Three cases exhibited Increase (all in intracellular conditions) and seven cases exhibited Decrease. There are 13 Intermediate cases and a minority of cases (7/66) were categorized as Undetermined. In these undetermined cases, we observed no significant difference (Fisher’s exact test, two-sided, p-value = 0.34), when comparing intracellular and intercellular conditions in terms of the six possible outcomes.
Discussion
Conjugative plasmids have been shown to enhance bacterial biofilm formation due to the expression of conjugative pili (Ghigo, 2001; Reisner et al., 2006). Here we confirmed that most of the tested conjugative plasmids enhanced biofilm formation (Figure 1) and further showed that this ability is correlated with conjugative efficiency (Figure 2) which supports previous studies (Ghigo, 2001; Reisner et al., 2006). Nevertheless, we observed that plasmid R6K is an exception to this rule since it displays low conjugative efficiency but increased biofilm formation. This indicates and is in line with other findings (Gallant et al., 2005; Barrios et al., 2006; Burmolle et al., 2008; May and Okabe, 2008; Yang et al., 2008; May et al., 2009; Ong et al., 2009; D’Alvise et al., 2010; Lim et al., 2010; Nakao et al., 2018) showing that besides sex pili, other factors like adhesion factors or altered gene expression due to plasmid-chromosome cross-talk affect biofilm formation. Examples of these include IncX1 plasmids that encode type 3 fimbriae promoting biofilm formation (Burmolle et al., 2012). However, although the IncX2 plasmid R6K is related to IncX1 plasmids, we did not find sequences encoding such an appendage.
Not all plasmids led to increased biofilm formation (Figure 1), and two of them (RN3 and R16a) even decreased it. In fact, lower biofilm formation due to plasmids was reported before (Roder et al., 2013) and several factors, like the action of β-lactamases (Gallant et al., 2005; May et al., 2009), can influence it. Since R16a, one of the five plasmids encoding β-lactamases, was the only one leading to lower biofilm formation, we infer that coding for a β-lactamase is not a sufficient feature to diminish the development of biofilms. Plasmid RN3, on the other hand, does not encode such enzymes. Altogether, other factors could be accountable for this phenomenon, such as quorum sensing inhibition, alteration of cell-surface, or downregulation of chemotaxis [reviewed in Rendueles and Ghigo (2012)].
Plasmid carriage is known to affect host growth rate (Carroll and Wong, 2018; Gama et al., 2018) and could have consequences on biofilm formation. The method used in this work can only detect substantial growth effects. To precisely measure growth effects a more robust method would be preferred (Morton et al., 2014). Yet, although we detected significant growth rate reduction associated with four plasmids, it was not significantly correlated with their effect on biofilm formation.
Cross-talk between plasmids and chromosomes affects biofilm formation (Barrios et al., 2006; May and Okabe, 2008; Yang et al., 2008; May et al., 2009; Lim et al., 2010; Roder et al., 2013; Nakao et al., 2018), and interactions between plasmids can also impact biofilm development (Dudley et al., 2006). We previously tested intracellular and intercellular interactions in 33 pairs of conjugative plasmids and showed that conjugative efficiency tends to decrease when both plasmids were present in the same cell, and to increase if they were carried in different cells (Gama et al., 2017). Here we tried to relate how plasmid interactions may affect biofilm formation. In essence, we showed that, although biofilm formation differs between these two co-inhabitation conditions (intra and intercellular), it does not seem to be consistently favored by one or the other (Figures 3, 4 and Supplementary Table S3). Rather, biofilm formation is determined by particular combinations of plasmids.
Dominance was the main outcome in both intra- and intercellular conditions (Supplementary Table S4), meaning that one of the two plasmids determines the extent of biofilm formation. The features responsible for the effects observed were not evaluated here. We consider that they could be multiple. For instance, plasmid R16a had a dominant effect in 5/6 of intracellular interactions (the other one was undetermined), and could thus encode a single factor that consistently decreases biofilm formation, exerting a dominant effect when another plasmid inhabits the same cell. On the other hand, plasmid R388 when alone had a weak effect on biofilm formation and in 9/10 of intracellular interactions was recessive, but in combination with R702 decreased biofilm formation. These two plasmids inhibit each others’ conjugative transfer (Olsen and Shipley, 1975; Fong and Stanisich, 1989; Getino et al., 2017), which could explain reduced biofilm development. However, interactions between other plasmid genes or even more complex plasmid-chromosome-plasmid cross-talk cannot be ruled out. Future research is required to dissect the mechanisms responsible for the plasmid interactions affecting biofilm formation.
