- 1Université Clermont Auvergne, INRAE, MEDiS, Clermont-Ferrand, France
- 2UMR Inserm 1071, USC-INRAE 2018, M2iSH, Université Clermont Auvergne, Clermont-Ferrand, France
- 3Laboratoire de Bactériologie, CHU Clermont-Ferrand, Clermont-Ferrand, France
Escherichia coli is a versatile bacterial species that includes both harmless commensal strains and pathogenic strains found in the gastrointestinal tract in humans and warm-blooded animals. The growing amount of DNA sequence information generated in the era of “genomics” has helped to increase our understanding of the factors and mechanisms involved in the diversification of this bacterial species. The pathogenic side of E. coli that is afforded through horizontal transfers of genes encoding virulence factors enables this bacterium to become a highly diverse and adapted pathogen that is responsible for intestinal or extraintestinal diseases in humans and animals. Many of the accessory genes acquired by horizontal transfers form syntenic blocks and are recognized as genomic islands (GIs). These genomic regions contribute to the rapid evolution, diversification and adaptation of E. coli variants because they are frequently subject to rearrangements, excision and transfer, as well as to further acquisition of additional DNA. Here, we review a subgroup of GIs from E. coli termed pathogenicity islands (PAIs), a concept defined in the late 1980s by Jörg Hacker and colleagues in Werner Goebel’s group at the University of Würzburg, Würzburg, Germany. As with other GIs, the PAIs comprise large genomic regions that differ from the rest of the genome by their G + C content, by their typical insertion within transfer RNA genes, and by their harboring of direct repeats (at their ends), integrase determinants, or other mobility loci. The hallmark of PAIs is their contribution to the emergence of virulent bacteria and to the development of intestinal and extraintestinal diseases. This review summarizes the current knowledge on the structure and functional features of PAIs, on PAI-encoded E. coli pathogenicity factors and on the role of PAIs in host–pathogen interactions.
Introduction
Escherichia coli is a versatile bacterial species that has an extensive phylogenetic substructure comprising eight phylogroups (A, B1, B2, C, D, E, F, and G) that are roughly linked to the lifestyles of the different strains (Milkman, 1973; Selander et al., 1987; Escobar-Páramo et al., 2004a; Clermont et al., 2019). E. coli strains colonize the gastrointestinal tract of human infants and coexist in good health with the host, with strains providing mutual benefits for decades. These commensal strains rarely cause diseases in healthy hosts, as they lack specialized virulence traits; they typically originate from phylogroup A (Herzer et al., 1990; Escobar-Páramo et al., 2004b). However, other E. coli strains acquire virulence attributes that confer them with the ability to adapt to new niches and cause intestinal and extraintestinal diseases (Le Gall et al., 2007; Tenaillon et al., 2010). Consequently, E. coli strains can be classified into three groups: commensal/probiotic strains, intestinal-pathogenic strains and extraintestinal-pathogenic strains.
Intestinal-pathogenic E. coli (InPEC), which are rarely encountered in the fecal flora of healthy hosts, cause gastroenteritis or colitis. Six well-described pathotypes of diarrheagenic E. coli (DEC) have been identified: enteropathogenic E. coli (EPEC), enterohemorrhagic E. coli (EHEC), enterotoxigenic E. coli (ETEC), enteroaggregative E. coli (EAEC), enteroinvasive E. coli (EIEC) and diffusely adherent E. coli (DAEC) (Nataro and Kaper, 1998; Kaper et al., 2004). The DEC pathotypes can share certain virulence features; however, each pathotype possesses a specific combination of virulence traits that results in a distinctive pathogenic mechanism. The EPEC strains are not specifically classified as a phylogroup, although some studies preferentially associate them with phylogroup B1 (Reid et al., 2000; Wang et al., 2013). The EHEC are distributed preferentially between phylogroups A and B1 (Askari Badouei et al., 2015; Martins et al., 2015). However, the EHEC of serotype O157: H7 belongs to phylogroup E (Girardeau et al., 2005).
Extraintestinal E. coli (ExPEC) are facultative pathogens responsible for 80% of urinary tract infections (UTIs) in outpatients. Infection by ExPEC leads to a large portion of nosocomial UTIs (50%) and is the leading cause of abscesses, accounting for 30% of meningitis in neonates (Gransden et al., 1990; Johnson, 1991; Russo and Johnson, 2000; Foxman, 2003; Nielubowicz and Mobley, 2010). Bacteremia and septic shock can accompany infections at any site. ExPEC typically belong to the phylogroup B2 – an E. coli genetic background that accumulates virulence factors – and occasionally to phylogroups D, F or G (Tourret and Denamur, 2016; Clermont et al., 2019). Group B2 has the greatest diversity among all E. coli phylogroups (Touchon et al., 2009), suggesting that it has subspecies status and includes subgroups correlated with a flexible gene pool (Le Gall et al., 2007; Lescat et al., 2009). This flexible gene pool comprises genes for various combinations of virulence factors, such as adhesins, iron-acquisition systems, host defense-avoidance mechanisms and toxins (Croxen and Finlay, 2010). The link between the evolutionary lineages of E. coli, certain extraintestinal virulence genes and infection sites has led to the concepts of uropathogenic E. coli (UPEC), sepsis-associated E. coli (SEPEC), neonatal meningitis E. coli (NMEC) and avian pathogenic E. coli (APEC; which cause extraintestinal infections in poultry). Finally, a non-diarrheagenic InEC pathotype, called adherent-invasive E. coli (AIEC), has been noted for its association with inflammatory bowel diseases such as Crohn’s disease (Darfeuille-Michaud et al., 2004). AIEC is considered a pathobiont bacterium rather than a bacterium responsible for acute infection, and it frequently belongs to the phylogroup B2, like ExPEC, and shares with ExPEC the most virulence traits.
Whole-genome E. coli phylogeny suggests an evolution of ExPEC from commensal E. coli strains that were originally devoid of virulence factors but became pathogenic because of horizontal gene transfers involving transduction, transformation, and conjugation events (Lo et al., 2015). Phages, plasmids and large parts of the genome, designated as genomic islands (GIs), were transferred from one bacterium to another (Benedek and Schubert, 2007; Schubert et al., 2009; Schneider et al., 2011; Messerer et al., 2017). These types of GIs carrying virulence-associated genes were identified in UPEC in the early 1980s by Hacker et al. (1983, 1990) and were designated as pathogenicity islands (PAIs) (Blum et al., 1994). Since then, PAIs have been described from SEPEC, MNEC and diarrheagenic isolates and in other species, such as Salmonella enterica. The species Escherichia coli and Salmonella enterica share 70% of their genome (McClelland et al., 2001) and have recently diverged (Doolittle et al., 1996). The divergence of both species has been, in large part, due the acquisition of specific PAIs and has resulted in very different lifestyles (Nieto et al., 2016). This review summarizes the current knowledge on the structure and functional features of PAIs, on E. coli PAI-encoded pathogenicity factors and on the role of PAIs in host-pathogen interactions.
Structural Features of Pathogenicity Islands
Pathogenicity islands are a group of large (>10 kb) integrative elements that encode one or more virulence genes that are absent from the genomes of non-pathogenic representative bacteria of the same species or of closely related species (Blum et al., 1994; Hacker and Carniel, 2001; Dobrindt et al., 2004; Schmidt and Hensel, 2004). In contrast to other integrative elements, such as bacteriophages, plasmids or integrative and conjugative elements (ICEs), PAIs are non-replicative and lack the ability to self-mobilize.
Comparison of the genomic region of PAIs and the remaining parts of the host genome shows that PAIs have their own genomic characteristics, which is strong evidence of their foreign origin and horizontal acquisition. The G + C contents (i.e., the percentage of guanine and cytosine bases), the frequency of dinucleotides or high-order oligonucleotides and the codon usage in PAIs often differ from those of the host organisms (Groisman and Ochman, 1996; Karlin, 2001; Dobrindt et al., 2004). For instance, the G + C content of the 536 UPEC core genome is 50%, while the G + C content is 41% in the PAIs I536, II536, IV536, and V536. In the EPEC genome, the G + C content of the LEE (locus of enterocyte effacement) PAI is only 39%, while the G + C content of the E. coli core genome is ∼50%. However, the donor and recipient organisms may possibly have a similar G + C sequence composition, thereby complicating the extraction of PAIs from the core genome. Even for donor and recipient organisms with different sequence compositions, the PAI region can be “ameliorated” throughout evolution, making the sequence composition or codon usage of the PAI region similar to that of the core genome (Lawrence and Ochman, 1997). The divergences in GC content can therefore reflect a recent acquisition and/or an evolutionary mechanism that maintains a divergence in GC content. This divergence can have a functional role, since curved and AT-rich PAIs are preferential targets of the global gene silencer H-NS (Lucchini et al., 2006; Navarre et al., 2006; Oshima et al., 2006). The H-NS-mediated silencing prevents the uncontrolled transcription of genes within PAIs to ensure that bacterial fitness is maintained, and it may also have evolutionary consequences by influencing the acquisition and maintenance of foreign DNA (Lucchini et al., 2006; Navarre et al., 2006; Oshima et al., 2006).
Nonetheless, most GIs contain a recombination module (Figure 1A) that is also observed in other integrative elements, such as phages, integrons, conjugative transposons and ICEs. The module consists of three parts: (i) an integrase of the tyrosine recombinase family; (ii) two flanking attachment sites forming direct repeats resembling the attR and attL sites of prophages; and, in some cases, and (iii) a recombination directionality factor (RDF). This recombination module is characteristic of the integrative elements that insert a circular intermediate into the host genome. However, island-encoded integrases form a separate clade within the tyrosine recombinase family (Boyd et al., 2009). Therefore, PAIs are a distinct class of integrative elements and are not degenerate remnants of other mobile elements (Fogg et al., 2011).
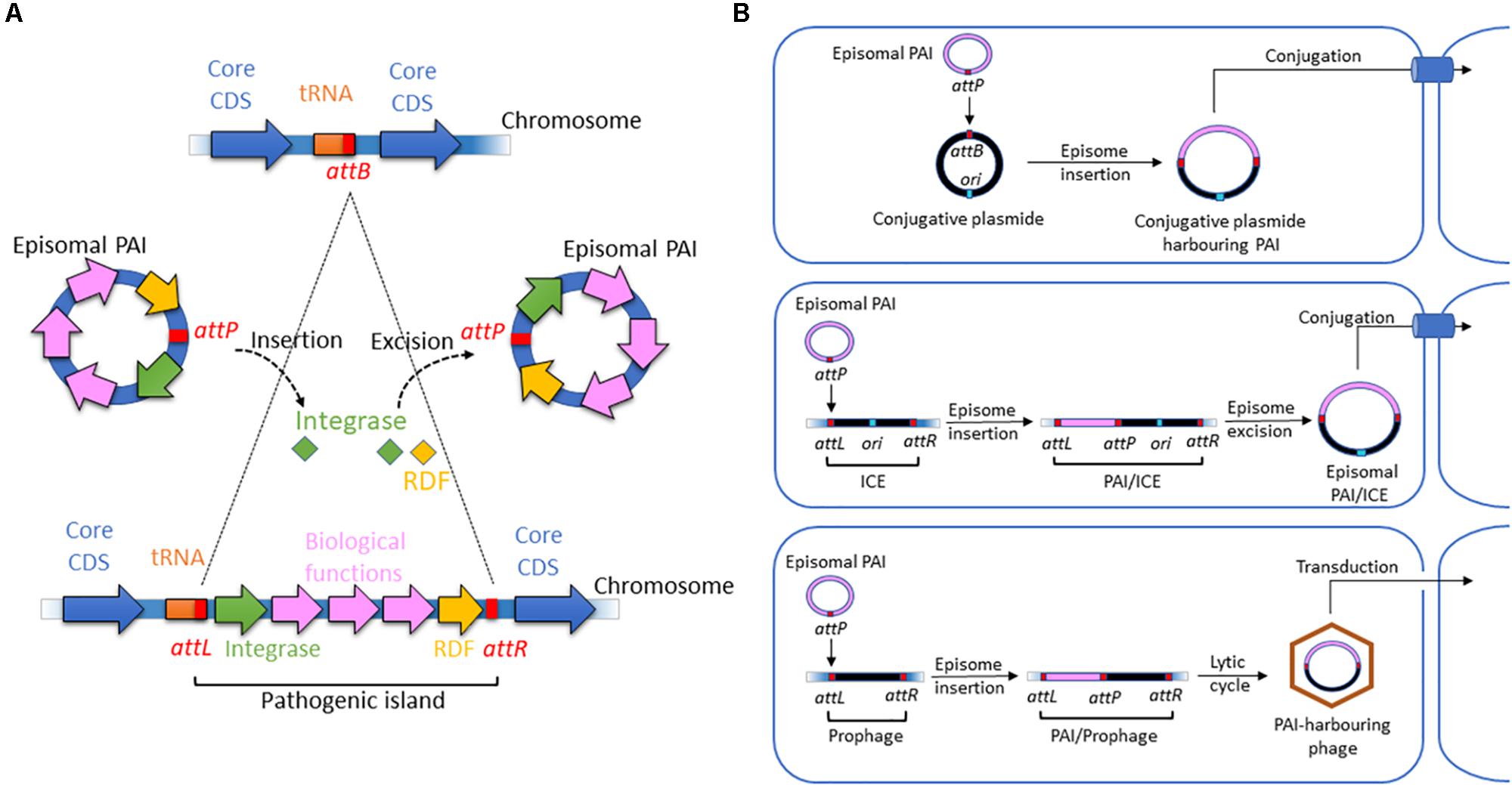
Figure 1. Structure and motility functions of PAIs. (A) Insertion and excision processes of PAIs. PAIs are chromosomal fragments of pathogenic bacteria that encode biological functions involved in virulence. Their insertion in the chromosome is due to the presence of att sites at a chromosomal acceptor site (attB) and in the episomal PAI (attP). They are recognized by integrases, which catalyze a recombination of att sites. It results in the insertion of the episomal element at the attB site and the formation of direct repeated sequences (DRS) also named attL (left DRS) and attR (right DRS) in the ends of the inserted PAI. The excision of the PAI results from recombination between the direct repeats attL and attR. Catalyzed by integrases and recombination directionality factors (RDFs) also called excisionases, it generates an episomal element that contains one of the att sites (attP), while the other att site remains in the chromosome (attB). (B) Horizontal transfer of PAIs via conjugative plasmids, ICEs, and phages harboring att sites. Episomal PAIs can be inserted at att sites in conjugative plasmids, ICEs and phages as described above and then transferred into a bacterial recipient via conjugation for ICE-type and plasmid-type navettes or via transduction for phage-type navettes.
Most island-related integrases are inserted adjacent to tRNA or transfer-messenger RNA (tmRNA) loci (Figure 1A). However, the number of specific tRNA genes used is limited. Within the E. coli genome, ∼87 tRNA genes have been annotated, and integrative genetic elements use only a handful of these sites. Fifteen tDNAs are hotspots of integration, with asnT, aspV, leuX, metV, pheV, and thrW as the most frequently targeted genes (Reiter et al., 1989; Cheetham and Katz, 1995; Williams, 2003). The tDNA pattern targeted by insertions may differ in B2 and A/B1 E. coli strains, potentially influencing their ability to acquire and lose PAIs (Escobar-Páramo et al., 2004a; Germon et al., 2007).
Pathogenicity islands often include mobile elements (or fragments thereof), such as bacteriophages, plasmids and insertion sequences. These mobile elements play an important role in recombination, resulting in genetic rearrangements, insertions, deletions and, therefore, variation of PAIs. The elements contribute to the formation of mosaic-like structures, another hallmark of PAIs that promotes the accretion of traits into islands (Dobrindt et al., 2010).
A number of E. coli PAIs have the PAI features mentioned above; however, some lack one, two or even more features, making the detection of PAIs from sequenced genomes a challenge. The detection methods usually use the following indicative features of the horizontal origin of GIs and PAIs: (i) biased sequence composition, (ii) gene or motif content (i.e., tRNA/tmRNA, direct repeats, integrases, mobility-related genes, high prevalence of hypothetical proteins and virulence/metabolic/antibiotic resistance genes to subclassify the GIs), and (iii) sporadic phylogenetic distribution assessed by the identification of regions only present in a subset of genomes and/or containing genes usually found in PAIs, such as virulence genes, transposase, integrases or genes coding unknown functions (Langille et al., 2010; Lu and Leong, 2016; Bertelli et al., 2019). Composite detection methods, such as IslandViewer (Bertelli et al., 2017) and GIHunter (Han Wang, 2014), are the most sensitive methods (Bertelli et al., 2019). The data with the highest accuracy are provided by tools such as Islander, which can detect tRNA sequence direct repeats (Hudson et al., 2015). Using these in silico approaches, the databases of predicted or curated GIs have been developed from publicly available genome sequences and provide a large sampling of structural variations and gene content in PAIs (Bi et al., 2012; Hudson et al., 2015; Yoon et al., 2015; Bertelli et al., 2017; Li et al., 2018).
Instability and Motiliy of Pathogenicity Islands
Pathogenicity islands in ExPEC and InPEC can undergo deletion at frequencies ranging from 5 × 10–3 to 1 × 10–6 (Turner et al., 2001; Tauschek et al., 2002; Middendorf et al., 2004; Bielaszewska et al., 2011). In many cases, excision and integration seem to be mediated by PAI-encoded integrases (Hochhut et al., 2006). These harbor a highly conserved C-terminal domain involved in recombination and a more divergent N-terminal domain that specifically recognizes the integration site attB (Figure 1A). During the acquisition, the PAI-specific attachment site attP is frequently integrated adjacent to the 3’ end of tDNAs, as observed for phage integration. The recombination results in the direct duplication of the attachment site attB, which forms 16 to 130 bp direct repeats (attL and attR) that flank the PAIs. If multiple isoacceptor tDNAs exist, chromosomal insertion may occur at all available loci, as observed for the high-pathogenicity island (HPI), LEE PAI or PAI IIJ96 (Buchrieser et al., 1998; Tauschek et al., 2002; Bidet et al., 2005). Integrase also excises PAIs from the genome as circular non-replicative intermediates (Figure 1A) using a site-specific recombination process (Rajanna et al., 2003; Hochhut et al., 2006; Wilde et al., 2008). In UPEC strain 536, excision of PAIs I536, II536 and III536 depends on their own integrases. However, PAI V536 undergoes excision even in the absence of its integrase, which can be substituted by the integrase of PAI V536, suggesting crosstalk between PAIs (Hochhut et al., 2006). Enzymes called excisionases or recombination directionality factors (RDFs) can assist integrases (Lewis and Hatfull, 2001; Sakellaris et al., 2004). A bioinformatic analysis showed that each PAI in UPEC 536 contained its own cognate putative RDF (Napolitano et al., 2011). RDFs act as positive or negative integrase transcriptional regulators and offer stability to their integrase protein partners at the excision site (Numrych et al., 1992; Panis et al., 2010). The mobility of PAI can also be independent of the recombination module and involve homologous DNA recombination (Schubert et al., 2009).
Horizontal transfer (HT) is another aspect of PAI mobility (Figure 1B). Because most islands do not contain an origin of replication and are not able to self-mobilize, HT of excised PAIs has been hypothesized to occur with the help of bacteriophages, ICEs or conjugative plasmids (Middendorf et al., 2004). The presence of phage-related sequences on most PAIs suggests that phages have a key role in HT (Boyd et al., 2001; O’Shea and Boyd, 2002). Alternatively, PAIs can be transferred by conjugation in the presence of an attB-presenting helper replicon and accessory transfer genes (Schubert et al., 2009; Schneider et al., 2011). An alternative mechanism, independent of the att site, is homologous DNA recombination, which involves sequences shared by plasmids, a PAI or its environment, and the recipient genome (Schubert et al., 2009). The stabilization of beneficial genetic information localized on mobile genetic elements can then be achieved by the selective loss of transfer or mobilization functions encoded by these elements.
Non-Diarrheagenic Escherichia coli
Physiopathology of ExPEC and AIEC Infections
The non-diarrheagenic E. coli are opportunistic pathogens. ExPEC take advantage of host behavior and susceptibility by employing virulence factors (Figure 2) to colonize the digestive tract and then move on to the bladder, where they cause cystitis. The cystitis infection can ascend through the ureters to the kidneys, eventually causing pyelonephritis, and potentially reaching the blood compartment to cause sepsis (Johnson, 1991; Nielubowicz and Mobley, 2010). ExPEC use cell-surface adhesins to adhere to the host’s epithelial cells (Croxen and Finlay, 2010). Adhesin-receptor interactions, invasion factors and bacterial engulfment-enhancing toxins stimulate bacterial internalization into apical uroepithelium cells, leading to invading bacteria that are endocytosed into membrane vesicles (Martinez et al., 2000; Eto et al., 2007). The ExPEC then escape from the endocytic vesicles (Mulvey et al., 1998, 2001; Anderson et al., 2003; Eto et al., 2007; Rosen et al., 2007; Flores-Mireles et al., 2015), proliferate inside the cellular cytoplasm, and form intracellular bacterial communities (IBCs) (Mulvey et al., 1998, 2000; Wright et al., 2007; Flores-Mireles et al., 2015).
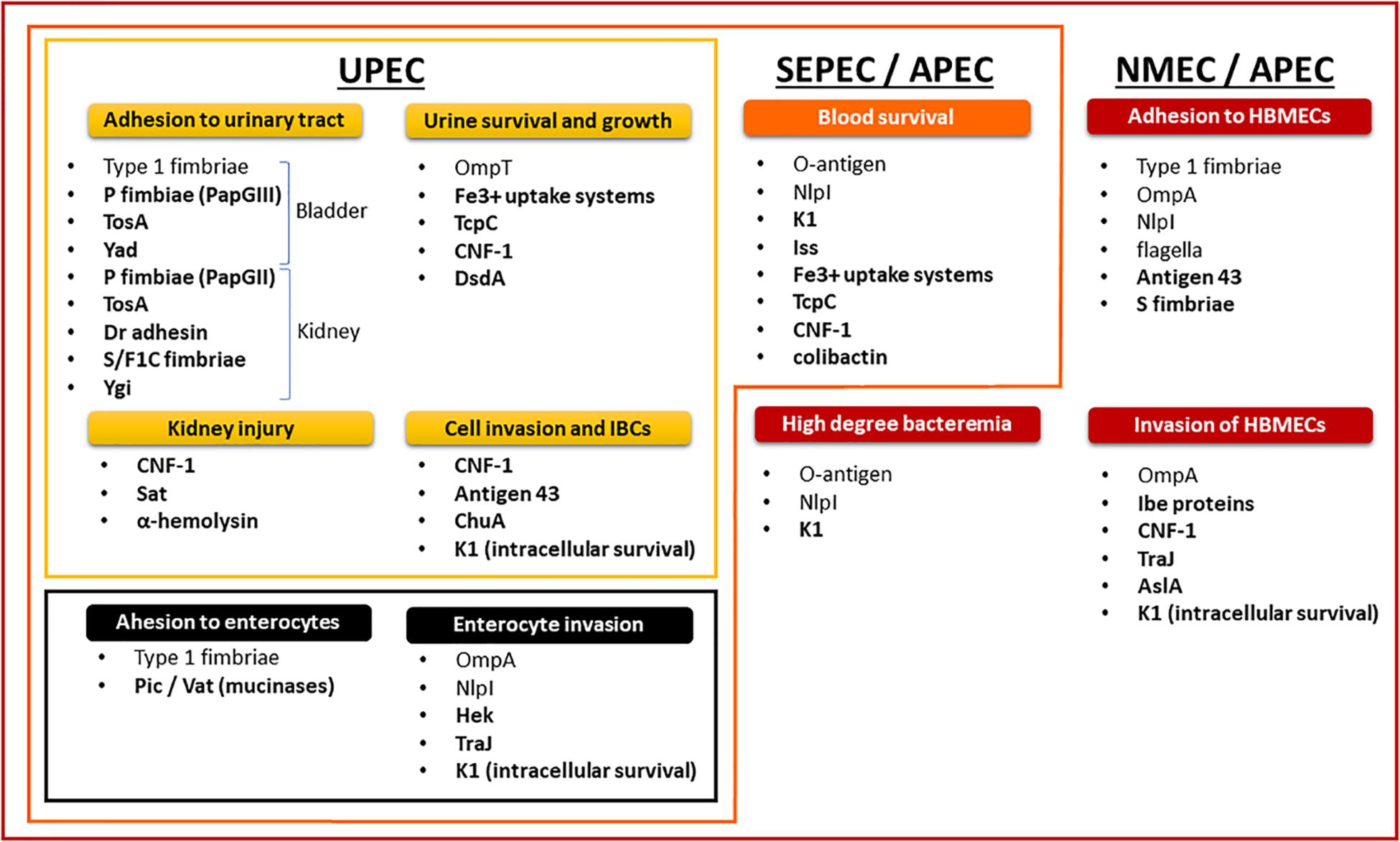
Figure 2. Nesting distribution of pathogenicity factors in ExPEC pathotypes and their role in the physiopathology of infections.
Intracellular bacterial communities constitute biofilm-like quiescent reservoirs that preserves ExPEC viability and can lead to recurrent UTIs (Eto et al., 2006). Cytosolic E. coli also induce cell lysis and exfoliation (Mulvey et al., 1998, 2000). The innate immune response initiated by infected uroepithelium cells and exfoliation clears many bacteria from the urinary tract with the flow of urine, but this also leaves the underlying layers of immature bladder epithelial cells exposed and more susceptible to infection (Mulvey et al., 1998, 2000, 2001; Bower et al., 2005; Wiles et al., 2008). In a small proportion of UTIs, ExPEC will decrease the expression of bladder-targeting adhesins and enhance the expression of both kidney-targeting adhesins and bacterial motility (Flores-Mireles et al., 2015). Adhesion and mobility allow ExPEC to ascend through the ureters to the kidneys, where they cause acute pyelonephritis (Svanborg et al., 2001).
Asymptomatic bacteriuria (ABU) occurs in up to 6% of healthy individuals and in 20% of elderly individuals. ABU-associated E. coli persist for months or years without evoking a destructive mucosal host response (Kunin and Halmagyi, 1962; Lindberg, 1975). E. coli strains lacking virulence genes and a defect in TLR4 signaling lead to the asymptomatic carrier state (Andersson et al., 1991; Hull et al., 1999; Frendéus et al., 2001; Svanborg et al., 2001; Bergsten et al., 2005; Fischer et al., 2006; Klemm et al., 2006; Ragnarsdóttir et al., 2008; Grönberg-Hernández et al., 2011).
Bacterial destabilization of the renal epithelium and subsequent inflammation result in renal scarring, which acts as a conduit to the bloodstream. One study showed that 91% of E. coli strains isolated from the blood and urine of the same hospitalized patients were closely related, suggesting that UPEC is the main origin of septicemias and SEPEC (Vollmerhausen et al., 2014). The strains originating from UTIs can also be found in the guts of the patients (Moreno et al., 2008). Accordingly, overgrowing intestinal ExPEC strains can also translocate through the gut epithelium and survive in mesenteric lymph nodes to enter the bloodstream (Bark et al., 1995; MacFie et al., 1999; Ljungdahl et al., 2000; Owrangi et al., 2018). Throughout their progression, ExPEC use numerous capture systems to find the necessary elements, particularly iron, to aid their growth (Braun, 2003). In the bloodstream, SEPEC protect themselves from the immune system by surface structures and effectors which allow them to escape the bactericidal activity of complement and phagocytosis (Cross et al., 1984; Kim et al., 1992; Kim, 2016). This resistance is a factor in the induction of a high degree of bacteremia (Kim, 2016).
Several in vivo studies on NMEC have pointed out a relationship between the magnitude of bacteremia and the development of meningitis (Kim, 2016). E. coli binding and the invasion of human brain microvascular endothelial cells (HBMECs) mediated by E. coli pathogenicity factors is also a prerequisite (Huang et al., 1995, 1999; Prasadarao et al., 1996; Wang et al., 1999; Hoffman et al., 2000; Zhao et al., 2018). The vacuoles containing NMEC that result from HBMEC invasion evolve as endosomes without fusion with lysosomes, thereby allowing E. coli to cross the blood-brain barrier alive (Kim et al., 2003).
Avian pathogenic E. coli strains cause avian colibacillosis, a poultry extraintestinal infection. Extensive genetic similarity has been documented between APEC and ExPEC strains (Rodriguez-Siek et al., 2005; Moulin-Schouleur et al., 2006; Johnson et al., 2007, 2012; Mora et al., 2009, 2012). APEC can cause diseases in mammalian infection models, and these diseases mimic human ExPEC infections (Tivendale et al., 2010). Conversely, human sources of ExPEC can cause diseases in avian species. Human ExPEC and APEC therefore share similar pathogenic potential, and some human-associated ExPEC may have evolved from APEC, and vice versa (Skyberg et al., 2006).
Adherent-invasive E. coli strains have an increased prevalence in inflammatory bowel disease (IBD). AIEC strains harbor genetic similarity with ExPEC in terms of phylogenetic origin and pathogenicity genotype. However, only 6.3% of ExPEC strains exhibit AIEC phenotypic features, suggesting that the AIEC pathotype is disease specific and contributes to the pathophysiology of chronic IBD (Palmela et al., 2018). AIEC bacteria interact with the intestinal mucosa and can (i) cross the mucus layer and resist antimicrobial peptides, (ii) adhere to the surface of the mucosa and increase intestinal permeability, and (iii) penetrate the lamina propria, persist in macrophage vacuoles and chronically stimulate the immune system. Apart from having a high genotypic variability, AIEC belong mainly to phylogroup B2 and resemble ExPEC in terms of virulence factor content. However, AIEC could have selected an original regulatory system and/or pathoadaptive mutations to enable better adaptation to intestinal conditions.
PAIs in ExPEC and AIEC
Experimental and epidemiological investigations of ExPEC pathogenicity factors (PFs) show that no PF is limited to, and no single clone is required for, any particular extraintestinal infection. Statistical analyses of PF content and the resulting lethality in an animal model suggested that the virulence of ExPEC is more likely linked to the number of PFs than to the genetic background (Picard et al., 1999; Johnson and Kuskowski, 2000). In addition, the success of pathogens at various host sites can be due to multiple combinations of PFs, and virulence potential probably exists as a continuum that depends on the number and type of PFs and the inoculum size (Russo and Johnson, 2000). The prevalence of PFs in ExPEC infections is reported in Table 1.
