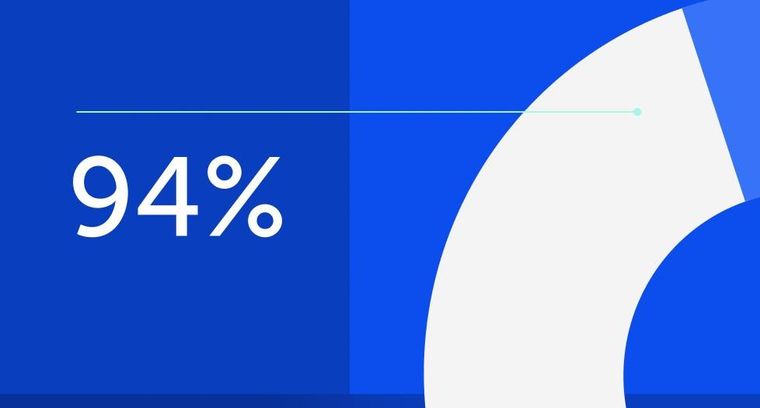
94% of researchers rate our articles as excellent or good
Learn more about the work of our research integrity team to safeguard the quality of each article we publish.
Find out more
ORIGINAL RESEARCH article
Front. Microbiol., 07 August 2020
Sec. Plant Pathogen Interactions
Volume 11 - 2020 | https://doi.org/10.3389/fmicb.2020.01874
This article is part of the Research TopicPlant Root Interaction with Associated Microbiomes to Improve Plant Resiliency and Crop BiodiversityView all 34 articles
Biocontrol bacteria can be used for plant protection against some plant diseases. Pseudomonas chlororaphis PCL1606 (PcPCL1606) is a model bacterium isolated from the avocado rhizosphere with strong antifungal antagonism mediated by the production of 2-hexyl, 5-propil resorcinol (HPR). Additionally, PcPCL1606 has biological control against different soil-borne fungal pathogens, including the causal agent of the white root rot of many woody crops and avocado in the Mediterranean area, Rosellinia necatrix. The objective of this study was to assess whether the semicommercial application of PcPCL1606 to soil can potentially affect avocado soil and rhizosphere microbial communities and their activities in natural conditions and under R. necatrix infection. To test the putative effects of PcPCL1606 on soil eukaryotic and prokaryotic communities, a formulated PcPCL1606 was prepared and applied to the soil of avocado plants growing in mesocosm experiments, and the communities were analyzed by using 16S/ITS metagenomics. PcPCL1606 survived until the end of the experiments. The effect of PcPCL1606 application on prokaryotic communities in soil and rhizosphere samples from natural soil was not detectable, and very minor changes were observed in eukaryotic communities. In the infested soils, the presence of R. necatrix strongly impacted the soil and rhizosphere microbial communities. However, after PcPCL1606 was applied to soil infested with R. necatrix, the prokaryotic community reacted by increasing the relative abundance of few families with protective features against fungal soilborne pathogens and organic matter decomposition (Chitinophagaceae, Cytophagaceae), but no new prokaryotic families were detected. The treatment of PcPCL1606 impacted the fungal profile, which strongly reduced the presence of R. necatrix in avocado soil and rhizosphere, minimizing its effect on the rest of the microbial communities. The bacterial treatment of formulated PcPCL1606 on avocado soils infested with R. necatrix resulted in biological control of the pathogen. This suppressiveness phenotype was analyzed, and PcPCL1606 has a key role in suppressiveness induction; in addition, this phenotype was strongly dependent on the production of HPR.
Soil is a complex and variable habitats on earth. Soil organisms have developed different mechanisms to survive, function and replicate into a changing environment, with variable moisture, temperature, and chemical contents. Soil conditions can vary in very short distances, but also there is variability over time; therefore, soil organisms must be able to adapt rapidly to different and changing conditions (Thies and Grossman, 2006). Additionally, most of the upper layer of the soils are under the influence of plant roots. Thus, the plant rhizosphere was previously defined as the zone around the root where microorganisms and processes important for plant growth and health are located (Hiltner, 1904). Rhizosphere soil a kind of layer between roots and the surrounding soil, that takes part in the large fluxes of nutrients and non-nutrient compounds (Belnap et al., 2003). Moreover, plant rhizosphere provides a special habitat that promotes higher microbial growth, abundance, and diversity (Praeg et al., 2019).
It is well known that in soil ecosystems, the establishment of plants helps to stabilize microbial community structures and are further modulated after interactions with the plant rhizospheres (Donhauser and Frey, 2018). Plant rhizospheres can be colonized by a high number of microorganisms, reaching cell numbers higher than the number of plant cells, covering between 7% and 15% of the rhizoplane (Gray and Smith, 2005). Plant roots photosynthetically fix carbon, and deposit this carbon directly into their surroundings. These exudates can be used as nutrients by the microbial community, finally influencing their composition and activities (Raaijmakers et al., 2009; Berendsen et al., 2012; Vorholt, 2012).
Soil microorganisms interacts with plant roots and interfere with plant behavior and microbial communities. Many rhizosphere-associated microorganisms can modify seed germination, seedling vigor, plant growth and development, nutrition, diseases, and productivity (Berg et al., 2016). Among them, soilborne plant pathogens are the major limitation in plant production. This group of pathogens is adapted to live in bulk soil; however, the rhizosphere is the place where the pathogen meets the plant and initiates the infection (Raaijmakers et al., 2009). This is also where the complex rhizosphere community of microorganisms can interact with the pathogen and influence the outcome of pathogen infection. Among these microbes, some bacteria positively affect plants and can be considered beneficial bacteria, many of which are designated plant growth-promoting rhizobacteria (PGPR; Lugtenberg and Kamilova, 2009). PGPR can promote beneficial effects on plants. Indirectly, they can inhibit pathogens through competition, colonizing the rhizoplane, inducing plant resistance, and solubilizing minerals, which can cause a modification in the rhizosphere. Directly, PGPR can release antifungal compounds and lytic enzymes (Lugtenberg and Kamilova, 2009).
The increase in knowledge on plant beneficial bacteria has prompted interest in the biological control of plant diseases, which has increased recently because the use of chemicals in the environment provoke public concerns, aiming to the need of finding alternatives to the chemicals used for disease control. Historically, strains with biocontrol potential have been isolated from suppressive soils, studied and used against different soil pathogens (Köhl et al., 2019). Successful, reproducible biological control requires knowledge on the interactions at the root environments, in order to understand the conditions where biocontrol can be obtained (Deacon, 1994; Whipps, 1997) and, indeed, may be part of the reason why more biocontrol agents are reaching the market-place (Whipps and Davies, 2000; Whipps and Lumsden, 2001). The beneficial microorganisms must be mass produced and applied to the crops in a way that optimizes their activities in the corresponding habitat. Microbes can be delivered under different ways, including as liquids (sprays, drenches, and root dips) or as dry formulations applied in-furrow at the time of planting (O’Callaghan, 2016). Several biological control agents (BCAs), composed by living microbial products have been commercialized. A few examples of PGPR developed as commercial products for biological control are Bacillus subtilis GBO3 (Kodiak®; Gustafson Inc., Dallas, TX, United States), Pseudomonas fluorescens A506 (BlightBan®; Nufarm Americas, Burr Ridge, IL, United States), Pseudomonas. aureofaciens Tx-1 (Spot-Less®; Eco Soil Systems Inc., San Diego, CA, United States), P. syringae ESC-10, and ESC-11 (Bio-save®; Jet Harvest Solutions, Longwood, FL, United States), Streptomyces griseoviridis K61 (Mycostop®; Verdera Oy, Espoo, Finland), and S. lydicus WYEC 108 (Actinovate®; Novozymes BioAg Inc. WI, United States) (Figueiredo et al., 2010; Dey et al., 2014).
Among the bacterial biocontrol agents reported, the group of Pseudomonas spp. have been extensively studied due to its colonizing ability, inducing plant systemic resistance and to produce antifungal compounds (Haas and Défago, 2005; Weller, 2007). The antifungal compounds produced by rhizospheric Pseudomonas spp. are usually the basis for its biological effectiveness and include phenazine-1-carboxylic acid (PCA), phenazine carboxamide (PCN), 2,4-diacetylphloroglucinol (DAPG), pyrrolnitrin (PRN), pyoluteorin (PLT), 2-hexyl 5-propyl resorcinol (HPR), hydrogen cyanide (HCN), siderophores, and some hydrolytic enzymes such as proteases (Ligon et al., 2000; Raaijmakers et al., 2002; Cazorla et al., 2006). Among the antifungal pseudomonads commonly isolated from soil and rhizosphere, Pseudomonas chlororaphis is a root-associated bacterial species that displays a wide weaponry of antifungals and shows efficient colonizing abilities (Arrebola et al., 2019; Biessy et al., 2019). Due to these characteristics, commercial or formulated P. chlororaphis and related species have been developed to fight soil diseases, especially those due to pathogenic fungi; for example, the commercial product Cerall®, composed of the strain P. chlororaphis MA342, which shows biological control against phytopathogenic fungi in wheat, rye, and triticale, such as Fusarium and Septoria (Koppert Biological Systems, Netherlands). Other examples of commercial products based on P. chlororaphis and related strains are Cedomon® (P. chlororaphis MA 342, BioAgri AB, Sweden), Spot-Less® (P. aureofaciens Tx-1, Turf Science Laboratories, Carlsbad, CA, United States) or AtEze® (P. chlororaphis 63-28, Turf Science Laboratories, Carlsbad, CA, United States). These formulated Pseudomonas spp. have additional activities since they can also be used as bioinsecticides (Kupferschmied et al., 2013) or as rhizobacteria with plant growth-promoting activity (Chen et al., 2015).
Although Pseudomonas spp. are widely assayed against different soil diseases, few studies have analyzed the effect of Pseudomonas spp. on non-target soil microbial populations. For instance, in barley plants, a transient modification of 3 weeks were observed after Pseudomonas spp. DSMZ13134 application (Buddrus-Schiemann et al., 2010). In cucumber, after the application of P. fluorescens 2P24 and CPF10, no differences in the bacterial population structure compared to the control were observed after 8 weeks (Yin et al., 2013). On the other hand, in lettuce, the impact of Pseudomonas jessenii RU47 on the rhizosphere microbiota was influenced by the soil type 2 or 3 weeks after treatment (Schreiter et al., 2014). Furthermore, all available literature is mainly related to the effect in herbaceous plants, but there is no available information on the effect of Pseudomonas application on soil and rhizospheric microbial populations in woody crops.
Rosellinia necatrix causes white root rot, a devastating disease in woody plants worldwide (Pliego et al., 2012). Since the 1990s, different studies have established the importance of this disease in avocado (Persea Americana Mill.) crops in the Mediterranean area (López-Herrera et al., 1998, 1999). However, the control of avocado white root rot is considered very complex; therefore, several studies have focused on microbial species with the ability to control R. necatrix as additional tools to help manage this disease in the future (Cazorla et al., 2006; Ruano-Rosa et al., 2010). Different strategies have been followed to obtain bacterial isolates with biocontrol potential of R. necatrix. Pseudomonas spp. and Bacillus spp. are commonly isolated from avocado soil and rhizosphere, and some of these strains show antifungal activity and plant protection against soilborne fungal pathogens (Cazorla et al., 2006, 2007; González-Sánchez et al., 2010). Additionally, previous results reported the development of suppressiveness-induced soil after the amendment of commercial soil with composted almond shells in avocado orchards. Induced suppressiveness was directly related to the increase in abundance of specific members of Gammaproteobacteria (including a group of Pseudomonas spp. producing antifungal compounds; Vida et al., 2016). These results increased the interest in P. chlororaphis strains as potential BCAs against different avocado phytopathogens (Cazorla et al., 2006; Pliego et al., 2008; Arrebola et al., 2019).
In the present study, the model biocontrol rhizobacterium Pseudomonas chlororaphis PCL1606 (PcPCL1606) was used. This bacterium showed strong antagonism against various phytopathogenic fungi (including R. necatrix) and displayed biocontrol against Fusarium oxysporum f. sp. radicis-lycopersici and R. necatrix (Cazorla et al., 2006; González-Sánchez et al., 2013). The production of the antifungal compound HPR is directly related to the effectiveness of biocontrol and antagonistic activity (Cazorla et al., 2006; Calderón et al., 2013). Furthermore, PcPCL1606 showed additional characteristics related to its fitness in soil and plant roots, such as efficient plant root colonization (González-Sánchez et al., 2010), increased fungal stress symptoms on hyphae after cell-to-cell contact, accelerated hyphal death (Calderón et al., 2014), survival in soil and potential competitiveness with other bacteria associated with the rhizosphere, as PcPCL1606 can produce two recently described bacteriocins (Dorosky et al., 2017).