A weakness of this work is the usage of lab strains, known to be weak biofilm formers and we report small albeit significant effects. To further expand on the relevance of our findings beyond lab strains, future studies comprising clinical strains carrying multiple plasmids would be of relevance to address the importance of plasmid interactions on biofilms of representative E. coli isolates circulating in the community.
Our work focused on plasmid interactions during the early phases of biofilm formation on polystyrene plates. Nevertheless, studies with different conditions and environments are important to assess the robustness of our results. It is relevant to explore how bacterial growth phases and different biofilm stages affect plasmid interactions. For some plasmids longer incubation times are required to promote biofilm formation (Ghigo, 2001), and, while sex pili are important for initial adhesion, other plasmid factors affect later biofilm maturation (May and Okabe, 2008). Initial adhesion is also determined by the type of surface (e.g., polystyrene, glass or cultured cells) (Dudley et al., 2006), and the rate of plasmid transfer also varies depending on the physical region of the biofilm (Krol et al., 2011). Therefore, plasmid interactions may depend on the habitat and culture conditions and contribute to distinct adherence patterns. In conclusion, not only the mechanisms behind plasmid interactions, but also the interplay between these interactions and the environment should be the target of future research.
Data Availability Statement
All datasets generated for this study are included in the article/Supplementary Material.
Author Contributions
JG, RZ, and FD conceived the study. JG, AR, and EF designed the experiments. JG, EF, and FC performed the experiments. JG and FD analyzed the data. JG wrote the first draft of the manuscript, with contributions from AR, RZ, and FD. All authors contributed to manuscript revision, read and approved the submitted version.
Funding
JG received a Ph.D. grant from Fundação para a Ciência e Tecnologia (SFRH/BD/86103/2012). Fundação para a Ciência e Tecnologia also supported cE3c through UID/BIA/00329/2013. UiT The Arctic University of Norway through UiT publication fund.
Conflict of Interest
The authors declare that the research was conducted in the absence of any commercial or financial relationships that could be construed as a potential conflict of interest.
Acknowledgments
We thank Dr. Cristina Cruz for the Microplate Reader (Tecan Spec 11.A Rainbow) required to determine biofilm formation.
Supplementary Material
The Supplementary Material for this article can be found online at: https://www.frontiersin.org/articles/10.3389/fmicb.2020.02070/full#supplementary-material
Footnotes
References
Barrios, A. F. G., Zuo, R. J., Ren, D. C., and Wood, T. K. (2006). Hha, YbaJ, and OmpA regulate Escherichia coli K12 biofilm formation and conjugation plasmids abolish motility. Biotechnol. Bioeng. 93, 188–200. doi: 10.1002/bit.20681
Burmolle, M., Bahl, M. L., Jensen, L. B., Sorensen, S. J., and Hansen, L. H. (2008). Type 3 fimbriae, encoded by the conjugative plasmid pOLA52, enhance biofilm formation and transfer frequencies in Enterobacteriaceae strains. Microbiol. Sgm. 154, 187–195. doi: 10.1099/mic.0.2007/010454-0