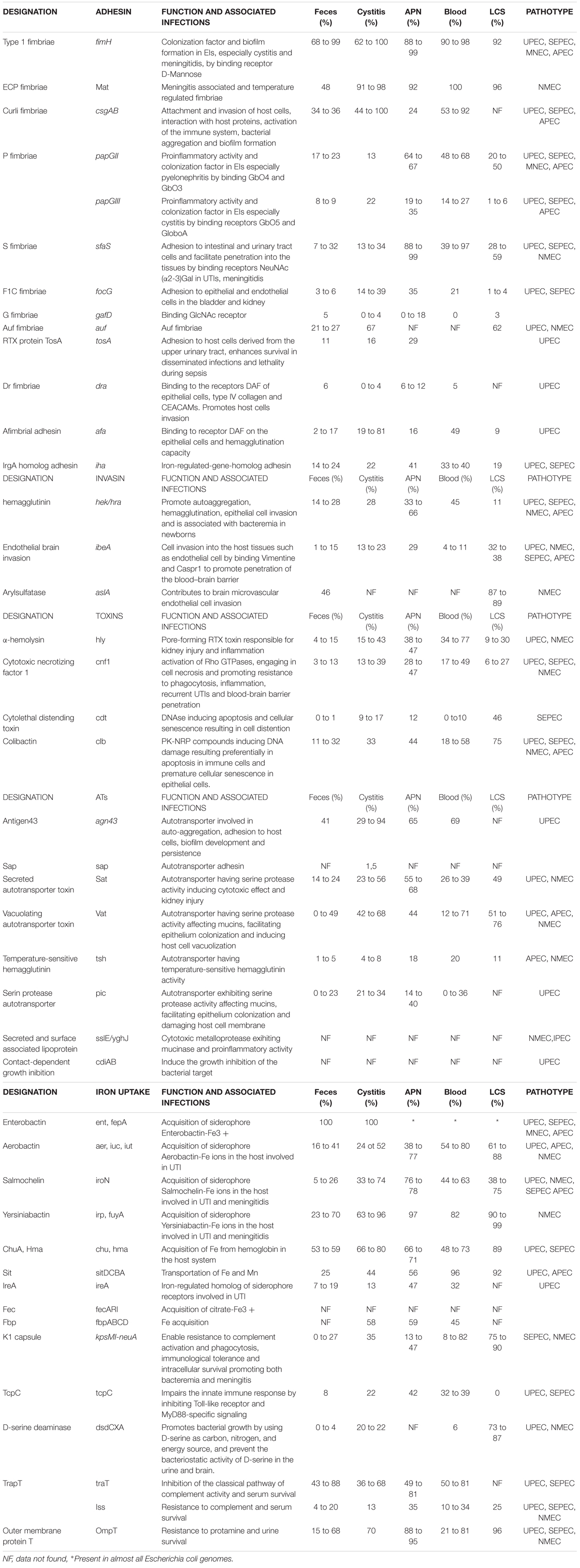
Table 1. Prevalence of virulence factors in ExPEC according to the source (Korhonen et al., 1985; Guyer et al., 2000, 2000; Russo et al., 2001; Bingen-Bidois et al., 2002; Johnson et al., 2002, 2004, 2008; Marrs et al., 2002; Bonacorsi et al., 2003; Russo, 2003; Srinivasan et al., 2003; Buckles et al., 2004; Bonacorsi and Bingen, 2005; Parham et al., 2005a; Rendón et al., 2007; Restieri et al., 2007; Ananias and Yano, 2008; Henderson et al., 2009; Schubert et al., 2010; Ejrnæs et al., 2011; Mahjoub-Messai et al., 2011; Vejborg et al., 2011; Lehti et al., 2012; Qin et al., 2013; Tarchouna et al., 2013; Firoozeh et al., 2014; Basmaci et al., 2015; Nagarjuna et al., 2015; Wijetunge et al., 2015; Beck et al., 2016; Cordeiro et al., 2016; Lee and Lee, 2018; Daga et al., 2019; Nojoomi and Ghasemian, 2019; Raimondi et al., 2019; Sarowska et al., 2019).
Most ExPEC PFs are encoded by PAIs (Table 2), and the encoded functions are involved in critical steps of the infectious process. The cooperative effect of PAIs has been experimentally confirmed with the prototype strains 536, CFT073 and RS218 (Silver et al., 1980; Hacker et al., 1983; Brzuszkiewicz et al., 2006) by monitoring UTI, septicemia and meningitis mouse models using single-PAI and/or multiple-PAI deletion mutants (Schubert et al., 2002; Xie et al., 2006; Lloyd et al., 2009a; Tourret et al., 2010). These studies showed an additive contribution of PAIs to extraintestinal virulence, but redundancies (i.e., iron uptake) were also evident. The involvement of PAIs in virulence also depends on the genetic background and on the infection model used to test them, suggesting a complex network of pathogenetic possibilities resulting from multiple and independent acquisitions of functions without an unequivocal goal. Given the functional redundancies in PAIs, and to provide an overview of the pathogenicity functions encoded by ExPEC PAIs, their PF content is presented here by PF type and the main structural features of corresponding PAIs is reported in Table 2 and Figure 3 for reference strains.
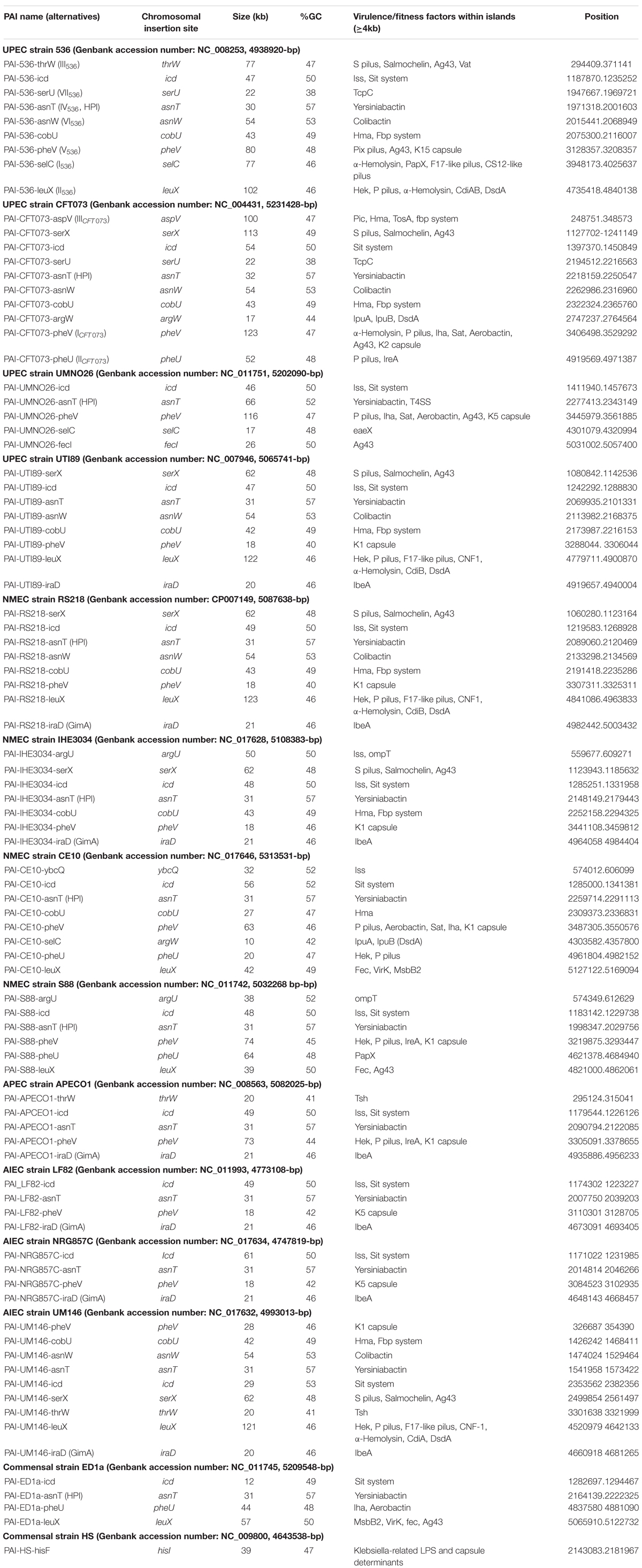
Table 2. Islands predicted by IslandViewer and/or genomic comparison to E. coli K12 from sequenced strains belonging to pathotypes UPEC (536, CFT073, UMNO26, and UTI89), NMEC (RS218, IHE3034, CE10, and S88), APEC (APECO1) and AIEC (LF82, NRG857C, and UM146) and commensal strains (ED1a and HS).
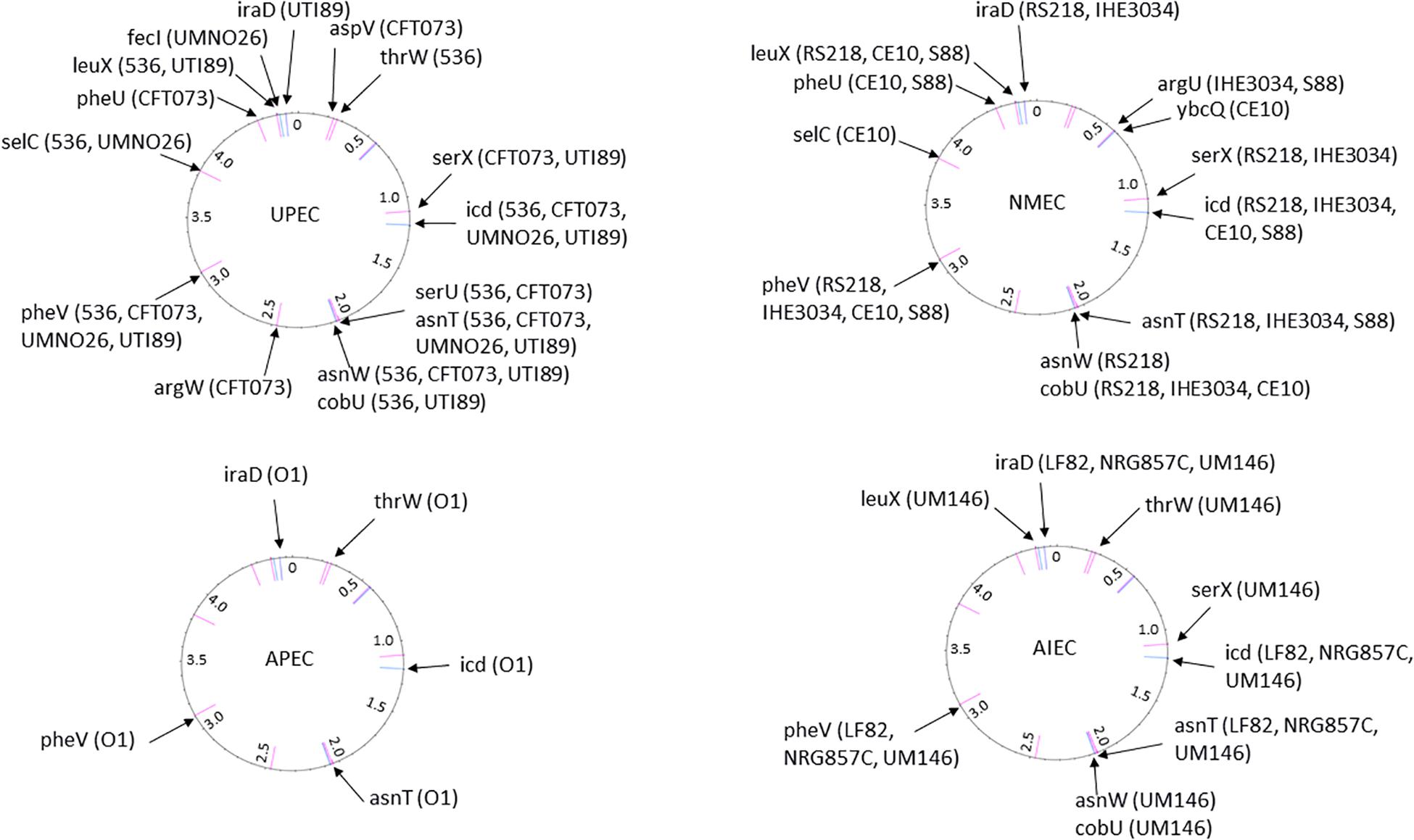
Figure 3. The position of PAI insertion sites in the non-diarrheagenic E. coli pathotypes. The name of genetic features associated with the insertion is indicated with the name of bacterial strains harboring PAI at the corresponding site. The position of the insertion site is indicated in pink for tRNA genes and in blue for coding genes. The numbers reported in the inner ring are the position (megabase) in the genome of the non-pathogenic E. coli strain MG1565 used as a reference.
Adhesins Encoded or Regulated by PAIs
The capacity of E. coli to adhere to host cells plays a fundamental role in the colonization of any site. Adhesion is mediated by proteins called adhesins, which specifically recognize receptors and are often contain antigenic sugars that are present on the surface of host cells. Adhesins are exposed directly to the surface of bacteria or are carried by filamentous structures called “fimbriae” or “pili” (Table 1). Most adhesins are encoded by PAIs, except for conserved fimbriae, such as type 1 fimbriae, curly fibers, outer membrane protein A (OmpA), chitin-binding domain of chiA and new lipoprotein I (NlpI) that are involved in the adhesion of AIEC and NMEC to enterocytes and HBMECs (Kim, 2003, 2008).
Type 1 fimbriae are present in more than 80% of E. coli strains and other Enterobacteriales (Abraham et al., 1988). However, they are major players in ExPEC virulence, and their expression is regulated by PAIs. The type 1 fimbria adhesin FimH binds specifically to α-D-mannose residues attached to membrane glycoproteins exposed at the surface of bladder cells, enterocytes and the endothelial cells of brain capillaries (Keith et al., 1986; Teng et al., 2005). The role of type 1 pili in cystitis has been demonstrated by mutagenesis and in animal models (Keith et al., 1986; Johnson, 1991; Connell et al., 1996). Their adhesin FimH binds to glycosylated uroplakin and α1β3 integrins covering the apical surface of uroepithelium cells, thereby promoting the invasion of host cells and the development of IBCs (Connell et al., 1996; Martinez et al., 2000; Gunther et al., 2002; Mysorekar and Hultgren, 2006; Eto et al., 2007; Flores-Mireles et al., 2015). Notably, in some AIEC, UPEC, MNEC and APEC strains, the protein sequence of FimH adhesin has polymorphisms that confer a greater ability to interact with D-mannose.
The expression of type 1 pili depends on a promoter encoded by the phase-variation invertible element fimS. The orientation of fimS can be reversed by the recombinases FimB and FimE encoded within the operon Fim, and by recombinases FimX, IpuA and IpbA encoded by PAIs (Klemm, 1986; McClain et al., 1991; Bryan et al., 2006; Hannan et al., 2008). IpbA is ubiquitous, as it is found approximately half the time in both commensal fecal and uropathogenic isolates of E. coli (Bryan et al., 2006). FimX-encoded PAI is observed in more than 80% of UPEC and is more prevalent in the UPEC of a lower urinary tract origin (87.5%) than of upper urinary tract origin (74%) or of commensal isolates (36%) (Bryan et al., 2006; Bateman et al., 2013). IpuA-encoding PAIs also tend to occur more frequently among UPEC strains than in commensal fecal isolates (Bateman et al., 2013). This observation is consistent with the hypothesis that FimW and IpuA recombinases play a role in the regulation of E. coli uropathogenesis. FimX, IpuA and IpbA promote inversion of fimS from OFF to ON and probably promote bladder colonization, while FimE inverts the promoter from ON to OFF and could therefore promote the release of bacteria from the bladder epithelium and the infection of the higher tract. The latter might be enhanced by a coordinated upregulation of both bacterial mobility and other adhesins, such as P fimbriae (Klemm, 1986; McClain et al., 1991; Snyder et al., 2005; Bryan et al., 2006; Hannan et al., 2008).
FimX also regulates the predicted LuxR-like response regulator HyxR, which is encoded from the same PAI and is a negative regulator of the nitrosative stress response and intracellular macrophage survival (Bateman and Seed, 2012b,a). IpuA and the associated recombinase IpuB, which lacks activity on fimS, regulate the orientation of a phase-variable invertible element ipuS, which is located in the same PAI, proximal to ipuA and ipuB (Battaglioli et al., 2018). The orientation of ipuS drives the transcription of a two-gene operon containing ipuR (a predicted LuxR-type regulator) and upaE (an autotransporter involved in biofilm formation and extracellular matrix adhesion) (Battaglioli et al., 2018). Consistent with this phenotype, the ipuS orientation ON results in (i) defective swimming motility, (ii) an increase in adhesion to human kidney epithelial cells, and (iii) promotion of kidney colonization in experimental UTI mouse models (Battaglioli et al., 2018). Overall, therefore, PAIs not only encode PFs, but they are also involved in virulence regulation networks.
P fimbriae are encoded by the pap operon (pyelonephritis associated pili), which is associated with different PAIs (Lane and Mobley, 2007). The non-structural gene papX of the pap operon is another example of the PAI gene that is involved in virulence regulation. The protein PapX binds directly to the flhDC promoter, thereby inhibiting the transcription of the master regulator of flagellar biosynthesis, motility and chemotaxis (Li et al., 2001; Simms and Mobley, 2008; Reiss and Mobley, 2011; Reiss et al., 2012). The fimbrial-tip adhesin of the P fimbria PapG (Källenius et al., 1980) binds to glycosphingolipids containing the digalactoside Gal(α1-4β) Gal moieties found in the renal epithelium, P blood-group antigen and gut epithelium (Antão et al., 2009). Three major alleles of the papG locus (GI to III) have been described, with alleles GII and GIII being the most frequently encountered in human disease (Marklund et al., 1992). Allele GII recognizes globotriosylceramide (GbO3) and globotetraosylceramide (GbO4),which are particularly abundant in the kidneys, and it is preferentially found in strains isolated from pyelonephritis because allele GIII recognizes globopentaosylceramide (GbO5) and is mainly found in strains isolated from cystitis (Karr et al., 1989; Lindstedt et al., 1991; Marrs et al., 2002). GbO5 is found in the urothelium of animals but is not present in humans, except in certain individuals expressing an analog (GloboA) and who are particularly susceptible to lower UTIs with PapGIII strains (Lindstedt et al., 1991).
PAI-CFT073-aspV harbors the tos operon, which encodes the repeat-in-toxin (RTX) family member TosA (Lloyd et al., 2009a). The RTX protein family members can be involved in a range of functions, including pore and biofilm formation and adhesion to host cells (Linhartová et al., 2010; Dhakal and Mulvey, 2012). The presence of tosA promotes bladder and kidney colonization in a UTI mouse model and is a marker of UPEC (Vigil et al., 2011b,a). Localized to the bacterial surface, TosA mediates adhesion to host cells derived from the upper urinary tract, increases bacterial survival in disseminated infections and enhances lethality during sepsis (Vigil et al., 2012). The TosCBD proteins mediate the production and export of TosA, while TosE and TosF suppress motility by an unknown regulatory function. TosR is a member of the PapB family of transcriptional regulators, including the fimbria-associated regulators PapB and FocB (Xia et al., 1998; Lindberg et al., 2008; Hultdin et al., 2010). TosR regulates the tos operon (Engstrom and Mobley, 2016) and other genes involved in adhesion (P, F1C, and Auf fimbriae) and biofilm formation (Luterbach et al., 2018).
Other fimbrial gene clusters are found in ExPEC PAIs (Table 2). F1C/S fimbriae promote adhesion to primary human renal proximal-tubular cells by binding to α-sialyl-2-3-β-galactoside (Kreft et al., 1995). The F1C/S fimbriae are frequently found in NMEC and could also be involved in the crossing of the blood-brain barrier, as occurs with type 1 fimbriae (Korhonen et al., 1985; Ott et al., 1986). However, in a meningitis animal model, the deletion of the F1C/S fimbriae operon in E. coli K1 did not significantly affect bacterial binding to and invasion of HBMECs, and the deletion also did not affect bacterial penetration into the central nervous system (Kim, 2008). Ygi fimbriae promote renal tropism, biofilm formation and in vivo fitness in the urine and kidneys (Spurbeck et al., 2011). Yad fimbriae are necessary for adhesion to a bladder epithelial cell line and biofilm formation, and the deletion of these fimbrial genes has been shown to increase motility (Spurbeck et al., 2011). A double deletion strain, Δygi Δyad, showed impaired colonization of the urine, bladder, and kidneys in a mouse model, demonstrating that these fimbriae contribute to uropathogenesis (Spurbeck et al., 2011). ExPEC also produces non-fimbrial adhesin FdeC that is highly prevalent in both commensal strains and InPEC. This adhesin binds epithelial cells and, collagen V and VI, and in vivo experiments suggest that it has a role in UTI infections (Nesta et al., 2012).
Overall, PAIs provide the ability to produce multiple fimbria that confer a selective advantage within particular niches and are involved in crosstalk between regulators of various fimbrial types and in motility.
Invasins Encoded by PAIs
Extraintestinal E. coli PAIs can produce invasins, which are structures that promote bacterial internalization within host cells. This process allows bacteria to escape targeting by the immune system and is involved in the formation of the IBCs observed in UTIs and Crohn’s disease with UPEC and AIEC, respectively. These internalizations also allow bacteria to cross epithelial and endothelial barriers by transcytosis.
The PAI-encoded protein Hek/Tia, which is more frequently found in UPEC than in commensal isolates (Table 1), is responsible for bacterial aggregation on the surface of enterocytes, as is observed for enteroaggregative E. coli, and is involved in the invasion of T84 enterocytic cells (Fagan and Smith, 2007). The mechanism behind the invasion of enterocytes and the bladder epithelium and the requirement for preliminary adhesion are still largely unknown. The invasion of HBMECs by NMEC requires binding to and the invasion of the HBMECs and involves the PAI-encoded microbial factors IbeA and cytotoxic necrotizing factor-1 (CNF-1), as well as the housekeeping factors OmpA, NlpI and arylsulfatase-like gene aslA (Huang et al., 1995, 1999, 2001; Prasadarao et al., 1996; Wang et al., 1999; Hoffman et al., 2000; Khan et al., 2002; Kim, 2003, 2008). Studies have attempted to identify the receptors at the surface of HBMECs for these bacterial factors: CNF-1 interacts with laminin receptors, while IbeA binds to both vimentin and HBMEC contactin-associated protein 1 (CASPR1) (Zou et al., 2006; Zhao et al., 2018). This latter interaction activates focal adhesion and kinase signaling, which causes E. coli internalization (Zhao et al., 2018).
Toxins Encoded by PAIs
Extraintestinal E. coli strains can use PAI-encoded genes to produce several toxins, including α-hemolysin, CNF-1, cytolethal and distending toxin (Cdt), and colibactin (Clb).
E. coli α-hemolysin is a pore-forming toxin belonging to the class of RTX toxins that are encoded by the hly operon. Although most UPEC strains carry one copy of the hemolysin operon, pyelonephritogenic strains 536 and J96 harbor two copies of the hly operon and both loci are required for full virulence (Nagy et al., 2006). The toxin is encoded by the hlyA gene. The gene hlyC encodes an acyl transferase that is required for activation of the toxin, while genes hlyB and hlyD are involved in the energy-dependent secretion of HlyA (Welch, 1991). HlyA is a hemolysin that targets different cell types, including leukocytes (Galmiche and Boquet, 2001). The involvement of α-hemolysin in virulence has been suggested because of its high prevalence in UPEC compared to its prevalence in fecal strains and because of its high expression within IBCs (Johnson, 1991; Reigstad et al., 2007). In upper urinary tract infections, α-hemolysin is thought to play both a direct cytotoxic role in renal cells and a pro-inflammatory role that causes the secretion of cytokines IL-6 and IL-8 and alters Ca2+ membrane flow (Uhlén et al., 2000). The combination of these two actions weakens the renal epithelium and promotes the passage of bacteria into the blood (Bonacorsi et al., 2006). By interfering with NF-kB-mediated proinflammatory signaling pathways and triggering the breakdown of paxillin and other host regulatory proteins, HlyA also dampens the host’s immune response to infection and enhances the exfoliation of bladder epithelial cells (Dhakal and Mulvey, 2012).
CNF-1 induces the formation of giant multinucleated cells, changes actin and tubulin organization, and most likely promotes cell spreading (Fiorentini et al., 1988). Its toxic activity arises because of the post-translational activating mutation of Rho GTPases by deamidation, an essential control factor in the shape, adhesion, mobility, phagocytosis, and oxidative burst in host cells (Flatau et al., 1997). The CNF-1 prevalence is higher in strains isolated from UTIs and prostatitis than in fecal isolates (Caprioli et al., 1987; Bingen-Bidois et al., 2002). An animal model of UTIs has shown an involvement of CNF-1 in resistance to phagocytosis by macrophages and in the induction of deep and persistent infections of the bladder (Rippere-Lampe et al., 2001). The CNF-1-mediated disruption of the Rho GTPase signaling pathways has antiapoptotic abilities in uroepithelium cells and leads to immune dysregulation, while conferring a survival advantage in the presence of neutrophils (Davis et al., 2005). CNF-1 also plays an important role in the rearrangement of the HBMEC cytoskeleton that allows crossing of the blood-brain barrier (Badger et al., 2000; Kim, 2002).
The pks genomic island present in E. coli strains of phylogroup B2 encodes colibactin, a hybrid polyketide/non-ribosomal peptide that causes DNA damage and cell cycle arrest of eukaryotes (Nougayrède et al., 2006). The colibactin-encoding determinant has been detected primarily in extraintestinal pathogenic isolates of E. coli, other Enterobacteriales, and commensal E. coli (Nougayrède et al., 2006; Putze et al., 2009). E. coli persisting in the infant gut microbiota tends more often to carry the pks island than do either intermediate-term colonizers or transient strains, suggesting that the pks island contributes to the gut-colonizing capacity of group B2 strains (Nowrouzian and Oswald, 2012). The frequent detection of the pks island in E. coli isolated from biopsies of patients suffering from colon cancer also suggests involvement in long-term intestinal colonization, raising the question of its role in colorectal cancer (Swidsinski et al., 1998; Martin et al., 2004; Bronowski et al., 2008; Cuevas-Ramos et al., 2010; Arthur et al., 2012; Buc et al., 2013; Cougnoux et al., 2014, 2016; Pleguezuelos-Manzano et al., 2020). The presence of the pks island in ExPEC could also indicate that colibactin contributes to fitness or virulence during extraintestinal infections (Johnson et al., 2008; Krieger et al., 2011; McCarthy et al., 2015). A recent observation indicates that the probiotic effects of the E. coli Nissle 1917 strain to ameliorate colitis severity and to modulate cytokine expression cannot be separated from the strain’s ability to express functional colibactin (Olier et al., 2012). This finding demonstrates that, depending on the niche or context, colibactin-producing PAI can be considered either a virulence factor and/or a probiotic factor.
The TLRs are an important family of innate sensors that recognize diverse microbial products and launch the signaling pathways that ultimately lead to the clearance of the pathogen from the host and the establishment of a memory response in anticipation of any subsequent attack. ExPEC PAI-serU can interfere directly with the TLR signaling pathway by producing an inhibitor homolog of TLR receptors to dampen the NF-kB-induced proinflammatory response (Cirl et al., 2008). This protein, called the TLR domain containing-protein C (TcpC), is secreted by an efflux pump and then internalized into macrophages, where it can disable TLR signaling through direct binding of MyD88, the key TLR signaling adaptor (Cirl et al., 2008). The tcpC gene is present in 30–40% of the E. coli strains isolated from septicemia and in almost half of those causing pyelonephritis but is less common in cystitis (22%), ABU (16%) and E. coli strains of the fecal microbiota (8%) (Table 1). TcpC can increase the severity of UTIs in humans, which is consistent with the important role of TLR4 in host defense in the urinary tract (Samuelsson et al., 2004).
Autotransporters Encoded by PAIs
Extraintestinal E. coli PAIs encode several autotransporters (ATs), which are a family of proteins that mediate their own secretion through the outer membrane of gram-negative bacteria. Also known as the type V secretion system, ATs contain a C-terminal membrane anchor region that forms a pore through which the passenger domain is translocated to the cell surface. The passenger domain of ATs is exposed at the bacterial surface and/or secreted after autoproteolytic cleavage. The AT passenger domains are diverse in function and can act as enzymes (lipases, esterases, or proteases) and/or as adhesins (Tapader et al., 2019).
AT antigen 43 (Ag43) is widely distributed among E. coli strains, including UPEC. Ag43 mediates autoaggregation, biofilm formation and host cell adhesion. It promotes persistent colonization in a UTI mouse model and is expressed within IBCs, suggesting that Ag43 plays a role in the intracellular growth phase of UPEC (Anderson et al., 2003; Kim, 2003, 2008). Ag43 is also involved in the adhesion of NMEC to HBMECs (Ulett et al., 2007). The ATs UpaB and UpaC and their homologs are found in UPEC and in various strains of E. coli (Allsopp et al., 2012). UpaC promotes adhesion to abiotic surfaces and biofilm formation, while UpaB promotes adhesion to components of the extracellular matrix. In vivo experiments have suggested that UpaB, unlike UpaC, is important for bacterial fitness during UTI. Finally, the UpaG autotransporter promotes UPEC biofilm formation on abiotic surfaces and facilitates binding to extracellular matrix proteins, fibronectin, and laminin (Valle et al., 2008).
Extraintestinal E. coli PAIs also produce serine protease ATs that are designated as SPATEs (serine protease autotransporters of Enterobacteriaceae) such as Tsh (temperature sensitive hemagglutinin), Hbp (hemoglobin binding protein or hemoglobin protease), Sat (secreted autotransporter toxin), Vat (vacuolating autotransporter toxin) and Pic (protein involved in colonization, cf. DEC section). Tsh was initially identified in APEC as a mannose-resistant hemagglutinin that is overexpressed at low temperatures (Provence and Curtiss, 1994). It is observed in more than half of APEC isolates and its prevalence increases in high-lethality isolates (Dozois et al., 2000). The association of Tsh with the virulence of APEC isolates was further reinforced by the detection of fewer and less pronounced lesions in the air sacs of chickens infected with a Tsh mutant than with the wild type strain (Dozois et al., 2000). The tsh gene is also observed in UPEC (4.5%) and NMEC (11.5%) (Ewers et al., 2007). Frequently encoded by plasmids, tsh is also observed in PAIs and promotes adhesion to red blood cells, hemoglobin, and the extracellular matrix proteins fibronectin and collagen IV (Kostakioti and Stathopoulos, 2004). Tsh only differs from Hbp by two amino acid residues in the passenger domain and probably shares functional similarities regarding the breakdown of hemoglobin factor V and mucin (Dautin, 2010; Tapader et al., 2019).
The vat gene was also originally identified in an APEC strain as a cytotoxin of chicken embryonic fibroblast cells that contributes to avian cellulitis infection (Parreira and Gyles, 2003). It only shares 78% identity with Tsh and has different proteolytic activities (Parham et al., 2005b). However, Tsh/Hbp, Vat and Pic have mucinolytic activity and enhance epithelium colonization (Dautin, 2010; Gibold et al., 2016; Tapader et al., 2019). Pic and Tsh/Hbp also target the major human leukocyte-adhesion molecules CD43, CD44, CD45, and CD93, thereby deregulating leukocyte migration and inflammation (Ruiz-Perez et al., 2011). The Sat ATs induce vacuolation of renal cells, and the serine-protease activity is required for the cytopathic effects of Sat (Guyer et al., 2002; Maroncle et al., 2006). Mention has been made of the role of Sat in breaking down the glomerular barrier to allow bacteria to pass into the blood (Nataro and Kaper, 1998). Three new SPATEs—Sha (Serine-protease hemagglutinin autotransporter), TagB and TagC (tandem autotransporter genes B and C)—have also been recently reported in an ExPEC O1:K1 strain isolated from turkeys and they induce cytopathic effects on a bladder epithelial cell line (Habouria et al., 2019). However, their functional role in UTIs remains to be investigated.
SslE/YghJ (secreted and surface associated lipoprotein) is secreted by the type II secretion system and probably anchored at the bacterial surface in ExPEC. It is a metalloprotease domain initially observed in NMEC (Tapader et al., 2019). SslE, which is widely found in both commensal and pathogenic E. coli, has greater prevalence in pathogenic isolates than in commensal isolates, which may also be defective for its secretion (Moriel et al., 2010). SsIE targets mucins and has cytotoxic and proinflammatory activities that can promote the virulence of pathogenic E. coli and sepsis in neonates (Nesta et al., 2014).