The objective of this work was to elucidate the impact of PcPCL1606 applications in native communities of soil and rhizosphere, and to unravel the key role of PcPCL1606 applications in soil inducing suppressiveness against Rosellinia necatrix.
Rhizospheric P. chlororaphis PCL1606 (PcPCL1606; NCBI complete genome accession number GCA_000963835.1) isolated from healthy avocado roots of trees growing in a R. necatrix infested area (Cazorla et al., 2006) was used as model pathogen in this study (Table 1). Additionally, a previously obtained Gfp-tagged derivative PcPCL1606 strain (PcPCL1606-GFP, resistant to gentamycin at 80 μg/ml; Calderón et al., 2014) was used as a control to assess survival features (Table 1). To test the role of PcPCL1606 in suppressiveness, a derivative deletion mutant in the darB gene (ΔdarB), impaired in antagonism and biocontrol, was used (Calderón et al., 2019).
Tryptone-peptone-glycerol (TPG; Calderón et al., 2014) medium was used to routinely grow Pseudomonas strains at 25°C. Agar–agar (Difco Laboratories, Detroit, MI) was added to a final concentration of 1.5% to produce solid media. The isolates were maintained as glycerol (30%) stocks at −80°C and revived on TPG, and a single colony was used to inoculate each culture.
In this work, a semi-commercial formulated product based on PcPCL1606 was used for experimentation. To obtain a formulated PcPCL1606 product, a standard fermentation of the biologically active product (PcPCL1606) was performed by Koppert B.V. (Berkel en Rodenrijs, Netherlands). Briefly, TPG culture medium was used, and a 3-liter bioreactor was inoculated with a starter culture of PcPCL1606. The culture was grown for 24 h at pH 7.0 and 25°C. The fermentation parameters (oxygen supply and pH) were controlled throughout the fermentation procedure. Antifoam was required during the fermentation process. The fermentation product was harvested 2 to 3 h after the measured oxygen consumption indicated a shift in secondary metabolism (approximately 24 h of growth). Finally, the fermentation product was formulated in a suspension concentrate, comprising cells of the isolate in TPG medium (approximately 5 × 109 colony forming units (cfu)/ml) and was stored at 4°C until its utilization.
For biocontrol and suppressiveness assays, virulent avocado pathogenic R. necatrix CH53 was used (López-Herrera and Zea-Bonilla, 2007; Pliego et al., 2009; Table 1). The fungus was grown at 25°C on potato dextrose agar (PDA; Difco Laboratories, Detroit, MI) or TPG plates, and the fungal strain was stored in TPG at 4°C as previously described (Gutiérrez-Barranquero et al., 2012).
Two independent 1-year-long mesocosm experiments were conducted as previously described (Bonilla et al., 2015) to perform disease assessment, bacterial survival and metagenomic analysis. The independent experiments were named “assay 1” (season 2017/18) and “assay 2” (season 2018/19). These assays started 120 days before R. necatrix was inoculated (which was considered day 0) and ended 110 days after pathogen inoculation (the experiments last 230 days in total; Figure 1).
Figure 1. Experimental design of the mesocosm assays. Two independent experiments were performed, treatments in each independent experiment are detailed in Table 2. Seventeen independent mesocosms were used for each of the treatments assayed (eleven mesocosms were inoculated with R. necatrix, and six mesocosms remained non-inoculated). (A) Schematic view of the mesocosms timeline. Experiments started 120 days (–120 days) prior to the R. necatrix inoculation (considered as day 0). One hundred and two 2 years-old avocado plants were sown in commercial soil. At this time started the treatments with the positive control, amending composted almond shells to the corresponding pots. At –50 days, the preventive treatment with PcPCL1606 and PcPCL1606-GFP were applied. 70 days after R. necatrix inoculation, the curative PcPCL1606 treatment was applied. Disease monitoring was performed just after R. necatrix inoculation. Sampling points for further analysis were stablished at T0 (previous to the preventive treatment), T1 (after preventive treatment), T2 (after R. necatrix inoculation), T3* (taken only during biocontrol in “assay 2”) and T4 (end of the experiment). (B) Aspect of the mesocosms experiment at –70, 30 and 110 days along the experiment.
Briefly, an experimental microplot platform that mimicked field conditions was designed and constructed for the plant assays at the IHSM-UMA-CSIC “La Mayora” (Algarrobo Costa, Spain, 36°45′37.74″ N – 4°02′26.28″ W). The greenhouse was built as an open structure with double roofing to allow air passage for improved ventilation, and the microplots (mesocosms of 35 liter plant pots) were planted in a white gravel bank to reduce oscillation of the soil temperature. Environmental conditions were monitored during each experiment by using a portable data logger, which recorded the air temperature and relative humidity.
In each independent assay, a total of 102 two-year-old commercial avocado seedling plants (cv. Topa-Topa) were independently transplanted to 35 liter pots filled with a blend (1:1) of solarized natural soil and peat and randomly placed into the experimental area. Each plant into its pot constitute an independent mesocosom. Seventeen independent avocado plants were used for each of the treatments assayed in these studies as listed in Table 2. For each independent treatment, eleven plants were inoculated with R. necatrix to study multitrophic interactions during biocontrol, and the remaining six non-inoculated plants were used as controls to study multitrophic interactions without the presence of the pathogen. Fungal inoculation was performed as previously described (Sztejnberg and Madar, 1980; Cazorla et al., 2006). Briefly, four holes per pot were made on the soil surface using a punch, and 16 g of wheat colonized with R. necatrix strain CH53 was distributed in the holes before filling them with the surrounding soil.
The first assay, “assay 1,” was designed to test the biocontrol of different treatments with formulated PcPCL1606 as a biologically active product against R. necatrix and to study the impact of the application of PcPCL1606 on natural microbial populations with R. necatrix inoculation. PcPCL1606 treatments were applied by irrigating a final cell concentration of 1.0 × 1010 cfu suspended in 200 ml of sterile water. Treatments were applied using watering to properly distribute the bacterial cells onto the whole pot surface. One of the bacterial treatments was a preventive application (PcPCL1606 preventive), consisting of a single application with a semi-commercial formulate of PcPCL1606 (PcPCL1606 preventive) performed 50 days before inoculation with R. necatrix. A second treatment was a curative application (PcPCL1606 curative), using the same semi-commercial formulate of PcPCL1606, which was added after symptoms appeared. The application was performed 70 days after inoculation with R. necatrix. A third treatment was included and consisted of a control treatment designed to compare the accuracy of the bacterial counts in different culture media. For this, the PcPCL1606-GFP derivative strain was used in the experiments, following the preventive protocol described above (Table 1 and Figure 1). A bacterial suspension of PcPCL1606-GFP (growing in liquid TPG medium for 24 h at 25°C and 180 rpm) reached a bacterial concentration of 1.4 × 109 cfu/ml. A total of 1010 cfu per plant was applied in this treatment (as described above) and allowed specific bacterial counts from soil and rhizosphere samples. In a second assay (“assay 2”), only the preventive semi-commercial formulated PcPCL1606 treatment was repeated to confirm its biocontrol efficacy on the pathogen in the previous assay and to study the impact of PcPCL1606 application on the microbial communities during biocontrol (Table 1 and Figure 1).
In both assay 1 and assay 2, two control treatments were included. First, a positive control of biocontrol consisted of developing soil-induced suppressiveness to R. necatrix (Vida et al., 2016). For this, 19 liters of composted almond shells (ASO treatment) was placed on the top layer of 16 liters of soil and peat. This positive control treatment was initiated 150 days before R. necatrix inoculation to allow the soil to induce suppressiveness (Figure 1 and Table 1; Vida et al., 2016). The negative control consisted of a group of 17 avocado plants without bacterial treatments.
To study the biological control response of the different treatments, plant disease was monitored weekly for the next 110 days after pathogen inoculation (day 0) in both experiments. Aerial symptoms of white root rot in symptomatic plants was measured using a symptom scale (Bonilla et al., 2015): 0, healthy plant; 1, plant with first symptoms of wilt; 2, overall wilted plant; 3, wilted plant with first symptoms of leaf desiccation; and 4, completely dried plant (dead plant) (see Supplementary Figure S1). The disease index (DI) was calculated for each treatment as previously described (Cazorla et al., 2006). The experiment was considered finished 110 days after inoculation. The area under the disease progress curve (AUDPC) was calculated for statistical comparison of the treatments (Campbell and Madden, 1990) and analized as González-Sánchez et al. (2013).
Along the experiment, soil, and rhizosphere samples were taken from each of the assayed treatments at the different sampling points to study the effect of the treatments with formulated PcPCL1606 on the microbial community (Figure 1). During assay 1, soil and rhizosphere samples were taken at different sampling times. Two sampling points were taken before inoculation with R. necatrix (considered day 0): one sample was collected before preventive bacterial treatment (T0, −70 days) and a second sample was collected after preventive treatment (T1, −20 days). Samples were collected at two more times after inoculation with R. necatrix: at 30 days after treatment (T2) and at the end of the experiment, 110 days after R. necatrix inoculation (T4). Following the indications reported in assay 1 and taking into account the biocontrol results from assay 1, in assay 2, soil and rhizosphere sampling was only conducted 80 days after the inoculation with R. necatrix (sampling point T3), when the biocontrol was effective after the preventive bacterial treatment with formulated PcPCL1606.
Sampling was performed as described below. Three plants per treatment were randomly selected. Fifteen-centimeter-deep soil core samples were obtained using a 4-cm-diameter core sampler, avoiding to collect close to the inoculation points. Three equidistant points around each plant were sampled and pooled to provide a single composite sample from each plant. Each composite soil and rhizosphere sample per plant was individually processed and analyzed. In the case of the soil and rhizosphere samples taken from three plants challenged with R. necatrix in assay 2 at T3, the 3 samples from the untreated control plants were taken from individual symptomatic plants displaying different disease indexes (with disease index 1, 2, or 3; Supplementary Figure S1).
The collected soil and rhizosphere samples were placed in cold storage and transported to the laboratory. From these samples, the roots of avocado, which had adjacent soil, were carefully separated and considered the rhizosphere samples. The rest of the soil was sieved through a 2 mm pore-size sieve. The bulk soil sample was the sieved soil carefully cleared from the roots was considered the bulk soil sample. Fresh soil and rhizosphere samples were used for culture-dependent and culture-independent approaches to perform microbial population analysis. DNA extraction from the soil and rhizosphere samples was also performed immediately after sample collection.
Culture-based microbial analysis of Pseudomonas present in the soil and rhizosphere samples was performed. For the soil sample analysis, subsamples of 5 g of the bulk soil were suspended in 40 ml of saline solution (0.85% NaCl) with 5 g of sterile gravel (2 to 4 mm in diameter) and mixed at 250 rpm for 30 min on an orbital shaker, which was followed by 20 min of decantation (Bonilla et al., 2015). For the rhizosphere sample analysis, one gram of the fine roots was homogenized for 2 min in a Stomacher bag with 4 ml of saline solution (Bonilla et al., 2015). The supernatants of both the soil and rhizosphere samples was serially diluted 10-fold; 100 μl of each dilution was plated on different selective media, and bacterial counts were recorded after 48 h at 25°C.
To obtain the bacterial counts of fast-growing heterotrophic bacteria, plates of LB medium amended with cycloheximide (100 mg/liter) were used to prevent fungal growth. To count the number of pseudomonads, the previously described Pseudomonas selective medium (PSM) was used. PSM is composed of King’s B (KB) agar amended with 75 mg of penicillin G, 45 mg of novobiocin, 50 mg of nitrofurantoin and 100 mg of cycloheximide per liter (Larkin and Honeycutt, 2006; Vida et al., 2017).
To study PcPCL1606 survival and to correlate these values with the bacterial count values of PcPCL1606 obtained in PSM with antibiotics, a preventive treatment with PcPCL1606-GFP (gentamicin-resistant strain; Table 1) following an identical protocol as described above was performed during assay 1. Soil and rhizosphere samples were taken along assay 1 to compare the bacterial counts in PSM with those in TPG medium amended with gentamycin (80 mg per liter; TPG-Gm). The same soil and rhizosphere samples were processed as described above and plated on PSM and TPG-Gm medium to compare the bacterial counts after 48 h at 25°C. Typical colony morphology of PcPCL1606-GFP and fluorescence validated the counts of Gm-resistant bacteria.