Burmolle, M., Norman, A., Sorensen, S. J., and Hansen, L. H. (2012). Sequencing of IncX-plasmids suggests ubiquity of mobile forms of a biofilm-promoting gene cassette recruited from Klebsiella pneumoniae. PLoS One 7:e41259. doi: 10.1371/journal.pone.0041259
Carroll, A. C., and Wong, A. (2018). Plasmid persistence: costs, benefits, and the plasmid paradox. Can. J. Microbiol. 64, 293–304. doi: 10.1139/cjm-2017-0609
Cheah, U. E., Weigand, W. A., and Stark, B. C. (1987). Effects of recombinant plasmid size on cellular processes in Escherichia coli. Plasmid 18, 127–134. doi: 10.1016/0147-619x(87)90040-0
Christensen, G. D., Simpson, W. A., Younger, J. J., Baddour, L. M., Barrett, F. F., Melton, D. M., et al. (1985). Adherence of coagulase-negative staphylococci to plastic tissue-culture plates - a quantitative model for the adherence of staphylococci to medical devices. J. Clin. Microbiol. 22, 996–1006. doi: 10.1128/jcm.22.6.996-1006.1985
Costerton, J. W., Stewart, P. S., and Greenberg, E. P. (1999). Bacterial biofilms: a common cause of persistent infections. Science 284, 1318–1322. doi: 10.1126/science.284.5418.1318
D’Alvise, P. W., Sjoholm, O. R., Yankelevich, T., Jin, Y. J., Wuertz, S., and Smets, B. F. (2010). TOL plasmid carriage enhances biofilm formation and increases extracellular DNA content in Pseudomonas putida KT2440. FEMS Microbiol. Lett. 312, 84–92. doi: 10.1111/j.1574-6968.2010.02105.x
Dionisio, F., Zilhão, R., and Gama, J. A. (2019). Interactions between plasmids and other mobile genetic elements affect their transmission and persistence. Plasmid 102, 29–36. doi: 10.1016/j.plasmid.2019.01.003
Dudley, E. G., Abe, C., Ghigo, J. M., Latour-Lambert, P., Hormazabal, J. C., and Nataro, J. P. (2006). An IncI1 plasmid contributes to the adherence of the atypical enteroaggregative Escherichia coli strain C1096 to cultured cells and abiotic surfaces. Infect. Immun. 74, 2102–2114. doi: 10.1128/Iai.74.4.2102-2114.2006
Fernandez-Lopez, R., de Toro, M., Moncalian, G., Garcillan-Barcia, M. P., and De la Cruz, F. (2016). Comparative genomics of the conjugation region of f-like plasmids: five shades of F. Front. Mol. Biosci. 3:71. doi: 10.3389/fmolb.2016.00071
Flemming, H. C., Wingender, J., Szewzyk, U., Steinberg, P., Rice, S. A., and Kjelleberg, S. (2016). Biofilms: an emergent form of bacterial life. Nat. Rev. Microbiol. 14, 563–575. doi: 10.1038/nrmicro.2016.94
Fong, S. T., and Stanisich, V. A. (1989). Location and characterization of 2 functions on Rp1 that inhibit the fertility of the Incw plasmid-R388. J. Gen. Microbiol. 135, 499–502. doi: 10.1099/00221287-135-3-499
Gallant, C. V., Daniels, C., Leung, J. M., Ghosh, A. S., Young, K. D., Kotra, L. P., et al. (2005). Common beta-lactamases inhibit bacterial biofilm formation. Mol. Microbiol. 58, 1012–1024. doi: 10.1111/j.1365-2958.2005.04892.x
Gama, J. A., Zilhão, R., and Dionisio, F. (2017). Conjugation efficiency depends on intra and intercellular interactions between distinct plasmids: plasmids promote the immigration of other plasmids but repress co-colonizing plasmids. Plasmid 93, 6–16. doi: 10.1016/j.plasmid.2017.08.003
Gama, J. A., Zilhao, R., and Dionisio, F. (2018). Impact of plasmid interactions with the chromosome and other plasmids on the spread of antibiotic resistance. Plasmid 99, 82–89.