Iron Uptake Systems Encoded by PAIs
Iron is essential for bacteria because of its involvement in many metabolic functions, such as the transport of oxygen and electrons. Most iron in humans is complexed with the transport molecules transferrin (found in blood) and lactoferrin (found in the digestive tract and in salivary and pulmonary secretions) and with reserve molecules (ferritin) or it is incorporated into the heme of hemoglobin and myoglobin. This complexation results in iron limitation, which in turn serves as one of the innate defenses against the survival of bacteria within hosts. To use iron from the host, bacteria have developed capture systems that target these iron-complexed forms within citrate ions (fec system) or heme (chu system). The bacteria use transport systems (Sit systems) or produce PK-NRP compounds called siderophores that capture iron from host transporters or reserve systems and transport it back to the bacteria via specific receptors (Braun, 2003). The siderophore enterobactin, which is found in almost all E. coli, is encoded by the core genome. Thus, Lipocalin-2, which is capable of sequestering enterobactin, prevents its uptake by the bacteria via the FepA receptor (Fischbach et al., 2006). Therefore, ExPEC acquires PAI-encoding iron-uptake systems to thwart this host defense mechanism. The most frequently encountered siderophores are aerobactin, yersiniabactin and salmochelin (Braun, 2003). Salmochelin is a modified enterobactin formed by glycosylation of one of its derivatives, and this modification makes salmochelin insensitive to inhibition by human Lipocalin-2 (Fischbach et al., 2006). The PAI-encoded siderophores salmochelin and yersiniabactin are both produced at significantly higher levels in ExPEC isolated from urine than in rectal isolates and could play a greater role than enterobactin within the human urinary tract (Henderson et al., 2009). In a newborn rat meningitis model, salmochelin plays an important role in maintaining a high level of bacteremia, which is a necessary step for bacterial crossing of the blood-brain barrier (Nègre et al., 2004).
Receptor-mediated uptake of heme is another iron-uptake mechanism encoded by ExPEC PAIs. Two outer membrane heme receptors, ChuA and Hma, have been characterized, and both were required for bacterial optimal fitness in a UTI mouse model (Hagan and Mobley, 2009). ChuA could also be important during IBC formation (Reigstad et al., 2007). The PAI-encoded Sit system transports manganese and ferrous iron into the ExPEC cytoplasm and enhances resistance to oxidative stress. The sit genes are upregulated during murine UTI and are expressed during human UTIs, suggesting that they contribute to urofitness (Snyder et al., 2004; Hagan et al., 2010).
Protectins Encoded by PAIs
Protectins are the bacterial elements that protect bacteria against host weapons, such as antibacterial factors (i.e., defensins, D-serine), serum complement and immune cells. PAI-encoded capsules (K antigen) confer this type of protection (Whitfield, 2006). The resulting homopolymers exposed at the surface of the bacteria can resemble various glycoconjugates found within vertebrate hosts (e.g., sialic acid), such as K1 or K4 capsules, and can contribute to the bacterium’s immune-evasion strategy. The K1 antigen is associated with E. coli strains responsible for invasive infections (Sarff et al., 1975). K1 antigen is critical for the induction of a high degree of bacteremia, is associated with a high resistance to both phagocytosis and serum and is required for bacterial crossing of the blood-meningeal barrier (Opal et al., 1982; Cross et al., 1984; Kim et al., 1992). Other PAI-encoded protective elements are surface proteins such as the Iss (increased serum survival) protein, which has been implicated in serum resistance (Xu et al., 2018).
D-serine is a bacteriostatic amino acid that is found in urine and brain (Cosloy and McFall, 1973; Kumashiro et al., 1995). UPEC and NMEC strains frequently harbor a PAI-encoded dsdCXA locus (Bloom and McFall, 1975; Moritz and Welch, 2006). The dsdCXA locus has been hypothesized to be important for UPEC/NMEC pathogenesis. Accordingly, isogenic strains defective in dsdA have a growth defect in urine relative to dsdA wild-type strains. However, dsdA neither positively nor negatively affected the ability of UPEC strain CFT073 to colonize urinary tract in a mouse model (Hryckowian et al., 2015).
Diarrheagenic Escherichia coli
As already described above, InPEC essentially regroups (i) E. coli strains responsible for diarrhea (DEC) and (ii) AIEC, which are not involved in diarrhea but are involved in inflammatory bowel diseases. Among the DEC, the EPEC and EHEC have undoubtedly received the most attention regarding PAIs. By contrast, little information is available about PAIs in DAEC, except for the presence PAI pic/set in some strains (Czeczulin et al., 1999). Compared to ExPEC, the information about PAIs in DEC is generally scarce.
Physiopathology of DEC
The six recognized categories of DEC are primarily based on clinical symptoms, including the type of diarrhea, related syndromes or bacterial interaction with intestinal epithelium cells, as revealed by histological observation, as well as associated molecular determinants of virulence and pathogenicity (Nataro and Kaper, 1998; Kaper et al., 2004).
ETEC are mainly associated with traveler’s diarrhea but can also appear in weanling diarrhea among children (Nataro and Kaper, 1998). The diarrhea is generally not bloody, but watery, and is sometimes associated with vomiting that ranges from mild to severe purging, similar to cholera. The pathogenicity of ETEC mainly relies on heat-labile (LT) and/or heat-stable (ST) enterotoxins responsible for net secretion of intestinal fluid (Turner et al., 2006a; Sack, 2011). The LT form belongs to the cholera toxin family and is secreted by a Type II secretion system (T2SS) with the contribution of LeoA (labile enterotoxin output) for its efficient secretion (Tauschek et al., 2002; Jobling and Holmes, 2006). Unlike the cholera toxins that are secreted as free soluble proteins, LT associates with the outer membrane vesicles by binding to LPS (Horstman and Kuehn, 2002). Sequence divergences confer LT with the ability to bind different ganglioside receptors that mediate entry into the host cell (Fukuta et al., 1988; Jobling and Holmes, 2006). LT is an ADP-ribosylating toxin that inactivates the GTPase activity of the G protein complex by ADP-ribosylation. This, in turn, leads to prolonged activation of the adenylate cyclase activity and elevated intracellular levels of cAMP and results in electrolyte release and stimulation of intestinal excretion (Spangler, 1992; Kaper et al., 2004; Jobling and Holmes, 2006).
By contrast, ST can be discriminated into type I (ST-I, also called STa) and type II (ST-II, also called STb), which target different receptors (Peterson and Whipp, 1995; Turner et al., 2006a). ST-I binds and activates a guanylate cyclase C receptor and the subsequent increase in intracellular cGMP levels activates the cystic fibrosis transmembrane conductance regulator (CFTR) chloride channel (Vaandrager, 2002; Turner et al., 2006a). ST-II, which binds the acidic glycosphingolipid sulphatide, activates a pertussis toxin-sensitive GTP-binding regulatory protein that ultimately activates CFTR (Peterson and Whipp, 1995; Fujii et al., 1997; Rousset et al., 1998). Surface colonization is an important in step in the physiopathology of ETEC, which involves a large and diverse repertoire of pili, called colonization factor antigens (CFAs), that bind to different receptors at the surface of the host cells (Turner et al., 2006a; Isidean et al., 2011; Ageorges et al., 2020). Additional virulence factors can be present, including some surface-exposed adhesins like TibA or Tia, the toxins ClyA and EAST1, or the protease EatA; however, this does not appear to be a common feature among ETEC (Turner et al., 2006a,b).
Enteropathogenic E. coli infection manifests as acute diarrhea in infants and is often associated with vomiting (Nataro and Kaper, 1998; Kaper et al., 2004). A hallmark of EPEC is its attaching and effacing (A/E) histopathology. This is accompanied by intimate attachment of bacterial cells expressing cell-surface intimin (Eae) with the cognate translocated intimin receptor (Tir) expressed on the surface of eukaryotic cell following injection into their cytosol by a Type III, subtype a, secretion system (T3aSS) (Nougayrède et al., 2003; Desvaux et al., 2006; Lai et al., 2013). A large repertoire of T3aSS effectors participate in the infection process (Dean et al., 2005; Dean and Kenny, 2009). However, and despite decades of investigations, the exact molecular mechanisms by which EPEC induce diarrhea are not well understood (Ochoa and Contreras, 2011).
The presence or absence of plasmid pEAF (E. coli adhesion factor) encoding bundle-forming pili (BFP), can be used to further categorize EPEC into typical (tEPEC) and atypical EPEC (aEPEC), respectively (Trabulsi et al., 2002; Hernandes et al., 2009; Hu and Torres, 2015). In tEPEC, BFP are type 4 pili (T4P) encoded by the bfp operon and induce a localized adhesion (LA) pattern at the surface of intestinal epithelial cells to form microcolonies (Scaletsky et al., 1984; Ageorges et al., 2020). In aEPEC, no localized LA patterns are observed but instead localized-adhesion-like (LAL) or aggregative adhesion (AA) patterns form loose bacterial cell clusters involving alternative adhesion factors such as EspA filaments, LifA (lymphocyte inhibitory factor) or ECP (E. coli common pili) (Rodrigues et al., 1996; Trabulsi et al., 2002; Ageorges et al., 2020).
EHEC are responsible for pediatric diseases ranging from watery diarrhea to hemorrhagic colitis that can further lead to thrombotic microangiopathies, especially hemolytic and uremic syndrome (HUS) and thrombotic thrombocytopenic purpura (TTP) (Karmali et al., 1983; Ruggenenti and Remuzzi, 1998; Tarr et al., 2005; Tarr, 2009; Wada et al., 2014). EHEC are primarily characterized by the expression of the key virulence shigatoxin (Stx) that binds to glycosphingolipid Gb3 before penetrating the cell and inducing apoptosis of kidney epithelial cells (Melton-Celsa, 2014). Nonetheless, and somewhat surprisingly, the way that Stx crosses the intestinal mucosa is not fully elucidated. While tEHEC possess the locus of enterocyte effacement (LEE) as in any EPEC, the aEHEC do not express the injectisome and instead rely on alternative monomeric and multimeric surface colonization factors, such as adhesins and pili (McWilliams and Torres, 2014; Monteiro et al., 2016; Ageorges et al., 2020). Similar to EPEC, however, the precise molecular mechanisms that induce bloody diarrhea remain elusive (Croxen et al., 2013; Stevens and Frankel, 2014).
Of note, and contrary to EPEC, A/E lesions are never detected on colonic biopsies from EHEC infection (Nataro and Kaper, 1998), although they are observed in vitro using epithelial cell culture or human colonic explants (Golan et al., 2011; Battle et al., 2014; Lewis et al., 2015). Some E. coli isolated from other sources (environment, animals, food and the agri-food chain) can be genotypically characterized as possessing the stx gene and can be regrouped as shigatoxin-encoding E. coli (STEC, or shigatoxin-producing E. coli when Stx production has been experimentally confirmed). The pathogenicity of EHEC has been clinically ascertained, and STEC can have very different virulence levels, up to hyper-virulence; alternatively, they can even be potentially avirulent and are not systematically and rigorously considered as pathogenic (Karmali et al., 2003; Caprioli et al., 2005; Laing et al., 2009; Monteiro et al., 2016). With more than 400 distinct serotypes revealed in STEC, only a handful of EHEC serotypes has been clinically associated with epidemic outbreaks, although they can all be potentially involved in human infections (Karmali et al., 2003; Blanco et al., 2004; Cristancho et al., 2008; Mathusa et al., 2010). The search for genetic markers has been the Grail for decades; nevertheless, the differences in the virulence level is most certainly associated with various compensatory combinations of molecular determinants, including PAIs, together with regulatory networks that can act at various levels and not just regarding the transcriptional aspects of genetic expression (Ageorges et al., 2020).
After ETEC, EAEC is the second most common cause of tourista that causes acute and sometimes persistent diarrhea (Jenkins, 2018). The diarrhea is typically non-bloody and without inflammation or emetic symptoms. Upon adhesion onto intestinal epithelial cells, EAEC exhibit a typical stacked brick-like pattern known as aggregative adhesion (Kaper et al., 2004). EAEC form a loose biofilm at the mucosal surface involving pili and especially AAF (aggregative adhesion fimbriae, encoded by a large plasmid pAA) (Kaper et al., 2004; Ageorges et al., 2020). Additional virulence factors include the pAA-encoded enterotoxins EAST1 and Pet (plasmid-encoded toxin) and the chromosome-encoded ShET1 (Shigella enterotoxin 1) and Pic (protein involved in intestinal colonization) (Chaudhuri et al., 2010; Jenkins, 2018). However, the molecular basis that mediates diarrhea upon infection by EAEC remains to be elucidated.
Extensive and compelling evidence indicates that EIEC and Shigella cannot be discriminated from a taxonomic point of view as they both belong to the same species, E. coli, and basically correspond to the same pathotype (Lan et al., 2004; Peng et al., 2009; Chaudhuri and Henderson, 2012; Pettengill et al., 2015; Michelacci et al., 2016). Differences in the virulence level have sometimes been put forward, with Shigella considered hyper-virulent relative to EIEC, which generally induces less severe disease. Nevertheless, significant exceptions do exist and the intestinal illness is in fact indistinguishable (Parsot, 2005; Michelacci et al., 2016; Belotserkovsky and Sansonetti, 2018; Hendriks et al., 2020). As with EHEC/STEC, the search for genetic markers that could solely explain the difference in the virulence levels might have somehow hindered the potential in the heterogeneity of the regulation of the genetic expression from one EIEC/Shigella strain to another as well as between individual cells in an isogenic population. The hallmarks of EIEC/Shigella are the invasion plasmid pINV, which encodes a T3aSS (like in EPEC and EHEC), and cognate effectors of key importance in host invasion of this intracellular pathogen, which have been extensively investigated (Zagaglia et al., 1991; Lan et al., 2001, 2003; Lan and Reeves, 2002; Schnupf and Sansonetti, 2019). Additional virulence factors include the mucinase Pic (previously called ShMu for Shigella haemagglutin and mucinase), also found in EAEC and contributing to the initial step of intestinal colonization (Henderson et al., 1999; Parham et al., 2004). Another is the protease SigA (Shigella immunoglobulin A protease) that contributes to the watery prodromal phase of the infection (Al-Hasani et al., 2000; Chua et al., 2015), together with the enterotoxins ShET1 and ShET2 (Henderson et al., 1999). The exact function and mode of action of the latter enterotoxins remain to be determined (Mattock and Blocker, 2017; Belotserkovsky and Sansonetti, 2018).
DAEC, rather than DEC, are essentially involved in diarrhea in young children (Servin, 2005, 2014), and some DAEC are ExPEC involved in urinary tract infections. EIEC are known to preferentially colonize the colon, as well as the distal ileum for EHEC, whereas the ETEC and EPEC colonize the small intestine, as well as the colon for EAEC (Clements et al., 2012). By contrast, the primary site of intestinal colonization of DAEC has not been determined (Servin, 2014). DAEC exhibits a scattered adhesion pattern on intestinal epithelial cells, called diffuse adhesion (Kaper et al., 2004; Croxen et al., 2013). DAEC have previously been subdivided into those expressing and those not expressing Afa/Dr (afimbrial adhesin/decay-accelerating factor receptor) adhesins (Servin, 2005), but the latter subclass is now considered to belong to aEPEC, leaving Afa/Dr adhesins as a hallmark of DAEC (Servin, 2014). Previously, daaE and/or daaC were briefly suggested as biomarkers of DAEC, but F1845 pili are rare among DAEC strains (Campos et al., 1999). The group of Afa/Dr adhesins is quite diverse and can induce rearrangement of the cytoskeleton upon binding to the eukaryotic cell and can even promote bacterial internalization (Servin, 2005; Le Bouguénec and Servin, 2006). Additional virulence factors include pks island products (such as colibactin), flagella and the secreted autotransporter toxin (Sat) (Servin, 2014). Notably, while some DAEC strains have been sequenced (Lindsey et al., 2018; Tang et al., 2019), no complete assembled genomes are as yet available. In addition, contrary to EHEC, ETEC, ETEC or EAEC with E. coli strains EDL933, H10407, E2348/69 or O42, respectively (Iguchi et al., 2009; Chaudhuri et al., 2010; Crossman et al., 2010; Latif et al., 2014), no prototypical DAEC strains have been sequenced to date, which limits our understanding of PAIs within this DEC pathotype.
PAI-Encoded Virulence Factors of DEC
While PAIs were originally described in ExPEC, they later appeared to be present in InPEC. To date, 13 PAIs have been characterized, some of which are present in different DEC pathotypes (Figure 4; Table 3).
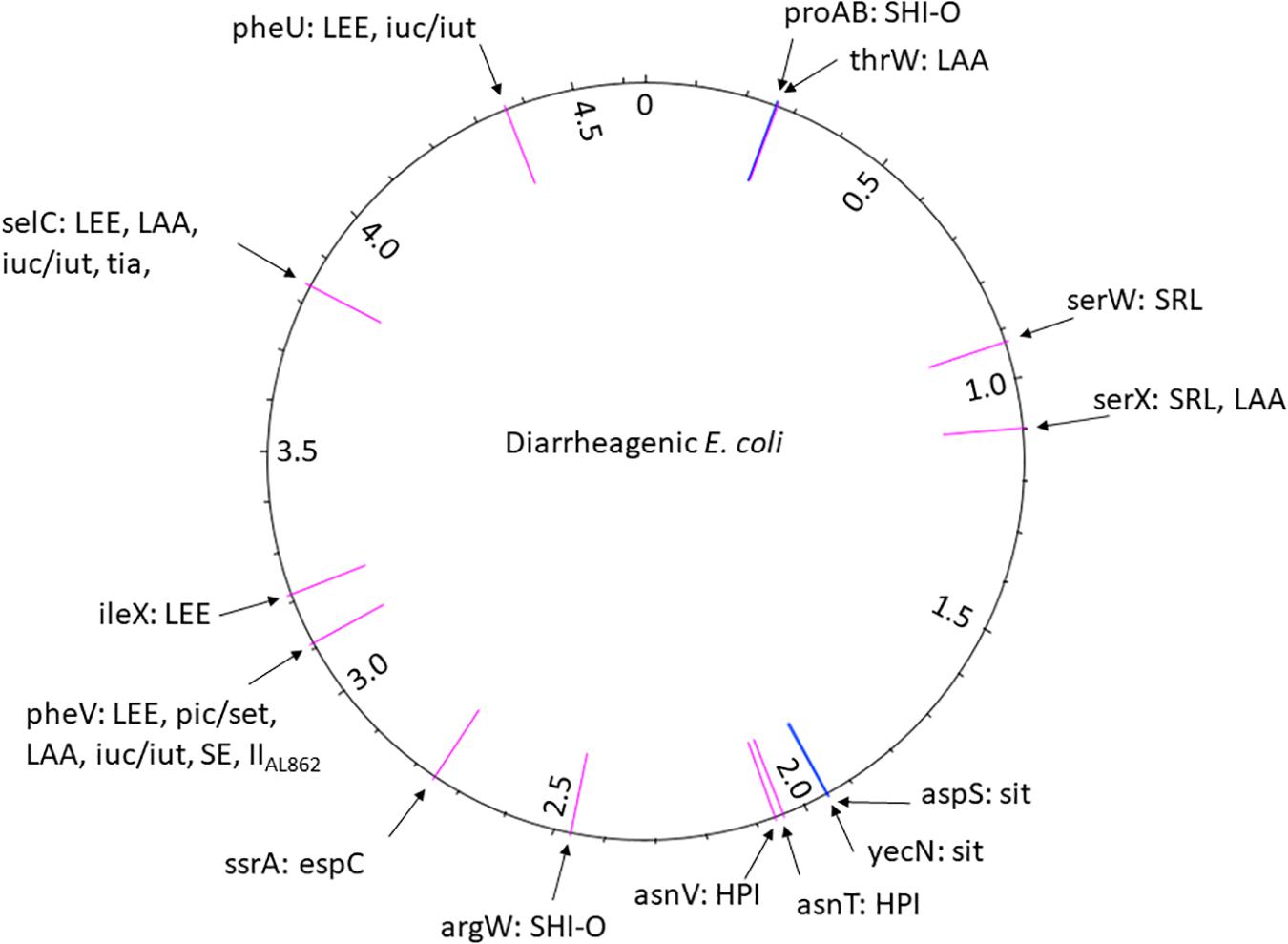
Figure 4. The position of PAI insertion sites in the diarrheagenic E. coli pathotypes. The name of the genetic features associated with the insertion is indicated with the name of PAIs found at the corresponding site. The position of the insertion site is indicated in pink for tRNA genes and in blue for coding genes. The numbers reported in the inner ring are the positions (megabase) in the genome of the non-pathogenic E. coli strain MG1565 used as a reference.
LEE PAI
The LEE is undoubtedly the most investigated PAI in DEC, and readers are referred to recent reviews solely dedicated to this PAI for detailed information (Kirsch et al., 2004; Parham et al., 2005a; Schmidt, 2010; Stevens and Frankel, 2014; Connolly et al., 2015; Franzin and Sircili, 2015; Kendall, 2016; Furniss and Clements, 2018; Turner et al., 2019). The presence of the LEE is the hallmark of EPEC, but it is also found in tEHEC (McDaniel et al., 1995; McDaniel and Kaper, 1997). As first described in prototypical EPEC strain E2348/69 (Perna et al., 1998; Iguchi et al., 2009) and EHEC strain EDL933 (or strain Sakai) (Hayashi et al., 2001; Perna et al., 2001; Latif et al., 2014), the core region of the PAI consists of 41 CDS, organized into (i) 5 operons named LEE-1 to LEE-5 encoding transcriptional regulators, including the master LEE-encoded regulator (Ler), chaperones and structural components of the T3aSS, as well as some effectors, (ii) one grlAB bicistron encoding transcriptional regulators, and (iii) some monocistrons. The T3aSS constitutes a major virulence factor that allows the assembly of a cell-surface needle structure, the injectisome which enables the direct transport of virulence effectors into a host cell (Cornelis, 2006; Desvaux et al., 2006; Brutinel and Yahr, 2008; Mueller et al., 2008; Diepold and Wagner, 2014, 2014; Platenkamp and Mellies, 2018; Lara-Tejero and Galán, 2019; Ageorges et al., 2020). The regulation and function of the T3aSS has been extensively reviewed and readers are invited to consult the above reviews for further details on this vast field of research. Apart from the LEE core region, a certain degree of variability exists between LEEs found in different EPEC or EHEC strains, with estimated sizes ranging from approximately 35 to 110 kb (Sperandio et al., 1998; Jores et al., 2005; Müller et al., 2009). The LEE core region can be completed by prophage genes, as in EHEC ELD933 or Sakai, whereas the fusion with OI-43/48 SpLE1 (Sakai prophage-like element 1) containing a ter operon conferring resistance to tellurite (Tarr et al., 2000; Hayashi et al., 2001). The OI-122 SplE3, which contains a lifA (efa1) region that encodes the virulence factor lymphostatin (Klapproth et al., 2000; Badea et al., 2003; Morabito et al., 2003) or some truncated versions of these islands, can also be found in some variants of the LEE PAI (Gärtner and Schmidt, 2004; Kirsch et al., 2004; Müller et al., 2009). The LEE PAI is inserted at the selC tRNA gene, but the pheV and pheU tRNA genes were also reported as alternative insertion sites for the LEE among these variants (Rumer et al., 2003; Bertin et al., 2004). Phylogenetic analyses based on selected genes indicated that the LEE core region could be discriminated into two main core clusters, one harboring eae-α and -γ and the other one harboring eae-β and -ε independently from the tRNA insertion site (Jores et al., 2004). Nonetheless, the current knowledge on the distribution of the LEE indicates complex events of genetic acquisitions and recombinational rearrangements in E. coli genomes and the evolutionary network remains quite incomplete (Jores et al., 2004; Rasko et al., 2008; Montero et al., 2019).
PAI pic/set
PAI pic/set is inserted into the pheV gene and was identified in EAEC, as well as in EIEC/Shigella (called SHI-1 PAI, previously also known as She) and UPEC (called PAI IICFT073), and in some EHEC and EPEC strains; however, they differ in size, organization, gene composition and genome localization (Al-Hasani et al., 2001a,b; Behrens et al., 2002; Parham et al., 2005a). Island probing revealed this PAI to be quite unstable and to undergo spontaneous excision from the chromosome at a frequency of 10–5–10–6 (Rajakumar et al., 1997). The number of IS-like element flanking the gene (especially IS629 and IS911 also found around other genes encoding SPATEs) suggested that pic (previously called she) is part of a mobile element that could move independently of the PAI (Henderson et al., 1998, 1999; Parham et al., 2005a).
The setB and setA genes, contained within the pic gene but on the complementary strand, encode the oligomeric enterotoxin ShET1 (Fasano et al., 1995; Henderson et al., 1999; Behrens et al., 2002). The transcription of setB was induced in simulated human intestinal microbial systems, although the exact signals promoting gene expression remain to be identified (Behrens et al., 2002). The genetic expression of the pic/set locus is quite unusual and complex. At least three promoters, Ppic1, 2, and 3, could direct the transcription of pic but with different forces and constitutive/inducible expression (Behrens et al., 2002). A PsetA promoter was identified just upstream of setA, whereas a PsetB was identified 1.5 kb upstream of setB with a silencer DRE (downstream regulatory element) region in between that repressed the expression of setBA without any effect on pic transcription (Behrens et al., 2002). Apart from regulation by its own promoter, setA also appears to be expressed by transcription readthrough from setB.
LAA PAI
The locus of adhesion and autoaggregation (LAA) is a composite 86-kb PAI composed of four modules (Montero et al., 2017). In addition to the host immune response suppressor SisA (shiA-like inflammation suppressor genes A) (Lloyd et al., 2009b), module I encodes an autoaggregative factor with Hes (hemagglutinin from STEC), a member of the Hra (heat-resistant agglutinin) family (Montero et al., 2014), together with module IV encoding Ag43, a member of the SAAT (self-associating autotransporter). Module III encodes the adhesin Iha (iron regulated gene homolog adhesin) and the SPATE LesP (LAA encoded SPATE), while module IV actually corresponds to the PAI previously known as PAI ICL3 (Shen et al., 2004).
PAI ICL3 was originally described is a hybrid genomic region that entered B1 E. coli genomes on multiple and independent occasions (Shen et al., 2004). It is composed of segments of the EHEC EDL933 OI-48 at both its extremities and two genomic segments, GS-I and GS-II. GS-I comprises fragments of the Z1640 gene encoding the ShlB transporter family of the T2bSS, whereas GS-II is a composite region encoding a member of the ShlA exoprotein family secreted by the T5bSS. GS-II encodes several transposase genes, genes originating from EHEC EDL933 OI-122, including pagC (phoP-activated gene C), and an additional fragment of Z1640 (Shen et al., 2004; Girardeau et al., 2009). Different variants and often deleted versions of PAI ICL3 were reported as carried by genomic islands, including GIpheV-CRICC168 from Citrobacter rodentium, inserted at pheV, selC or serW tRNA (Girardeau et al., 2009). However, the mosaic structure of LAA PAI was not understood at the time, and these GIs represent complete and incomplete LAA PAI variants (i.e., comprising less or all of the four modules) belonging to two major lineages (Montero et al., 2017). In agreement with PAI ICL3 investigations (Girardeau et al., 2009; Hauser et al., 2013), the LAA PAI appeared to be exclusively present in aEHEC in the first instance and could thus complement the absence of the LEE PAI (Colello et al., 2018, 2019).
PAI iuc/iut
The SHI-2 (Shigella pathogenicity island 2) PAI is inserted at the selC tRNA gene and encodes an integrase almost identical to its ortholog in the LEE PAI. It possesses an abundant number of partial transposases and IS elements, which are remnants of the multiple stepwise assembly of the island (Moss et al., 1999; Vokes et al., 1999). SHI-2 codes the iucABCD operon for synthesis of aerobactin (Lawlor and Payne, 1984), a hydroxamate siderophore involved in iron uptake (Vokes et al., 1999; Braun, 2003), as well as the iutA gene encoding the outer membrane receptor for aerobactin complexed to iron (Crosa, 1989). In Shigella boydi, a 21-kb PAI harboring the iucABCD-iutA operon but inserted at the pheU tRNA gene and possessing LEE prophage genes absent from SHI-2, was called SHI-3 (Purdy and Payne, 2001; Nie et al., 2006). Nonetheless, SHI-3 remains very closely related to the 30-kb SHI-2 in S. flexneri 2a SA100 (Vokes et al., 1999) and 23.8-kb SHI-2 in S. flexneri 5a M90T (Moss et al., 1999), and it belongs to the same family of PAI.
The iut/iuc operon is highly conserved in SHI-2 and SHI-3 and can be inserted in at different loci, especially in paralogs to phe tRNA genes (at least pheV, pheR and pheU) (Al-Hasani et al., 2001a; Nie et al., 2006). The SHI-2/3 PAIs are regrouped under the designation of PAI iuc/iut to rationalize the naming in a way similar to that of PAI pic/set. The PAI iuc/iut would have been acquired horizontally, as suggested by its high degree of conservation with the aerobactin operon harbored on plasmid pColV in some E. coli strains (Moss et al., 1999). SHI-2 also harbors several genes encoding the predicted cytoplasmic proteins, including ShiA (a putative quinone reductase) that has been demonstrated to attenuate inflammation by abrogating the innate T-cell response (Moss et al., 1999; Ingersoll et al., 2003; Ingersoll and Zychlinsky, 2006). SHI-2 also encodes potential integral inner membrane proteins, including ShiF (a putative transporter of the major facilitator superfamily), a dispensable auxiliary factor involved in aerobactin export and/or synthesis (Moss et al., 1999; Genuini et al., 2019). Like pic/set in SHI-1, the shiF and shiG genes overlap and would be transcribed in reverse orientation on a complementary strand, although experimental investigations of genetic regulation and function of ShiG are still required (Moss et al., 1999).
Importantly, the imm (immunity) gene (shiD) appears responsible for immunity to different colicins, including colicin V (ColV) (Vokes et al., 1999). Colicins are proteins produced by E. coli that cause cell lysis of sensitive strains (Cascales et al., 2007). In some strains, PAI iuc/iut is inverted and is thus expressed from the complementary strand, but it is also differently localized and orientated with respect to the oriC (Nie et al., 2006), which could have consequences on the expression of the aerobactin genes (Schmid and Roth, 1987; Eisen et al., 2000; Kothapalli et al., 2005). Among the EIEC/Shigella, the size of PAI iuc/iut clearly differs, but further investigations are needed to determine their diversity and to gain insight into their gene content, organization, phylogeny and distribution in E. coli genomes (Moss et al., 1999; Vokes et al., 1999; Yang et al., 2005).
PAI tia
The prototyptical ETEC strain H10407 contains 25 identified ROD (regions of difference) on the chromosome, two of which are currently viewed as PAIs with the tia (toxigenic invasion locus A) and tibA (toxigenic invasion locus b) loci (Elsinghorst and Weitz, 1994; Crossman et al., 2010). Tia is encoded in a large PAI of approximately 46 kb (corresponding to ROD-20), inserted at selC and flanked by direct repeat sequences (DRSs) identical to those found in PAI-1 in UPEC (Fleckenstein et al., 1996, 2000; Crossman et al., 2010). Tia can act both as an invasin, contributing to the invasion of intestinal epithelium cells (Bondì et al., 2017), and as an adhesin, binding to heparin-sulfate proteoglycans (Mammarappallil and Elsinghorst, 2000; Fleckenstein et al., 2002; Turner et al., 2006b). In addition to int, PAI tia encodes LeoA, an accessory factor for efficient LT secretion, as well as three additional CDS encoding a putative transport system (Fleckenstein et al., 2000). DNA hybridization studies indicate that PAI tia is present in numerous ETECs, as well as in some EPEC and EHEC (Fleckenstein et al., 2000). Nonetheless, the hybridization and multiplex PCR patterns exhibit a considerable level of heterogeneity, suggesting differences in the organization and content of the PAI. The spread of this PAI among DEC and broadly among E. coli strains requires further in-depth investigations (Swenson et al., 1996; Fleckenstein et al., 2000).