At each sampling point, DNA was extracted from soil and rhizosphere samples to specifically detect the presence of PcPCL1606 and R. necatrix. Additionally, to analyze the effect of PcPCL1606 application on microbial communities of soil and rhizosphere of avocado plants, samples were taken at T2 (80 days after inoculating the bacteria) in assay 1 from the control and preventive treatment where R. necatrix had not been applied and analyzed. To test the effect of the preventive PcPCL1606 application during biocontrol, samples from T3 of assay 2 (80 days after inoculating the fungal pathogen) in the control and preventive treatment where R. necatrix was inoculated were analyzed. DNA was extracted from each of the 3 independent composite samples where formulated PcPCL1606 was applied (PcPCL1606 preventive) and 3 composite samples where it was not applied (Control). Soil and rhizosphere DNA extractions were performed using 2.0 g of soil or rhizosphere sample and a PowerSoil® DNA Isolation Kit (Qiagen Iberia S.L., Madrid, Spain). The DNA extraction quantity and quality (A260/A230 > 1.8 and A260/A280 > 1.7) were evaluated using a NanoDrop ND-1000 spectrophotometer (NanoDrop Technologies Inc., Wilmington, DE, United States). Additionally, DNA quality was analyzed by agarose gel electrophoresis and RedSafeTM staining (Labotaq, Seville, Spain). DNA was stored at −20°C for further analyses.
Specific detection of PcPCL1606 in each soil and rhizosphere samples at different sampling times (T0, T1, T2, T3 and T4) was performed by PCR-amplification of a partial sequence inside the gene PCL1606_04860 (with certain homology to the sequence of a glutamine-fructose-6-phosphate aminotransferase from P. aeruginosa), which contains a specific 378 nt sequence in PcPCL1606. Specific primers 04860F and 04860R (Supplementary Table S1) were used, and the amplification product was revealed after electrophoresis. Specific detection of R. necatrix was performed following previously described procedures (Schena et al., 2002).
To analyze the effect of PcPCL1606 on the microbial communities on the uninoculated samples or during the biocontrol process, metagenomic approaches were followed. For metagenomics analysis, the DNA samples taken from each rhizosphere/soil type were sent to be sequenced by ChunLab (Seoul, South Korea) to obtain the microbial DNA sequences of the 16S rRNA gene and ITS hypervariable regions. For this, a partial16S fragment (428 bp in size) corresponding to the V3-V4 region, was amplified using the PCR primers 341F (CCTACGGGNGGCWGCAG) and 805R (GACTACHVGGGTATCTAATCC), resulting in a 428 bp amplicon (Herlemann et al., 2011). For the ITS amplification, PCR primers ITS1F (CTTGG TCATTTAGAGGAAGTAA; Gardes and Bruns, 1993) and ITS2 (GCTGCGTTCTTCATCGATGC; White et al., 1990) targeted the ITS1F-ITS2 region, resulting in a PCR product of 230 bp in size. PCR products were sequenced by using MiSeq technology (Illumina). Sequences were analyzed using EZbioCloud software (ChunLab)1 as follows. Processing raw reads started with quality check and filtering of low quality (<Q25) reads by Trimmomatic version 0.32 (Bolger et al., 2014). After QC pass, paired-end sequence data were merged together using fastq_mergepairs command of VSEARCH version 2.13.4 (Rognes et al., 2016) with default parameters. Primers were then trimmed with the alignment algorithm of Myers and Miller (1988) at a similarity cut off of 0.8. Non-specific amplicons that do not encode 16S rRNA were detected by nhmmer (Wheeler and Eddy, 2013) in HMMER software package version 3.2.1 with hmm profiles. Unique reads were extracted and redundant reads were clustered with the unique reads by derep_fulllength command of VSEARCH (Rognes et al., 2016). The EzBioCloud 16S and ITS rRNA database (Yoon et al., 2017) was used for taxonomic assignment using usearch_global command of VSEARCH (Rognes et al., 2016) followed by more precise pairwise alignment (Myers and Miller, 1988). Chimeric reads were filtered on reads with <97% similarity by reference based chimeric detection using UCHIME algorithm (Edgar et al., 2011) and the non-chimeric 16S and ITS rRNA database from EzBioCloud. After chimeric filtering, reads that are not identified to the species level (with <97% similarity) in the EzBioCloud database were compiled and cluster_fast command (Rognes et al., 2016) was used to perform de novo clustering to generate additional OTUs. Finally, OTUs with single reads (singletons) are omitted from further analysis. Finally, the relative abundance of each treatment of eukaryotes/prokaryotes at different taxonomic levels was calculated as the average from three independent samples and was used to perform the comparative distribution analysis.
The secondary analysis, which includes alpha-diversity calculation and biomarker discovery, was conducted by in-house programs of Chunlab, Inc (Seoul, South Korea). All analytics mentioned above were performed in EzBioCloud 16S-ITS based MTP (Microbiome Taxonomic Profile), which is a ChunLab’s bioinformatics cloud platform. The Chao1 index (Chao, 1987) and the Shannon index (Magurran, 2013) were performed as previously described. Rarefaction curves were also applied as previously described (Heck et al., 1975). Beta-diversity was calculated from the relative abundance data (at genus level) from all the samples. The Bray-Curtis index was used to calculate samples similarities. For all analyses, the Fitopac 2.1 software (Shepherd, 2010) was used.
To test R. necatrix inhibition by different treated soils, soil suppressiveness assays were performed using a diffusion chamber experiment (Bonilla et al., 2015; Vida et al., 2016). A fungal disk of R. necatrix (0.6-cm in diameter) grown on potato dextrose agar (PDA) was placed on a disk of water-agar medium (1% agar; 5 cm in diameter) and transferred to a nitrocellulose filter (pore size 0.45 μm). These systems were placed on soil samples with different treatments taken from assay 2 at sampling point T3. The diffusion chamber was incubated for 5 days at 25°C. The total area of R. necatrix growth was measured using Quantity One 1-D analysis software (Bio-Rad Laboratories, Inc., Madrid, Spain) for each soil. Nine replicate chambers per soil type were analyzed. Composted almond shell (ASO)-amended soil was used as suppressiveness positive control. Unamended and untreated controls (Control) and soil where formulated PcPCL1606 had been applied (PcPCL1606 preventive) were assayed.
To verify the role of PcPCL1606 in the suppressiveness of soil samples under the preventive treatment of PcPCL1606, we prepared heat-treated PcPCL1606 preventive soil (HT). Briefly, heat-treated soil consisted of heating the soil in 2 autoclave steps as previously described (Vida et al., 2016). To analyze the restoration of suppressiveness, complemented soil was constructed; HT soil was inoculated with 102 cfu/g soil of PcPCL1606 from a bacterial culture growing overnight (HT + PcPCL1606).
Due to the important role of the antifungal compound HPR in the biocontrol and antagonism of PcPCL1606 (Calderón et al., 2013), supplementation of the HT soil with the ΔdarB mutant (HT + ΔdarB) was also performed. ΔdarB is a mutant deficient in HPR production (not antagonistic and no biocontrol strain) and was used to verify the implication of this compound in suppressiveness (Table 1).
Data distributions were tested using one-way analysis of variance (ANOVA) followed by Fisher’s least significant different test with Bonferroni’s correction (P = 0.05). All data analyses were performed using IBM SPSS statistics 25 software (SPSS, Inc., Chicago, IL, United States). Based on the standard error, the 95% confidence interval for each response variable was obtained, and the significant differences between the soils were estimated.
In mesocosm studies designed to unravel the biocontrol effect of PcPCL1606 application, the first aerial symptoms of white root rot appeared approximately 30 to 40 days after inoculation with R. necatrix in both independent microplot assays. The disease index evolution with time is detailed in Figure 2 for each treatment. From the assayed treatments in both assays, the first to show aerial symptoms of white root rot was the unamended control treatment (Control), which reach a disease index above 80%, with many of the inoculated plants already dead at 110 days after R. necatrix inoculation.
Figure 2. Effect of formulated PcPCL1606 application on avocado white root rot in mesocosm assays. Aerial symptoms of white root rot of the avocado plants under different treatments were calculated, and the time course of the disease index represented in (A) assays 1 and assay 2. Area under the disease progress curve (AUDPC) calculated at the end of the (B) assay 1 and assay 2. Control, no organic amendment and no bacteria; PcPCL1606 preventive, preventive treatment with formulated PcPCL1606; ASO, composted almond shell; PcPCL1606 curative, curative treatment with formulated PcPCL1606; PcPCL1606-GFP, preventive treatment with the derivative PcPCL1606-GFP. Data were analyzed for significance after arcsine square root transformation with analysis of variance, followed by Fisher’s least significant difference test (P = 0.05). Values of bars with different letter indications denote a statistically significant difference.
During assay 1, the positive control of suppressiveness (ASO treatment) showed a delay in symptom appearance but not in symptom reduction. However, during assay 2, ASO treatment induced a delay in appearance and a decrease in white root rot symptoms (Figure 2). In those experiments, the most evident biocontrol effect was produced by the formulated PcPCL1606 treatments. Preventive and curative PcPCL1606 treatments reduced symptom development and reached disease index values of 65 and 70%, respectively (Figure 2). The treatment with PcPCL1606-GFP showed a delay in symptom development but at the end of the experiment reached a disease index level similar to the untreated control, similar to the results of ASO treatment. The temperature and relative humidity data recorded for each experiment showed some differences among seasons, which usually occurs under real field conditions (Supplementary Figure S2). It is important to remark that during assay 1, several days showed unusually low temperatures approximately 60 days after R. necatrix inoculation (March–April 2018), coincident with an increase in disease index in some treatments in assay 1. However, a statistical comparison of AUDPC data revealed that all of the assayed PcPCL1606 treatments resulted in a significant reduction (ANOVA, P < 0.05) in the disease index when compared to the untreated control plants.
To count the bacterial levels of PcPCL1606 isolated from soil and rhizosphere samples, the PcPCL1606-GFP strain (Table 1) was used for comparison studies. Bacterial counts from samples under preventive treatment with PcPCL1606-GFP were calculated on TPG-Gm medium to specifically select this bacterial strain and PSM. Plate counts revealed the presence of this strain during the experiment, with levels ranging from 103 to 105 cfu/g and with almost no differences at different sampling times in the rhizospheric and soil samples. A high correlation among the bacterial counts of the two media was obtained, with a regression equation of y = 0.106 + 1.097x (R = 0.972) (Supplementary Figure S3).
Bacterial counts in the bulk soil and rhizosphere samples during assay 1 were performed to analyze the effect of PcPCL1606 treatment on the culturable bacterial populations, especially the group pseudomonas. At T0 (50 days after the initiation of the experiments), bacterial counts were very similar among the samples from the soil and rhizosphere, independent of whether they were taken from the untreated control or the ASO treatments. Bacterial counts of total heterotrophic bacteria were approximately 106 cfu/g of soil or rhizosphere. Pseudomonas-like counts were also approximately 106 cfu/g, with a decrease in count value in rhizosphere samples compared with untreated control plants, with 105 cfu/g of rhizosphere (T0, Figure 3).
Figure 3. Culturable bacterial populations (total heterotrophic bacteria and pseudomonas-like) during the “assay 1” microcosms experiments. The population densities of fast-growing heterotrophic bacteria and pseudomonas-like were assessed by plate counts at different times (T0, T1, T2, and T4). Bacterial counts obtained from samples taken from inculated plants with R. necatrix are noted as +Rn.
Thirty days after PcPCL1606 preventive inoculations (T1, 20 days before inoculation with R. necatrix), bacterial counts maintained similar values among soil and rhizosphere samples from the different treatments, with values of total heterotrophic bacteria of approximately 106 cfu/g and slightly lower values for Pseudomonas-like counts, mainly below 105 cfu/g. The samples from untreated control plants had higher total heterotrophic bacterial counts (5 × 106 cfu/g rhizosphere) and, in contrast, showed lower values for Pseudomonas-like counts of approximately 4 × 104 cfu/g of rhizosphere (T1, Figure 3).