Getino, M., Palencia-Gandara, C., Garcillan-Barcia, M. P., and de la Cruz, F. (2017). PifC and Osa, plasmid weapons against rival conjugative coupling proteins. Front. Microbiol. 8:2260. doi: 10.3389/fmicb.2017.02260
Ghigo, J. M. (2001). Natural conjugative plasmids induce bacterial biofilm development. Nature 412, 442–445. doi: 10.1038/35086581
Hall, B. G., Acar, H., Nandipati, A., and Barlow, M. (2014). Growth rates made easy. Mol. Biol. Evol. 31, 232–238. doi: 10.1093/molbev/mst187
Hall-Stoodley, L., Costerton, J. W., and Stoodley, P. (2004). Bacterial biofilms: from the natural environment to infectious diseases. Nat. Rev. Microbiol. 2, 95–108. doi: 10.1038/nrmicro821
Joo, H. S., and Otto, M. (2012). Molecular basis of in vivo biofilm formation by bacterial pathogens. Chem. Biol. 19, 1503–1513. doi: 10.1016/j.chembiol.2012.10.022
Koraimann, G., Koraimann, C., Koronakis, V., Schlager, S., and Hogenauer, G. (1991). Repression and derepression of conjugation of plasmid R1 by wild-type and mutated finp antisense rna. Mol. Microbiol. 5, 77–87. doi: 10.1111/j.1365-2958.1991.tb01828.x
Krol, J. E., Nguyen, H. D., Rogers, L. M., Beyenal, H., Krone, S. M., and Top, E. M. (2011). Increased transfer of a multidrug resistance plasmid in Escherichia coli Biofilms at the air-liquid interface. Appl. Environ. Microbiol. 77, 5079–5088. doi: 10.1128/AEM.00090-11
Krol, J. E., Wojtowicz, A. J., Rogers, L. M., Heuer, H., Smalla, K., Krone, S. M., et al. (2013). Invasion of E-coli biofilms by antibiotic resistance plasmids. Plasmid 70, 110–119. doi: 10.1016/j.plasmid.2013.03.003
Lim, J. Y., La, H. J., Sheng, H. Q., Forney, L. J., and Hovde, C. J. (2010). Influence of Plasmid pO157 on Escherichia coli O157:H7 sakai biofilm formation. Appl. Environ. Microb. 76, 963–966. doi: 10.1128/Aem.01068-09
Mathlouthi, A., Pennacchietti, E., and De Biase, D. (2018). Effect of temperature, pH and plasmids on in vitro biofilm formation in Escherichia coli. Acta Naturae 10, 129–132. doi: 10.32607/20758251-2018-10-4-129-132
Matthew, M., and Hedges, R. W. (1976). Analytical isoelectric focusing of R-Factor-determined beta-lactamases - correlation with plasmid compatibility. J. Bacteriol. 125, 713–718. doi: 10.1128/jb.125.2.713-718.1976
May, T., Ito, A., and Okabe, S. (2009). Induction of multidrug resistance mechanism in Escherichia coli biofilms by interplay between tetracycline and ampicillin resistance genes. Antimicrob. Agents Ch. 53, 4628–4639. doi: 10.1128/Aac.00454-09
May, T., and Okabe, S. (2008). Escherichia coli harboring a natural incf conjugative f plasmid develops complex mature biofilms by stimulating synthesis of colanic acid and curli. J. Bacteriol. 190, 7479–7490. doi: 10.1128/Jb.00823-08
Molin, S., and Tolker-Nielsen, T. (2003). Gene transfer occurs with enhanced efficiency in biofilms and induces enhanced stabilisation of the biofilm structure. Curr. Opin. Biotech. 14, 255–261. doi: 10.1016/S0958-1669(03)00036-3
Morton, E. R., Platt, T. G., Fuqua, C., and Bever, J. D. (2014). Non-additive costs and interactions alter the competitive dynamics of co-occurring ecologically distinct plasmids. Proc. R. Soc. BBiol. Sci. 281:20132173. doi: 10.1098/rspb.2013.2173
Nakao, R., Myint, S. L., Wai, S. N., and Uhlin, B. E. (2018). Enhanced biofilm formation and membrane vesicle Release by Escherichia coli expressing a commonly occurring plasmid gene, kil. Front. Microbiol. 9:2605. doi: 10.3389/fmicb.2018.02605
Olsen, R. H., and Shipley, P. L. (1975). Rp1 properties and fertility inhibition among P-Incompatibility, N-Incompatibility, W-Incompatibility, and X-Incompatibility group plasmids. J. Bacteriol. 123, 28–35. doi: 10.1128/jb.123.1.28-35.1975
Ong, C. L. Y., Beatson, S. A., McEwan, A. G., and Schembri, M. A. (2009). Conjugative plasmid transfer and adhesion dynamics in an Escherichia coli Biofilm. Appl. Environ. Microb. 75, 6783–6791. doi: 10.1128/Aem.00974-09
R Core Team (2018). R: A Language and Environment for Statistical Computing. Vienna: R Foundation for Statistical Computing.