PAI tibA
In ETECs, TibA is encoded on a 21.6-kb PAI of low G + C content, corresponding to ROD-13 (Elsinghorst and Weitz, 1994; Crossman et al., 2010). Besides the tibDBCA operon, the PAI tibA codes 14 transposases and IS66, but the insertion site remain elusive. TibA belongs to the SAAT family, is secreted by the T5aSS and is glycosylated by the heptosyltransferase TibC (Masuda et al., 1983; Turner et al., 2006b). Besides cell aggregation and biofilm formation, this glycosylated surface protein is involved in adhesion to intestinal epithelial cells (Lindenthal and Elsinghorst, 1999). The functions of TibD and TibB are yet to be determined. The prevalence and distribution of PAI tibA among E. coli remains to be investigated, though it is not systematically present in all ETEC strains (Elsinghorst and Weitz, 1994; Crossman et al., 2010).
SE PAI
LEE-negative EHEC (i.e., aEHEC) contain an 8-kb PAI coding a subAB2 operon, expressing a subtilase (Michelacci et al., 2013). The subAB1 allelic variant is located on a plasmid, but no additional DNA regions corresponding to the PAI were identified, suggesting that it was not horizontally acquired from this plasmid. SubAB is a well-known cytotoxin inducing apoptosis in LEE-negative EHEC (Wang et al., 2007; Wolfson et al., 2008; Yahiro et al., 2012). This so-called SE (subtilase encoding) PAI also harbors genes encoding an integrase and a sulfate, as well as Tia and ShiA, and is inserted in the pheV tRNA gene. The presence of tia, as in PAI tia, and shiA, as in PAI pic/set, as well as the presence of subAB2 in some ETEC strains (Michelacci et al., 2013), raises questions about the origin and evolution of the SE PAI with respect to these PAIs and demands further phylogenetic analyses. The SE and LEE PAIs have the same insertion site, which led to the hypothesis that mutual exclusion events could have occurred between these PAIs competing for integration at pheV tRNA gene and could further explain the strong association of the subAB2 operon with aEHEC versus tEHEC (Orden et al., 2011; Michelacci et al., 2013). As with PAI iuc/iut, however, insertion at homologous phe tRNA genes cannot be excluded. In general, the prevalence of SE PAI in DEC, and in other E. coli, requires further in-depth investigations.
SHI-O PAI
The SHI-O PAI harbors genes involved in serotype conversion in Shigella by modification of the structure of the O-antigens (Allison and Verma, 2000; Ingersoll et al., 2002; Torres, 2004). SHI-O would appear to be a remnant of an ancient lysogenic bacteriophage that lost its ability to enter into a lytic cycle and underwent excision upon deletions and mutations of sensitive key regions. This PAI codes three genes, gtrA, gtrB and gtrV, that express the glycosyltransferases that allow differential glycosylation of bacterial lipopolysaccharide (LPS) and thus antigenic conversion (Huan et al., 1997; Adams et al., 2001; Sun et al., 2013). SHI-O was also shown to be inverted and to differ in its composition between Shigella strains (Nie et al., 2006) and to have a different insertion in E. coli (Allison and Verma, 2000). Besides the generation of a large variety of shigella serotypes, the modification of O antigens by SHI-O contributes to the modulation of the virulence level through different abilities to evade the host immune response (Robbins et al., 1992; Hong and Payne, 1997; Van den Bosch et al., 1997). The prevalence and distribution of SHI-O in E. coli has not been questioned as yet, although it is present in prototypical EAEC O42 (Chaudhuri et al., 2010).
SRL PAI
The SRL (shigella resistance locus) PAI is a 66-kb element containing at least 59 CDS, especially a 16-kb cluster designated as SLR with genes conferring resistance to streptomycin (aadA1), ampicillin (oxa-1), chloramphenicol (cat) and tetracycline (tetABCD-R) (Rajakumar et al., 1996; Turner et al., 2001, 2003). The SRL PAI is a mobile element flanked by short 14-bp DRSs, which can excise from its insertion site at tRNA gene serX (Turner et al., 2001). The deletion rate was estimated at approximately 10–5 and led to antibiotic-sensitive variants. The int (integrase) gene was required for precision deletion of the island through site-specific recombination. Alternative insertion sites are strongly suspected, such as serW, paralogous to serX, and orf58 (Turner et al., 2003). Besides antibiotic resistance, SRL PAI encodes a functional ferric dicitrate iron transport system (fecABCDE-I-R), which expands the battery of iron acquisition mechanisms in the bacterial strain (Luck et al., 2001). Functional genetic analysis revealed that SRL PAI encoded a functional aspartate racemase converting D- to L-aspartate (AspR) and a cognate transporter (DcuA) enabling catabolism of D-asparate as a sole carbon source (Henríquez et al., 2020). Catabolism of D-amino acids, such as D-aspartate, as a carbon source and/or detoxification of their toxic effects on protein biosynthesis could have an important role in the colonization of the human gastrointestinal tract, an aspect which undoubtedly requires further in-depth investigations. The aspR and dcuA homologs are present in some EHEC O104:H4 isolates (Henríquez et al., 2020), but the broad prevalence of SLR PAI in E. coli needs thorough investigation, although this PAI seems quite prevalent in multiresistant Shigella isolates (Turner et al., 2003).
PAI espC
EspC (E. coli secreted protein C) is a SPATE with enterotoxic activity that induces necrosis and apoptosis in epithelial cells (Mellies et al., 2001; Navarro-Garcia et al., 2014; Serapio-Palacios and Navarro-Garcia, 2016). EspC actually cleaves the cytoskeletal actin-associated protein α-fodrin, the focal adhesion protein paxillin and a focal adhesion kinase (FAK). In EPEC, EspC is encoded in a 15.2-kb PAI inserted between orf360 and tRNA ssrA genes, with respect to the E. coli K12 chromosome (Mellies et al., 2001). Besides five putative transposases, PAI espC encodes an integrase and a homolog to the virulence effector VirA from S. flexneri that is involved in cellular invasion and intracellular spreading (Campbell-Valois et al., 2015; Agaisse, 2016), as well as additional CDS with low sequence similarities that require further analysis to ascertain their function. The low G + C content of this PAI suggests it was acquired horizontally. PAI espC seems present in other DEC, including some EPEC and EHEC, but additional studies are needed to characterize the possible diversity in the genetic organization, prevalence and distribution (Mellies et al., 2001).
PAI sit
Originally described in S. flexneri, the PAI sit primarily harbors a sitABCD locus encoding a ferrous iron and manganese uptake system belonging to the ABC transporter family, homologous to Sit (salmonella iron transport) (Kehres et al., 2002; Runyen-Janecky et al., 2006; Fisher et al., 2009). The PAI sit is found in some commensal and pathogenic E. coli at various chromosomic locations, with insertion sites that fall into four clusters (Fisher et al., 2009). The size and orientation of the island seem to differ considerably, but further studies are required to assess the degree of variation in the gene content of the PAI sit (Chen and Schneider, 2006; Fisher et al., 2009). This PAI has multiple chromosomic insertion sites and a large number of genetic rearrangements and deletion and insertion sequences that would occur through homologous recombination rather than site-specific mechanisms. Therefore, this PAI appears quite unstable (Fisher et al., 2009).
HPI
The HPI (high pathogenicity island) is a PAI originally identified in Yersinia enterocolitica (Schubert et al., 1998; Carniel, 2001). It later appeared widely disseminated in Enterobacteriaceae, including DEC, but essentially in EAEC, as it is rarely present in EIEC/Shigella, EPEC, ETEC or EHEC (Czeczulin et al., 1999; Karch et al., 1999; Schubert et al., 2000). The HPI encodes an iron uptake system based on the siderophore yersioniabactin (Ybt) (Schubert et al., 2004b), which was demonstrated to be functional in several EAEC strains and contributes to the virulence (Carniel, 2001; Hu et al., 2005). While fyuA (also called psn) and ybtPQ (also called irp6-7) encode an outer-membrane receptor and an inner-membrane transporter for iron uptake, respectively, irp1-5 and ybtS (irp9) are involved in the biosynthesis of Ybt (Carniel, 2001). In E. coli, the HPI is approximately 40 kb and is generally inserted at the asnT tRNA gene (Buchrieser et al., 1998; Schubert et al., 2004b). However, it can also be inserted at the asnV tRNA gene harbored in place of IS600, an additional 34.4-kb fragment constituting a functional ICE encoding a T4SS (Schubert et al., 2004a). This element could constitute the progenitor and missing link that would explain the dissemination of HPI among E. coli.
PAI IIAL862
Escherichia coli AL862 was primarily isolated from a septic patient with a cancer, but the PAI containing the afa-8 operon, and further called PAI IIAL862, appeared as a preferential insertion into the pheV gene in several human and bovine pathogenic E. coli strains (Lalioui and Le Bouguénec, 2001; Redford and Welch, 2002). The afa-8 operon, encoding the Afa/Dr adhesin AfaEVIII (Lalioui et al., 1999; Lalioui and Le Bouguénec, 2001), is expressed in some E. coli strains isolated from animals with diarrhea, including calves, pigs, and poultry (Gérardin et al., 2000). PAI IIAL862 can be identified in some human intestinal E. coli isolates but evidence of its implication in diarrhea in humans remains to be demonstrated (Le Bouguénec et al., 2001; Girardeau et al., 2003). This PAI is supposedly present in some DAEC, but investigations are still required (Servin, 2014). Among the four sRNAs encoded in PAI IIAL862, the thermoregulated sRNA AfaR further regulates the expression of the AfaD-VIII subunit. PAI IIAL862 also includes the deoK operon, which confers the ability to use deoxyribose as a carbon source and would increase competitiveness with respect to host infectivity (Lalioui and Le Bouguénec, 2001).
Conclusion
As reviewed here above, PAIs are widespread in E. coli and are major actors in the genome plasticity of this bacterium. The PAIs have shaped the genome of this bacterial species, which further explains the emergence of different pathotypes that result in different intestinal and extraintestinal diseases. The transfer of these GIs in a one-step acquisition of the entire functional determinants, thereby enabling bacterial evolution in quantum leaps, opens the bacterium to new ecological niches. The functional elements encoded by PAIs include pathogenicity effectors, but they also contain regulatory elements involved in crosstalk between PAIs and the genetic background. This crosstalk may be key factors in genome evolution, including PAIs, and probably result in a diversity of expression profiles. This requires more investigations to obtain the best understanding of E. coli virulence and the apparent redundancy of pathogenicity factors in ExPEC.
As exemplified by the LEE and LAA PAIs, these genomic islands are also quite plastic in terms of their insertion or deletion events, resulting in PAIs of different sizes in different strains but with a common evolutionary history. This review has further stressed that, in some cases, further investigations are still required to fill the existing knowledge gaps, for example, about the phylogenetic relationship between SE PA, PAI tia, and PAI pic/set (Michelacci et al., 2013), which may belong to the same family as was later identified for PAI ICL3 and PAI LAA (Montero et al., 2017). However, the PAI nomenclature is quite erratic and requires a more rigorous formulization to avoid different naming of homologous genomic islands, such as SH-2 and SH-3, found in different strains, as was done for HPI. This is necessary to facilitate PAI tracing and our understanding of their phylogeny and evolution.
Additional PAIs are most certainly present in E. coli; examples are the genomic islands OI-57 (Coombes et al., 2008; Imamovic et al., 2010), OI-122 (Karmali et al., 2003; Wickham et al., 2006; Konczy et al., 2008), OI-43/48 (Taylor et al., 2002; Yin et al., 2009; Ju et al., 2013) and GI-selC17584/1 (Schmidt et al., 2001; Saile et al., 2018) in EHEC or some ROD in ETEC (Crossman et al., 2010). However, beyond a correlation of their association with outbreaks or severe diseases, experimental confirmation is still required to demonstrate their involvement and exact role in E. coli virulence and pathogenicity.
The evolution of the pathogenesis of E. coli constitutes a challenge for public health authorities in both developed and developing countries. This is especially evident given the burden of disease and the emergence of new epidemic strains, as dramatically exemplified by the hybrid diarrheagenic EHEC/EAEC O104:H4 strain (Navarro-Garcia, 2014; Prager et al., 2014; Kampmeier et al., 2018). The efficient horizontal gene transfer and high genetic plasticity of the E. coli genome, at both the plasmidic and chromosomic levels, represent potential public health issues that require a full comprehension and determination of the molecular mechanisms at play in the emergence of new E. coli pathotypes and/or E. coli strains with higher virulence levels. The description of numerous E. coli pathotypes with respect to intestinal and extraintestinal pathogenic diseases should not hide the fact that E. coli is primarily a normal and harmless commensal of the human intestinal microbiota, and that all these strains belong to the very same and unique bacterial species, E. coli (including Shigella).
To avoid biases in our understanding of E. coli physiology when solely focusing on anthropocentric medical and pathogenic aspects, we must consider not only pathogenic human and animal isolates but also the commensal strains that occur all along the food chains. Very diverse selection pressures can be encountered in the trophic network and can work together to drive evolution. In the various ecosystems that E. coli calls home (e.g., animal and human GIT, food matrices and soil, water, agri-food environments), the colibiote consists of different commensal and/or pathogenic E. coli strains co-existing and interacting along with the rest of the microbiota of the biocoenoses in the various environmental conditions of the biotopes. E. coli is not intentionally pathogenic, as suggested by its pathoadaptation (Maurelli, 2007; Rahman and Gadjeva, 2014; Pasqua et al., 2017). However, ecocentric and biocentric views allow the perception that these different levels of interactions can induce evolution and selection of genetic traits for specific niche factors for the necessity of fitness and adaptation, which can inadvertently, collaterally, coincidentally and/or randomly result in virulence as a by-product of commensalism (Le Gall et al., 2007; Tenaillon et al., 2010; Hill, 2012; Leimbach et al., 2013). Integrating the physiopathology to the ecophysiology of this species is certainly the next frontier in understanding the forces at play in the E. coli genome plasticity and the emergence of new virulence traits and/or pathotypes.
Author Contributions
MD and RB wrote the first overall draft of the manuscript. RB drew the original pictures. MD, GD, RBe, NB, JD, and RBo wrote sections of the manuscript. RB contributed to conceptualize the overarching aims and had management as well as coordination responsibility for the execution of the work. All authors contributed to the critical revision of the manuscript, read and approved the submitted version.
Funding
This study was supported by the Ministère de la Recherche et de la Technologie, Inserm (U1071), and INRAE (USC-2018 and UMR0454).
Conflict of Interest
The authors declare that the research was conducted in the absence of any commercial or financial relationships that could be construed as a potential conflict of interest.
Abbreviations
A/E, attaching and effacing; AA, aggregative adherence; AAF, aggregative adherence fimbriae; ABU, asymptomatic bacteriuria; aEHEC, atypical enterohemorrhagic E. coli; aEPEC, atypical enteropathogenic E. coli; Afa, afimbrial adhesin; Ag43, antigen 43; AIEC, adherent-invasive E. coli; APEC, avian pathogenic E. coli; AT, autotransporter; CASPR1, contactin-associated protein 1; CDS, coding sequence; Cdt, cytolethal and distending toxin; CFA, colonization factor antigen; CFTR, cystic fibrosis transmembrane conductance regulator; CLB, colibactin; CNF-1, cytotoxic necrotizing factor-1; DAEC, diffusely adherent E. coli; DEC, diarrheagenic E. coli; Dr, decay-accelerating factor receptor; DRE, downstream regulatory element; DRS, direct repeat sequences; EAEC, enteroaggregative E. coli; ECP, E. coli common pili; EHEC, enterohemorrhagic E. coli; EIEC, enteroinvasive E. coli; EPEC, enteropathogenic E. coli; EspC, E. coli secreted protein C; ETEC, enterotoxigenic E. coli; ExPEC, extraintestinal E. coli; FAK, focal adhesion kinase; GbO3, globotriosylceramide; GbO4, globotetraosylceramide; GbO5, globopentaosylceramide; GI, genomic islands; GS, genome segment; HBMEC, human brain microvascular endothelial cell; Hbp, hemoglobin binding protein or hemoglobin protease; HPI, high-pathogenicity island; Hra, heat-resistant agglutinin; HT, horizontal transfer; HUS, syndrome hemolytic and uremic; IBC, intracellular bacterial community; IBD, inflammatory bowel disease; ICE, integrative and conjugative elements; Iha, iron regulated gene homolog adhesin; InPEC, intestinal pathogenic E. coli; LA, localized adherence; LAA, Locus of adhesion and autoaggregation; LAL, localized-adherence like; LEE, locus of enterocyte effacement; Ler, LEE-encoded regulator; LifA, lymphocyte inhibitory factor; LPS, lipopolysaccharide; LT, heat-labile enterotoxin; NlpI, new lipoprotein I; NMEC, neonatal meningitis E. coli; OmpA, outer membrane protein A; PagG, PhoP-activated gene C; PAI, pathogenicity islands; Pap, pyelonephritis associated pili; Pet, plasmid-encoded toxin; PF, Pathogenicity factors; Pic, protein involve in colonization; RDF, recombination directionality factor; RTX, repeat-in-toxin; SAAT, self-assocoiating autotransporter; Sat, secreted autotransporter toxin; SEPEC, sepsis-associated E. coli; Sha, serine-protease hemagglutinin autotransporter; ShET1, shigella enterotoxin 1; ShET2, shigella enterotoxin 2; SHI, shigella pathogenicity island; SigA, Shigella immunoglobulin A protease; Sit, Salmonella iron transport; SPATE, serine protease autotransporters of Enterobacteriaceae; SpLE, sakai prophage-like element; SslE, secreted and surface associated lipoprotein; ST, heat-stable enterotoxin; STEC, shigatoxin-producing E. coli; Stx, shigatoxin; T2SS, type II secretion system; T3aSS, type III, subtype a, secretion system; T3SS, type III secretion system; T4SS, type IV secretion system; Tag, tandem autotransporter gene; TcpC, TLR domain containing-protein C; tEHEC, typical enterohemorrhagic E. coli; tEPEC, typical enteropathogenic E. coli; Tia, toxigenic invasion locus A; TLR, toll-like receptor; Tsh, temperature sensitive hemagglutinin; TTP, thrombotic thrombocytopenic purpura; UPEC, uropathogenic E. coli; UTI, urinary tract infection; Vat, vacuolating autotransporter toxin; Ybt, yersioniabactin.
References
Abraham, S. N., Sun, D., Dale, J. B., and Beachey, E. H. (1988). Conservation of the D-mannose-adhesion protein among type 1 fimbriated members of the family Enterobacteriaceae. Nature 336, 682–684. doi: 10.1038/336682a0
Adams, M. M., Allison, G. E., and Verma, N. K. (2001). Type IV O antigen modification genes in the genome of Shigella flexneri NCTC 8296. Microbiology 147, 851–860. doi: 10.1099/00221287-147-4-851
Agaisse, H. (2016). Molecular and cellular mechanisms of Shigella flexneri dissemination. Front. Cell. Infect. Microbiol. 6:29. doi: 10.3389/fcimb.2016.00029
Ageorges, V., Monteiro, R., Leroy, S., Burgess, C. M., Pizza, M., Chaucheyras-Durand, F., et al. (2020). Molecular Determinants of Surface Colonisation in Diarrhoeagenic Escherichia coli (DEC): from Bacterial Adhesion to Biofilm Formation. FEMS Microbiol. Rev. 44, 314–350. doi: 10.1093/femsre/fuaa008
Al-Hasani, K., Adler, B., Rajakumar, K., and Sakellaris, H. (2001a). Distribution and structural variation of the she pathogenicity island in enteric bacterial pathogens. J. Med. Microbiol. 50, 780–786. doi: 10.1099/0022-1317-50-9-780
Al-Hasani, K., Henderson, I. R., Sakellaris, H., Rajakumar, K., Grant, T., Nataro, J. P., et al. (2000). The sigA gene which is borne on the she pathogenicity island of Shigella flexneri 2a encodes an exported cytopathic protease involved in intestinal fluid accumulation. Infect. Immun. 68, 2457–2463. doi: 10.1128/iai.68.5.2457-2463.2000
Al-Hasani, K., Rajakumar, K., Bulach, D., Robins-Browne, R., Adler, B., and Sakellaris, H. (2001b). Genetic organization of the she pathogenicity island in Shigella flexneri 2a. Microb. Pathog. 30, 1–8. doi: 10.1006/mpat.2000.0404
Allison, G. E., and Verma, N. K. (2000). Serotype-converting bacteriophages and O-antigen modification in Shigella flexneri. Trends Microbiol. 8, 17–23. doi: 10.1016/s0966-842x(99)01646-7
Allsopp, L. P., Beloin, C., Ulett, G. C., Valle, J., Totsika, M., Sherlock, O., et al. (2012). Molecular characterization of UpaB and UpaC, two new autotransporter proteins of uropathogenic Escherichia coli CFT073. Infect. Immun. 80, 321–332. doi: 10.1128/IAI.05322-11
Ananias, M., and Yano, T. (2008). Serogroups and virulence genotypes of Escherichia coli isolated from patients with sepsis. Braz. J. Med. Biol. Res. 41, 877–883. doi: 10.1590/s0100-879x2008001000008
Anderson, G. G., Palermo, J. J., Schilling, J. D., Roth, R., Heuser, J., and Hultgren, S. J. (2003). Intracellular bacterial biofilm-like pods in urinary tract infections. Science 301, 105–107. doi: 10.1126/science.1084550
Andersson, P., Engberg, I., Lidin-Janson, G., Lincoln, K., Hull, R., Hull, S., et al. (1991). Persistence of Escherichia coli bacteriuria is not determined by bacterial adherence. Infect. Immun. 59, 2915–2921.
Antão, E.-M., Wieler, L. H., and Ewers, C. (2009). Adhesive threads of extraintestinal pathogenic Escherichia coli. Gut Pathog. 1:22. doi: 10.1186/1757-4749-1-22
Arthur, J. C., Perez-Chanona, E., Mühlbauer, M., Tomkovich, S., Uronis, J. M., Fan, T.-J., et al. (2012). Intestinal inflammation targets cancer-inducing activity of the microbiota. Science 338, 120–123. doi: 10.1126/science.1224820
Askari Badouei, M., Jajarmi, M., and Mirsalehian, A. (2015). Virulence profiling and genetic relatedness of Shiga toxin-producing Escherichia coli isolated from humans and ruminants. Comp. Immunol. Microbiol. Infect. Dis. 38, 15–20. doi: 10.1016/j.cimid.2014.11.005
Badea, L., Doughty, S., Nicholls, L., Sloan, J., Robins-Browne, R. M., and Hartland, E. L. (2003). Contribution of Efa1/LifA to the adherence of enteropathogenic Escherichia coli to epithelial cells. Microb. Pathog. 34, 205–215. doi: 10.1016/s0882-4010(03)00026-3
Badger, J. L., Wass, C. A., Weissman, S. J., and Kim, K. S. (2000). Application of signature-tagged mutagenesis for identification of Escherichia coli K1 genes that contribute to invasion of human brain microvascular endothelial cells. Infect. Immun. 68, 5056–5061. doi: 10.1128/iai.68.9.5056-5061.2000
Bark, T., Katouli, M., Svenberg, T., and Ljungqvist, O. (1995). Food deprivation increases bacterial translocation after non-lethal haemorrhage in rats. Eur. J. Surg. 161, 67–71.
Basmaci, R., Bonacorsi, S., Bidet, P., Biran, V., Aujard, Y., Bingen, E., et al. (2015). Escherichia Coli meningitis features in 325 children from 2001 to 2013 in France. Clin. Infect. Dis. 61, 779–786. doi: 10.1093/cid/civ367
Bateman, S. L., and Seed, P. (2012a). Intracellular Macrophage Infections with E. coli under Nitrosative Stress. Bio Protoc. 2:e275. doi: 10.21769/BioProtoc.275
Bateman, S. L., and Seed, P. (2012b). Promoter orientation of prokaryotic phase-variable genes by PCR. Bio Protoc. 2:e274. doi: 10.21769/bioprotoc.274
Bateman, S. L., Stapleton, A. E., Stamm, W. E., Hooton, T. M., and Seed, P. C. (2013). The type 1 pili regulator gene fimX and pathogenicity island PAI-X as molecular markers of uropathogenic Escherichia coli. Microbiology 159, 1606–1617. doi: 10.1099/mic.0.066472-0
Battaglioli, E. J., Goh, K. G. K., Atruktsang, T. S., Schwartz, K., Schembri, M. A., and Welch, R. A. (2018). Identification and characterization of a phase-variable element that regulates the autotransporter upae in uropathogenic Escherichia coli. mBio 9:e01360-18. doi: 10.1128/mBio.01360-18
Battle, S. E., Brady, M. J., Vanaja, S. K., Leong, J. M., and Hecht, G. A. (2014). Actin pedestal formation by enterohemorrhagic Escherichia coli enhances bacterial host cell attachment and concomitant type III translocation. Infect. Immun. 82, 3713–3722. doi: 10.1128/IAI.01523-13
Beck, C. M., Willett, J. L. E., Cunningham, D. A., Kim, J. J., Low, D. A., and Hayes, C. S. (2016). CdiA effectors from uropathogenic Escherichia coli use heterotrimeric osmoporins as receptors to recognize target bacteria. PLoS Pathog. 12:e1005925. doi: 10.1371/journal.ppat.1005925
Behrens, M., Sheikh, J., and Nataro, J. P. (2002). Regulation of the overlapping pic/set locus in Shigella flexneri and enteroaggregative Escherichia coli. Infect. Immun. 70, 2915–2925. doi: 10.1128/iai.70.6.2915-2925.2002
Belotserkovsky, I., and Sansonetti, P. J. (2018). Shigella and Enteroinvasive Escherichia Coli. Curr. Top. Microbiol. Immunol. 416, 1–26. doi: 10.1007/82_2018_104
Benedek, O., and Schubert, S. (2007). Mobility of the Yersinia High-Pathogenicity Island (HPI): transfer mechanisms of pathogenicity islands (PAIS) revisited (a review). Acta Microbiol. Immunol. Hung. 54, 89–105. doi: 10.1556/AMicr.54.2007.2.1
Bergsten, G., Wullt, B., and Svanborg, C. (2005). Escherichia coli, fimbriae, bacterial persistence and host response induction in the human urinary tract. Int. J. Med. Microbiol. 295, 487–502. doi: 10.1016/j.ijmm.2005.07.008
Bertelli, C., Laird, M. R., Williams, K. P., Simon Fraser University Research Computing Group, Lau, B. Y., Hoad, G., et al. (2017). IslandViewer 4: expanded prediction of genomic islands for larger-scale datasets. Nucleic Acids Res. 45, W30–W35. doi: 10.1093/nar/gkx343
Bertelli, C., Tilley, K. E., and Brinkman, F. S. L. (2019). Microbial genomic island discovery, visualization and analysis. Brief. Bioinformatics 20, 1685–1698. doi: 10.1093/bib/bby042
Bertin, Y., Boukhors, K., Livrelli, V., and Martin, C. (2004). Localization of the insertion site and pathotype determination of the locus of enterocyte effacement of shiga toxin-producing Escherichia coli strains. Appl. Environ. Microbiol. 70, 61–68. doi: 10.1128/aem.70.1.61-68.2004
Bi, D., Xu, Z., Harrison, E. M., Tai, C., Wei, Y., He, X., et al. (2012). ICEberg: a web-based resource for integrative and conjugative elements found in Bacteria. Nucleic Acids Res. 40, D621–D626. doi: 10.1093/nar/gkr846
Bidet, P., Bonacorsi, S., Clermont, O., De Montille, C., Brahimi, N., and Bingen, E. (2005). Multiple insertional events, restricted by the genetic background, have led to acquisition of pathogenicity island IIJ96-like domains among Escherichia coli strains of different clinical origins. Infect. Immun. 73, 4081–4087. doi: 10.1128/IAI.73.7.4081-4087.2005
Bielaszewska, M., Middendorf, B., Tarr, P. I., Zhang, W., Prager, R., Aldick, T., et al. (2011). Chromosomal instability in enterohaemorrhagic Escherichia coli O157:H7: impact on adherence, tellurite resistance and colony phenotype. Mol. Microbiol. 79, 1024–1044. doi: 10.1111/j.1365-2958.2010.07499.x
Bingen-Bidois, M., Clermont, O., Bonacorsi, S., Terki, M., Brahimi, N., Loukil, C., et al. (2002). Phylogenetic analysis and prevalence of urosepsis strains of Escherichia coli bearing pathogenicity island-like domains. Infect. Immun. 70, 3216–3226. doi: 10.1128/iai.70.6.3216-3226.2002
Blanco, M., Blanco, J. E., Mora, A., Dahbi, G., Alonso, M. P., González, E. A., et al. (2004). Serotypes, virulence genes, and intimin types of Shiga toxin (verotoxin)-producing Escherichia coli isolates from cattle in Spain and identification of a new intimin variant gene (eae-xi). J. Clin. Microbiol. 42, 645–651. doi: 10.1128/jcm.42.2.645-651.2004
Bloom, F. R., and McFall, E. (1975). Isolation and characterization of D-serine deaminase constitutive mutants by utilization of D-serine as sole carbon or nitrogen source. J. Bacteriol. 121, 1078–1084.
Blum, G., Ott, M., Lischewski, A., Ritter, A., Imrich, H., Tschäpe, H., et al. (1994). Excision of large DNA regions termed pathogenicity islands from tRNA-specific loci in the chromosome of an Escherichia coli wild-type pathogen. Infect. Immun. 62, 606–614.