In T2 (30 days after inoculation with R. necatrix; 80 days after the preventive treatment with formulated PcPCL1606), comparisons among the mesocosm experiments with or without the fungal pathogen were also performed. In general, samples from plants not inoculated with R. necatrix showed a slight reduction in total heterotrophic bacterial counts, with higher values of approximately from 7 × 105 cfu/g to 9 × 105 cfu/g in soil and rhizosphere samples from PcPCL1606- and ASO-treated plants (with similar values to those obtained in T1). However, a strong reduction in Pseudomonas-like counts (almost two orders of magnitude less when compared with total heterotrophic bacteria) was also observed, with values ranging from 103 to 104 cfu/g of sample, with lower values detected in samples from the untreated control plants and higher values in the soil and rhizosphere samples from ASO-treated plants (T2, Figure 3). In the plants challenged with R. necatrix, lower counts of total heterotrophic bacteria were obtained in general when compared with the unchallenged plants at this same sampling time. Higher values (1–8 × 105 cfu/g) were also displayed by samples from plants under ASO and PcPCL1606-GFP treatments, and lower values were observed with the untreated control plants, with values of approximately from 1 × 104 cfu/g to 3 × 104 cfu/g. On the other hand, in the samples from soil inoculated with R. necatrix, the Pseudomonas-like counts were clearly higher when compared with the samples not inoculated with R. necatrix. Values ranged from 104 to 105 cfu/g, reaching almost the same value as total heterotrophic bacteria (samples from untreated control plants). In some cases, the Pseudomonas-like levels were not affected by the presence of R. necatrix, as shown by the samples taken from the ASO treatment (T2, Figure 3).
Finally, the counts of total heterotrophic bacteria at the end of the experiment (T4; 110 days after R. necatrix inoculation; 160 days after preventive treatment with PcPCL1606; 30 days after curative treatment with PcPCL1606), when no R. necatrix inoculation was performed, were very similar to those reported in T2, but with a slight reduction in almost all the samples. In these samples, the Pseudomonas-like counts were approximately two orders of magnitude lower than the total heterotrophic bacteria counts. However, the total heterotrophic bacteria counts were higher in some treatments, such as the curative applications of PcPCL1606 (applied 30 days before this sampling point), as well as in the treatment with composted almond shells (ASO) and in the rhizosphere of the preventive treatment with PcPCL1606 (applied 160 before this sampling point) (T4, Figure 3). When the plants were inoculated with R. necatrix, bacterial counts from T4 soil and rhizosphere samples showed a similar behavior to T2, with lower levels of total heterotrophic bacterial counts but higher Pseudomonas-like counts. Additionally, in most of the samples, the Pseudomonas-like counts reached similar values as the total heterotrophic bacterial counts. Higher total heterotrophic bacterial counts were detected in ASO samples and in rhizosphere samples preventively treated with PcPCL1606. Interestingly, the Pseudomonas-like counts were also higher in rhizosphere samples preventively treated with PcPCL1606. Remarkably, typical colonies of PcPCL1606-GFP from soil and rhizosphere samples taken from plants that survived after 160 days after inoculation were recovered on TPG-Gm plates with counts above 103 cfu/g.
Because in assay 2, we focused on the confirmation of the biocontrol results previously observed with the PcPCL1606 preventive treatment in assay 1, only one sampling point was taken at T3, when biocontrol was observed. Total heterotrophic bacteria and Pseudomonas-like counts (Supplementary Figure S4) showed values intermediate to those between T2 and T4 in assay 1, ranging from 105 to 106 cfu of total heterotrophic bacteria/g and 103 to 104 cfu of Pseudomonas-like/g.
Sequencing of 16S rDNA and the ITS variable regions elucidated the relative abundances of microbial clades at different taxonomic levels. Comparative distribution analysis were performed only with the most abundant OTUs (≥1% of relative abundance), quantified with a sufficient level of precision due to the high level of OTU richness. In all samples, after sequencing of 16S rDNA, a very low relative abundance of Archaea was found (<0.1%).
In assay 1 (at sampling time T2, data available at https://doi.org/10.6084/m9.figshare.12310253.v1), soil and rhizosphere samples from untreated control plants and from plants under preventive treatment with PcPCL1606 and not challenged with R. necatrix were analyzed to study the impact of PcPCL1606 treatments on microbial communities. OTUs with a relative abundance above 1% comprised approximately 65% of the relative abundance of prokaryotic microorganisms in soil and rhizosphere samples from plants under the two different treatments (Figure 4). No relevant changes were observed in the relative abundance of prokaryotic families in any of the analyzed samples, with the seven more abundant OTUs (Rhodospirillaceae, Cytophagaceae, Acidobacteriaceae, Micropepsaceae, Pedosphera_f, Opitutaceae and Steroidobacter_f) covering approximately 30% of the relative abundance in all analyzed samples (Figure 4A). The 5 most abundant phyla (above 79% of relative abundance) were Proteobacteria (42.89%), Acidobacteria (10.86%), Bacteroidetes (10.42%), Verrucomicrobia (8.95%) and Actinobacteria (6.04%). At the class level, Alpha- and Gammaproteobacteria comprised approximately 30% of the relative abundance in the four analyzed samples. Focusing on the relative abundance of members of the Pseudomonas genus, the relative abundance was approximately 0.1% in plants under PcPCL1606 treatment and in soil samples of untreated control plants but was 0.3% in rhizosphere samples from untreated plants. The specific relative abundance of P. chlororaphis showed levels below 0.01% of the total relative abundance, except in the rhizosphere of PcPCL1606-treated plants, with 3 times more relative abundance (approximately 0.03%; Figure 4B).
Figure 4. Analysis of prokaryotic communities present in samples of soil and rhizosphere taken from avocado plants non-inoculated with R. necatrix during “assay 1” at T2. Negative control of soil (Control soil) and rhizosphere (Control rhizosphere) and samples from formulated PcPCL1606 preventive treatment of soil (PcPCL1606 preventive soil) and rhizosphere (PcPCL1606 preventive rhizosphere) were showed. (A) Relative abundance (percentage) of different prokaryotic groups detected by 16S rDNA gene sequencing analysis of soil and rhizosphere DNA at family level. The group with < 1% relative abundance was represented in gray. (B) Relative abundance of Pseudomonas genus and Pseudomonas chlororaphis specie at different treatments.
In the same samples, the relative abundance of eukaryotes revealed a similar distribution in the rhizosphere and soil samples treated with PcPCL1606 (Figure 5A). A higher abundance for a family representative of the kingdom of Chromista can be observed in samples taken from the untreated control plants. Additionally, in the rhizosphere samples from untreated control plants, a higher relative abundance was observed for an unclassified group and for the class Sordariomycetes. Similar results could also be observed at the class level, where the samples from the PcPCL1606 treatment showed a high relative abundance of Agaricomycetes, Sordariomycetes, Aphelidiomycetes and unclassified fungi; however, in the untreated control samples, the relative abundance of Agaricomycetes was lower, and the relative abundance of Chromista (Chromista_g_uc and Chromista_c) was higher. In these samples, the relative abundance of Xylariaceae was very low (Figure 5B), ranging from 0.02 to 0.04%.
Figure 5. Analysis of eukaryotic communities present in samples of soil and rhizosphere taken from avocado plants non-inoculated with R. necatrix during “assay 1” at T2. Negative control of soil (Control soil) and rhizosphere (Control rhizosphere) and samples from formulated PcPCL1606 preventive treatment of soil (PcPCL1606 preventive soil) and rhizosphere (PcPCL1606 preventive rhizosphere) were showed. (A) Relative abundance (percentage) of different eukaryotic groups detected by internal transcribed sequences (ITS) sequencing analysis of soil and rhizosphere DNA at family level. The group with < 1% relative abundance was represented in gray. (B) Relative abundance of the family Xylariaceae at different treatments.
In soil and rhizosphere samples from microplots artificially inoculated with R. necatrix, the analysis of the 16S rRNA sequences (data available at https://doi.org/10.6084/m9.figshare.12309788.v1), revealed a decrease in the relative abundance of the family Acidobacteriaceae, but similar groups of microorganisms were found at the family level (Figure 6A) among the different independent experiments (assay 1 and assay 2). Prokaryotic communities from soil and rhizosphere samples treated with PcPCL1606 were almost identical among each treatment and differed from the communities detected in the control samples, which were also more similar among each treatment (Figure 6A). The phylum Proteobacteria comprised approximately 50% of the relative abundance, where the class Alphaproteobacteria was the most abundant (approximately 30%), followed by the class Gammaproteobacteria (ranging from 6.8 to 9.3%). The relative abundance of the genus Pseudomonas ranged from 0.2 to 0.3%, with higher values in the soil from samples treated with PcPCL1606 (Figure 6B). Interestingly, the relative abundance of the species P. chlororaphis was detectable in the samples from the rhizosphere and soil treated with PcPCL1606 (Figure 6B), but with low values of relative abundance (0.01–0.03%, respectively).
Figure 6. Analysis of prokaryotic communities present in samples of soil and rhizosphere taken from avocado plants inoculated with R. necatrix during “assay 2” at T3. Negative control of soil (Control soil) and rhizosphere (Control rhizosphere) and samples from formulated PcPCL1606 preventive treatment of soil (PcPCL1606 preventive soil) and rhizosphere (PcPCL1606 preventive rhizosphere) were showed. (A) Relative abundance (percentage) of different prokaryotic groups detected by 16S rDNA gene sequencing analysis of soil and rhizosphere DNA at family level. The group with <1% relative abundance was represented in gray. (B) Relative abundance of Pseudomonas genus and Pseudomonas chlororaphis specie at different treatments.
The ITS sequences were analyzed to reveal the abundance of eukaryotic microbes on the microplots inoculated with the soilborne phytopathogen R. necatrix; this allowed us to identify differences in the composition and relative abundance of fungal microbes. A higher number of eukaryotic families with relative abundances equal to or greater than 1% was found in the rhizosphere samples taken from plants under treatments with PcPCL1606, and a lower number was detected in rhizosphere samples from untreated control plants (Figures 7A,B). Eukaryotic communities from samples of rhizosphere treated with PcPCL1606 were very similar among samples, independent of whether they were inoculated or not with R. necatrix (Figures 5A, 7A). In general, the introduction of the fungal pathogen R. necatrix disturbed the eukaryotic community, leading to different patterns in the different samples and showing a high relative abundance of Xylariaceae and R. necatrix (Figures 7A,B). To highlight the differences generated by the pathogen, plants displaying different disease indexes (from 1 to 3) were taken, and the soil and rhizosphere of control plants not treated with PcPCL1606 were sampled. The individual analysis of these soil and rhizosphere samples coming from symptomatic plants in the untreated control plants revealed the profound effect of the pathogen, with an increase in the relative abundance of Xylariaceae (the family where R. necatrix belongs) with an increase in the disease index (Figures 7B,C). However, almost no detection of Xylariales or the species R. necatrix was observed from the samples treated with PcPCL1606 (Figure 7C). Interestingly, after treatment with PcPCL1606, soil samples showed a high increase in the family Entolomataceae (Figure 7A).
Figure 7. Analysis of eukaryotic communities present in samples of soil and rhizosphere taken from avocado plants inoculated with R. necatrix during “assay 2” at T3. Negative control of soil (Control soil) and rhizosphere (Control rhizosphere) and samples from formulated PcPCL1606 preventive treatment of soil (PcPCL1606 preventive soil) and rhizosphere (PcPCL1606 preventive rhizosphere) were showed. (A) Relative abundance (percentage) of different eukaryotic groups detected by internal transcribed sequences (ITS) sequencing analysis of soil and rhizosphere DNA at family level. The group with <1% relative abundance was represented in gray. (B) Relative abundance of eukaryotic communities on individual samples of soil and rhizosphere from control plants displaying different disease index of white root rot. (C) Relative abundance of the family Xylariaceae and the specie R. necatrix at different treatments.
The Shannon diversity index and Chao richness index for prokaryotes and eukaryotes in bulk and rhizosphere soils are shown for assay 1 and assay 2, and overall, no differences in microbial diversities between bulk and rhizosphere soil was observed (Figure 8). The Shannon diversity index for prokaryotes was not influenced by the treatment with PcPCL1606, which was independent of whether R. necatrix was present (Figure 8). PcPCL1606 preventive application did not significantly influence prokaryotic diversity (Figure 8A). For eukaryotes, no significant differences were observed in non-artificially infected samples; however, a significant difference was found in the Shannon diversity index among rhizosphere samples treated or untreated with PcPCL1606 (Figure 8A). Prokaryotic and eukaryotic richness using the Chao index showed no significant differences among the different samples and treatments (Figure 8B).