Reisner, A., Holler, B. M., Molin, S., and Zechner, E. L. (2006). Synergistic effects in mixed Escherichia coli biofilms: conjugative plasmid transfer drives biofilm expansion. J.. Bacteriol.. 188, 3582–3588. doi: 10.1128/Jb.188.10.3582-3588.2006
Rendueles, O., and Ghigo, J. M. (2012). Multi-species biofilms: how to avoid unfriendly neighbors. FEMS Microbiol. Rev. 36, 972–989. doi: 10.1111/j.1574-6976.2012.00328.x
Roder, H. L., Hansen, L. H., Sorensen, S. J., and Burmolle, M. (2013). The impact of the conjugative IncP-1 plasmid pKJK5 on multispecies biofilm formation is dependent on the plasmid host. FEMS Microbiol. Lett. 344, 186–192. doi: 10.1111/1574-6968.12175
Sorensen, S. J., Bailey, M., Hansen, L. H., Kroer, N., and Wuertz, S. (2005). Studying plasmid horizontal transfer in situ: a critical review. Nat. Rev. Microbiol. 3, 700–710. doi: 10.1038/nrmicro1232
Stalder, T., and Top, E. (2016). Plasmid transfer in biofilms: a perspective on limitations and opportunities. Npj Biofilms Microbi. 2:16022. doi: 10.1038/npjbiofilms.2016.22
Szabo, M., Nagy, T., Wilk, T., Farkas, T., Hegyi, A., Olasz, F., et al. (2016). Characterization of two multidrug-resistant IncA/C Plasmids from the 1960s by Using the MinION sequencer device. Antimicrob. Agents Ch. 60, 6780–6786. doi: 10.1128/Aac.01121-16
Teodosio, J. S., Simoes, M., and Mergulhao, F. J. (2012). The influence of nonconjugative Escherichia coli plasmids on biofilm formation and resistance. J. Appl. Microbiol. 113, 373–382. doi: 10.1111/j.1365-2672.2012.05332.x
Vogwill, T., and MacLean, R. C. (2015). The genetic basis of the fitness costs of antimicrobial resistance: a meta-analysis approach. Evol. Appl. 8, 284–295. doi: 10.1111/eva.12202
Yang, X. O., Ma, Q., and Wood, T. K. (2008). The R1 conjugative plasmid increases Escherichia coli biofilm formation through an envelope stress response. Appl. Environ. Microb. 74, 2690–2699. doi: 10.1128/Aem.02809-07
Yoshioka, Y., Ohtsubo, H., and Ohtsubo, E. (1987). Repressor gene fino in plasmids R100 and Plasmid-F - constitutive transfer of Plasmid-F Is caused by insertion of Is3 into F-Fino. J. Bacteriol. 169, 619–623. doi: 10.1128/jb.169.2.619-623.1987
Keywords: conjugative plasmids, biofilms, interactions, dominance, Escherichia coli
Citation: Gama JA, Fredheim EGA, Cléon F, Reis AM, Zilhão R and Dionisio F (2020) Dominance Between Plasmids Determines the Extent of Biofilm Formation. Front. Microbiol. 11:2070. doi: 10.3389/fmicb.2020.02070
Received: 24 February 2020; Accepted: 06 August 2020;
Published: 26 August 2020.
Edited by:
Clay Fuqua, Indiana University Bloomington, United StatesReviewed by:
Jean-Marc Ghigo, Institut Pasteur, FrancePascale Beauregard, Université de Sherbrooke, Canada
Copyright © 2020 Gama, Fredheim, Cléon, Reis, Zilhão and Dionisio. This is an open-access article distributed under the terms of the Creative Commons Attribution License (CC BY). The use, distribution or reproduction in other forums is permitted, provided the original author(s) and the copyright owner(s) are credited and that the original publication in this journal is cited, in accordance with accepted academic practice. No use, distribution or reproduction is permitted which does not comply with these terms.
*Correspondence: João Alves Gama, am9hby5nYW1hQHVpdC5ubw==; Francisco Dionisio, ZmFkaW9uaXNpb0BmYy51bC5wdA==; ZnJhbmNpc2NvLmRpb25pc2lvQGdtYWlsLmNvbQ==