Bonacorsi, S., and Bingen, E. (2005). Molecular epidemiology of Escherichia coli causing neonatal meningitis. Int. J. Med. Microbiol. 295, 373–381. doi: 10.1016/j.ijmm.2005.07.011
Bonacorsi, S., Clermont, O., Houdouin, V., Cordevant, C., Brahimi, N., Marecat, A., et al. (2003). Molecular analysis and experimental virulence of French and North American Escherichia coli neonatal meningitis isolates: identification of a new virulent clone. J. Infect. Dis. 187, 1895–1906. doi: 10.1086/375347
Bonacorsi, S., Houdouin, V., Mariani-Kurkdjian, P., Mahjoub-Messai, F., and Bingen, E. (2006). Comparative prevalence of virulence factors in Escherichia coli causing urinary tract infection in male infants with and without bacteremia. J. Clin. Microbiol. 44, 1156–1158. doi: 10.1128/JCM.44.3.1156-1158.2006
Bondì, R., Chiani, P., Michelacci, V., Minelli, F., Caprioli, A., and Morabito, S. (2017). The Gene tia, harbored by the subtilase-encoding pathogenicity island, is involved in the ability of locus of enterocyte effacement-negative shiga toxin-producing Escherichia coli strains to invade monolayers of epithelial cells. Infect. Immun. 85:e00613-17. doi: 10.1128/IAI.00613-17
Bower, J. M., Eto, D. S., and Mulvey, M. A. (2005). Covert operations of uropathogenic Escherichia coli within the urinary tract. Traffic 6, 18–31. doi: 10.1111/j.1600-0854.2004.00251.x
Boyd, E. F., Almagro-Moreno, S., and Parent, M. A. (2009). Genomic islands are dynamic, ancient integrative elements in bacterial evolution. Trends Microbiol. 17, 47–53. doi: 10.1016/j.tim.2008.11.003
Boyd, E. F., Davis, B. M., and Hochhut, B. (2001). Bacteriophage-bacteriophage interactions in the evolution of pathogenic bacteria. Trends Microbiol. 9, 137–144. doi: 10.1016/s0966-842x(01)01960-6
Bronowski, C., Smith, S. L., Yokota, K., Corkill, J. E., Martin, H. M., Campbell, B. J., et al. (2008). A subset of mucosa-associated Escherichia coli isolates from patients with colon cancer, but not Crohn’s disease, share pathogenicity islands with urinary pathogenic E. coli. Microbiology 154, 571–583. doi: 10.1099/mic.0.2007/013086-0
Brutinel, E. D., and Yahr, T. L. (2008). Control of gene expression by type III secretory activity. Curr. Opin. Microbiol. 11, 128–133. doi: 10.1016/j.mib.2008.02.010
Bryan, A., Roesch, P., Davis, L., Moritz, R., Pellett, S., and Welch, R. A. (2006). Regulation of type 1 fimbriae by unlinked FimB- and FimE-like recombinases in uropathogenic Escherichia coli strain CFT073. Infect. Immun. 74, 1072–1083. doi: 10.1128/IAI.74.2.1072-1083.2006
Brzuszkiewicz, E., Brüggemann, H., Liesegang, H., Emmerth, M., Olschläger, T., Nagy, G., et al. (2006). How to become a uropathogen: comparative genomic analysis of extraintestinal pathogenic Escherichia coli strains. Proc. Natl. Acad. Sci. U.S.A. 103, 12879–12884. doi: 10.1073/pnas.0603038103
Buc, E., Dubois, D., Sauvanet, P., Raisch, J., Delmas, J., Darfeuille-Michaud, A., et al. (2013). High prevalence of mucosa-associated E. coli producing cyclomodulin and genotoxin in colon cancer. PLoS One 8:e56964. doi: 10.1371/journal.pone.0056964
Buchrieser, C., Brosch, R., Bach, S., Guiyoule, A., and Carniel, E. (1998). The high-pathogenicity island of Yersinia pseudotuberculosis can be inserted into any of the three chromosomal asn tRNA genes. Mol. Microbiol. 30, 965–978. doi: 10.1046/j.1365-2958.1998.01124.x
Buckles, E. L., Bahrani-Mougeot, F. K., Molina, A., Lockatell, C. V., Johnson, D. E., Drachenberg, C. B., et al. (2004). Identification and characterization of a novel uropathogenic Escherichia coli-associated fimbrial gene cluster. Infect. Immun. 72, 3890–3901. doi: 10.1128/IAI.72.7.3890-3901.2004
Campbell-Valois, F.-X., Sachse, M., Sansonetti, P. J., and Parsot, C. (2015). Escape of Actively Secreting Shigella flexneri from ATG8/LC3-positive vacuoles formed during cell-to-cell spread is facilitated by IcsB and VirA. mBio 6:e02567-14. doi: 10.1128/mBio.02567-14
Campos, L. C., Vieira, M. A., Trabulsi, L. R., da Silva, L. A., Monteiro-Neto, V., and Gomes, T. A. (1999). Diffusely adhering Escherichia coli (DAEC) strains of fecal origin rarely express F1845 adhesin. Microbiol. Immunol. 43, 167–170. doi: 10.1111/j.1348-0421.1999.tb02388.x
Caprioli, A., Falbo, V., Ruggeri, F. M., Baldassarri, L., Bisicchia, R., Ippolito, G., et al. (1987). Cytotoxic necrotizing factor production by hemolytic strains of Escherichia coli causing extraintestinal infections. J. Clin. Microbiol. 25, 146–149.
Caprioli, A., Morabito, S., Brugère, H., and Oswald, E. (2005). Enterohaemorrhagic Escherichia coli: emerging issues on virulence and modes of transmission. Vet. Res. 36, 289–311. doi: 10.1051/vetres:2005002
Carniel, E. (2001). The Yersinia high-pathogenicity island: an iron-uptake island. Microbes Infect. 3, 561–569. doi: 10.1016/s1286-4579(01)01412-5
Cascales, E., Buchanan, S. K., Duché, D., Kleanthous, C., Lloubès, R., Postle, K., et al. (2007). Colicin biology. Microbiol. Mol. Biol. Rev. 71, 158–229. doi: 10.1128/MMBR.00036-06
Chaudhuri, R. R., and Henderson, I. R. (2012). The evolution of the Escherichia coli phylogeny. Infect. Genet. Evol. 12, 214–226. doi: 10.1016/j.meegid.2012.01.005
Chaudhuri, R. R., Sebaihia, M., Hobman, J. L., Webber, M. A., Leyton, D. L., Goldberg, M. D., et al. (2010). Complete genome sequence and comparative metabolic profiling of the prototypical enteroaggregative Escherichia coli strain 042. PLoS One 5:e8801. doi: 10.1371/journal.pone.0008801
Cheetham, B. F., and Katz, M. E. (1995). A role for bacteriophages in the evolution and transfer of bacterial virulence determinants. Mol. Microbiol. 18, 201–208. doi: 10.1111/j.1365-2958.1995.mmi_18020201.x
Chen, Z., and Schneider, T. D. (2006). Comparative analysis of tandem T7-like promoter containing regions in enterobacterial genomes reveals a novel group of genetic islands. Nucleic Acids Res. 34, 1133–1147. doi: 10.1093/nar/gkj511
Chua, E. G., Al-Hasani, K., Scanlon, M., Adler, B., and Sakellaris, H. (2015). Determinants of proteolysis and cell-binding for the Shigella flexneri Cytotoxin, SigA. Curr. Microbiol. 71, 613–617. doi: 10.1007/s00284-015-0893-8
Cirl, C., Wieser, A., Yadav, M., Duerr, S., Schubert, S., Fischer, H., et al. (2008). Subversion of Toll-like receptor signaling by a unique family of bacterial Toll/interleukin-1 receptor domain-containing proteins. Nat. Med. 14, 399–406. doi: 10.1038/nm1734
Clements, A., Young, J. C., Constantinou, N., and Frankel, G. (2012). Infection strategies of enteric pathogenic Escherichia coli. Gut Microbes 3, 71–87. doi: 10.4161/gmic.19182
Clermont, O., Dixit, O. V. A., Vangchhia, B., Condamine, B., Dion, S., Bridier-Nahmias, A., et al. (2019). Characterization and rapid identification of phylogroup G in Escherichia coli, a lineage with high virulence and antibiotic resistance potential. Environ. Microbiol 21, 3107–3117. doi: 10.1111/1462-2920.14713
Colello, R., Krüger, A., Velez, M. V., Del Canto, F., Etcheverría, A. I., Vidal, R., et al. (2019). Identification and detection of iha subtypes in LEE-negative Shiga toxin-producing Escherichia coli (STEC) strains isolated from humans, cattle and food. Heliyon 5:e03015. doi: 10.1016/j.heliyon.2019.e03015
Colello, R., Vélez, M. V., González, J., Montero, D. A., Bustamante, A. V., Del Canto, F., et al. (2018). First report of the distribution of Locus of Adhesion and Autoaggregation (LAA) pathogenicity island in LEE-negative Shiga toxin-producing Escherichia coli isolates from Argentina. Microb. Pathog. 123, 259–263. doi: 10.1016/j.micpath.2018.07.011
Connell, I., Agace, W., Klemm, P., Schembri, M., Mãrild, S., and Svanborg, C. (1996). Type 1 fimbrial expression enhances Escherichia coli virulence for the urinary tract. Proc. Natl. Acad. Sci. U.S.A. 93, 9827–9832. doi: 10.1073/pnas.93.18.9827
Connolly, J. P. R., Finlay, B. B., and Roe, A. J. (2015). From ingestion to colonization: the influence of the host environment on regulation of the LEE encoded type III secretion system in enterohaemorrhagic Escherichia coli. Front. Microbiol. 6:568. doi: 10.3389/fmicb.2015.00568
Coombes, B. K., Wickham, M. E., Mascarenhas, M., Gruenheid, S., Finlay, B. B., and Karmali, M. A. (2008). Molecular analysis as an aid to assess the public health risk of non-O157 Shiga toxin-producing Escherichia coli strains. Appl. Environ. Microbiol. 74, 2153–2160. doi: 10.1128/AEM.02566-07
Cordeiro, M. A., Werle, C. H., Milanez, G. P., and Yano, T. (2016). Curli fimbria: an Escherichia coli adhesin associated with human cystitis. Braz. J. Microbiol. 47, 414–416. doi: 10.1016/j.bjm.2016.01.024
Cornelis, G. R. (2006). The type III secretion injectisome. Nat. Rev. Microbiol. 4, 811–825. doi: 10.1038/nrmicro1526
Cosloy, S. D., and McFall, E. (1973). Metabolism of D-serine in Escherichia coli K-12: mechanism of growth inhibition. J. Bacteriol. 114, 685–694.
Cougnoux, A., Dalmasso, G., Martinez, R., Buc, E., Delmas, J., Gibold, L., et al. (2014). Bacterial genotoxin colibactin promotes colon tumour growth by inducing a senescence-associated secretory phenotype. Gut 63, 1932–1942. doi: 10.1136/gutjnl-2013-305257
Cougnoux, A., Delmas, J., Gibold, L., Faïs, T., Romagnoli, C., Robin, F., et al. (2016). Small-molecule inhibitors prevent the genotoxic and protumoural effects induced by colibactin-producing bacteria. Gut 65, 278–285. doi: 10.1136/gutjnl-2014-307241
Cristancho, L., Johnson, R. P., McEwen, S. A., and Gyles, C. L. (2008). Escherichia coli O157:H7 and other Shiga toxin-producing E. coli in white veal calves. Vet. Microbiol. 126, 200–209. doi: 10.1016/j.vetmic.2007.06.012
Crosa, J. H. (1989). Genetics and molecular biology of siderophore-mediated iron transport in bacteria. Microbiol. Rev. 53, 517–530.
Cross, A. S., Gemski, P., Sadoff, J. C., Orskov, F., and Orskov, I. (1984). The importance of the K1 capsule in invasive infections caused by Escherichia coli. J. Infect. Dis. 149, 184–193. doi: 10.1093/infdis/149.2.184
Crossman, L. C., Chaudhuri, R. R., Beatson, S. A., Wells, T. J., Desvaux, M., Cunningham, A. F., et al. (2010). A commensal gone bad: complete genome sequence of the prototypical enterotoxigenic Escherichia coli strain H10407. J. Bacteriol. 192, 5822–5831. doi: 10.1128/JB.00710-10
Croxen, M. A., and Finlay, B. B. (2010). Molecular mechanisms of Escherichia coli pathogenicity. Nat. Rev. Microbiol. 8, 26–38. doi: 10.1038/nrmicro2265
Croxen, M. A., Law, R. J., Scholz, R., Keeney, K. M., Wlodarska, M., and Finlay, B. B. (2013). Recent advances in understanding enteric pathogenic Escherichia coli. Clin. Microbiol. Rev. 26, 822–880. doi: 10.1128/CMR.00022-13
Cuevas-Ramos, G., Petit, C. R., Marcq, I., Boury, M., Oswald, E., and Nougayrède, J.-P. (2010). Escherichia coli induces DNA damage in vivo and triggers genomic instability in mammalian cells. Proc. Natl. Acad. Sci. U.S.A. 107, 11537–11542. doi: 10.1073/pnas.1001261107
Czeczulin, J. R., Whittam, T. S., Henderson, I. R., Navarro-Garcia, F., and Nataro, J. P. (1999). Phylogenetic analysis of enteroaggregative and diffusely adherent Escherichia coli. Infect. Immun. 67, 2692–2699.
Daga, A. P., Koga, V. L., Soncini, J. G. M., de Matos, C. M., Perugini, M. R. E., Pelisson, M., et al. (2019). Escherichia coli bloodstream infections in patients at a university hospital: virulence factors and clinical characteristics. Front. Cell. Infect. Microbiol. 9:191. doi: 10.3389/fcimb.2019.00191
Darfeuille-Michaud, A., Boudeau, J., Bulois, P., Neut, C., Glasser, A.-L., Barnich, N., et al. (2004). High prevalence of adherent-invasive Escherichia coli associated with ileal mucosa in Crohn’s disease. Gastroenterology 127, 412–421. doi: 10.1053/j.gastro.2004.04.061
Dautin, N. (2010). Serine protease autotransporters of enterobacteriaceae (SPATEs): biogenesis and function. Toxins 2, 1179–1206. doi: 10.3390/toxins2061179
Davis, J. M., Rasmussen, S. B., and O’Brien, A. D. (2005). Cytotoxic necrotizing factor type 1 production by uropathogenic Escherichia coli modulates polymorphonuclear leukocyte function. Infect. Immun. 73, 5301–5310. doi: 10.1128/IAI.73.9.5301-5310.2005
Dean, P., and Kenny, B. (2009). The effector repertoire of enteropathogenic E. coli: ganging up on the host cell. Curr. Opin. Microbiol. 12, 101–109. doi: 10.1016/j.mib.2008.11.006
Dean, P., Maresca, M., and Kenny, B. (2005). EPEC’s weapons of mass subversion. Curr. Opin. Microbiol. 8, 28–34. doi: 10.1016/j.mib.2004.12.010
Desvaux, M., Hébraud, M., Henderson, I. R., and Pallen, M. J. (2006). Type III secretion: What’s in a name? Trends Microbiol. 14, 157–160. doi: 10.1016/j.tim.2006.02.009
Dhakal, B. K., and Mulvey, M. A. (2012). The UPEC pore-forming toxin α-hemolysin triggers proteolysis of host proteins to disrupt cell adhesion, inflammatory, and survival pathways. Cell Host Microbe 11, 58–69. doi: 10.1016/j.chom.2011.12.003
Diepold, A., and Wagner, S. (2014). Assembly of the bacterial type III secretion machinery. FEMS Microbiol. Rev. 38, 802–822. doi: 10.1111/1574-6976.12061
Dobrindt, U., Hochhut, B., Hentschel, U., and Hacker, J. (2004). Genomic islands in pathogenic and environmental microorganisms. Nat. Rev. Microbiol. 2, 414–424. doi: 10.1038/nrmicro884
Dobrindt, U., Zdziarski, J., Salvador, E., and Hacker, J. (2010). Bacterial genome plasticity and its impact on adaptation during persistent infection. Int. J. Med. Microbiol. 300, 363–366. doi: 10.1016/j.ijmm.2010.04.010
Doolittle, R. F., Feng, D. F., Tsang, S., Cho, G., and Little, E. (1996). Determining divergence times of the major kingdoms of living organisms with a protein clock. Science 271, 470–477. doi: 10.1126/science.271.5248.470
Dozois, C. M., Dho-Moulin, M., Brée, A., Fairbrother, J. M., Desautels, C., and Curtiss, R. (2000). Relationship between the Tsh autotransporter and pathogenicity of avian Escherichia coli and localization and analysis of the Tsh genetic region. Infect. Immun. 68, 4145–4154. doi: 10.1128/iai.68.7.4145-4154.2000
Eisen, J. A., Heidelberg, J. F., White, O., and Salzberg, S. L. (2000). Evidence for symmetric chromosomal inversions around the replication origin in bacteria. Genome Biol. 1:RESEARCH0011. doi: 10.1186/gb-2000-1-6-research0011
Ejrnæs, K., Stegger, M., Reisner, A., Ferry, S., Monsen, T., Holm, S. E., et al. (2011). Characteristics of Escherichia coli causing persistence or relapse of urinary tract infections: phylogenetic groups, virulence factors and biofilm formation. Virulence 2, 528–537. doi: 10.4161/viru.2.6.18189
Elsinghorst, E. A., and Weitz, J. A. (1994). Epithelial cell invasion and adherence directed by the enterotoxigenic Escherichia coli tib locus is associated with a 104-kilodalton outer membrane protein. Infect. Immun. 62, 3463–3471.
Engstrom, M. D., and Mobley, H. L. T. (2016). Regulation of Expression of Uropathogenic Escherichia coli Nonfimbrial Adhesin TosA by PapB Homolog TosR in Conjunction with H-NS and Lrp. Infect. Immun. 84, 811–821. doi: 10.1128/IAI.01302-15
Escobar-Páramo, P., Clermont, O., Blanc-Potard, A.-B., Bui, H., Le Bouguénec, C., and Denamur, E. (2004a). A specific genetic background is required for acquisition and expression of virulence factors in Escherichia coli. Mol. Biol. Evol. 21, 1085–1094. doi: 10.1093/molbev/msh118
Escobar-Páramo, P., Grenet, K., Le Menac’h, A., Rode, L., Salgado, E., Amorin, C., et al. (2004b). Large-scale population structure of human commensal Escherichia coli isolates. Appl. Environ. Microbiol. 70, 5698–5700. doi: 10.1128/AEM.70.9.5698-5700.2004
Eto, D. S., Jones, T. A., Sundsbak, J. L., and Mulvey, M. A. (2007). Integrin-mediated host cell invasion by type 1-piliated uropathogenic Escherichia coli. PLoS Pathog. 3:e100. doi: 10.1371/journal.ppat.0030100
Eto, D. S., Sundsbak, J. L., and Mulvey, M. A. (2006). Actin-gated intracellular growth and resurgence of uropathogenic Escherichia coli. Cell. Microbiol. 8, 704–717. doi: 10.1111/j.1462-5822.2006.00691.x
Ewers, C., Li, G., Wilking, H., Kiessling, S., Alt, K., Antáo, E.-M., et al. (2007). Avian pathogenic, uropathogenic, and newborn meningitis-causing Escherichia coli: how closely related are they? Int. J. Med. Microbiol. 297, 163–176. doi: 10.1016/j.ijmm.2007.01.003
Fagan, R. P., and Smith, S. G. J. (2007). The Hek outer membrane protein of Escherichia coli is an auto-aggregating adhesin and invasin. FEMS Microbiol. Lett. 269, 248–255. doi: 10.1111/j.1574-6968.2006.00628.x
Fasano, A., Noriega, F. R., Maneval, D. R., Chanasongcram, S., Russell, R., Guandalini, S., et al. (1995). Shigella enterotoxin 1: an enterotoxin of Shigella flexneri 2a active in rabbit small intestine in vivo and in vitro. J. Clin. Invest. 95, 2853–2861. doi: 10.1172/JCI117991
Fiorentini, C., Arancia, G., Caprioli, A., Falbo, V., Ruggeri, F. M., and Donelli, G. (1988). Cytoskeletal changes induced in HEp-2 cells by the cytotoxic necrotizing factor of Escherichia coli. Toxicon 26, 1047–1056. doi: 10.1016/0041-0101(88)90203-6
Firoozeh, F., Saffari, M., Neamati, F., and Zibaei, M. (2014). Detection of virulence genes in Escherichia coli isolated from patients with cystitis and pyelonephritis. Int. J. Infect. Dis. 29, 219–222. doi: 10.1016/j.ijid.2014.03.1393
Fischbach, M. A., Lin, H., Zhou, L., Yu, Y., Abergel, R. J., Liu, D. R., et al. (2006). The pathogen-associated iroA gene cluster mediates bacterial evasion of lipocalin 2. Proc. Natl. Acad. Sci. U.S.A. 103, 16502–16507. doi: 10.1073/pnas.0604636103
Fischer, H., Yamamoto, M., Akira, S., Beutler, B., and Svanborg, C. (2006). Mechanism of pathogen-specific TLR4 activation in the mucosa: fimbriae, recognition receptors and adaptor protein selection. Eur. J. Immunol. 36, 267–277. doi: 10.1002/eji.200535149
Fisher, C. R., Davies, N. M. L. L., Wyckoff, E. E., Feng, Z., Oaks, E. V., and Payne, S. M. (2009). Genetics and virulence association of the Shigella flexneri sit iron transport system. Infect. Immun. 77, 1992–1999. doi: 10.1128/IAI.00064-09
Flatau, G., Lemichez, E., Gauthier, M., Chardin, P., Paris, S., Fiorentini, C., et al. (1997). Toxin-induced activation of the G protein p21 Rho by deamidation of glutamine. Nature 387, 729–733. doi: 10.1038/42743
Fleckenstein, J. M., Holland, J. T., and Hasty, D. L. (2002). Interaction of an uuter membrane protein of enterotoxigenic Escherichia coli with cell surface heparan sulfate proteoglycans. Infect. Immun. 70, 1530–1537. doi: 10.1128/iai.70.3.1530-1537.2002
Fleckenstein, J. M., Kopecko, D. J., Warren, R. L., and Elsinghorst, E. A. (1996). Molecular characterization of the tia invasion locus from enterotoxigenic Escherichia coli. Infect. Immun. 64, 2256–2265.
Fleckenstein, J. M., Lindler, L. E., Elsinghorst, E. A., and Dale, J. B. (2000). Identification of a gene within a pathogenicity island of enterotoxigenic Escherichia coli H10407 required for maximal secretion of the heat-labile enterotoxin. Infect. Immun. 68, 2766–2774. doi: 10.1128/iai.68.5.2766-2774.2000
Flores-Mireles, A. L., Walker, J. N., Caparon, M., and Hultgren, S. J. (2015). Urinary tract infections: epidemiology, mechanisms of infection and treatment options. Nat. Rev. Microbiol. 13, 269–284. doi: 10.1038/nrmicro3432
Fogg, P. C. M., Rigden, D. J., Saunders, J. R., McCarthy, A. J., and Allison, H. E. (2011). Characterization of the relationship between integrase, excisionase and antirepressor activities associated with a superinfecting Shiga toxin encoding bacteriophage. Nucleic Acids Res. 39, 2116–2129. doi: 10.1093/nar/gkq923
Foxman, B. (2003). Epidemiology of urinary tract infections: incidence, morbidity, and economic costs. Dis. Mon. 49, 53–70. doi: 10.1067/mda.2003.7
Franzin, F. M., and Sircili, M. P. (2015). Locus of enterocyte effacement: a pathogenicity island involved in the virulence of enteropathogenic and enterohemorragic Escherichia coli subjected to a complex network of gene regulation. Biomed Res. Int. 2015:534738. doi: 10.1155/2015/534738
Frendéus, B., Wachtler, C., Hedlund, M., Fischer, H., Samuelsson, P., Svensson, M., et al. (2001). Escherichia coli P fimbriae utilize the Toll-like receptor 4 pathway for cell activation. Mol. Microbiol. 40, 37–51. doi: 10.1046/j.1365-2958.2001.02361.x
Fujii, Y., Nomura, T., Yamanaka, H., and Okamoto, K. (1997). Involvement of Ca(2+)-calmodulin-dependent protein kinase II in the intestinal secretory action of Escherichia coli heat-stable enterotoxin II. Microbiol. Immunol. 41, 633–636. doi: 10.1111/j.1348-0421.1997.tb01904.x
Fukuta, S., Magnani, J. L., Twiddy, E. M., Holmes, R. K., and Ginsburg, V. (1988). Comparison of the carbohydrate-binding specificities of cholera toxin and Escherichia coli heat-labile enterotoxins LTh-I, LT-IIa, and LT-IIb. Infect. Immun. 56, 1748–1753.
Furniss, R. C. D., and Clements, A. (2018). Regulation of the locus of enterocyte effacement in attaching and effacing pathogens. J. Bacteriol. 200:e00336-17. doi: 10.1128/JB.00336-17
Galmiche, A., and Boquet, P. (2001). Toxines bactériennes?: facteurs de virulence et outils de biologie cellulaire. Med. Sci. 17, 691–700. doi: 10.4267/10608/1993
Gärtner, J. F., and Schmidt, M. A. (2004). Comparative analysis of locus of enterocyte effacement pathogenicity islands of atypical enteropathogenic Escherichia coli. Infect. Immun. 72, 6722–6728. doi: 10.1128/IAI.72.11.6722-6728.2004
Genuini, M., Bidet, P., Benoist, J.-F., Schlemmer, D., Lemaitre, C., Birgy, A., et al. (2019). ShiF acts as an auxiliary factor of aerobactin secretion in meningitis Escherichia coli strain S88. BMC Microbiol. 19:298. doi: 10.1186/s12866-019-1677-2
Gérardin, J., Lalioui, L., Jacquemin, E., Le Bouguénec, C., and Mainil, J. G. (2000). The afa-related gene cluster in necrotoxigenic and other Escherichia coli from animals belongs to the afa-8 variant. Vet. Microbiol. 76, 175–184. doi: 10.1016/s0378-1135(00)00234-0
Germon, P., Roche, D., Melo, S., Mignon-Grasteau, S., Dobrindt, U., Hacker, J., et al. (2007). tDNA locus polymorphism and ecto-chromosomal DNA insertion hot-spots are related to the phylogenetic group of Escherichia coli strains. Microbiology 153, 826–837. doi: 10.1099/mic.0.2006/001958-0
Gibold, L., Garenaux, E., Dalmasso, G., Gallucci, C., Cia, D., Mottet-Auselo, B., et al. (2016). The Vat-AIEC protease promotes crossing of the intestinal mucus layer by Crohn’s disease-associated Escherichia coli. Cell. Microbiol. 18, 617–631. doi: 10.1111/cmi.12539
Girardeau, J. P., Bertin, Y., and Martin, C. (2009). Genomic analysis of the PAI ICL3 locus in pathogenic LEE-negative Shiga toxin-producing Escherichia coli and Citrobacter rodentium. Microbiology 155, 1016–1027. doi: 10.1099/mic.0.026807-0
Girardeau, J. P., Dalmasso, A., Bertin, Y., Ducrot, C., Bord, S., Livrelli, V., et al. (2005). Association of virulence genotype with phylogenetic background in comparison to different seropathotypes of Shiga toxin-producing Escherichia coli isolates. J. Clin. Microbiol. 43, 6098–6107. doi: 10.1128/JCM.43.12.6098-6107.2005
Girardeau, J. P., Lalioui, L., Said, A. M. O., De Champs, C., and Le Bouguénec, C. (2003). Extended virulence genotype of pathogenic Escherichia coli isolates carrying the afa-8 operon: evidence of similarities between isolates from humans and animals with extraintestinal infections. J. Clin. Microbiol. 41, 218–226. doi: 10.1128/jcm.41.1.218-226.2003
Golan, L., Gonen, E., Yagel, S., Rosenshine, I., and Shpigel, N. Y. (2011). Enterohemorrhagic Escherichia coli induce attaching and effacing lesions and hemorrhagic colitis in human and bovine intestinal xenograft models. Dis. Model. Mech. 4, 86–94. doi: 10.1242/dmm.005777
Gransden, W. R., Eykyn, S. J., Phillips, I., and Rowe, B. (1990). Bacteremia due to Escherichia coli: a study of 861 episodes. Rev. Infect. Dis. 12, 1008–1018. doi: 10.1093/clinids/12.6.1008
Groisman, E. A., and Ochman, H. (1996). Pathogenicity islands: bacterial evolution in quantum leaps. Cell 87, 791–794. doi: 10.1016/s0092-8674(00)81985-6
Grönberg-Hernández, J., Hernández, J. G., Sundén, F., Connolly, J., Svanborg, C., and Wullt, B. (2011). Genetic control of the variable innate immune response to asymptomatic bacteriuria. PLoS One 6:e28289. doi: 10.1371/journal.pone.0028289
Gunther, N. W., Snyder, J. A., Lockatell, V., Blomfield, I., Johnson, D. E., and Mobley, H. L. T. (2002). Assessment of virulence of uropathogenic Escherichia coli type 1 fimbrial mutants in which the invertible element is phase-locked on or off. Infect. Immun. 70, 3344–3354. doi: 10.1128/iai.70.7.3344-3354.2002
Guyer, D. M., Henderson, I. R., Nataro, J. P., and Mobley, H. L. (2000). Identification of sat, an autotransporter toxin produced by uropathogenic Escherichia coli. Mol. Microbiol. 38, 53–66. doi: 10.1046/j.1365-2958.2000.02110.x
Guyer, D. M., Radulovic, S., Jones, F.-E., and Mobley, H. L. T. (2002). Sat, the secreted autotransporter toxin of uropathogenic Escherichia coli, is a vacuolating cytotoxin for bladder and kidney epithelial cells. Infect. Immun. 70, 4539–4546. doi: 10.1128/iai.70.8.4539-4546.2002
Habouria, H., Pokharel, P., Maris, S., Garénaux, A., Bessaiah, H., Houle, S., et al. (2019). Three new serine-protease autotransporters of Enterobacteriaceae (SPATEs) from extra-intestinal pathogenic Escherichia coli and combined role of SPATEs for cytotoxicity and colonization of the mouse kidney. Virulence 10, 568–587. doi: 10.1080/21505594.2019.1624102
Hacker, J., Bender, L., Ott, M., Wingender, J., Lund, B., Marre, R., et al. (1990). Deletions of chromosomal regions coding for fimbriae and hemolysins occur in vitro and in vivo in various extraintestinal Escherichia coli isolates. Microb. Pathog. 8, 213–225. doi: 10.1016/0882-4010(90)90048-u
Hacker, J., and Carniel, E. (2001). Ecological fitness, genomic islands and bacterial pathogenicity. A Darwinian view of the evolution of microbes. EMBO Rep. 2, 376–381. doi: 10.1093/embo-reports/kve097
Hacker, J., Knapp, S., and Goebel, W. (1983). Spontaneous deletions and flanking regions of the chromosomally inherited hemolysin determinant of an Escherichia coli O6 strain. J. Bacteriol. 154, 1145–1152.
Hagan, E. C., Lloyd, A. L., Rasko, D. A., Faerber, G. J., and Mobley, H. L. T. (2010). Escherichia coli global gene expression in urine from women with urinary tract infection. PLoS Pathog. 6:e1001187. doi: 10.1371/journal.ppat.1001187
Hagan, E. C., and Mobley, H. L. T. (2009). Haem acquisition is facilitated by a novel receptor Hma and required by uropathogenic Escherichia coli for kidney infection. Mol. Microbiol. 71, 79–91. doi: 10.1111/j.1365-2958.2008.06509.x
Han Wang, D. C. (2014). An accurate genomic island prediction method for sequenced bacterial and archaeal genomes. J. Proteomics Bioinform. 07, 214–221. doi: 10.4172/jpb.1000322
Hannan, T. J., Mysorekar, I. U., Chen, S. L., Walker, J. N., Jones, J. M., Pinkner, J. S., et al. (2008). LeuX tRNA-dependent and -independent mechanisms of Escherichia coli pathogenesis in acute cystitis. Mol. Microbiol. 67, 116–128. doi: 10.1111/j.1365-2958.2007.06025.x
Hauser, E., Mellmann, A., Semmler, T., Stoeber, H., Wieler, L. H., Karch, H., et al. (2013). Phylogenetic and molecular analysis of food-borne shiga toxin-producing Escherichia coli. Appl. Environ. Microbiol. 79, 2731–2740. doi: 10.1128/AEM.03552-12
Hayashi, T., Makino, K., Ohnishi, M., Kurokawa, K., Ishii, K., Yokoyama, K., et al. (2001). Complete genome sequence of enterohemorrhagic Escherichia coli O157:H7 and genomic comparison with a laboratory strain K-12. DNA Res. 8, 11–22. doi: 10.1093/dnares/8.1.11
Henderson, I. R., Czeczulin, J., Eslava, C., Noriega, F., and Nataro, J. P. (1999). Characterization of pic, a secreted protease of Shigella flexneri and enteroaggregative Escherichia coli. Infect. Immun. 67, 5587– 5596.