Figure 8. Analysis of diversity with Shannon (A) and CHAO (B) index of 16S rRNA and ITS sequences from samples of soil/rhizosphere the avocado plant during bicontrol against R. necatrix. Samples analyzed were obtained from the negative control of soil (Control soil) and rhizosphere (Control rhizosphere), and samples from formulated PcPCL1606 preventive treatment of soil (PcPCL1606 preventive soil) and rhizosphere (PcPCL1606 preventive rhizosphere). –Rn, uninoculated plants; +Rn, plants inoculated with R. necatrix. Values of bars with same letter indications denote a non-statistically significant difference.
Additionally, the Beta-diversity analysis using Bray-Curtis dissimilarities, showed that a preventive application of PcPCL1606 had no influence on the prokaryotic community structure, clustering together with the non-treated samples (Supplementary Figure S5A); however, the presence of R. necatrix resulted in a separate clustering of the samples treated or non-treated with PcPCL1606. For the eukaryotic community (Supplementary Figure S5B), the results are very similar, separating the samples with the preventive treatment of PcPCL106 to the samples without bacterial treatment. It is remarkable that eukaryotic communities taken from diseased plants with the lower disease index (samples P1), were allocated in between these two main groups (Supplementary Figure S5B).
The ability of the different soil samples to inhibit R. necatrix was tested using the diffusion chamber assay. The suppressively induced soil after ASO application, as well as combinations with soil treated and untreated with PcPCL1606, were tested. The highest fungal growth inhibition was displayed by the fresh soil amended with composted almond shell and the combinations including a preventive treatment with PcPCL1606, which had a significantly lower area (ANOVA, P < 0.05) than the untreated control soil and the rest of the soil combinations (Figure 9). To reveal the microbial nature from suppressiveness, suppressive soil after PcPCL1606 preventive treatment was heat-treated, which abolished its protective phenotype. To assign the protective effect to PcPCL1606, the heat-treated soil was complemented with PcPCL1606, which significantly recovered the suppressiveness. On the other hand, heat-treated soil complemented with the non-antagonistic HPR-defective strain ΔdarB (Table 1) displayed a clear failure of soil suppressiveness, showing higher fungal colony area of growth (ANOVA, P < 0.05) very similar to the growth in heat-treated soil and control soil without any treatment.
Figure 9. Soil suppressivity assay against R. necatrix in diffusion chambers. R. necatrix growth area of different soil samples from “assay 2” at T3, used in mesocosm assays. Treatment assayed were Unamended/negative control (Control), composted almond shell (ASO) amended soil was used as positive control of suppressiveness, soil where PcPCL1606 formulated had been applied (PcPCL1606 precentive), heat-treated soil from PcPCL1606 preventive (HT), HT suplemented with PcPCL1606 (HT + PcPCL1606) and HT suplemented with ΔdarB mutant, non-HPR-producing (HT + ΔdarB). Values of bars with different letter indications denote a statistically significant difference.
Pseudomonas chlororaphis PCL1606 (PcPCL1606) has emerged as a potential biocontrol agent in previous works (Pliego et al., 2011). PcPCL1606 has a strong antagonistic and biocontrol activity against several phytopathogenic fungi, among them R. necatrix (Cazorla et al., 2006), mainly due to the production of the antifungal compound HPR (Calderón et al., 2013). Previous studies have shown the biocontrol efficacy of PcPCL1606 at different levels (Cazorla et al., 2006; González-Sánchez et al., 2013), and recently, the potential of this strain as a biocontrol agent was also demonstrated in the integrated control against R. necatrix in avocado plants; the strain was combined with low concentrations of fungicide, which had a higher plant protection, leading to a reduction in chemical residues and appearance of fungal resistance (Arjona-López et al., 2019). However, one of the final steps to propose PcPCL1606 as a useful and safe biocontrol agent against soilborne fungal pathogens includes the assessment of the presence and abundance of the applied biocontrol agent in soil, testing the efficacy and possible impact on autochthonous soil microbial communities. It is worth noting that this analysis is required previouslu to the registration in Europe of any plant protection products (Commission Regulation No. 544/2011, L155/66).
Survival of inoculated Pseudomonas sp. in the soil and plant rhizosphere would be dependent on many factors, such as inoculate formulation, soil conditions, and physiological status of the plant (Wessendorf and Lingens, 1989; van Elsas et al., 1992). In our study, PcPCL1606 was experimentally formulated by Koppert B.V. (Netherlands) and applied by watering the soil around R. necatrix-infested plants to mimic the commercial conditions for this treatment. Formulated PcPCL1606 was previously tested in the laboratory and displayed equal antagonism, HPR production, biocontrol and specific amplification by PCR compared with the wild-type strain (data not shown).
It is worth mentioning that PcPCL1606 was previously isolated from avocado roots (Cazorla et al., 2006) in this same area, so it was expected that this bacterium would be very well adapted to this environment and could easily establish its interaction with natural avocado soils and avocado rhizosphere. The PcPCL1606 strain was found in both soil and rhizosphere samples, indicating that this strain can actively move and colonize avocado roots. Survival of PcPCL1606 was confirmed in the soil and rhizosphere at least 160 days after a single inoculation and under environmental conditions. This survival feature has also been shown by other previously reported Pseudomonas sp. (e.g., Gao et al., 2012), suggesting that the PcPCL1606 population stabilizes quickly after inoculating into soils and can persist for several months. At the end of the experiment, the survival of PcPCL1606 ranged from 103 to 104 cfu/g, correlating the counts obtained in both media used, TPG-Gm and PSM, indicating that the bacterial counts of PcPCL1606-treated samples in PSM could correspond mainly to the originally formulated PcPCL1606 strain.
It was observed that with only one preventive application (50 days prior to R. necatrix inoculation), PcPCL1606 was able to significantly reduce the disease index (25–30%) compared with the untreated plants at the end of two independent experiments, confirming the previously observed biocontrol activity for this strain (Cazorla et al., 2006; González-Sánchez et al., 2013). The results of bacterial counts on the soil and rhizosphere of mesoscosms under different treatments provided a first indication that the presence of R. necatrix has an impact on total heterotrophic bacteria and Pseudomonas-like bacterial counts. It was observed that the presence of the fungus stimulated the Pseudomonas-like counts but reduced the total heterotrophic bacteria counts. Similar results have been previously reported for culturable bacterial populations when interacting with other soil fungi. Some bacteria could use fungal-derived substrates and establish different bacteria-fungi relations ranging from mutualistic exudate-consuming to mycophagous interactions (de Boer et al., 2005). This could help to explain the higher survival of PcPCL1606 in the rhizosphere and soil when R. necatrix was introduced. Thus, the survival of PcPCL1606 over time would be due to the increase in the available fungal metabolites in the nearby surroundings, which could be easily used by the bacterium. It has been reported that PcPCL1606 is strongly chemotactically attracted by avocado root exudates (Polonio et al., 2017). Once on the root surface, PcPCL1606 can establish microcolonies along the avocado root with the help of exudate compounds in the rhizosphere (Lugtenberg et al., 2001; Calderón et al., 2014). Since PcPCL1606 occupies the same root niches where R. necatrix initiates plant infection, the probability that both microorganisms will meet on the avocado root surface and compete for available nutrients is high (Lugtenberg et al., 2001). However, R. necatrix also produces exudates that strongly attract PCL1606 (Polonio et al., 2017), finally leading PcPCL1606 to contact the R. necatrix hyphae. As a result of such interactions, the bacterial production of antifungal compounds and enzymes would result in a deleterious effect on the fungus, favoring the increase in the Pseudomonas-like counts in the soil and rhizosphere.
The effect of PcPCL1606 applications on the microbial communities when no R. necatrix was present was elucidated from natural soil and rhizosphere samples (results from assay 1). In those analyses, no relevant differences in the prokaryotic community structure and composition were observed, independent of whether the samples were taken from rhizosphere or bulk soil or from samples with or without the preventive treatment with PcPCL1606. In these samples, soil and rhizosphere communities were predominated by the phyla Acidobacteria, Actinobacteria, Proteobacteria, Verrucomicrobia and Bacteroidetes, which are the major phyla observed in soils with moderate inputs of organic matter (Lladó et al., 2017). The classes Alpha-, Beta- and Gammaproteobacteria, and Acidobacteria were, in proportion, the dominant bacterial taxa in the avocado soil and rhizosphere samples. These bacterial classes are the most frequently found in high C:N soil (Hermans et al., 2017) and are easily found in soil with the presence of organic matter in decomposition (Johnston et al., 2019). This is the case of the avocado soils of southern Spain, where attempts have been made to increase the low levels of organic matter by leaving leaf litter and chopped pruning waste on the top layer of soil every year (Bonilla et al., 2012) or where organic matter are currently used as amendments (Vida et al., 2016). The effect of PcPCL1606 on eukaryotic communities also revealed minor changes when formulated PcPCL1606 was applied to samples without R. necatrix. The clear majority of the eukaryotic communities were composed of fungi, with less than 10% of the eukaryotic ITS sequences belonging to organisms different than fungi (Cercozoa_f, Chlororphyta_f, Ciliophora_f, Plantae_f, etc.). It is worthy to note the presence of members from the family Chromista_f in all analyzed natural samples. This taxonomic group is widespread (Messenger et al., 2000) but is mainly associated with soils poorly drained, particularly clay soils, were the fundamental factor for the dissemination of spores is water, as observed in the experimental area of this work (Pérez-Jiménez, 2008). Regarding the typical composition of the fungal communities of these samples, members belonging to the saprophytic families commonly associated with organic matter decomposition, such as Agaricaceae, Chytridiomycetes_f and Sordariomycetes_f, were also reported; these families are typically found in environments where leaf litter is decomposed (Kerekes et al., 2013), which occurs with avocado crops. No relevant changes in fungal communities could be observed among the analyzed samples, and the presence of Xylariaceae (where the pathogenic fungi R. necatrix belongs) was almost not detected, with very low relative abundance. These results were also supported by the absence of significant differences among the diversity and richness indexes. Other studies have reported similar results indicating no relevant changes in natural microbial populations after the application of a biocontrol microorganism. For example, repetitive applications of a soil P. putida strain within a citrus orchard showed no effect on the resident microbial community (Steddom et al., 2002) or the treatment with P. fluorescens 2P24 and CPF10; after 8 weeks of application in cucumber, the differences in bacterial population structure compared with the control disappeared (Yin et al., 2013). The same have also been observed for Gram-positive biocontrol agents, such as B. subtilis B579, which was applied in cucumber plants, with a minimal and transient effect on the rhizosphere bacterial population 4 and 9 weeks after treatment (Chen et al., 2015), and Bacillus amyloliquefaciens FZB42, where no taxonomic differences were observed in the rhizosphere microbiota of lettuce 2 and 5 weeks after treatment (Kröber et al., 2014).
When the fungal soilborne pathogen R. necatrix was introduced in the mesocosm experiments, the microbial communities of soil and rhizosphere were strongly impacted in different ways. This fungus can attack the plant roots, multiply and expand its hyphae inside the roots, necrotizing the plant living tissues, and finally survive in the decomposing organic matter overwinter (Pliego et al., 2009, 2012). It has been previously described that the presence of a dominant soil fungus can influence the soil and rhizosphere microbial communities (de Boer et al., 2015; Johnston et al., 2019), and in our study, the presence of R. necatrix impacted the microbial communities, mainly because they are dependent on the ecological strategy of the dominant fungus (Johnston et al., 2019). The prokaryotic populations of soil and rhizosphere samples showed a slight response to this new biological factor; mainly, the relative abundance of families containing chitinolytic bacteria increased (Chitinophagaceae and Cytophagaceae). The relative abundance of these groups of chitinolytic bacteria increased in response to the presence of this new and available substrate, resulted by the presence of a soilborne fungal infection (Carrión et al., 2019). The relative abundance of the genus Pseudomonas was still low, and under this condition, the species P. chlororaphis was only detected in samples taken from plants treated with PcPCL1606, which indicates the strong adaptation of the bacterial biocontrol agent to this specific environment, as previously mentioned.
On the other hand, the eukaryotic community was more impacted by the presence of R. necatrix, especially in the taxonomic groups, with relative abundances of approximately 1–2%, which were completely different from samples collected from plants not inoculated. The rhizosphere and soil samples from untreated control plants showed the most evident impact, with 4 taxonomic groups (Nectriaceae, Chromista-f, Xylariaceae and Trichocomaceae) responsible for more than 50% of the relative abundance of eukaryotic communities. These observations agree with the obtained results for Beta-diversity, where the main impact onbserved in the analyzed samples was observed in those infested with R. necatrix. These impacts on eukaryotic organisms also resulted in a significant difference in the Shannon diversity index for the eukaryotic community, but no differences were observed when analyzing the Chao richness index. The dominant presence of R. necatrix in these soils resulted in white root rot disease, which can be considered conducive for this fungus because it shaped the surrounding environment, promoting the appearance of different fungi, mainly saprobes with aggressive colonization strategies and related to the degradation of wood and organic matter (Lasota et al., 2019). In those samples, the relative abundance of Xylariaceae and especially the species R. necatrix was almost the same in both control soil and rhizosphere samples, with average values above 12%. Since the avocado infection by R. necatrix is very aggressive (Pliego et al., 2012), samples to be analyzed were taken from 3 plants showing different disease indexes at the moment of the sampling time. Individual analysis revealed the increase and predominance of R. necatrix according to aerial symptoms and the important differences among the relative abundance of fungal families. Although these results were not conclusive, it seems that a succession of different fungi that change in relative abundance takes place during the infection process, with a progressive increase in R. necatrix.
However, in these same samples but treated with PcPCL1606, almost no Xylariaceae and/or R. necatrix were detected (<1%). This preventive PcPCL1606 treatment keeps the microbial communities of the rhizosphere more stabilized resulting in less changes compared with the fungal population from non-inoculated samples treated with PcPCL1606. Notably, the family Nectriaceae also increased with the treatment with R. necatrix, and several of the species contained in this family are saprobes or weak to virulent, facultative or obligate plant pathogens (Lombard et al., 2015). It is interesting to highlight the increase in relative abundance of the family Sebacinales in the rhizospheric and soil samples where formulated PcPCL1606 was applied. Sebacinales are highly diverse root symbionts that form various mycorrhizae and endophytic interactions and promote beneficial effects on host plants at diverse levels (Nautiyal et al., 2010; Franken, 2012; Kumar et al., 2012), enhancing abiotic stress resistance (Waller et al., 2005; Baltruschat et al., 2008; Ghimire and Craven, 2011) and resistance to pathogens (Waller et al., 2005; Serfling et al., 2007; Fakhro et al., 2010; Harrach et al., 2013). Interestingly, in soil samples treated with PcPCL1606, a clear impact was observed in fungal communities because the family Entolomataceae became predominant (>20% relative abundance). This fungal family is very species-rich, with most of them saprophytic on soil, wood or moss, but some members could be parasitic on plants or even ectomycorrhizal (Co-David et al., 2009). Since no symptoms were observed, these families are more likely to be related to organic matter decomposition or could be in a non-active form.
The suppressiveness displayed by the samples treated with PcPCL1606 can be inferred by the biocontrol experiments, but a more direct analysis showed that the application of PcPCL1606 can confer suppressiveness to the soil 130 days (T3) after the single treatment, similar to the positive control of suppressiveness-induced soil amended with composted almond shells (Vida et al., 2016). This suppressiveness is microbial-based since it completely disappeared after the soil samples were heat treated and can be recovered with a low-dose inoculation of PcPCL1606. Additionally, since HPR was described as the main factor involved in antagonism and biocontrol, soil complementation with the derivative mutant ΔdarB confirmed the direct involvement of HPR in soil suppressiveness by PcPCL1606.
A conclusion of this work is represented in Figure 10. The application of a formulated PcPCL1606 treatment to the commercial soil of avocado plants did not impact the soil and rhizosphere natural microbial populations (prokaryotic or eukaryotic). However, in a situation under R. necatrix infection, the application of PcPCL1606 reduced the symptom development of white root rot disease. Interestingly, the single preventive application allowed us to determine the survival of PcPCL1606 in the soil and avocado rhizosphere for at least 160 days, also conferring biocontrol to the avocado plants against R. necatrix infection. During biocontrol, R. necatrix impacted the microbial communities; however, that impact was reduced by the preventive application of PcPCL1606. The bacterial populations were poorly influenced by R. necatrix introduction and by PcPCL1606 treatment. On the other hand, the severe impact of R. necatrix introduction on fungal communities was partially restored by the preventive PcPCL1606 treatment, which inhibited the development of R. necatrix and other saprophytic families of fungi that finally led to suppressiveness against this fungus. The basis for this protection is directly related to the production of the compound HPR, which confers the suppressive phenotype to the treated soil.
Figure 10. Schematic model for the effect of the preventive application of PcPCL1606. The preventive application of the biocontrol PcPCL1606 strain has no effect on natural prokaryotic and eukariotic populations in the absence of the pathogenic fungus R. necatrix. If R. necatrix was inoculated in the model, it causes the white root rot disease. The presence of R. necatrix disturbed the natural populations and became the predominant fungus. However, when the preventive application of PcPCL1606 take place, resulted in biocontrol of the fungus, reducing its presence and the modification of the microbial communities. A sligh shift in the prokaryotic population was observed, appearing members with potential antifungal activity. And for the eukaryotic communities, reduced the relative abundance of R. necatrix. Allowing the development of other fast-growing well adapted fungi likely to be natural competitors.
The datasets presented in this study can be found in online repositories. The names of the repository/repositories and accession number(s) can be found at: https://doi.org/10.6084/m9.figshare.12310253.v1, 10.6084/m9.figshare.12309788.v1, and https://www.ebi.ac.uk/ena/data/view/ERS4551058-ERS4551081.
ST and FC designed the experiments. EL and SW formulated the bacterium. ST, CV, IL, EG, and JG-F performed the experiments. ST, AV, and FC analyzed the results and wrote the manuscript. All the authors read and approved the final manuscript.
This research was supported by the Spanish Plan Nacional I+D+I. Grants AGL2014-52518-C2-1-R and AGL2017-83368-C2-1-R, and both were partially supported by the European Union (FEDER). CV and ST were supported by a grant from FPI, Ministerio de Ciencia e Innovación, Spain.
EL and SW were employed by Koppert Biological Systems.
The remaining authors declare that the research was conducted in the absence of any commercial or financial relationships that could be construed as a potential conflict of interest.
We wish to thank IHSM-UMA-CSIC “La Mayora” for the technical installations used during this work.
The Supplementary Material for this article can be found online at: https://www.frontiersin.org/articles/10.3389/fmicb.2020.01874/full#supplementary-material
FIGURE S1 | Disease index of white root rot on 2-years old avocado plants during the microcosms assays. 0, healthy plant; 1, plant with first symptoms of wilt; 2, overall wilted plant; 3, wilted plant with first symptoms of leaf desiccation; and 4, completely dried plant (dead plant).
FIGURE S2 | Climatic data during the biocontrol experiments (seasons 2017/18 and 2018/19). Blue bars indicate average relative humidity (HR), and red line indicated average temperature. Data are taken every ten days. Dotted lines indicated season average HR (blue) and temperature (red).
FIGURE S3 | Regression analysis of the bacterial counts of PcPCL1606-GFP growing in Pseudomonas selective medium and in TPG amended with gentamicin.
FIGURE S4 | Effect of formulated PCL1606 application on culturable microbial populations during the biocontrol, taken at T3 during the “assay 2” microcosms experiments. The population densities of fast-growing heterotrophic bacteria and pseudomonads-like were assessed by plate counts at different times (T0, T1, T2, and T3). Bacterial counts from samples inoculated with R. necatrix were showed as +Rn.
FIGURE S5 | Analysis of structure using the Bray-Curtis index of 16S rRNA (A) and ITS (B) sequences from samples of soil/rhizosphere the avocado plant during bicontrol against R. necatrix. Samples analyzed were obtained from the negative control of soil (Control soil) and rhizosphere (Control rhizosphere), and samples from formulated PcPCL1606 preventive treatment of soil (PcPCL1606 preventive soil) and rhizosphere (PcPCL1606 preventive rhizosphere). +Rn: plants inoculated with R. necatrix.
TABLE S1 | Specific primers for specific amplification of Pseudomonas chlororaphis PCL1606 (PcPCL1606) and R. necatrix from soil and rhizosphere DNA.
Arjona-López, J. M., Tienda, S., Arjona-Girona, I., Cazorla, F. M., and López-Herrera, C. J. (2019). Combination of low concentrations of fluazinam and antagonistic rhizobacteria to control avocado white root rot. Biol. Control 136:103996. doi: 10.1016/j.biocontrol.2019.05.015
Arrebola, E., Tienda, S., Vida, C., de Vicente, A., and Cazorla, F. M. (2019). Fitness features involved in the biocontrol interaction of Pseudomonas chlororaphis with host plants: the case study of PcPCL1606. Front. Microbiol. 10:719. doi: 10.3389/fmicb.2019.00719
Baltruschat, H., Fodor, J., Harrach, B. D., Niemczyk, E., Barna, B., Gullner, G., et al. (2008). Salt tolerance of barley induced by the root endophyte Piriformospora indica is associated with a strong increase in antioxidants. New Phytol. 180, 501–510. doi: 10.1111/j.1469-8137.2008.02583.x
Belnap, J., Prasse, R., and Harper, K. T. (2003). “Influence of biological soil crusts on soil environments and vascular plants,” in Biological Soil Crusts: Structure, Function and Management, eds J. Belnap and O. L. Lange (Berlin: Springer-Verlag), 281–300.
Berendsen, R., Pieterse, C., and Bakker, P. (2012). The rhizosphere microbiome and plant health. Trends Plant Sci. 17, 478–486. doi: 10.1016/j.tplants.2012.04.001
Berg, G., Rybakova, D., Grube, M., and Köberl, M. (2016). The plant microbiome explored: implications for experimental botany. J. Exp. Bot. 6, 995–1002. doi: 10.1093/jxb/erv466
Biessy, A., Novinscak, A., Blom, J., Léger, G., Thomashow, L., Cazorla, F. M., et al. (2019). Diversity of phytobeneficial traits revealed by whole genome analysis of worldwide-isolated phenazine-producing Pseudomonas spp. Environ. Microbiol. 21, 437–455. doi: 10.1111/1462-2920.14476
Bolger, A. M., Lohse, M., and Usadel, B. (2014). Trimmomatic: a flexible trimmer for Illumina sequence data. Bioinformatics 30, 2114–2120. doi: 10.1093/bioinformatics/btu170
Bonilla, N., Cazorla, F. M., Maira Martínez-Alonso, M., Hermoso, J. M., González-Fernández, J., Gaju, N., et al. (2012). Organic amendments and land management affect bacterial community composition, diversity and biomass in avocado crop soils. Plant Soil 357, 215–226. doi: 10.1007/s11104-012-1155-1
Bonilla, N., Vida, C., Martínez-Alonso, M., Landa, B. B., Gaju, N., Cazorla, F. M., et al. (2015). Organic amendments to avocado crops induce suppressiveness and influence the composition and activity of soil microbial communities. Appl. Environ. Microbiol. 81, 3405–3418. doi: 10.1128/AEM.03787-14
Buddrus-Schiemann, K., Schmid, M., Schreiner, K., Welzl, G., and Hartmann, A. (2010). Root colonization by Pseudomonas sp. DSMZ 13134 and impact on the indigenous rhizosphere bacterial community of barley. Microbial Ecol. 60, 381–393. doi: 10.1007/s00248-010-9720-8
Calderón, C. E., de Vicente, A., and Cazorla, F. M. (2014). Role of 2-hexyl, 5-propyl resorcinol production by Pseudomonas chlororaphis PCL1606 in the multitrophic interactions in the avocado rhizosphere during the biocontrol process. FEMS Microbiol. Ecol. 89, 20–31. doi: 10.1111/1574-6941.12319
Calderón, C. E., Pérez-garcía, A., de Vicente, A., and Cazorla, F. M. (2013). The dar genes of Pseudomonas chlororaphis PCL1606 are crucial for biocontrol activity via production of the antifungal compound 2-hexyl, 5-propyl resorcinol. Mol. Plant Microbe Interact. 26, 554–565. doi: 10.1094/MPMI-01-13-0012-R
Calderón, C. E., Tienda, S., Heredia-Ponce, Z., Arrebola, E., Cárcamo-Oyarce, G., Eberl, L., et al. (2019). The compound 2-hexyl, 5-propyl resorcinol has a key role in biofilm formation by the biocontrol rhizobacterium Pseudomonas chlororaphis PCL1606. Front. Microbiol. 10:396. doi: 10.3389/fmicb.2019.00396
Campbell, C. L., and Madden, L. V. (1990). Introduction to Plant Disease Epidemiology. New York, NY: Jonh Wiley & Sons, 532.