Henderson, I. R., Navarro-Garcia, F., and Nataro, J. P. (1998). The great escape: structure and function of the autotransporter proteins. Trends Microbiol. 6, 370–378. doi: 10.1016/s0966-842x(98)01318-3
Henderson, J. P., Crowley, J. R., Pinkner, J. S., Walker, J. N., Tsukayama, P., Stamm, W. E., et al. (2009). Quantitative metabolomics reveals an epigenetic blueprint for iron acquisition in uropathogenic Escherichia coli. PLoS Pathog. 5:e1000305. doi: 10.1371/journal.ppat.1000305
Hendriks, A. C. A., Reubsaet, F. A. G., Kooistra-Smid, A. M. D. M., Rossen, J. W. A., Dutilh, B. E., Zomer, A. L., et al. (2020). Genome-wide association studies of Shigella spp. and Enteroinvasive Escherichia coli isolates demonstrate an absence of genetic markers for prediction of disease severity. BMC Genomics 21:138. doi: 10.1186/s12864-020-6555-7
Henríquez, T., Salazar, J. C., Marvasi, M., Shah, A., Corsini, G., and Toro, C. S. (2020). SRL pathogenicity island contributes to the metabolism of D-aspartate via an aspartate racemase in Shigella flexneri YSH6000. PLoS One 15:e0228178. doi: 10.1371/journal.pone.0228178
Hernandes, R. T., Elias, W. P., Vieira, M. A. M., and Gomes, T. A. T. (2009). An overview of atypical enteropathogenic Escherichia coli. FEMS Microbiol. Lett. 297, 137–149. doi: 10.1111/j.1574-6968.2009.01664.x
Herzer, P. J., Inouye, S., Inouye, M., and Whittam, T. S. (1990). Phylogenetic distribution of branched RNA-linked multicopy single-stranded DNA among natural isolates of Escherichia coli. J. Bacteriol. 172, 6175–6181. doi: 10.1128/jb.172.11.6175-6181.1990
Hill, C. (2012). Virulence or niche factors: what’s in a name? J. Bacteriol. 194, 5725–5727. doi: 10.1128/JB.00980-12
Hochhut, B., Wilde, C., Balling, G., Middendorf, B., Dobrindt, U., Brzuszkiewicz, E., et al. (2006). Role of pathogenicity island-associated integrases in the genome plasticity of uropathogenic Escherichia coli strain 536. Mol. Microbiol. 61, 584–595. doi: 10.1111/j.1365-2958.2006.05255.x
Hoffman, J. A., Badger, J. L., Zhang, Y., Huang, S. H., and Kim, K. S. (2000). Escherichia coli K1 aslA contributes to invasion of brain microvascular endothelial cells in vitro and in vivo. Infect. Immun. 68, 5062–5067. doi: 10.1128/iai.68.9.5062-5067.2000
Hong, M., and Payne, S. M. (1997). Effect of mutations in Shigella flexneri chromosomal and plasmid-encoded lipopolysaccharide genes on invasion and serum resistance. Mol. Microbiol. 24, 779–791. doi: 10.1046/j.1365-2958.1997.3731744.x
Horstman, A. L., and Kuehn, M. J. (2002). Bacterial surface association of heat-labile enterotoxin through lipopolysaccharide after secretion via the general secretory pathway. J. Biol. Chem. 277, 32538–32545. doi: 10.1074/jbc.M203740200
Hryckowian, A. J., Baisa, G. A., Schwartz, K. J., and Welch, R. A. (2015). dsdA does not affect colonization of the murine urinary tract by Escherichia coli CFT073. PLoS One 10:e0138121. doi: 10.1371/journal.pone.0138121
Hu, J., Kan, B., Liu, Z.-H., and Yu, S.-Y. (2005). Enteroaggregative Escherichia coli isolated from Chinese diarrhea patients with high-pathogenicity island of Yersinia is involved in synthesis of siderophore yersiniabactin. World J. Gastroenterol. 11, 5816–5820. doi: 10.3748/wjg.v11.i37.5816
Hu, J., and Torres, A. G. (2015). Enteropathogenic Escherichia coli: foe or innocent bystander? Clin. Microbiol. Infect. 21, 729–734. doi: 10.1016/j.cmi.2015.01.015
Huan, P. T., Bastin, D. A., Whittle, B. L., Lindberg, A. A., and Verma, N. K. (1997). Molecular characterization of the genes involved in O-antigen modification, attachment, integration and excision in Shigella flexneri bacteriophage SfV. Gene 195, 217–227. doi: 10.1016/s0378-1119(97)00143-1
Huang, S. H., Chen, Y. H., Fu, Q., Stins, M., Wang, Y., Wass, C., et al. (1999). Identification and characterization of an Escherichia coli invasion gene locus, ibeB, required for penetration of brain microvascular endothelial cells. Infect. Immun. 67, 2103–2109.
Huang, S. H., Wan, Z. S., Chen, Y. H., Jong, A. Y., and Kim, K. S. (2001). Further characterization of Escherichia coli brain microvascular endothelial cell invasion gene ibeA by deletion, complementation, and protein expression. J. Infect. Dis. 183, 1071–1078. doi: 10.1086/319290
Huang, S. H., Wass, C., Fu, Q., Prasadarao, N. V., Stins, M., and Kim, K. S. (1995). Escherichia coli invasion of brain microvascular endothelial cells in vitro and in vivo: molecular cloning and characterization of invasion gene ibe10. Infect. Immun. 63, 4470–4475.
Hudson, C. M., Lau, B. Y., and Williams, K. P. (2015). Islander: a database of precisely mapped genomic islands in tRNA and tmRNA genes. Nucleic Acids Res. 43, D48–D53. doi: 10.1093/nar/gku1072
Hull, R. A., Rudy, D. C., Donovan, W. H., Wieser, I. E., Stewart, C., and Darouiche, R. O. (1999). Virulence properties of Escherichia coli 83972, a prototype strain associated with asymptomatic bacteriuria. Infect. Immun. 67, 429–432.
Hultdin, U. W., Lindberg, S., Grundström, C., Huang, S., Uhlin, B. E., and Sauer-Eriksson, A. E. (2010). Structure of FocB–a member of a family of transcription factors regulating fimbrial adhesin expression in uropathogenic Escherichia coli. FEBS J. 277, 3368–3381. doi: 10.1111/j.1742-4658.2010.07742.x
Iguchi, A., Thomson, N. R., Ogura, Y., Saunders, D., Ooka, T., Henderson, I. R., et al. (2009). Complete genome sequence and comparative genome analysis of enteropathogenic Escherichia coli O127:H6 strain E2348/69. J. Bacteriol. 191, 347–354. doi: 10.1128/JB.01238-08
Imamovic, L., Tozzoli, R., Michelacci, V., Minelli, F., Marziano, M. L., Caprioli, A., et al. (2010). OI-57, a genomic island of Escherichia coli O157, is present in other seropathotypes of Shiga toxin-producing E. coli associated with severe human disease. Infect. Immun. 78, 4697–4704. doi: 10.1128/IAI.00512-10
Ingersoll, M., Groisman, E. A., and Zychlinsky, A. (2002). Pathogenicity islands of Shigella. Curr. Top. Microbiol. Immunol. 264, 49–65.
Ingersoll, M. A., Moss, J. E., Weinrauch, Y., Fisher, P. E., Groisman, E. A., and Zychlinsky, A. (2003). The ShiA protein encoded by the Shigella flexneri SHI-2 pathogenicity island attenuates inflammation. Cell. Microbiol. 5, 797–807. doi: 10.1046/j.1462-5822.2003.00320.x
Ingersoll, M. A., and Zychlinsky, A. (2006). ShiA abrogates the innate T-cell response to Shigella flexneri infection. Infect. Immun. 74, 2317–2327. doi: 10.1128/IAI.74.4.2317-2327.2006
Isidean, S. D., Riddle, M. S., Savarino, S. J., and Porter, C. K. (2011). A systematic review of ETEC epidemiology focusing on colonization factor and toxin expression. Vaccine 29, 6167–6178. doi: 10.1016/j.vaccine.2011.06.084
Jenkins, C. (2018). Enteroaggregative Escherichia coli. Curr. Top. Microbiol. Immunol. 416, 27–50. doi: 10.1007/82_2018_105
Jobling, M. G., and Holmes, R. K. (2006). Heat-Labile Enterotoxins. EcoSal Plus 2, 1–28. doi: 10.1128/ecosalplus.8.7.5
Johnson, J. R. (1991). Virulence factors in Escherichia coli urinary tract infection. Clin. Microbiol. Rev. 4, 80–128. doi: 10.1128/cmr.4.1.80
Johnson, J. R., Johnston, B., Kuskowski, M. A., Nougayrede, J.-P., and Oswald, E. (2008). Molecular epidemiology and phylogenetic distribution of the Escherichia coli pks genomic island. J. Clin. Microbiol. 46, 3906–3911. doi: 10.1128/JCM.00949-08
Johnson, J. R., and Kuskowski, M. (2000). Clonal origin, virulence factors, and virulence. Infect. Immun. 68, 424–425.
Johnson, J. R., Kuskowski, M. A., Gajewski, A., Sahm, D. F., and Karlowsky, J. A. (2004). Virulence characteristics and phylogenetic background of multidrug-resistant and antimicrobial-susceptible clinical isolates of Escherichia coli from across the United States, 2000-2001. J. Infect. Dis. 190, 1739–1744. doi: 10.1086/425018
Johnson, J. R., Oswald, E., O’Bryan, T. T., Kuskowski, M. A., and Spanjaard, L. (2002). Phylogenetic distribution of virulence-associated genes among Escherichia coli isolates associated with neonatal bacterial meningitis in the Netherlands. J. Infect. Dis. 185, 774–784. doi: 10.1086/339343
Johnson, T. J., Kariyawasam, S., Wannemuehler, Y., Mangiamele, P., Johnson, S. J., Doetkott, C., et al. (2007). The genome sequence of avian pathogenic Escherichia coli strain O1:K1:H7 shares strong similarities with human extraintestinal pathogenic E. coli genomes. J. Bacteriol. 189, 3228–3236. doi: 10.1128/JB.01726-06
Johnson, T. J., Logue, C. M., Johnson, J. R., Kuskowski, M. A., Sherwood, J. S., Barnes, H. J., et al. (2012). Associations between multidrug resistance, plasmid content, and virulence potential among extraintestinal pathogenic and commensal Escherichia coli from humans and poultry. Foodborne Pathog. Dis. 9, 37–46. doi: 10.1089/fpd.2011.0961
Jores, J., Rumer, L., and Wieler, L. H. (2004). Impact of the locus of enterocyte effacement pathogenicity island on the evolution of pathogenic Escherichia coli. Int. J. Med. Microbiol. 294, 103–113. doi: 10.1016/j.ijmm.2004.06.024
Jores, J., Wagner, S., Rumer, L., Eichberg, J., Laturnus, C., Kirsch, P., et al. (2005). Description of a 111-kb pathogenicity island (PAI) encoding various virulence features in the enterohemorrhagic E. coli (EHEC) strain RW1374 (O103:H2) and detection of a similar PAI in other EHEC strains of serotype 0103:H2. Int. J. Med. Microbiol. 294, 417–425. doi: 10.1016/j.ijmm.2004.09.009
Ju, W., Shen, J., Toro, M., Zhao, S., and Meng, J. (2013). Distribution of pathogenicity islands OI-122, OI-43/48, and OI-57 and a high-pathogenicity island in Shiga toxin-producing Escherichia coli. Appl. Environ. Microbiol. 79, 3406–3412. doi: 10.1128/AEM.03661-12
Källenius, G., Möllby, R., Svenson, S. B., Winberg, J., and Hultberg, H. (1980). Identification of a carbohydrate receptor recognized by uropathogenic Escherichia coli. Infection 8(Suppl. 3), 288–293. doi: 10.1007/bf01639597
Kampmeier, S., Berger, M., Mellmann, A., Karch, H., and Berger, P. (2018). The 2011 German enterohemorrhagic Escherichia Coli O104:H4 outbreak-the danger is still out there. Curr. Top. Microbiol. Immunol. 416, 117–148. doi: 10.1007/82_2018_107
Kaper, J. B., Nataro, J. P., and Mobley, H. L. T. (2004). Pathogenic Escherichia coli. Nat. Rev. Microbiol. 2, 123–140. doi: 10.1038/nrmicro818
Karch, H., Schubert, S., Zhang, D., Zhang, W., Schmidt, H., Olschläger, T., et al. (1999). A genomic island, termed high-pathogenicity island, is present in certain non-O157 Shiga toxin-producing Escherichia coli clonal lineages. Infect. Immun. 67, 5994–6001.
Karlin, S. (2001). Detecting anomalous gene clusters and pathogenicity islands in diverse bacterial genomes. Trends Microbiol. 9, 335–343. doi: 10.1016/s0966-842x(01)02079-0
Karmali, M. A., Mascarenhas, M., Shen, S., Ziebell, K., Johnson, S., Reid-Smith, R., et al. (2003). Association of genomic O island 122 of Escherichia coli EDL 933 with verocytotoxin-producing Escherichia coli seropathotypes that are linked to epidemic and/or serious disease. J. Clin. Microbiol. 41, 4930–4940. doi: 10.1128/jcm.41.11.4930-4940.2003
Karmali, M. A., Steele, B. T., Petric, M., and Lim, C. (1983). Sporadic cases of haemolytic-uraemic syndrome associated with faecal cytotoxin and cytotoxin-producing Escherichia coli in stools. Lancet 1, 619–620. doi: 10.1016/s0140-6736(83)91795-6
Karr, J. F., Nowicki, B., Truong, L. D., Hull, R. A., and Hull, S. I. (1989). Purified P fimbriae from two cloned gene clusters of a single pyelonephritogenic strain adhere to unique structures in the human kidney. Infect. Immun. 57, 3594–3600.
Kehres, D. G., Janakiraman, A., Slauch, J. M., and Maguire, M. E. (2002). SitABCD is the alkaline Mn(2+) transporter of Salmonella enterica serovar Typhimurium. J. Bacteriol. 184, 3159–3166. doi: 10.1128/jb.184.12.3159-3166.2002
Keith, B. R., Maurer, L., Spears, P. A., and Orndorff, P. E. (1986). Receptor-binding function of type 1 pili effects bladder colonization by a clinical isolate of Escherichia coli. Infect. Immun. 53, 693–696.
Kendall, M. M. (2016). Interkingdom Chemical Signaling in Enterohemorrhagic Escherichia coli O157:H7. Adv. Exp. Med. Biol. 874, 201–213. doi: 10.1007/978-3-319-20215-0_9
Khan, N. A., Wang, Y., Kim, K. J., Chung, J. W., Wass, C. A., and Kim, K. S. (2002). Cytotoxic necrotizing factor-1 contributes to Escherichia coli K1 invasion of the central nervous system. J. Biol. Chem. 277, 15607–15612. doi: 10.1074/jbc.M112224200
Kim, K. J., Elliott, S. J., Di Cello, F., Stins, M. F., and Kim, K. S. (2003). The K1 capsule modulates trafficking of E. coli-containing vacuoles and enhances intracellular bacterial survival in human brain microvascular endothelial cells. Cell. Microbiol. 5, 245–252. doi: 10.1046/j.1462-5822.2003.t01-1-00271.x
Kim, K. S. (2002). Strategy of Escherichia coli for crossing the blood-brain barrier. J. Infect. Dis. 186(Suppl. 2), S220–S224. doi: 10.1086/344284
Kim, K. S. (2003). Pathogenesis of bacterial meningitis: from bacteraemia to neuronal injury. Nat. Rev. Neurosci. 4, 376–385. doi: 10.1038/nrn1103
Kim, K. S. (2008). Mechanisms of microbial traversal of the blood-brain barrier. Nat. Rev. Microbiol. 6, 625–634. doi: 10.1038/nrmicro1952
Kim, K. S. (2016). Human meningitis-associated Escherichia coli. EcoSal Plus 7, 1–15. doi: 10.1128/ecosalplus.ESP-0015-2015
Kim, K. S., Itabashi, H., Gemski, P., Sadoff, J., Warren, R. L., and Cross, A. S. (1992). The K1 capsule is the critical determinant in the development of Escherichia coli meningitis in the rat. J. Clin. Invest. 90, 897–905. doi: 10.1172/JCI115965
Kirsch, P., Jores, J., and Wieler, L. H. (2004). [Plasticity of bacterial genomes: pathogenicity islands and the locus of enterocyte effacement (LEE)]. Berl. Munch. Tierarztl. Wochenschr. 117, 116–129.
Klapproth, J. M., Scaletsky, I. C., McNamara, B. P., Lai, L. C., Malstrom, C., James, S. P., et al. (2000). A large toxin from pathogenic Escherichia coli strains that inhibits lymphocyte activation. Infect. Immun. 68, 2148–2155. doi: 10.1128/iai.68.4.2148-2155.2000
Klemm, P. (1986). Two regulatory fim genes, fimB and fimE, control the phase variation of type 1 fimbriae in Escherichia coli. EMBO J. 5, 1389–1393.
Klemm, P., Roos, V., Ulett, G. C., Svanborg, C., and Schembri, M. A. (2006). Molecular characterization of the Escherichia coli asymptomatic bacteriuria strain 83972: the taming of a pathogen. Infect. Immun. 74, 781–785. doi: 10.1128/IAI.74.1.781-785.2006
Konczy, P., Ziebell, K., Mascarenhas, M., Choi, A., Michaud, C., Kropinski, A. M., et al. (2008). Genomic O island 122, locus for enterocyte effacement, and the evolution of virulent verocytotoxin-producing Escherichia coli. J. Bacteriol. 190, 5832–5840. doi: 10.1128/JB.00480-08
Korhonen, T. K., Valtonen, M. V., Parkkinen, J., Väisänen-Rhen, V., Finne, J., Orskov, F., et al. (1985). Serotypes, hemolysin production, and receptor recognition of Escherichia coli strains associated with neonatal sepsis and meningitis. Infect. Immun. 48, 486–491.
Kostakioti, M., and Stathopoulos, C. (2004). Functional analysis of the Tsh autotransporter from an avian pathogenic Escherichia coli strain. Infect. Immun. 72, 5548–5554. doi: 10.1128/IAI.72.10.5548-5554.2004
Kothapalli, S., Nair, S., Alokam, S., Pang, T., Khakhria, R., Woodward, D., et al. (2005). Diversity of genome structure in Salmonella enterica serovar Typhi populations. J. Bacteriol. 187, 2638–2650. doi: 10.1128/JB.187.8.2638-2650.2005
Kreft, B., Placzek, M., Doehn, C., Hacker, J., Schmidt, G., Wasenauer, G., et al. (1995). S fimbriae of uropathogenic Escherichia coli bind to primary human renal proximal tubular epithelial cells but do not induce expression of intercellular adhesion molecule 1. Infect. Immun. 63, 3235–3238.
Krieger, J. N., Dobrindt, U., Riley, D. E., and Oswald, E. (2011). Acute Escherichia coli prostatitis in previously health young men: bacterial virulence factors, antimicrobial resistance, and clinical outcomes. Urology 77, 1420–1425. doi: 10.1016/j.urology.2010.12.059
Kumashiro, S., Hashimoto, A., and Nishikawa, T. (1995). Free D-serine in post-mortem brains and spinal cords of individuals with and without neuropsychiatric diseases. Brain Res. 681, 117–125. doi: 10.1016/0006-8993(95)00307-c
Kunin, C. M., and Halmagyi, N. E. (1962). Urinary-tract infections in schoolchildren. II. Characterization of invading organisms. N. Engl. J. Med. 266, 1297–1301. doi: 10.1056/NEJM196206212662502
Lai, Y., Rosenshine, I., Leong, J. M., and Frankel, G. (2013). Intimate host attachment: enteropathogenic and enterohaemorrhagic Escherichia coli. Cell. Microbiol. 15, 1796–1808. doi: 10.1111/cmi.12179
Laing, C. R., Buchanan, C., Taboada, E. N., Zhang, Y., Karmali, M. A., Thomas, J. E., et al. (2009). In silico genomic analyses reveal three distinct lineages of Escherichia coli O157:H7, one of which is associated with hyper-virulence. BMC Genomics 10:287. doi: 10.1186/1471-2164-10-287
Lalioui, L., Jouve, M., Gounon, P., and Le Bouguenec, C. (1999). Molecular cloning and characterization of the afa-7 and afa-8 gene clusters encoding afimbrial adhesins in Escherichia coli strains associated with diarrhea or septicemia in calves. Infect. Immun. 67, 5048–5059.
Lalioui, L., and Le Bouguénec, C. (2001). afa-8 Gene cluster is carried by a pathogenicity island inserted into the tRNA(Phe) of human and bovine pathogenic Escherichia coli isolates. Infect. Immun. 69, 937–948. doi: 10.1128/IAI.69.2.937-948.2001
Lan, R., Alles, M. C., Donohoe, K., Martinez, M. B., and Reeves, P. R. (2004). Molecular evolutionary relationships of enteroinvasive Escherichia coli and Shigella spp. Infect. Immun. 72, 5080–5088. doi: 10.1128/IAI.72.9.5080-5088.2004
Lan, R., Lumb, B., Ryan, D., and Reeves, P. R. (2001). Molecular evolution of large virulence plasmid in Shigella clones and enteroinvasive Escherichia coli. Infect. Immun. 69, 6303–6309. doi: 10.1128/IAI.69.10.6303-6309.2001
Lan, R., and Reeves, P. R. (2002). Escherichia coli in disguise: molecular origins of Shigella. Microbes Infect. 4, 1125–1132. doi: 10.1016/s1286-4579(02)01637-4
Lan, R., Stevenson, G., and Reeves, P. R. (2003). Comparison of two major forms of the Shigella virulence plasmid pINV: positive selection is a major force driving the divergence. Infect. Immun. 71, 6298–6306. doi: 10.1128/iai.71.11.6298-6306.2003
Lane, M. C., and Mobley, H. L. T. (2007). Role of P-fimbrial-mediated adherence in pyelonephritis and persistence of uropathogenic Escherichia coli (UPEC) in the mammalian kidney. Kidney Int. 72, 19–25. doi: 10.1038/sj.ki.5002230
Langille, M. G. I., Hsiao, W. W. L., and Brinkman, F. S. L. (2010). Detecting genomic islands using bioinformatics approaches. Nat. Rev. Microbiol. 8, 373–382. doi: 10.1038/nrmicro2350
Lara-Tejero, M., and Galán, J. E. (2019). The injectisome, a complex nanomachine for protein injection into mammalian cells. EcoSal Plus 8:10.1128/ecosalplus.ESP-0039-2018.
Latif, H., Li, H. J., Charusanti, P., Palsson, B. Ø., and Aziz, R. K. (2014). A Gapless, Unambiguous Genome Sequence of the Enterohemorrhagic Escherichia coli O157:H7 Strain EDL933. Genome Announc. 2:e00821-14. doi: 10.1128/genomeA.00821-14
Lawlor, K. M., and Payne, S. M. (1984). Aerobactin genes in Shigella spp. J. Bacteriol. 160, 266–272.
Lawrence, J. G., and Ochman, H. (1997). Amelioration of bacterial genomes: rates of change and exchange. J. Mol. Evol. 44, 383–397. doi: 10.1007/pl00006158
Le Bouguénec, C., Lalioui, L., du Merle, L., Jouve, M., Courcoux, P., Bouzari, S., et al. (2001). Characterization of AfaE adhesins produced by extraintestinal and intestinal human Escherichia coli isolates: PCR assays for detection of Afa adhesins that do or do not recognize Dr blood group antigens. J. Clin. Microbiol. 39, 1738–1745. doi: 10.1128/JCM.39.5.1738-1745.2001
Le Bouguénec, C., and Servin, A. L. (2006). Diffusely adherent Escherichia coli strains expressing Afa/Dr adhesins (Afa/Dr DAEC): hitherto unrecognized pathogens. FEMS Microbiol. Lett. 256, 185–194. doi: 10.1111/j.1574-6968.2006.00144.x
Le Gall, T., Clermont, O., Gouriou, S., Picard, B., Nassif, X., Denamur, E., et al. (2007). Extraintestinal virulence is a coincidental by-product of commensalism in B2 phylogenetic group Escherichia coli strains. Mol. Biol. Evol. 24, 2373–2384. doi: 10.1093/molbev/msm172
Lee, E., and Lee, Y. (2018). Prevalence of Escherichia coli carrying pks islands in bacteremia patients. Ann. Lab. Med. 38, 271–273. doi: 10.3343/alm.2018.38.3.271
Lehti, T. A., Heikkinen, J., Korhonen, T. K., and Westerlund-Wikström, B. (2012). The response regulator RcsB activates expression of Mat fimbriae in meningitic Escherichia coli. J. Bacteriol. 194, 3475–3485. doi: 10.1128/JB.06596-11
Leimbach, A., Hacker, J., and Dobrindt, U. (2013). E. coli as an all-rounder: the thin line between commensalism and pathogenicity. Curr. Top. Microbiol. Immunol. 358, 3–32. doi: 10.1007/82_2012_303
Lescat, M., Hoede, C., Clermont, O., Garry, L., Darlu, P., Tuffery, P., et al. (2009). aes, the gene encoding the esterase B in Escherichia coli, is a powerful phylogenetic marker of the species. BMC Microbiol. 9:273. doi: 10.1186/1471-2180-9-273
Lewis, J. A., and Hatfull, G. F. (2001). Control of directionality in integrase-mediated recombination: examination of recombination directionality factors (RDFs) including Xis and Cox proteins. Nucleic Acids Res. 29, 2205–2216. doi: 10.1093/nar/29.11.2205
Lewis, S. B., Cook, V., Tighe, R., and Schüller, S. (2015). Enterohemorrhagic Escherichia coli colonization of human colonic epithelium in vitro and ex vivo. Infect. Immun. 83, 942–949. doi: 10.1128/IAI.02928-14
Li, J., Tai, C., Deng, Z., Zhong, W., He, Y., and Ou, H.-Y. (2018). VRprofile: gene-cluster-detection-based profiling of virulence and antibiotic resistance traits encoded within genome sequences of pathogenic bacteria. Brief. Bioinformatics 19, 566–574. doi: 10.1093/bib/bbw141
Li, X., Rasko, D. A., Lockatell, C. V., Johnson, D. E., and Mobley, H. L. (2001). Repression of bacterial motility by a novel fimbrial gene product. EMBO J. 20, 4854–4862. doi: 10.1093/emboj/20.17.4854
Lindberg, S., Xia, Y., Sondén, B., Göransson, M., Hacker, J., and Uhlin, B. E. (2008). Regulatory Interactions among adhesin gene systems of uropathogenic Escherichia coli. Infect. Immun. 76, 771–780. doi: 10.1128/IAI.01010-07
Lindberg, U. (1975). Asymptomatic bacteriuria in school girls. V. The clinical course and response to treatment. Acta Paediatr. Scand. 64, 718–724. doi: 10.1111/j.1651-2227.1975.tb03910.x
Lindenthal, C., and Elsinghorst, E. A. (1999). Identification of a glycoprotein produced by enterotoxigenic Escherichia coli. Infect. Immun. 67, 4084–4091.
Lindsey, R. L., Batra, D., Smith, P., Patel, P. N., Tagg, K. A., Garcia-Toledo, L., et al. (2018). PacBio genome sequences of Escherichia coli serotype O157:H7, Diffusely Adherent E. coli, and Salmonella enterica Strains, All Carrying Plasmids with an mcr-1 Resistance Gene. Microbiol. Resour. Announc. 7:e01025-18. doi: 10.1128/MRA.01025-18
Lindstedt, R., Larson, G., Falk, P., Jodal, U., Leffler, H., and Svanborg, C. (1991). The receptor repertoire defines the host range for attaching Escherichia coli strains that recognize globo-A. Infect. Immun. 59, 1086–1092.
Linhartová, I., Bumba, L., Mašín, J., Basler, M., Osièka, R., Kamanová, J., et al. (2010). RTX proteins: a highly diverse family secreted by a common mechanism. FEMS Microbiol. Rev. 34, 1076–1112. doi: 10.1111/j.1574-6976.2010.00231.x
Ljungdahl, M., Lundholm, M., Katouli, M., Rasmussen, I., Engstrand, L., and Haglund, U. (2000). Bacterial translocation in experimental shock is dependent on the strains in the intestinal flora. Scand. J. Gastroenterol. 35, 389–397. doi: 10.1080/003655200750023958
Lloyd, A. L., Henderson, T. A., Vigil, P. D., and Mobley, H. L. T. (2009a). Genomic islands of uropathogenic Escherichia coli contribute to virulence. J. Bacteriol. 191, 3469–3481. doi: 10.1128/JB.01717-08
Lloyd, A. L., Smith, S. N., Eaton, K. A., and Mobley, H. L. T. (2009b). Uropathogenic Escherichia coli Suppresses the host inflammatory response via pathogenicity island genes sisA and sisB. Infect. Immun. 77, 5322–5333. doi: 10.1128/IAI.00779-09
Lo, Y., Zhang, L., Foxman, B., and Zöllner, S. (2015). Whole-genome sequencing of uropathogenic Escherichia coli reveals long evolutionary history of diversity and virulence. Infect. Genet. Evol. 34, 244–250. doi: 10.1016/j.meegid.2015.06.023
Lu, B., and Leong, H. W. (2016). Computational methods for predicting genomic islands in microbial genomes. Comput. Struct. Biotechnol. J. 14, 200–206. doi: 10.1016/j.csbj.2016.05.001
Lucchini, S., Rowley, G., Goldberg, M. D., Hurd, D., Harrison, M., and Hinton, J. C. D. (2006). H-NS mediates the silencing of laterally acquired genes in bacteria. PLoS Pathog. 2:e81. doi: 10.1371/journal.ppat.0020081
Luck, S. N., Turner, S. A., Rajakumar, K., Sakellaris, H., and Adler, B. (2001). Ferric dicitrate transport system (Fec) of Shigella flexneri 2a YSH6000 is encoded on a novel pathogenicity island carrying multiple antibiotic resistance genes. Infect. Immun. 69, 6012–6021. doi: 10.1128/IAI.69.10.6012-6021.2001
Luterbach, C. L., Forsyth, V. S., Engstrom, M. D., and Mobley, H. L. T. (2018). TosR-mediated regulation of adhesins and biofilm formation in uropathogenic Escherichia coli. mSphere 3:e00222-18. doi: 10.1128/mSphere.00222-18
MacFie, J., O’Boyle, C., Mitchell, C. J., Buckley, P. M., Johnstone, D., and Sudworth, P. (1999). Gut origin of sepsis: a prospective study investigating associations between bacterial translocation, gastric microflora, and septic morbidity. Gut 45, 223–228. doi: 10.1136/gut.45.2.223
Mahjoub-Messai, F., Bidet, P., Caro, V., Diancourt, L., Biran, V., Aujard, Y., et al. (2011). Escherichia coli isolates causing bacteremia via gut translocation and urinary tract infection in young infants exhibit different virulence genotypes. J. Infect. Dis. 203, 1844–1849. doi: 10.1093/infdis/jir189
Mammarappallil, J. G., and Elsinghorst, E. A. (2000). Epithelial cell adherence mediated by the enterotoxigenic Escherichia coli tia protein. Infect. Immun. 68, 6595–6601. doi: 10.1128/iai.68.12.6595-6601.2000
Marklund, B. I., Tennent, J. M., Garcia, E., Hamers, A., Båga, M., Lindberg, F., et al. (1992). Horizontal gene transfer of the Escherichia coli pap and prs pili operons as a mechanism for the development of tissue-specific adhesive properties. Mol. Microbiol. 6, 2225–2242. doi: 10.1111/j.1365-2958.1992.tb01399.x
Maroncle, N. M., Sivick, K. E., Brady, R., Stokes, F.-E., and Mobley, H. L. T. (2006). Protease activity, secretion, cell entry, cytotoxicity, and cellular targets of secreted autotransporter toxin of uropathogenic Escherichia coli. Infect. Immun. 74, 6124–6134. doi: 10.1128/IAI.01086-06
Marrs, C. F., Zhang, L., Tallman, P., Manning, S. D., Somsel, P., Raz, P., et al. (2002). Variations in 10 putative uropathogen virulence genes among urinary, faecal and peri-urethral Escherichia coli. J. Med. Microbiol. 51, 138–142. doi: 10.1099/0022-1317-51-2-138
Martin, H. M., Campbell, B. J., Hart, C. A., Mpofu, C., Nayar, M., Singh, R., et al. (2004). Enhanced Escherichia coli adherence and invasion in Crohn’s disease and colon cancer. Gastroenterology 127, 80–93.