Carrión, V. J., Perez-Jaramillo, J., Cordovez, V., Tracanna, V., de Hollander, M., Ruiz-Buck, D., et al. (2019). Pathogen-induced activation of disease-suppressive functions in the endophytic root microbiome. Science 366, 606–612. doi: 10.1126/science.aaw9285
Cazorla, F. M., Duckett, S., Bergström, E., Noreen, S., Odijk, R., Lugtenberg, B. J. J., et al. (2006). Biocontrol of avocado Dematophora root rot by antagonistic Pseudomonas fluorescens PCL1606 correlates with the production of 2-hexyl, 5-propyl resorcinol. Mol. Plant Microbe Interact. 19, 418–428. doi: 10.1094/MPMI-19-0418
Cazorla, F. M., Romero, D., Pérez-García, A., Lugtenberg, B. J. J., de Vicente, A., and Bloemberg, G. (2007). Isolation and characterization of antagonistic Bacillus subtilis strains from the avocado rhizoplane displaying biocontrol activity. J. Appl. Microbiol. 103, 1950–1959. doi: 10.1111/j.1365-2672.2007.03433.x
Chao, A. (1987). Estimating the population size for capture-recapture data with unequal catchability. Biometrics 43, 783–791.
Chen, Y., Shen, X., Peng, H., Hu, H., Wang, W., and Zhang, X. (2015). Comparative genomic analysis and phenazine production of Pseudomonas chlororaphis, a plant growth-promoting rhizobacterium. Genom. Data 4, 33–42. doi: 10.1010/j.gdata.2015.01.006
Co-David, D., Langeveld, D., and Noordeloos, M. (2009). Molecular phylogeny and spore evolution of Entolomataceae. Persoonia 23, 147–176. doi: 10.3767/003158509X480944
de Boer, W., Folman, L. B., Summerbell, R. C., and Boddy, L. (2005). Living in a fungal world: impact of fungi on soil bacterial niche development. FEMS Microbiol. Rev. 29, 795–811. doi: 10.1016/j.femsre.2004.11.005
de Boer, W., Hundscheid, M. P., Klein Gunnewiek, P. J., de Ridder-Duine, A. S., Thion, C., van Veen, J. A., et al. (2015). Antifungal rhizosphere bacteria can increase as response to the presence of saprotrophic fungi. PLoS One 10:e0137988. doi: 10.1371/journal.pone.0137988
Deacon, J. W. (1994). “Rhizosphere constraints affecting biocontrol organisms applied to seeds,” in BCPC Monograph 57:-Seed treatment: prospects and progress, (Hampshire: British Crop Protection Council), 315–327. Thornton Heath.
Dey, R., Pal, K. K., and Tilak, K. V. B. R. (2014). “Plant growth promoting rhizobacteria in crop protection and challenge,” in Future Challenges in Crop Protection Against Fungal Pathogens, eds A. Goyal and C. Manoharachary (New York, NY: Springer). doi: 10.1007/978-1-4939-1188-2_2
Donhauser, J., and Frey, B. (2018). Alpine soil microbial ecology in a changing world. FEMS Microbiol. Ecol. 94:fy099. doi: 10.1093/femsec/fiy099
Dorosky, R. J., Yu, J. M., Pierson, L. S. III, and Pierson, E. A. (2017). Pseudomonas chlororaphis produces two distinct R-tailocins that contribute to bacterial competition in biofilms and on roots. Appl. Environ. Microbiol. 83:e00706-17. doi: 10.1128/AEM.00706-17
Edgar, R. C., Haas, B. J., Clemente, J. C., Quince, C., and Knight, R. (2011). UCHIME improves sensitivity and speed of chimera detection. Bioinformatics 27, 2194–2200. doi: 10.1093/bioinformatics/btr381
Fakhro, A., Andrade-Linares, D. R., von Bargen, S. M., Büttner, C., Grosch, R., Schwarz, D., et al. (2010). Impact of Piriformospora indica on tomato growth and on interaction with fungal and viral pathogens. Mycorrhiza 20, 191–200. doi: 10.1007/s00572-009-0279-5
Figueiredo, M. V. B., Seldin, L., Araujo, F. F., and Mariano, R. L. R. (2010). “Plant growth promoting rhizobacteria: fundamentals and applications,” in Plant Growth and Health Promoting Bacteria. Microbiology Monographs, ed. D. K. Maheshwari (Berlin: Springer), 21–43.
Franken, P. (2012). The plant strengthening root endophyte Piriformospora indica: potential application and the biology behind. Appl. Microbiol. Biotechnol. 96, 1455–1464. doi: 10.1007/s00253-012-4506-1
Gao, G., Yin, D., Chen, S., Xia, F., Yang, J., Li, Q., et al. (2012). Effect of biocontrol agent Pseudomonas fluorescens 2P24 on soil fungal community in cucumber rhizosphere using T-RFLP and DGGE. PLoS One 7:e31806. doi: 10.1371/journal.pone.0031806
Gardes, M., and Bruns, T. D. (1993). ITS primers with enhanced specificity for basidiomycetes–application to the identification of mycorrhizae and rusts. Mol. Ecol. 2, 113–118.
Ghimire, S. R., and Craven, K. D. (2011). Enhancement of switchgrass (Panicum virgatum L.) biomass production under drought conditions by the ectomycorrhizal fungus Sebacina vermifera. Appl. Environ. Microbiol. 77, 7063–7067. doi: 10.1128/AEM.05225-11
González-Sánchez, M. Á, de Vicente, A., Pérez-García, A., Pérez-Jiménez, R., Romero, D., and Cazorla, F. M. (2013). Evaluation of the effectiveness of biocontrol bacteria against avocado white root rot occurring under commercial greenhouse plant production conditions. Biol. Control 67, 94–100. doi: 10.1016/j.biocontrol.2013.08.009
González-Sánchez, M. Á, Pérez-Jiménez, R. M., Pliego, C., Ramos, C., de Vicente, A., and Cazorla, F. M. (2010). Biocontrol bacteria selected by a direct plant protection strategy against avocado white root rot show antagonism as a prevalent trait. J. Appl. Microbiol. 109, 65–78.
Gray, E. J., and Smith, D. L. (2005). Intracellular and extracellular PGPR: commonalities and distinctions in the plant-bacterium signaling processes. Soil Biol. Biochem. 37, 395–412. doi: 10.1016/j.soilbio.2004.08.030
Gutiérrez-Barranquero, J. A., Arrebola, E., Bonilla, N., Sarmiento, D., Cazorla, F. M., and de Vicente, A. (2012). Environmentally friendly treatment alternatives to Bordeaux mixture for controlling bacterial apical necrosis (BAN) of mango. Plant Pathol. 61, 665–676. doi: 10.1128/AEM.02644-12
Haas, D., and Défago, G. (2005). Biological control of soil-borne pathogens by fluorescent pseudomonads. Nat. Rev. Microbiol. 3, 307–319. doi: 10.1038/nrmicro1129
Harrach, B. D., Baltruschat, H., Barna, B., Fodor, J., and Kogel, K. H. (2013). The mutualistic fungus Piriformospora indica protects barley roots from a loss of antioxidant capacity caused by the necrotrophic pathogen Fusarium culmorum. Mol. Plant Microbe Interact. 26, 599–605. doi: 10.1094/MPMI-09-12-0216-R
Heck, K. L., van Belle, G., and Simberloff, D. (1975). Explicit calculation of the rarefaction diversity measurement and the determination of sufficient sample size. Ecology 56, 1459–1461. doi: 10.2307/1934716
Herlemann, D. P., Labrenz, M., Jürgens, K., Bertilsson, S., Waniek, J. J., and Andersson, A. F. (2011). Transitions in bacterial communities along the 2000 Km salinity gradient of the Baltic Sea. ISME J. 5, 1571–1579. doi: 10.1038/ismej.2011.41
Hermans, S. M., Buckley, H. L., Case, B. S., Curran-Cournane, F., Taylor, M., and Lear, G. (2017). Bacteria as emerging indicators of soil condition. Appl. Environ. Microbiol. 83:e02826-16. doi: 10.1128/AEM.02826-16
Hiltner, L. (1904). Uber neuere erfahrungen und probleme auf dem gebiete der bodenbakteriologie unter besonderden berucksichtigung und brache. Arb. Dtsch. Landwirtsch. Gesellschaft. 98, 59–78.
Huber, B., Riedel, K., Köthe, M., Givskov, M., Molin, S., and Eberl, L. (2002). Genetic analysis of functions involved in the late stages of biofilm development in Burkholderia cepacia H111. Mol. Microbiol. 2, 411–426. doi: 10.1046/j.1365-2958.2002.03182.x
Johnston, E. R., Hatt, J. K., He, Z., Wu, L., Guo, X., Luo, Y., et al. (2019). Responses of tundra soil microbial communities to half a decade of experimental warming at two critical depths. Proc. Natl. Acad. Sci. U.S.A. 116, 15096–15105. doi: 10.1073/pnas.1901307116
Kerekes, J., Kaspari, M., Stevenson, B., Nilsson, R. H., Hartmann, M., Amend, A., et al. (2013). Nutrient enrichment increased species richness of leaf litter fungal assemblages in a tropical forest. Mol. Ecol. 22, 2827–2838. doi: 10.1111/mec.12259
Köhl, J., Kolnaar, R., and Ravensberg, W. J. (2019). Mode of action of microbial biological control agents against plant diseases: relevance beyond efficacy. Front. Plant Sci. 10:845. doi: 10.3389/fpls.2019.00845
Kröber, M., Wibberg, D., Grosch, R., Eikmeyer, F., Verwaaijen, B., Chowdhury, S. P., et al. (2014). Effect of the strain Bacillus amyloliquefaciens FZB42 on the microbial community in the rhizosphere of lettuce under field conditions analyzed by whole metagenome sequencing. Front. Microbiol. 5:252. doi: 10.3389/fmicb.2014.00252
Kumar, V., Sarma, M. V., Saharan, K., Srivastava, R., Kumar, L., Sahai, V., et al. (2012). Effect of formulated root endophytic fungus Piriformospora indica and plant growth promoting rhizobacteria fluorescent pseudomonads R62 and R81 on Vigna mungo. World J. Microbiol. Biotechnol. 28, 595–603. doi: 10.1007/s11274-011-0852-x
Kupferschmied, P., Maurhofer, M., and Keel, C. (2013). Promise for plant pest control: root-associated pseudomonads with insecticidal activities. Front. Plant Sci. 4:287. doi: 10.3389/fpls.2013.00287
Larkin, R. P., and Honeycutt, C. W. (2006). Effects of different 3-year cropping systems on soil microbial communities and rhizoctonia diseases of potato. Phytopathology 96, 68–79. doi: 10.1094/PHYTO-96-0068
Lasota, S., Stephan, I., Horn, M. A., Otto, W., and Noll, M. (2019). Copper in wood preservatives delayed wood decomposition and shifted soil fungal but not bacterial community composition. Appl. Environ. Microbiol. 85:e02391-18. doi: 10.1128/AEM.02391-18
Ligon, J. M., Hill, D. S., Hammer, P. E., Torkewitz, N. R., Hofmann, D., Kempf, H., et al. (2000). Natural products with antifungal activity from Pseudomonas biocontrol bacteria. Pest Manag. Sci. 56, 688–695. doi: 10.1002/1526-4998(200008)56:8<688::AID-PS186<3.0.CO;2-V
Lladó, S., López-Mondéjar, R., and Baldrian, P. (2017). Forest soil bacteria: diversity, involvement in ecosystem processes, and response to global change. Microbiol. Mol. Biol. Rev. 81:e00063-16. doi: 10.1128/MMBR.00063-16
Lombard, L., van der Merwe, N. A., Groenewald, J. Z., and Crous, P. W. (2015). Generic concepts in Nectriaceae. Stud. Mycol. 80, 189–245. doi: 10.1016/j.simyco.2014.12.002
López-Herrera, C. J., Pérez-Jiménez, R., Llobel, A., Monte-Vázquez, E., and Zea-Bonilla, T. (1999). Estudios in vivo de Trichoderma como agente de biocontrol contra Phytophthora cinnamomi y Rosellinia necatrix en aguacate. Rev. Chapingo Ser. Hortic. 5, 261–265.
López-Herrera, C. J., Pérez-Jiménez, R. M., Zea-Bonilla, T., Basallote-Ureba, M. J., and Melero-Vara, J. M. (1998). Soil solarization in established avocado trees for control of Dematophora necatrix. Plant Dis. 82, 1088–1092. doi: 10.1094/PDIS.1998.82.10.1088
López-Herrera, C. J., and Zea-Bonilla, T. (2007). Effects of benomyl, carbendazim, fluazinam and thiophanate methyl on white root rot of avocado. Crop Protect. 26, 1186–1192. doi: 10.1016/j.cropro.2006.10.015
Lugtenberg, B., and Kamilova, F. (2009). Plant-growth-promoting rhizobacteria. Annu. Rev. Microbiol. 63, 541–556. doi: 10.1146/annurev.micro.62.081307.162918
Lugtenberg, B. J., Dekkers, L., and Bloemberg, G. (2001). Molecular determinants of rhizosphere colonization by Pseudomonas. Annu. Rev. Phytopathol. 39, 461–490. doi: 10.1146/annurev.phyto.39.1.461
Messenger, B., Menge, J. A., and Pond, E. (2000). Effects of gypsum on zoospores and sporangia of Phytophthora cinnamomi in field soil. Plant Dis. 84, 617–621. doi: 10.1094/PDIS.2000.84.6.617
Myers, E. W., and Miller, W. (1988). Optimal alignments in linear space. Bioinformatics 4, 11–17. doi: 10.1093/bioinformatics/4.1.11
Nautiyal, C. S., Chauhan, P. S., DasGupta, S. M., Seem, K., Varma, A., and Staddon, W. J. (2010). Tripartite interactions among Paenibacillus lentimorbus NRRL B-30488, Piriformospora indica DSM 11827, and Cicer arietinum. L. World J Microbiol Biot. 26, 1393–1399. doi: 10.1007/s11274-010-0312-z
O’Callaghan, M. (2016). Microbial inoculation of seed for improved crop performance: issues and opportunities. Appl. Microbiol Biotechnol. 100, 5729–5746. doi: 10.1007/s00253-016-7590-9
Pérez-Jiménez, R. (2008). Significant avocado diseases caused by fungi and oomycetes. Eur. J. Plant Sci. Biotechnol. 2, 1–24.
Pérez-Jiménez, R. M. (1997). Podredumbres Radiculares Del Aguacate (Persea americana Mill.) en el sur de Andalucía. Ph.D. Thesis, Universidad de Málaga, Spain, 370.
Pliego, C., de Weert, S., Lamers, G., De Vicente, A., Bloemberg, G., Cazorla, F. M., et al. (2008). Two similar enhanced root-colonizing Pseudomonas strains differ largely in their colonization strategies of avocado roots and Rosellinia necatrix hyphae. Environ. Microbiol. 10, 3295–3304. doi: 10.1111/j.1462-2920.2008.01721.x
Pliego, C., Kanematsu, S., Ruano-Rosa, D., de Vicente, A., López-Herrera, C., Cazorla, F. M., et al. (2009). GFP sheds light on the infection process of avocado roots by Rosellinia necatrix. Fungal Gen. Biol. 46, 137–145. doi: 10.1016/j.fgb.2008.11.009
Pliego, C., López-herrera, C., Ramos, C., and Cazorla, F. M. (2012). Developing tools to unravel the biological secrets of Rosellinia necatrix, an emergent threat to woody crops. Mol. Plant Pathol. 13, 226–239. doi: 10.1111/j.1364-3703.2011.00753.x
Pliego, C., Ramos, C., De Vicente, A., and Cazorla, F. M. (2011). Screening for candidate bacterial biocontrol agents against soilborne fungal plant pathogens. Plant Soil 340, 505–520. doi: 10.1007/s11104-010-0615-8
Polonio, Á, Vida, C., de Vicente, A., and Cazorla, F. M. (2017). Impact of motility and chemotaxis features of the rhizobacterium Pseudomonas chlororaphis PCL1606 on its biocontrol of avocado white root rot. Int. Microbiol. 20, 95–104. doi: 10.2436/20.1501.01.289
Praeg, N., Pauli, H., and Illmer, P. (2019). Microbial diversity in bulk and rhizosphere soil of Ranunculus glacialis along a high-alpine altitudinal aradient. Front. Microbiol. 10:1429. doi: 10.3389/fmicb.2019.01429
Raaijmakers, J.M., Paulitz, T.C., Steinberg, C., Alabouvette, C., and Moënne-Loccoz, Y. (2009). The rhizosphere: a playground and battlefield for soilborne pathogens and beneficial microorganisms. Plant Soil 321, 321-341. doi: 10.1007/s11104-008-9568-1152 6
Raaijmakers, J. M., Vlami, M., and de Souza, J. T. (2002). Antibiotic production by bacterial biocontrol agents. Anton. Van Leeuw. 81, 537–547. doi: 10.1023/a:1020501420831
Rognes, T., Flouri, T., Nichols, B., Quince, C., and Mahé, F. (2016). VSEARCH: a versatile open source tool for metagenomics. PeerJ 4:e2584. doi: 10.7717/peerj.2584
Ruano-Rosa, D., Del Moral-Navarrete, L., and Lopez-Herrera, C. J. (2010). Selection of Trichoderma spp. isolates antagonistic to Rosellinia necatrix. Spanish J. Agric. Res. 8, 1084–1097. doi: 10.5424/sjar/2010084-1403
Schena, L., Nigro, F., and Ippolito, A. (2002). Identification and detection of Rosellinia necatrix by conventional and real-time Scorpion-PCR. Eur. J. Plant Pathol. 108, 355–366. doi: 10.1023/A:1015697813352
Schreiter, S., Ding, G. C., Heuer, H., Neumann, G., Sandmann, M., Grosch, R., et al. (2014). Effect of the soil type on the microbiome in the rhizosphere of field-grown lettuce. Front. Microbiol. 5:144. doi: 10.3389/fmicb.2014.00144
Serfling, A., Wirsel, S. G. R., Lind, V., and Deising, H. B. (2007). Performance of the biocontrol fungus Piriformospora indica on wheat under greenhouse and field conditions. Phytopathology 97, 523–531. doi: 10.1094/PHYTO-97-4-0523
Shepherd, G. J. (2010). Fitopac 2.1.2.85. Manual do Usuário. Departamento de Botânica. Campinas: UNICAMP, 91.
Steddom, K., Menge, J. A., Crowley, D., and Borneman, J. (2002). Effect of repetitive applications of the biocontrol bacterium Pseudomonas putida 06909-rif/nal on citrus soil microbial communities. Phytopathology 92, 857–862. doi: 10.1094/PHYTO.2002.92.8.857
Sztejnberg, A., and Madar, Z. (1980). Host range of Dematophora necatrix, the cause of white root rot disease in fruit trees. Plant Dis. 64, 662–664. doi: 10.1094/PD-64-662
Thies, J., and Grossman, J. (2006). “The soil habitat and soil ecology,” in Biological Approaches to Sustainable Soil Systems, ed. N. Uphoff A. S. Ball, E. Fernandes, H. Herren, O. Husson, and M. Laing et al. (Boca Raton, FL: CRC Press), 59–78.
van Elsas, J. D., Trevors, J. T., Jain, D., Wolters, A. C., Heijnen, C. E., and van Overbeek, L. S. (1992). Survival of, and root colonization by, alginate-encapsulated Pseudomonas fluorescens cells following introduction into soil. Biol. Fertil. Soils. 14, 14–22. doi: 10.1007/BF00336297
Vida, C., Bonilla, N., de Vicente, A., and Cazorla, F. M. (2016). Microbial profiling of a suppressiveness-induced agricultural soil amended with composted almond shells. Front. Microbiol. 7:4. doi: 10.3389/fmicb.2016.00004
Vida, C., Cazorla, F. M., and de Vicente, A. (2017). Characterization of biocontrol bacterial strains isolated from a suppressiveness-induced soil after amendment with composted almond shells. Res. Microbiol. 168, 583–593.
Vorholt, J. (2012). Microbial life in the phyllopshere. Nat. Rev. Microb. 10, 828–840. doi: 10.1038/nrmicro2910
Waller, F., Achatz, B., Baltruschat, H., Fodor, J., Becker, K., Fischer, M., et al. (2005). The endophytic fungus Piriformospora indica reprograms barley to salt-stress tolerance, disease resistance, and higher yield. Proc. Natl. Acad. Sci. U.S.A. 102, 386–313. doi: 10.1073/pnas.0504423102
Weller, D. M. (2007). Pseudomonas biocontrol agents of soilborne pathogens: Looking back over 30 years. Phytopathology. 97, 250–256. doi: 10.1094/phyto-97-2-0250
Wessendorf, J., and Lingens, F. (1989). Effect of culture and soil conditions on survival of Pseudomonas fluorescens R1 in soil. Appl. Microbiol. Biotechnol. 31, 97–101. doi: 10.1007/BF00252536
Wheeler, T. J., and Eddy, S. R. (2013). nhmmer: DNA homology search with profile HMMs. Bioinformatics 29, 2487–2489. doi: 10.1093/bioinformatics/btt403
Whipps, J. M. (1997). Developments in the biological control of soil-borne plant pathogens. Adv. Bot. Res. 26, 1–134. doi: 10.1016/S0065-2296(08)60119-6
Whipps, J. M., and Davies, K. G. (2000). “Success in biological control of plant pathogens and nematodes by microorganisms,” in Measures of Success in Biological Control, eds G. Gurr and S. D. Wratten (Dordrecht: Kluwer Academic Publishers), 231–269.
Whipps, J. M., and Lumsden, R. D. (2001). “Commercial use of fungi as plant disease biological control agents: status and prospects,” in Fungal Biocontrol Agents Progress, Problems and Potential, eds T. Butt, C. Jackson, and N. Magan (Wallingford: CAB International).
White, T. J., Bruns, T. D., Lee, S. B., and Taylor, J. W. (1990). “Amplification and direct sequencing of fungal ribosomal RNA genes for phylogenetics,” in PCR Protocols: A Guide to Methods and Applications, eds M. A. Innis, D. H. Gelfand, J. J. Sninsky, and T. J. White (Cambridge, MA: Academic Press.), 315–322.
Yin, D., Wang, N., Xia, F., Li, Q., and Wang, W. (2013). Impact of biocontrol agents Pseudomonas fluorescens 2P24 and CPF10 on the bacterial community in the cucumber rhizosphere. Eur. J. Soil Biol. 59, 36–42. doi: 10.1016/j.ejsobi.2013.09.001
Keywords: avocado, Rosellinia necatrix, antifungal, biocontrol, soil, rhizosphere, microbial community, suppressiveness
Citation: Tienda S, Vida C, Lagendijk E, de Weert S, Linares I, González-Fernández J, Guirado E, de Vicente A and Cazorla FM (2020) Soil Application of a Formulated Biocontrol Rhizobacterium, Pseudomonas chlororaphis PCL1606, Induces Soil Suppressiveness by Impacting Specific Microbial Communities. Front. Microbiol. 11:1874. doi: 10.3389/fmicb.2020.01874
Received: 02 April 2020; Accepted: 16 July 2020;
Published: 07 August 2020.
Edited by:
Eligio Malusà, Instytut Ogrodnictwa, PolandReviewed by:
Victor J. Carrion, Leiden University, NetherlandsCopyright © 2020 Tienda, Vida, Lagendijk, de Weert, Linares, González-Fernández, Guirado, de Vicente and Cazorla. This is an open-access article distributed under the terms of the Creative Commons Attribution License (CC BY). The use, distribution or reproduction in other forums is permitted, provided the original author(s) and the copyright owner(s) are credited and that the original publication in this journal is cited, in accordance with accepted academic practice. No use, distribution or reproduction is permitted which does not comply with these terms.
*Correspondence: Francisco M. Cazorla, Y2F6b3JsYUB1bWEuZXM=
Disclaimer: All claims expressed in this article are solely those of the authors and do not necessarily represent those of their affiliated organizations, or those of the publisher, the editors and the reviewers. Any product that may be evaluated in this article or claim that may be made by its manufacturer is not guaranteed or endorsed by the publisher.
Research integrity at Frontiers
Learn more about the work of our research integrity team to safeguard the quality of each article we publish.