Martinez, J. J., Mulvey, M. A., Schilling, J. D., Pinkner, J. S., and Hultgren, S. J. (2000). Type 1 pilus-mediated bacterial invasion of bladder epithelial cells. EMBO J. 19, 2803–2812. doi: 10.1093/emboj/19.12.2803
Martins, F. H., Guth, B. E. C., Piazza, R. M., Leão, S. C., Ludovico, A., Ludovico, M. S., et al. (2015). Diversity of Shiga toxin-producing Escherichia coli in sheep flocks of Paraná State, southern Brazil. Vet. Microbiol. 175, 150–156. doi: 10.1016/j.vetmic.2014.11.003
Masuda, H., Owaribe, K., and Hatano, S. (1983). Contraction of triton-treated culture cells. A calcium-sensitive contractile model. Exp. Cell Res. 143, 79–90. doi: 10.1016/0014-4827(83)90111-8
Mathusa, E. C., Chen, Y., Enache, E., and Hontz, L. (2010). Non-O157 Shiga toxin-producing Escherichia coli in foods. J. Food Prot. 73, 1721–1736. doi: 10.4315/0362-028x-73.9.1721
Mattock, E., and Blocker, A. J. (2017). How do the virulence factors of Shigella work together to cause disease? Front. Cell. Infect. Microbiol. 7:64. doi: 10.3389/fcimb.2017.00064
Maurelli, A. T. (2007). Black holes, antivirulence genes, and gene inactivation in the evolution of bacterial pathogens. FEMS Microbiol. Lett. 267, 1–8. doi: 10.1111/j.1574-6968.2006.00526.x
McCarthy, A. J., Martin, P., Cloup, E., Stabler, R. A., Oswald, E., and Taylor, P. W. (2015). The Genotoxin Colibactin Is a Determinant of Virulence in Escherichia coli K1 Experimental Neonatal Systemic Infection. Infect. Immun. 83, 3704–3711. doi: 10.1128/IAI.00716-15
McClain, M. S., Blomfield, I. C., and Eisenstein, B. I. (1991). Roles of fimB and fimE in site-specific DNA inversion associated with phase variation of type 1 fimbriae in Escherichia coli. J. Bacteriol. 173, 5308–5314. doi: 10.1128/jb.173.17.5308-5314.1991
McClelland, M., Sanderson, K. E., Spieth, J., Clifton, S. W., Latreille, P., Courtney, L., et al. (2001). Complete genome sequence of Salmonella enterica serovar Typhimurium LT2. Nature 413, 852–856. doi: 10.1038/35101614
McDaniel, T. K., Jarvis, K. G., Donnenberg, M. S., and Kaper, J. B. (1995). A genetic locus of enterocyte effacement conserved among diverse enterobacterial pathogens. Proc. Natl. Acad. Sci. U.S.A. 92, 1664–1668. doi: 10.1073/pnas.92.5.1664
McDaniel, T. K., and Kaper, J. B. (1997). A cloned pathogenicity island from enteropathogenic Escherichia coli confers the attaching and effacing phenotype on E. coli K-12. Mol. Microbiol. 23, 399–407. doi: 10.1046/j.1365-2958.1997.2311591.x
McWilliams, B. D., and Torres, A. G. (2014). Enterohemorrhagic Escherichia coli Adhesins. Microbiol. Spectr. 2:EHEC00032013. doi: 10.1128/microbiolspec.EHEC-0003-2013
Mellies, J. L., Navarro-Garcia, F., Okeke, I., Frederickson, J., Nataro, J. P., and Kaper, J. B. (2001). espC pathogenicity island of enteropathogenic Escherichia coli encodes an enterotoxin. Infect. Immun. 69, 315–324. doi: 10.1128/IAI.69.1.315-324.2001
Melton-Celsa, A. R. (2014). Shiga Toxin (Stx) classification, structure, and function. Microbiol. Spectr. 2:EHEC-0024-2013. doi: 10.1128/microbiolspec.EHEC-0024-2013
Messerer, M., Fischer, W., and Schubert, S. (2017). Investigation of horizontal gene transfer of pathogenicity islands in Escherichia coli using next-generation sequencing. PLoS One 12:e0179880. doi: 10.1371/journal.pone.0179880
Michelacci, V., Prosseda, G., Maugliani, A., Tozzoli, R., Sanchez, S., Herrera-León, S., et al. (2016). Characterization of an emergent clone of enteroinvasive Escherichia coli circulating in Europe. Clin. Microbiol. Infect. 22, 287.e11–287.e1. doi: 10.1016/j.cmi.2015.10.025
Michelacci, V., Tozzoli, R., Caprioli, A., Martínez, R., Scheutz, F., Grande, L., et al. (2013). A new pathogenicity island carrying an allelic variant of the Subtilase cytotoxin is common among Shiga toxin producing Escherichia coli of human and ovine origin. Clin. Microbiol. Infect. 19, E149–E156. doi: 10.1111/1469-0691.12122
Middendorf, B., Hochhut, B., Leipold, K., Dobrindt, U., Blum-Oehler, G., and Hacker, J. (2004). Instability of pathogenicity islands in uropathogenic Escherichia coli 536. J. Bacteriol. 186, 3086–3096. doi: 10.1128/jb.186.10.3086-3096.2004
Milkman, R. (1973). Electrophoretic variation in Escherichia coli from natural sources. Science 182, 1024–1026. doi: 10.1126/science.182.4116.1024
Monteiro, R., Ageorges, V., Rojas-Lopez, M., Schmidt, H., Weiss, A., Bertin, Y., et al. (2016). A secretome view of colonisation factors in Shiga toxin-encoding Escherichia coli (STEC): from enterohaemorrhagic E. coli (EHEC) to related enteropathotypes. FEMS Microbiol. Lett. 363:fnw179. doi: 10.1093/femsle/fnw179
Montero, D., Orellana, P., Gutiérrez, D., Araya, D., Salazar, J. C., Prado, V., et al. (2014). Immunoproteomic analysis to identify Shiga toxin-producing Escherichia coli outer membrane proteins expressed during human infection. Infect. Immun. 82, 4767–4777. doi: 10.1128/IAI.02030-14
Montero, D. A., Canto, F. D., Velasco, J., Colello, R., Padola, N. L., Salazar, J. C., et al. (2019). Cumulative acquisition of pathogenicity islands has shaped virulence potential and contributed to the emergence of LEE-negative Shiga toxin-producing Escherichia coli strains. Emerg. Microbes Infect. 8, 486–502. doi: 10.1080/22221751.2019.1595985
Montero, D. A., Velasco, J., Del Canto, F., Puente, J. L., Padola, N. L., Rasko, D. A., et al. (2017). Locus of Adhesion and Autoaggregation (LAA), a pathogenicity island present in emerging Shiga Toxin-producing Escherichia coli strains. Sci. Rep. 7:7011. doi: 10.1038/s41598-017-06999-y
Mora, A., López, C., Dabhi, G., Blanco, M., Blanco, J. E., Alonso, M. P., et al. (2009). Extraintestinal pathogenic Escherichia coli O1:K1:H7/NM from human and avian origin: detection of clonal groups B2 ST95 and D ST59 with different host distribution. BMC Microbiol. 9:132. doi: 10.1186/1471-2180-9-132
Mora, A., López, C., Herrera, A., Viso, S., Mamani, R., Dhabi, G., et al. (2012). Emerging avian pathogenic Escherichia coli strains belonging to clonal groups O111:H4-D-ST2085 and O111:H4-D-ST117 with high virulence-gene content and zoonotic potential. Vet. Microbiol. 156, 347–352. doi: 10.1016/j.vetmic.2011.10.033
Morabito, S., Tozzoli, R., Oswald, E., and Caprioli, A. (2003). A mosaic pathogenicity island made up of the locus of enterocyte effacement and a pathogenicity island of Escherichia coli O157:H7 is frequently present in attaching and effacing E. coli. Infect. Immun. 71, 3343–3348. doi: 10.1128/iai.71.6.3343-3348.2003
Moreno, E., Andreu, A., Pigrau, C., Kuskowski, M. A., Johnson, J. R., and Prats, G. (2008). Relationship between Escherichia coli strains causing acute cystitis in women and the fecal E. coli population of the host. J. Clin. Microbiol. 46, 2529–2534. doi: 10.1128/JCM.00813-08
Moriel, D. G., Bertoldi, I., Spagnuolo, A., Marchi, S., Rosini, R., Nesta, B., et al. (2010). Identification of protective and broadly conserved vaccine antigens from the genome of extraintestinal pathogenic Escherichia coli. Proc. Natl. Acad. Sci. U.S.A. 107, 9072–9077. doi: 10.1073/pnas.0915077107
Moritz, R. L., and Welch, R. A. (2006). The Escherichia coli argW-dsdCXA genetic island is highly variable, and E. coli K1 strains commonly possess two copies of dsdCXA. J. Clin. Microbiol. 44, 4038–4048. doi: 10.1128/JCM.01172-06
Moss, J. E., Cardozo, T. J., Zychlinsky, A., and Groisman, E. A. (1999). The selC-associated SHI-2 pathogenicity island of Shigella flexneri. Mol. Microbiol. 33, 74–83. doi: 10.1046/j.1365-2958.1999.01449.x
Moulin-Schouleur, M., Schouler, C., Tailliez, P., Kao, M.-R., Brée, A., Germon, P., et al. (2006). Common virulence factors and genetic relationships between O18:K1:H7 Escherichia coli isolates of human and avian origin. J. Clin. Microbiol. 44, 3484–3492. doi: 10.1128/JCM.00548-06
Mueller, C. A., Broz, P., and Cornelis, G. R. (2008). The type III secretion system tip complex and translocon. Mol. Microbiol. 68, 1085–1095. doi: 10.1111/j.1365-2958.2008.06237.x
Müller, D., Benz, I., Liebchen, A., Gallitz, I., Karch, H., and Schmidt, M. A. (2009). Comparative analysis of the locus of enterocyte effacement and its flanking regions. Infect. Immun. 77, 3501–3513. doi: 10.1128/IAI.00090-09
Mulvey, M. A., Lopez-Boado, Y. S., Wilson, C. L., Roth, R., Parks, W. C., Heuser, J., et al. (1998). Induction and evasion of host defenses by type 1-piliated uropathogenic Escherichia coli. Science 282, 1494–1497. doi: 10.1126/science.282.5393.1494
Mulvey, M. A., Schilling, J. D., and Hultgren, S. J. (2001). Establishment of a persistent Escherichia coli reservoir during the acute phase of a bladder infection. Infect. Immun. 69, 4572–4579. doi: 10.1128/IAI.69.7.4572-4579.2001
Mulvey, M. A., Schilling, J. D., Martinez, J. J., and Hultgren, S. J. (2000). Bad bugs and beleaguered bladders: interplay between uropathogenic Escherichia coli and innate host defenses. Proc. Natl. Acad. Sci. U.S.A. 97, 8829–8835. doi: 10.1073/pnas.97.16.8829
Mysorekar, I. U., and Hultgren, S. J. (2006). Mechanisms of uropathogenic Escherichia coli persistence and eradication from the urinary tract. Proc. Natl. Acad. Sci. U.S.A. 103, 14170–14175. doi: 10.1073/pnas.0602136103
Nagarjuna, D., Mittal, G., Dhanda, R. S., Verma, P. K., Gaind, R., and Yadav, M. (2015). Faecal Escherichia coli isolates show potential to cause endogenous infection in patients admitted to the ICU in a tertiary care hospital. New Microbes New Infections 7, 57–66. doi: 10.1016/j.nmni.2015.05.006
Nagy, G., Altenhoefer, A., Knapp, O., Maier, E., Dobrindt, U., Blum-Oehler, G., et al. (2006). Both alpha-haemolysin determinants contribute to full virulence of uropathogenic Escherichia coli strain 536. Microbes Infect. 8, 2006–2012. doi: 10.1016/j.micinf.2006.02.029
Napolitano, M. G., Almagro-Moreno, S., and Boyd, E. F. (2011). Dichotomy in the evolution of pathogenicity island and bacteriophage encoded integrases from pathogenic Escherichia coli strains. Infect. Genet. Evol. 11, 423–436. doi: 10.1016/j.meegid.2010.12.003
Nataro, J. P., and Kaper, J. B. (1998). Diarrheagenic Escherichia coli. Clin. Microbiol. Rev. 11, 142–201.
Navarre, W. W., Porwollik, S., Wang, Y., McClelland, M., Rosen, H., Libby, S. J., et al. (2006). Selective silencing of foreign DNA with low GC content by the H-NS protein in Salmonella. Science 313, 236–238. doi: 10.1126/science.1128794
Navarro-Garcia, F. (2014). Escherichia coli O104:H4 pathogenesis: an enteroaggregative E. coli/Shiga Toxin-Producing E. coli explosive cocktail of high virulence. Microbiol. Spectr. 2:EHEC-0008-2013. doi: 10.1128/microbiolspec.EHEC-0008-2013
Navarro-Garcia, F., Serapio-Palacios, A., Vidal, J. E., Salazar, M. I., and Tapia-Pastrana, G. (2014). EspC promotes epithelial cell detachment by enteropathogenic Escherichia coli via sequential cleavages of a cytoskeletal protein and then focal adhesion proteins. Infect. Immun. 82, 2255–2265. doi: 10.1128/IAI.01386-13
Nègre, V. L., Bonacorsi, S., Schubert, S., Bidet, P., Nassif, X., and Bingen, E. (2004). The siderophore receptor IroN, but not the high-pathogenicity island or the hemin receptor ChuA, contributes to the bacteremic step of Escherichia coli neonatal meningitis. Infect. Immun. 72, 1216–1220. doi: 10.1128/iai.72.2.1216-1220.2004
Nesta, B., Spraggon, G., Alteri, C., Moriel, D. G., Rosini, R., Veggi, D., et al. (2012). FdeC, a novel broadly conserved Escherichia coli adhesin eliciting protection against urinary tract infections. mBio 3:e00010-12. doi: 10.1128/mBio.00010-12
Nesta, B., Valeri, M., Spagnuolo, A., Rosini, R., Mora, M., Donato, P., et al. (2014). SslE elicits functional antibodies that impair in vitro mucinase activity and in vivo colonization by both intestinal and extraintestinal Escherichia coli strains. PLoS Pathog. 10:e1004124. doi: 10.1371/journal.ppat.1004124
Nie, H., Yang, F., Zhang, X., Yang, J., Chen, L., Wang, J., et al. (2006). Complete genome sequence of Shigella flexneri 5b and comparison with Shigella flexneri 2a. BMC Genomics 7:173. doi: 10.1186/1471-2164-7-173
Nielubowicz, G. R., and Mobley, H. L. T. (2010). Host-pathogen interactions in urinary tract infection. Nat. Rev. Urol. 7, 430–441. doi: 10.1038/nrurol.2010.101
Nieto, P. A., Pardo-Roa, C., Salazar-Echegarai, F. J., Tobar, H. E., Coronado-Arrázola, I., Riedel, C. A., et al. (2016). New insights about excisable pathogenicity islands in Salmonella and their contribution to virulence. Microbes Infect. 18, 302–309. doi: 10.1016/j.micinf.2016.02.001
Nojoomi, F., and Ghasemian, A. (2019). The relation of phylogroups, serogroups, virulence factors and resistance pattern of Escherichia coli isolated from children with septicemia. New Microbes New Infect 29, 100517. doi: 10.1016/j.nmni.2019.100517
Nougayrède, J.-P., Fernandes, P. J., and Donnenberg, M. S. (2003). Adhesion of enteropathogenic Escherichia coli to host cells. Cell. Microbiol. 5, 359–372. doi: 10.1046/j.1462-5822.2003.00281.x
Nougayrède, J.-P., Homburg, S., Taieb, F., Boury, M., Brzuszkiewicz, E., Gottschalk, G., et al. (2006). Escherichia coli induces DNA double-strand breaks in eukaryotic cells. Science 313, 848–851. doi: 10.1126/science.1127059
Nowrouzian, F. L., and Oswald, E. (2012). Escherichia coli strains with the capacity for long-term persistence in the bowel microbiota carry the potentially genotoxic pks island. Microb. Pathog. 53, 180–182. doi: 10.1016/j.micpath.2012.05.011
Numrych, T. E., Gumport, R. I., and Gardner, J. F. (1992). Characterization of the bacteriophage lambda excisionase (Xis) protein: the C-terminus is required for Xis-integrase cooperativity but not for DNA binding. EMBO J. 11, 3797–3806.
Ochoa, T. J., and Contreras, C. A. (2011). Enteropathogenic escherichia coli infection in children. Curr. Opin. Infect. Dis. 24, 478–483. doi: 10.1097/QCO.0b013e32834a8b8b
Olier, M., Marcq, I., Salvador-Cartier, C., Secher, T., Dobrindt, U., Boury, M., et al. (2012). Genotoxicity of Escherichia coli Nissle 1917 strain cannot be dissociated from its probiotic activity. Gut Microbes 3, 501–509. doi: 10.4161/gmic.21737
Opal, S., Cross, A., and Gemski, P. (1982). K antigen and serum sensitivity of rough Escherichia coli. Infect. Immun. 37, 956–960. doi: 10.1128/IAI.37.3.956-960.1982
Orden, J. A., Horcajo, P., de la Fuente, R., Ruiz-Santa-Quiteria, J. A., Domínguez-Bernal, G., and Carrión, J. (2011). Subtilase cytotoxin-coding genes in verotoxin-producing Escherichia coli strains from sheep and goats differ from those from cattle. Appl. Environ. Microbiol. 77, 8259–8264. doi: 10.1128/AEM.05604-11
O’Shea, Y. A., and Boyd, E. F. (2002). Mobilization of the Vibrio pathogenicity island between Vibrio cholerae isolates mediated by CP-T1 generalized transduction. FEMS Microbiol. Lett. 214, 153–157. doi: 10.1111/j.1574-6968.2002.tb11339.x
Oshima, T., Ishikawa, S., Kurokawa, K., Aiba, H., and Ogasawara, N. (2006). Escherichia coli histone-like protein H-NS preferentially binds to horizontally acquired DNA in association with RNA polymerase. DNA Res. 13, 141–153. doi: 10.1093/dnares/dsl009
Ott, M., Hacker, J., Schmoll, T., Jarchau, T., Korhonen, T. K., and Goebel, W. (1986). Analysis of the genetic determinants coding for the S-fimbrial adhesin (sfa) in different Escherichia coli strains causing meningitis or urinary tract infections. Infect. Immun. 54, 646–653.
Owrangi, B., Masters, N., Kuballa, A., O’Dea, C., Vollmerhausen, T. L., and Katouli, M. (2018). Invasion and translocation of uropathogenic Escherichia coli isolated from urosepsis and patients with community-acquired urinary tract infection. Eur. J. Clin. Microbiol. Infect. Dis. 37, 833–839. doi: 10.1007/s10096-017-3176-4
Palmela, C., Chevarin, C., Xu, Z., Torres, J., Sevrin, G., Hirten, R., et al. (2018). Adherent-invasive Escherichia coli in inflammatory bowel disease. Gut 67, 574–587. doi: 10.1136/gutjnl-2017-314903
Panis, G., Duverger, Y., Courvoisier-Dezord, E., Champ, S., Talla, E., and Ansaldi, M. (2010). Tight regulation of the intS gene of the KplE1 prophage: a new paradigm for integrase gene regulation. PLoS Genet. 6:e1001149. doi: 10.1371/journal.pgen.1001149
Parham, N. J., Pollard, S. J., Chaudhuri, R. R., Beatson, S. A., Desvaux, M., Russell, M. A., et al. (2005a). Prevalence of pathogenicity island IICFT073 genes among extraintestinal clinical isolates of Escherichia coli. J. Clin. Microbiol. 43, 2425–2434. doi: 10.1128/JCM.43.5.2425-2434.2005
Parham, N. J., Pollard, S. J., Desvaux, M., Scott-Tucker, A., Liu, C., Fivian, A., et al. (2005b). Distribution of the serine protease autotransporters of the Enterobacteriaceae among extraintestinal clinical isolates of Escherichia coli. J. Clin. Microbiol. 43, 4076–4082. doi: 10.1128/JCM.43.8.4076-4082.2005
Parham, N. J., Srinivasan, U., Desvaux, M., Foxman, B., Marrs, C. F., and Henderson, I. R. (2004). PicU, a second serine protease autotransporter of uropathogenic Escherichia coli. FEMS Microbiol. Lett. 230, 73–83. doi: 10.1016/S0378-1097(03)00862-0
Parreira, V. R., and Gyles, C. L. (2003). A novel pathogenicity island integrated adjacent to the thrW tRNA gene of avian pathogenic Escherichia coli encodes a vacuolating autotransporter toxin. Infect. Immun. 71, 5087–5096. doi: 10.1128/iai.71.9.5087-5096.2003
Parsot, C. (2005). Shigella spp. and enteroinvasive Escherichia coli pathogenicity factors. FEMS Microbiol. Lett. 252, 11–18. doi: 10.1016/j.femsle.2005.08.046
Pasqua, M., Michelacci, V., Di Martino, M. L., Tozzoli, R., Grossi, M., Colonna, B., et al. (2017). the intriguing evolutionary journey of enteroinvasive E. coli (EIEC) toward Pathogenicity. Front. Microbiol. 8:2390. doi: 10.3389/fmicb.2017.02390
Peng, J., Yang, J., and Jin, Q. (2009). The molecular evolutionary history of Shigella spp. and enteroinvasive Escherichia coli. Infect. Genet. Evol. 9, 147–152. doi: 10.1016/j.meegid.2008.10.003
Perna, N. T., Mayhew, G. F., Pósfai, G., Elliott, S., Donnenberg, M. S., Kaper, J. B., et al. (1998). Molecular evolution of a pathogenicity island from enterohemorrhagic Escherichia coli O157:H7. Infect. Immun. 66, 3810–3817.
Perna, N. T., Plunkett, G., Burland, V., Mau, B., Glasner, J. D., Rose, D. J., et al. (2001). Genome sequence of enterohaemorrhagic Escherichia coli O157:H7. Nature 409, 529–533. doi: 10.1038/35054089
Peterson, J. W., and Whipp, S. C. (1995). Comparison of the mechanisms of action of cholera toxin and the heat-stable enterotoxins of Escherichia coli. Infect. Immun. 63, 1452–1461.
Pettengill, E. A., Pettengill, J. B., and Binet, R. (2015). Phylogenetic analyses of Shigella and enteroinvasive Escherichia coli for the identification of molecular epidemiological markers: whole-genome comparative analysis does not support distinct genera designation. Front. Microbiol. 6:1573. doi: 10.3389/fmicb.2015.01573
Picard, B., Garcia, J. S., Gouriou, S., Duriez, P., Brahimi, N., Bingen, E., et al. (1999). The link between phylogeny and virulence in Escherichia coli extraintestinal infection. Infect. Immun. 67, 546–553.
Platenkamp, A., and Mellies, J. L. (2018). Environment Controls LEE Regulation in Enteropathogenic Escherichia coli. Front. Microbiol. 9:1694. doi: 10.3389/fmicb.2018.01694
Pleguezuelos-Manzano, C., Puschhof, J., Rosendahl Huber, A., van Hoeck, A., Wood, H. M., Nomburg, J., et al. (2020). Mutational signature in colorectal cancer caused by genotoxic pks+ E. coli. Nature 580, 269–273. doi: 10.1038/s41586-020-2080-8
Prager, R., Lang, C., Aurass, P., Fruth, A., Tietze, E., and Flieger, A. (2014). Two novel EHEC/EAEC hybrid strains isolated from human infections. PLoS One 9:e95379. doi: 10.1371/journal.pone.0095379
Prasadarao, N. V., Wass, C. A., Weiser, J. N., Stins, M. F., Huang, S. H., and Kim, K. S. (1996). Outer membrane protein A of Escherichia coli contributes to invasion of brain microvascular endothelial cells. Infect. Immun. 64, 146–153.
Provence, D. L., and Curtiss, R. (1994). Isolation and characterization of a gene involved in hemagglutination by an avian pathogenic Escherichia coli strain. Infect. Immun. 62, 1369–1380.
Purdy, G. E., and Payne, S. M. (2001). The SHI-3 iron transport island of Shigella boydii 0-1392 carries the genes for aerobactin synthesis and transport. J. Bacteriol. 183, 4176–4182. doi: 10.1128/JB.183.14.4176-4182.2001
Putze, J., Hennequin, C., Nougayrède, J.-P., Zhang, W., Homburg, S., Karch, H., et al. (2009). Genetic structure and distribution of the colibactin genomic island among members of the family Enterobacteriaceae. Infect. Immun. 77, 4696–4703. doi: 10.1128/IAI.00522-09
Qin, X., Hu, F., Wu, S., Ye, X., Zhu, D., Zhang, Y., et al. (2013). Comparison of adhesin genes and antimicrobial susceptibilities between uropathogenic and intestinal commensal Escherichia coli strains. PLoS One 8:e61169. doi: 10.1371/journal.pone.0061169
Ragnarsdóttir, B., Fischer, H., Godaly, G., Grönberg-Hernandez, J., Gustafsson, M., Karpman, D., et al. (2008). TLR- and CXCR1-dependent innate immunity: insights into the genetics of urinary tract infections. Eur. J. Clin. Invest. 38, 12–20. doi: 10.1111/j.1365-2362.2008.02004.x
Rahman, S., and Gadjeva, M. (2014). Does NETosis contribute to the bacterial pathoadaptation in cystic fibrosis? Front. Immunol. 5:378. doi: 10.3389/fimmu.2014.00378
Raimondi, S., Righini, L., Candeliere, F., Musmeci, E., Bonvicini, F., Gentilomi, G., et al. (2019). Antibiotic resistance, virulence factors, phenotyping, and genotyping of E. coli Isolated from the Feces of Healthy Subjects. Microorganisms 7:251. doi: 10.3390/microorganisms7080251
Rajakumar, K., Sasakawa, C., and Adler, B. (1996). A spontaneous 99-kb chromosomal deletion results in multi-antibiotic susceptibility and an attenuation of contact haemolysis in Shigella flexneri 2a. J. Med. Microbiol. 45, 64–75. doi: 10.1099/00222615-45-1-64
Rajakumar, K., Sasakawa, C., and Adler, B. (1997). Use of a novel approach, termed island probing, identifies the Shigella flexneri she pathogenicity island which encodes a homolog of the immunoglobulin A protease-like family of proteins. Infect. Immun. 65, 4606–4614.
Rajanna, C., Wang, J., Zhang, D., Xu, Z., Ali, A., Hou, Y.-M., et al. (2003). The vibrio pathogenicity island of epidemic Vibrio cholerae forms precise extrachromosomal circular excision products. J. Bacteriol. 185, 6893–6901. doi: 10.1128/jb.185.23.6893-6901.2003
Rasko, D. A., Rosovitz, M. J., Myers, G. S. A., Mongodin, E. F., Fricke, W. F., Gajer, P., et al. (2008). The pangenome structure of Escherichia coli: comparative genomic analysis of E. coli commensal and pathogenic isolates. J. Bacteriol. 190, 6881–6893. doi: 10.1128/JB.00619-08
Redford, P., and Welch, R. A. (2002). Extraintestinal Escherichia coli as a model system for the study of pathogenicity islands. Curr. Top. Microbiol. Immunol. 264, 15–30.
Reid, S. D., Herbelin, C. J., Bumbaugh, A. C., Selander, R. K., and Whittam, T. S. (2000). Parallel evolution of virulence in pathogenic Escherichia coli. Nature 406, 64–67. doi: 10.1038/35017546
Reigstad, C. S., Hultgren, S. J., and Gordon, J. I. (2007). Functional genomic studies of uropathogenic Escherichia coli and host urothelial cells when intracellular bacterial communities are assembled. J. Biol. Chem. 282, 21259–21267. doi: 10.1074/jbc.M611502200
Reiss, D. J., Howard, F. M., and Mobley, H. L. T. (2012). A novel approach for transcription factor analysis using SELEX with high-throughput sequencing (TFAST). PLoS One 7:e42761. doi: 10.1371/journal.pone.0042761
Reiss, D. J., and Mobley, H. L. T. (2011). Determination of target sequence bound by PapX, repressor of bacterial motility, in flhD promoter using systematic evolution of ligands by exponential enrichment (SELEX) and high throughput sequencing. J. Biol. Chem. 286, 44726–44738. doi: 10.1074/jbc.M111.290684
Reiter, W. D., Palm, P., and Yeats, S. (1989). Transfer RNA genes frequently serve as integration sites for prokaryotic genetic elements. Nucleic Acids Res. 17, 1907–1914. doi: 10.1093/nar/17.5.1907
Rendón, M. A., Saldaña, Z., Erdem, A. L., Monteiro-Neto, V., Vázquez, A., Kaper, J. B., et al. (2007). Commensal and pathogenic Escherichia coli use a common pilus adherence factor for epithelial cell colonization. Proc. Natl. Acad. Sci. U.S.A. 104, 10637–10642. doi: 10.1073/pnas.0704104104
Restieri, C., Garriss, G., Locas, M.-C., and Dozois, C. M. (2007). Autotransporter-encoding sequences are phylogenetically distributed among Escherichia coli clinical isolates and reference strains. Appl. Environ. Microbiol. 73, 1553–1562. doi: 10.1128/AEM.01542-06
Rippere-Lampe, K. E., O’Brien, A. D., Conran, R., and Lockman, H. A. (2001). Mutation of the gene encoding cytotoxic necrotizing factor type 1 (cnf(1)) attenuates the virulence of uropathogenic Escherichia coli. Infect. Immun. 69, 3954–3964. doi: 10.1128/IAI.69.6.3954-3964.2001
Robbins, J. B., Chu, C., and Schneerson, R. (1992). Hypothesis for vaccine development: protective immunity to enteric diseases caused by nontyphoidal Salmonellae and Shigellae may be conferred by serum IgG antibodies to the O-specific polysaccharide of their lipopolysaccharides. Clin. Infect. Dis. 15, 346–361. doi: 10.1093/clinids/15.2.346
Rodrigues, J., Scaletsky, I. C., Campos, L. C., Gomes, T. A., Whittam, T. S., and Trabulsi, L. R. (1996). Clonal structure and virulence factors in strains of Escherichia coli of the classic serogroup O55. Infect. Immun. 64, 2680–2686.
Rodriguez-Siek, K. E., Giddings, C. W., Doetkott, C., Johnson, T. J., Fakhr, M. K., and Nolan, L. K. (2005). Comparison of Escherichia coli isolates implicated in human urinary tract infection and avian colibacillosis. Microbiology 151, 2097–2110. doi: 10.1099/mic.0.27499-0
Rosen, D. A., Hooton, T. M., Stamm, W. E., Humphrey, P. A., and Hultgren, S. J. (2007). Detection of intracellular bacterial communities in human urinary tract infection. PLoS Med. 4:e329. doi: 10.1371/journal.pmed.0040329
Rousset, E., Harel, J., and Dubreuil, J. D. (1998). Sulfatide from the pig jejunum brush border epithelial cell surface is involved in binding of Escherichia coli enterotoxin b. Infect. Immun. 66, 5650–5658.
Ruggenenti, P., and Remuzzi, G. (1998). Pathophysiology and management of thrombotic microangiopathies. J. Nephrol. 11, 300–310.
Ruiz-Perez, F., Wahid, R., Faherty, C. S., Kolappaswamy, K., Rodriguez, L., Santiago, A., et al. (2011). Serine protease autotransporters from Shigella flexneri and pathogenic Escherichia coli target a broad range of leukocyte glycoproteins. Proc. Natl. Acad. Sci. U.S.A. 108, 12881–12886. doi: 10.1073/pnas.1101006108
Rumer, L., Jores, J., Kirsch, P., Cavignac, Y., Zehmke, K., and Wieler, L. H. (2003). Dissemination of pheU- and pheV-located genomic islands among enteropathogenic (EPEC) and enterohemorrhagic (EHEC) E. coli and their possible role in the horizontal transfer of the locus of enterocyte effacement (LEE). Int. J. Med. Microbiol. 292, 463–475. doi: 10.1078/1438-4221-00229
Runyen-Janecky, L., Dazenski, E., Hawkins, S., and Warner, L. (2006). Role and regulation of the Shigella flexneri sit and MntH systems. Infect. Immun. 74, 4666–4672. doi: 10.1128/IAI.00562-06
Russo, T. (2003). Medical and economic impact of extraintestinal infections due to Escherichia coli: focus on an increasingly important endemic problem. Microbes Infect. 5, 449–456. doi: 10.1016/S1286-4579(03)00049-2
Russo, T. A., Carlino, U. B., and Johnson, J. R. (2001). Identification of a new iron-regulated virulence gene, ireA, in an extraintestinal pathogenic isolate of Escherichia coli. Infect. Immun. 69, 6209–6216. doi: 10.1128/IAI.69.10.6209-6216.2001
Russo, T. A., and Johnson, J. R. (2000). Proposal for a new inclusive designation for extraintestinal pathogenic isolates of Escherichia coli: ExPEC. J. Infect. Dis. 181, 1753–1754. doi: 10.1086/315418
Sack, R. B. (2011). The discovery of cholera - like enterotoxins produced by Escherichia coli causing secretory diarrhoea in humans. Indian J. Med. Res. 133, 171–180.
Saile, N., Schuh, E., Semmler, T., Eichhorn, I., Wieler, L. H., Bauwens, A., et al. (2018). Determination of virulence and fitness genes associated with the pheU, pheV and selC integration sites of LEE-negative food-borne Shiga toxin-producing Escherichia coli strains. Gut Pathog. 10:43. doi: 10.1186/s13099-018-0271-8
Sakellaris, H., Luck, S. N., Al-Hasani, K., Rajakumar, K., Turner, S. A., and Adler, B. (2004). Regulated site-specific recombination of the she pathogenicity island of Shigella flexneri. Mol. Microbiol. 52, 1329–1336. doi: 10.1111/j.1365-2958.2004.04048.x
Samuelsson, P., Hang, L., Wullt, B., Irjala, H., and Svanborg, C. (2004). Toll-like receptor 4 expression and cytokine responses in the human urinary tract mucosa. Infect. Immun. 72, 3179–3186. doi: 10.1128/IAI.72.6.3179-3186.2004
Sarff, L. D., McCracken, G. H., Schiffer, M. S., Glode, M. P., Robbins, J. B., Orskov, I., et al. (1975). Epidemiology of Escherichia coli K1 in healthy and diseased newborns. Lancet 1, 1099–1104. doi: 10.1016/s0140-6736(75)92496-4
Sarowska, J., Futoma-Koloch, B., Jama-Kmiecik, A., Frej-Madrzak, M., Ksiazczyk, M., Bugla-Ploskonska, G., et al. (2019). Virulence factors, prevalence and potential transmission of extraintestinal pathogenic Escherichia coli isolated from different sources: recent reports. Gut Pathog. 11:10. doi: 10.1186/s13099-019-0290-0
Scaletsky, I. C., Silva, M. L., and Trabulsi, L. R. (1984). Distinctive patterns of adherence of enteropathogenic Escherichia coli to HeLa cells. Infect. Immun. 45, 534–536.
Schmid, M. B., and Roth, J. R. (1987). Gene location affects expression level in Salmonella typhimurium. J. Bacteriol. 169, 2872–2875. doi: 10.1128/jb.169.6.2872-2875.1987
Schmidt, H., and Hensel, M. (2004). Pathogenicity islands in bacterial pathogenesis. Clin. Microbiol. Rev. 17, 14–56. doi: 10.1128/cmr.17.1.14-56.2004
Schmidt, H., Zhang, W. L., Hemmrich, U., Jelacic, S., Brunder, W., Tarr, P. I., et al. (2001). Identification and characterization of a novel genomic island integrated at selC in locus of enterocyte effacement-negative, Shiga toxin-producing Escherichia coli. Infect. Immun. 69, 6863–6873. doi: 10.1128/IAI.69.11.6863-6873.2001
Schmidt, M. A. (2010). LEEways: tales of EPEC, ATEC and EHEC. Cell. Microbiol. 12, 1544–1552. doi: 10.1111/j.1462-5822.2010.01518.x
Schneider, G., Dobrindt, U., Middendorf, B., Hochhut, B., Szijártó, V., Emody, L., et al. (2011). Mobilisation and remobilisation of a large archetypal pathogenicity island of uropathogenic Escherichia coli in vitro support the role of conjugation for horizontal transfer of genomic islands. BMC Microbiol. 11:210. doi: 10.1186/1471-2180-11-210
Schnupf, P., and Sansonetti, P. J. (2019). Shigella pathogenesis: new insights through advanced methodologies. Microbiol. Spectr. 7:BAI-0023-2019. doi: 10.1128/microbiolspec.BAI-0023-2019
Schubert, S., Cuenca, S., Fischer, D., and Heesemann, J. (2000). High-pathogenicity island of Yersinia pestis in enterobacteriaceae isolated from blood cultures and urine samples: prevalence and functional expression. J. Infect. Dis. 182, 1268–1271. doi: 10.1086/315831
Schubert, S., Darlu, P., Clermont, O., Wieser, A., Magistro, G., Hoffmann, C., et al. (2009). Role of intraspecies recombination in the spread of pathogenicity islands within the Escherichia coli species. PLoS Pathog. 5:e1000257. doi: 10.1371/journal.ppat.1000257
Schubert, S., Dufke, S., Sorsa, J., and Heesemann, J. (2004a). A novel integrative and conjugative element (ICE) of Escherichia coli: the putative progenitor of the Yersinia high-pathogenicity island. Mol. Microbiol. 51, 837–848. doi: 10.1046/j.1365-2958.2003.03870.x
Schubert, S., Nörenberg, D., Clermont, O., Magistro, G., Wieser, A., Romann, E., et al. (2010). Prevalence and phylogenetic history of the TcpC virulence determinant in Escherichia coli. Int. J. Med. Microbiol. 300, 429–434. doi: 10.1016/j.ijmm.2010.02.006
Schubert, S., Picard, B., Gouriou, S., Heesemann, J., and Denamur, E. (2002). Yersinia high-pathogenicity island contributes to virulence in Escherichia coli causing extraintestinal infections. Infect. Immun. 70, 5335–5337. doi: 10.1128/iai.70.9.5335-5337.2002
Schubert, S., Rakin, A., and Heesemann, J. (2004b). The Yersinia high-pathogenicity island (HPI): evolutionary and functional aspects. Int. J. Med. Microbiol. 294, 83–94. doi: 10.1016/j.ijmm.2004.06.026
Schubert, S., Rakin, A., Karch, H., Carniel, E., and Heesemann, J. (1998). Prevalence of the “high-pathogenicity island” of Yersinia species among Escherichia coli strains that are pathogenic to humans. Infect. Immun. 66, 480–485.
Selander, R. K., Musser, J. M., Caugant, D. A., Gilmour, M. N., and Whittam, T. S. (1987). Population genetics of pathogenic bacteria. Microb. Pathog. 3, 1–7. doi: 10.1016/0882-4010(87)90032-5
Serapio-Palacios, A., and Navarro-Garcia, F. (2016). EspC, an autotransporter protein secreted by enteropathogenic Escherichia coli, causes apoptosis and necrosis through caspase and calpain activation, including direct procaspase-3 cleavage. mBio 7:e00479-16. doi: 10.1128/mBio.00479-16
Servin, A. L. (2005). Pathogenesis of Afa/Dr diffusely adhering Escherichia coli. Clin. Microbiol. Rev. 18, 264–292. doi: 10.1128/CMR.18.2.264-292.2005
Servin, A. L. (2014). Pathogenesis of human diffusely adhering Escherichia coli expressing Afa/Dr adhesins (Afa/Dr DAEC): current insights and future challenges. Clin. Microbiol. Rev. 27, 823–869. doi: 10.1128/CMR.00036-14
Shen, S., Mascarenhas, M., Rahn, K., Kaper, J. B., Karmali, M. A., and Karmal, M. A. (2004). Evidence for a hybrid genomic island in verocytotoxin-producing Escherichia coli CL3 (serotype O113:H21) containing segments of EDL933 (serotype O157:H7) O islands 122 and 48. Infect. Immun. 72, 1496–1503. doi: 10.1128/iai.72.3.1496-1503.2004
Silver, R. P., Aaronson, W., Sutton, A., and Schneerson, R. (1980). Comparative analysis of plasmids and some metabolic characteristics of Escherichia coli K1 from diseased and healthy individuals. Infect. Immun. 29, 200–206.
Simms, A. N., and Mobley, H. L. T. (2008). PapX, a P fimbrial operon-encoded inhibitor of motility in uropathogenic Escherichia coli. Infect. Immun. 76, 4833–4841. doi: 10.1128/IAI.00630-08
Skyberg, J. A., Johnson, T. J., Johnson, J. R., Clabots, C., Logue, C. M., and Nolan, L. K. (2006). Acquisition of avian pathogenic Escherichia coli plasmids by a commensal E. coli isolate enhances its abilities to kill chicken embryos, grow in human urine, and colonize the murine kidney. Infect. Immun. 74, 6287–6292. doi: 10.1128/IAI.00363-06
Snyder, J. A., Haugen, B. J., Buckles, E. L., Lockatell, C. V., Johnson, D. E., Donnenberg, M. S., et al. (2004). Transcriptome of uropathogenic Escherichia coli during urinary tract infection. Infect. Immun. 72, 6373–6381. doi: 10.1128/IAI.72.11.6373-6381.2004
Snyder, J. A., Haugen, B. J., Lockatell, C. V., Maroncle, N., Hagan, E. C., Johnson, D. E., et al. (2005). Coordinate expression of fimbriae in uropathogenic Escherichia coli. Infect. Immun. 73, 7588–7596. doi: 10.1128/IAI.73.11.7588-7596.2005
Spangler, B. D. (1992). Structure and function of cholera toxin and the related Escherichia coli heat-labile enterotoxin. Microbiol. Rev. 56, 622–647.
Sperandio, V., Kaper, J. B., Bortolini, M. R., Neves, B. C., Keller, R., and Trabulsi, L. R. (1998). Characterization of the locus of enterocyte effacement (LEE) in different enteropathogenic Escherichia coli (EPEC) and Shiga-toxin producing Escherichia coli (STEC) serotypes. FEMS Microbiol. Lett. 164, 133–139. doi: 10.1111/j.1574-6968.1998.tb13078.x
Spurbeck, R. R., Stapleton, A. E., Johnson, J. R., Walk, S. T., Hooton, T. M., and Mobley, H. L. T. (2011). Fimbrial profiles predict virulence of uropathogenic Escherichia coli strains: contribution of ygi and yad fimbriae. Infect. Immun. 79, 4753–4763. doi: 10.1128/IAI.05621-11
Srinivasan, U., Foxman, B., and Marrs, C. F. (2003). Identification of a gene encoding heat-resistant agglutinin in Escherichia coli as a putative virulence factor in urinary tract infection. J. Clin. Microbiol. 41, 285–289. doi: 10.1128/jcm.41.1.285-289.2003
Stevens, M. P., and Frankel, G. M. (2014). The locus of enterocyte effacement and associated virulence factors of enterohemorrhagic Escherichia coli. Microbiol. Spectr. 2:EHEC-0007-2013. doi: 10.1128/microbiolspec.EHEC-0007-2013<pmid<
Sun, Q., Lan, R., Wang, Y., Wang, J., Wang, Y., Li, P., et al. (2013). Isolation and genomic characterization of SfI, a serotype-converting bacteriophage of Shigella flexneri. BMC Microbiol. 13:39. doi: 10.1186/1471-2180-13-39
Svanborg, C., Bergsten, G., Fischer, H., Frendéus, B., Godaly, G., Gustafsson, E., et al. (2001). The “innate” host response protects and damages the infected urinary tract. Ann. Med. 33, 563–570. doi: 10.3109/07853890109002101
Swenson, D. L., Bukanov, N. O., Berg, D. E., and Welch, R. A. (1996). Two pathogenicity islands in uropathogenic Escherichia coli J96: cosmid cloning and sample sequencing. Infect. Immun. 64, 3736–3743.
Swidsinski, A., Khilkin, M., Kerjaschki, D., Schreiber, S., Ortner, M., Weber, J., et al. (1998). Association between intraepithelial Escherichia coli and colorectal cancer. Gastroenterology 115, 281–286.
Tang, F., Wang, J., Li, D., Gao, S., Ren, J., Ma, L., et al. (2019). Comparative genomic analysis of 127 Escherichia coli strains isolated from domestic animals with diarrhea in China. BMC Genomics 20:212. doi: 10.1186/s12864-019-5588-2
Tapader, R., Basu, S., and Pal, A. (2019). Secreted proteases: a new insight in the pathogenesis of extraintestinal pathogenic Escherichia coli. Int. J. Med. Microbiol. 309, 159–168. doi: 10.1016/j.ijmm.2019.03.002
Tarchouna, M., Ferjani, A., Ben-Selma, W., and Boukadida, J. (2013). Distribution of uropathogenic virulence genes in Escherichia coli isolated from patients with urinary tract infection. Int. J. Infect. Dis. 17, e450–e453. doi: 10.1016/j.ijid.2013.01.025
Tarr, P. I. (2009). Shiga toxin-associated hemolytic uremic syndrome and thrombotic thrombocytopenic purpura: distinct mechanisms of pathogenesis. Kidney Int. Suppl. S29–S32. doi: 10.1038/ki.2008.615
Tarr, P. I., Bilge, S. S., Vary, J. C., Jelacic, S., Habeeb, R. L., Ward, T. R., et al. (2000). Iha: a novel Escherichia coli O157:H7 adherence-conferring molecule encoded on a recently acquired chromosomal island of conserved structure. Infect. Immun. 68, 1400–1407. doi: 10.1128/iai.68.3.1400-1407.2000
Tarr, P. I., Gordon, C. A., and Chandler, W. L. (2005). Shiga-toxin-producing Escherichia coli and haemolytic uraemic syndrome. Lancet 365, 1073–1086. doi: 10.1016/S0140-6736(05)71144-2
Tauschek, M., Strugnell, R. A., and Robins-Browne, R. M. (2002). Characterization and evidence of mobilization of the LEE pathogenicity island of rabbit-specific strains of enteropathogenic Escherichia coli. Mol. Microbiol. 44, 1533–1550. doi: 10.1046/j.1365-2958.2002.02968.x
Taylor, D. E., Rooker, M., Keelan, M., Ng, L.-K., Martin, I., Perna, N. T., et al. (2002). Genomic variability of O islands encoding tellurite resistance in enterohemorrhagic Escherichia coli O157:H7 isolates. J. Bacteriol. 184, 4690–4698. doi: 10.1128/jb.184.17.4690-4698.2002
Tenaillon, O., Skurnik, D., Picard, B., and Denamur, E. (2010). The population genetics of commensal Escherichia coli. Nat. Rev. Microbiol. 8, 207–217. doi: 10.1038/nrmicro2298
Teng, C.-H., Cai, M., Shin, S., Xie, Y., Kim, K.-J., Khan, N. A., et al. (2005). Escherichia coli K1 RS218 interacts with human brain microvascular endothelial cells via type 1 fimbria bacteria in the fimbriated state. Infect. Immun. 73, 2923–2931. doi: 10.1128/IAI.73.5.2923-2931.2005
Tivendale, K. A., Logue, C. M., Kariyawasam, S., Jordan, D., Hussein, A., Li, G., et al. (2010). Avian-pathogenic Escherichia coli strains are similar to neonatal meningitis E. coli strains and are able to cause meningitis in the rat model of human disease. Infect. Immun. 78, 3412–3419. doi: 10.1128/IAI.00347-10
Torres, A. G. (2004). Current aspects of Shigella pathogenesis. Rev. Latinoam. Microbiol. 46, 89–97.
Touchon, M., Hoede, C., Tenaillon, O., Barbe, V., Baeriswyl, S., Bidet, P., et al. (2009). Organised genome dynamics in the Escherichia coli species results in highly diverse adaptive paths. PLoS Genet. 5:e1000344. doi: 10.1371/journal.pgen.1000344
Tourret, J., and Denamur, E. (2016). “Population phylogenomics of extraintestinal pathogenic Escherichia coli,” in Urinary Tract Infections: Molecular Pathogenesis and Clinical Management, 2nd Edn, eds M. Mulvey, D. Klumpp, and A. Stapleton, (Washington, DC: ASM Press), 207–233. doi: 10.1128/microbiolspec.UTI-0010-2012
Tourret, J., Diard, M., Garry, L., Matic, I., and Denamur, E. (2010). Effects of single and multiple pathogenicity island deletions on uropathogenic Escherichia coli strain 536 intrinsic extra-intestinal virulence. Int. J. Med. Microbiol. 300, 435–439. doi: 10.1016/j.ijmm.2010.04.013
Trabulsi, L. R., Keller, R., and Tardelli Gomes, T. A. (2002). Typical and atypical enteropathogenic Escherichia coli. Emerg. Infect. Dis. 8, 508–513. doi: 10.3201/eid0805.010385
Turner, N. C. A., Connolly, J. P. R., and Roe, A. J. (2019). Control freaks-signals and cues governing the regulation of virulence in attaching and effacing pathogens. Biochem. Soc. Trans. 47, 229–238. doi: 10.1042/BST20180546
Turner, S. A., Luck, S. N., Sakellaris, H., Rajakumar, K., and Adler, B. (2001). Nested deletions of the SRL pathogenicity island of Shigella flexneri 2a. J. Bacteriol. 183, 5535–5543. doi: 10.1128/JB.183.19.5535-5543.2001
Turner, S. A., Luck, S. N., Sakellaris, H., Rajakumar, K., and Adler, B. (2003). Molecular epidemiology of the SRL pathogenicity island. Antimicrob. Agents Chemother. 47, 727–734. doi: 10.1128/aac.47.2.727-734.2003
Turner, S. M., Chaudhuri, R. R., Jiang, Z.-D., DuPont, H., Gyles, C., Penn, C. W., et al. (2006a). Phylogenetic comparisons reveal multiple acquisitions of the toxin genes by enterotoxigenic Escherichia coli strains of different evolutionary lineages. J. Clin. Microbiol. 44, 4528–4536. doi: 10.1128/JCM.01474-06
Turner, S. M., Scott-Tucker, A., Cooper, L. M., and Henderson, I. R. (2006b). Weapons of mass destruction: virulence factors of the global killer enterotoxigenic Escherichia coli. FEMS Microbiol. Lett. 263, 10–20. doi: 10.1111/j.1574-6968.2006.00401.x
Uhlén, P., Laestadius, A., Jahnukainen, T., Söderblom, T., Bäckhed, F., Celsi, G., et al. (2000). Alpha-haemolysin of uropathogenic E. coli induces Ca2+ oscillations in renal epithelial cells. Nature 405, 694–697. doi: 10.1038/35015091
Ulett, G. C., Valle, J., Beloin, C., Sherlock, O., Ghigo, J.-M., and Schembri, M. A. (2007). Functional analysis of antigen 43 in uropathogenic Escherichia coli reveals a role in long-term persistence in the urinary tract. Infect. Immun. 75, 3233–3244. doi: 10.1128/IAI.01952-06
Vaandrager, A. B. (2002). Structure and function of the heat-stable enterotoxin receptor/guanylyl cyclase C. Mol. Cell. Biochem. 230, 73–83.
Valle, J., Mabbett, A. N., Ulett, G. C., Toledo-Arana, A., Wecker, K., Totsika, M., et al. (2008). UpaG, a new member of the trimeric autotransporter family of adhesins in uropathogenic Escherichia coli. J. Bacteriol. 190, 4147–4161. doi: 10.1128/JB.00122-08
Van den Bosch, L., Manning, P. A., and Morona, R. (1997). Regulation of O-antigen chain length is required for Shigella flexneri virulence. Mol. Microbiol. 23, 765–775. doi: 10.1046/j.1365-2958.1997.2541625.x
Vejborg, R. M., Hancock, V., Schembri, M. A., and Klemm, P. (2011). Comparative genomics of Escherichia coli strains causing urinary tract infections. Appl. Environ. Microbiol. 77, 3268–3278. doi: 10.1128/AEM.02970-10
Vigil, P. D., Alteri, C. J., and Mobley, H. L. T. (2011a). Identification of in vivo-induced antigens including an RTX family exoprotein required for uropathogenic Escherichia coli virulence. Infect. Immun. 79, 2335–2344. doi: 10.1128/IAI.00110-11
Vigil, P. D., Stapleton, A. E., Johnson, J. R., Hooton, T. M., Hodges, A. P., He, Y., et al. (2011b). Presence of putative repeat-in-toxin gene tosA in Escherichia coli predicts successful colonization of the urinary tract. mBio 2:e00066-11. doi: 10.1128/mBio.00066-11
Vigil, P. D., Wiles, T. J., Engstrom, M. D., Prasov, L., Mulvey, M. A., and Mobley, H. L. T. (2012). The repeat-in-toxin family member TosA mediates adherence of uropathogenic Escherichia coli and survival during bacteremia. Infect. Immun. 80, 493–505. doi: 10.1128/IAI.05713-11
Vokes, S. A., Reeves, S. A., Torres, A. G., and Payne, S. M. (1999). The aerobactin iron transport system genes in Shigella flexneri are present within a pathogenicity island. Mol. Microbiol. 33, 63–73. doi: 10.1046/j.1365-2958.1999.01448.x
Vollmerhausen, T. L., Woods, J. L., Faoagali, J., and Katouli, M. (2014). Interactions of uroseptic Escherichia coli with renal (A-498) and gastrointestinal (HT-29) cell lines. J. Med. Microbiol. 63, 1575–1583. doi: 10.1099/jmm.0.076562-0
Wada, H., Matsumoto, T., and Yamashita, Y. (2014). Natural history of thrombotic thrombocytopenic purpura and hemolytic uremic syndrome. Semin. Thromb. Hemost. 40, 866–873. doi: 10.1055/s-0034-1395154
Wang, H., Paton, J. C., and Paton, A. W. (2007). Pathologic changes in mice induced by subtilase cytotoxin, a potent new Escherichia coli AB5 toxin that targets the endoplasmic reticulum. J. Infect. Dis. 196, 1093–1101. doi: 10.1086/521364
Wang, L., Wakushima, M., Aota, T., Yoshida, Y., Kita, T., Maehara, T., et al. (2013). Specific properties of enteropathogenic Escherichia coli isolates from diarrheal patients and comparison to strains from foods and fecal specimens from cattle, swine, and healthy carriers in Osaka City. Japan. Appl. Environ. Microbiol. 79, 1232–1240. doi: 10.1128/AEM.03380-12
Wang, Y., Huang, S. H., Wass, C. A., Stins, M. F., and Kim, K. S. (1999). The gene locus yijP contributes to Escherichia coli K1 invasion of brain microvascular endothelial cells. Infect. Immun. 67, 4751–4756.
Welch, R. A. (1991). Pore-forming cytolysins of Gram-negative bacteria. Mol. Microbiol. 5, 521–528. doi: 10.1111/j.1365-2958.1991.tb00723.x
Whitfield, C. (2006). Biosynthesis and assembly of capsular polysaccharides in Escherichia coli. Annu. Rev. Biochem. 75, 39–68. doi: 10.1146/annurev.biochem.75.103004.142545
Wickham, M. E., Lupp, C., Mascarenhas, M., Vazquez, A., Coombes, B. K., Brown, N. F., et al. (2006). Bacterial genetic determinants of non-O157 STEC outbreaks and hemolytic-uremic syndrome after infection. J. Infect. Dis. 194, 819–827. doi: 10.1086/506620
Wijetunge, D. S. S., Gongati, S., DebRoy, C., Kim, K. S., Couraud, P. O., Romero, I. A., et al. (2015). Characterizing the pathotype of neonatal meningitis causing Escherichia coli (NMEC). BMC Microbiol. 15:211. doi: 10.1186/s12866-015-0547-9
Wilde, C., Mazel, D., Hochhut, B., Middendorf, B., Le Roux, F., Carniel, E., et al. (2008). Delineation of the recombination sites necessary for integration of pathogenicity islands II and III into the Escherichia coli 536 chromosome. Mol. Microbiol. 68, 139–151. doi: 10.1111/j.1365-2958.2008.06145.x
Wiles, T. J., Kulesus, R. R., and Mulvey, M. A. (2008). Origins and virulence mechanisms of uropathogenic Escherichia coli. Exp. Mol. Pathol. 85, 11–19. doi: 10.1016/j.yexmp.2008.03.007
Williams, K. P. (2003). Traffic at the tmRNA gene. J. Bacteriol. 185, 1059–1070. doi: 10.1128/jb.185.3.1059-1070.2003
Wolfson, J. J., May, K. L., Thorpe, C. M., Jandhyala, D. M., Paton, J. C., and Paton, A. W. (2008). Subtilase cytotoxin activates PERK, IRE1 and ATF6 endoplasmic reticulum stress-signalling pathways. Cell. Microbiol. 10, 1775–1786. doi: 10.1111/j.1462-5822.2008.01164.x
Wright, K. J., Seed, P. C., and Hultgren, S. J. (2007). Development of intracellular bacterial communities of uropathogenic Escherichia coli depends on type 1 pili. Cell. Microbiol. 9, 2230–2241. doi: 10.1111/j.1462-5822.2007.00952.x
Xia, Y., Forsman, K., Jass, J., and Uhlin, B. E. (1998). Oligomeric interaction of the PapB transcriptional regulator with the upstream activating region of pili adhesin gene promoters in Escherichia coli. Mol. Microbiol. 30, 513–523. doi: 10.1046/j.1365-2958.1998.01080.x
Xie, Y., Kolisnychenko, V., Paul-Satyaseela, M., Elliott, S., Parthasarathy, G., Yao, Y., et al. (2006). Identification and characterization of Escherichia coli RS218–derived islands in the pathogenesis of E. coli Meningitis. J. Infect. Dis. 194, 358–364. doi: 10.1086/505429
Xu, W.-Y., Li, Y.-J., and Fan, C. (2018). Different loci and mRNA copy number of the increased serum survival gene of Escherichia coli. Can. J. Microbiol. 64, 147–154. doi: 10.1139/cjm-2017-0363
Yahiro, K., Tsutsuki, H., Ogura, K., Nagasawa, S., Moss, J., and Noda, M. (2012). Regulation of subtilase cytotoxin-induced cell death by an RNA-dependent protein kinase-like endoplasmic reticulum kinase-dependent proteasome pathway in HeLa cells. Infect. Immun. 80, 1803–1814. doi: 10.1128/IAI.06164-11
Yang, F., Yang, J., Zhang, X., Chen, L., Jiang, Y., Yan, Y., et al. (2005). Genome dynamics and diversity of Shigella species, the etiologic agents of bacillary dysentery. Nucleic Acids Res. 33, 6445–6458. doi: 10.1093/nar/gki954
Yin, X., Wheatcroft, R., Chambers, J. R., Liu, B., Zhu, J., and Gyles, C. L. (2009). Contributions of O island 48 to adherence of enterohemorrhagic Escherichia coli O157:H7 to epithelial cells in vitro and in ligated pig ileal loops. Appl. Environ. Microbiol. 75, 5779–5786. doi: 10.1128/AEM.00507-09
Yoon, S. H., Park, Y.-K., and Kim, J. F. (2015). PAIDB v2.0: exploration and analysis of pathogenicity and resistance islands. Nucleic Acids Res. 43, D624–D630. doi: 10.1093/nar/gku985
Zagaglia, C., Casalino, M., Colonna, B., Conti, C., Calconi, A., and Nicoletti, M. (1991). Virulence plasmids of enteroinvasive Escherichia coli and Shigella flexneri integrate into a specific site on the host chromosome: integration greatly reduces expression of plasmid-carried virulence genes. Infect. Immun. 59, 792–799.
Zhao, W.-D., Liu, D.-X., Wei, J.-Y., Miao, Z.-W., Zhang, K., Su, Z.-K., et al. (2018). Caspr1 is a host receptor for meningitis-causing Escherichia coli. Nat. Commun. 9:2296. doi: 10.1038/s41467-018-04637-3
Keywords: Escherichia coli, pathogenicity islands, genomic island, virulence, ExPEC, AIEC, InPEC
Citation: Desvaux M, Dalmasso G, Beyrouthy R, Barnich N, Delmas J and Bonnet R (2020) Pathogenicity Factors of Genomic Islands in Intestinal and Extraintestinal Escherichia coli. Front. Microbiol. 11:2065. doi: 10.3389/fmicb.2020.02065
Received: 04 May 2020; Accepted: 05 August 2020;
Published: 25 September 2020.
Edited by:
Philippe Lehours, Université Bordeaux Segalen, FranceReviewed by:
David W. Ussery, University of Arkansas for Medical Sciences, United StatesSusan M. Bueno, Pontificia Universidad Católica de Chile, Chile
Copyright © 2020 Desvaux, Dalmasso, Beyrouthy, Barnich, Delmas and Bonnet. This is an open-access article distributed under the terms of the Creative Commons Attribution License (CC BY). The use, distribution or reproduction in other forums is permitted, provided the original author(s) and the copyright owner(s) are credited and that the original publication in this journal is cited, in accordance with accepted academic practice. No use, distribution or reproduction is permitted which does not comply with these terms.
*Correspondence: Richard Bonnet, cmJvbm5ldEBjaHUtY2xlcm1vbnRmZXJyYW5kLmZy