- 1Chair of Ecological Microbiology, University of Bayreuth, Bayreuth, Germany
- 2Chair of Hydrogeology, Technical University of Munich, Munich, Germany
- 3Regional University Center of Excellence in Environmental Industry, Szent Istvan University, Gödöllö, Hungary
Microorganisms are essential in the degradation of environmental pollutants. Aromatic hydrocarbons, e.g., benzene, toluene, ethylbenzene, and xylene (BTEX), are common aquifer contaminants, whose degradation in situ is often limited by the availability of electron acceptors. It is clear that different electron acceptors such as nitrate, iron, or sulfate support the activity of distinct degraders. However, this has not been demonstrated for the availability of nitrate vs. nitrite, both of which can be respired in reductive nitrogen cycling. Here via DNA-stable isotope probing, we report that nitrate and nitrite provided as electron acceptors in different concentrations and ratios not only modulated the microbial communities responsible for toluene degradation but also influenced how nitrate reduction proceeded. Zoogloeaceae members, mainly Azoarcus spp., were the key toluene degraders with nitrate-only, or both nitrate and nitrite as electron acceptors. In addition, a shift within Azoarcus degrader populations was observed on the amplicon sequence variant (ASV) level depending on electron acceptor ratios. In contrast, members of the Sphingomonadales were likely the most active toluene degraders when only nitrite was provided. Nitrate reduction did not proceed beyond nitrite in the nitrate-only treatment, while it continued when nitrite was initially also present in the microcosms. Likely, this was attributed to the fact that different microbial communities were stimulated and active in different microcosms. Together, these findings demonstrate that the availability of nitrate and nitrite can define degrader community selection and N-reduction outcomes. It also implies that nitrate usage efficiency in bioremediation could possibly be enhanced by an initial co-supply of nitrite, via modulating the active degrader communities.
Introduction
Aromatic hydrocarbons like benzene, toluene, ethylbenzene, and xylene (BTEX) are among the most common pollutants threatening the quality of groundwater and aquifer ecosystem status (Lueders, 2017). Since BTEX compounds are carcinogenic and neurotoxic to humans, and it is important to understand their behavior and fate in groundwater systems and to develop feasible and effective remediation strategies. Microorganisms can degrade hydrocarbons, including BTEX compounds, aerobically or phototrophically. In environments where the oxygen availability is limited, hydrocarbons can also be degraded anaerobically, carried out by microbes using nitrate, nitrite, sulfate, or iron (III) as electron acceptors or by methanogenic consortia (Rabus et al., 2016). Under anoxic conditions, nitrate and sulfate are the most important soluble electron acceptors used by anaerobic hydrocarbon-degraders in freshwater and marine environments, and a considerable number of enrichments and strains have been cultivated and characterized from different habitats (Widdel et al., 2010). Nearly all denitrifying hydrocarbon-degrading cultures have been obtained using nitrate as electron acceptor. Many of these cultures reduce nitrate via nitrite to nitrogen gas (N2); some others, such as Geobacter metallireducens form ammonia from nitrate (Lovley et al., 1993). There are also cultures reported to only produce and accumulate nitrite, e.g., the naphthalene-degrading Vibrio pelagius and the biphenyl-degrading Citrobacter freundii (Rockne et al., 2000; Grishchenkov et al., 2002).
Nitrate amendment is a frequently used strategy for enhancing bioremediation in-situ, due to its high solubility and ability to support a wide spectrum of hydrocarbon-degrading microbes. For instance, controlled-release of nitrate enhanced phenanthrene-degradation in undisturbed marine sediments, likely through stimulating the indigenous degraders (Tang et al., 2005). Injection of nitrate into a crude oil-contaminated aquifer effectively decreased dissolved hydrocarbon concentrations, including benzene, within 3 months (Ponsin et al., 2014). In anoxic laboratory microcosms of gasoline-impacted soil, nitrate amendment also stimulated toluene, ethylbenzene, and total petroleum hydrocarbon degradation (Yang et al., 2016). However, microbial community responses to such electron acceptor amendments are typically complex and often not well-documented. A study employing functional gene-arrays has reported that, besides N-cycling genes, functional genes involved in carbon-, sulfur-, and phosphorus-cycling were also enriched after nitrate injection to a hydrocarbon contaminated estuarine sediment (Xu et al., 2014), complicating the prediction of specific impacts of electron acceptor amendment.
Nitrate reduction routes can be influenced by many factors, including concentrations of available nitrate and hydrocarbons, indigenous microbial groups, as well as many other environmental parameters (Kraft et al., 2014). Nitrite, as an obligate intermediate of all nitrate reduction pathways, has been reported to transiently accumulate both in laboratory cultures and at bioremediation sites (Kelso et al., 1999; Chayabutra and Ju, 2000; Ponsin et al., 2014). However, nitrite alone or in combination with nitrate is rarely applied in bioremediation, and the impact of nitrite as an electron acceptor on hydrocarbon degradation processes and the selection of active degraders has not been well-investigated. Nevertheless, for denitrifying anaerobic methane oxidizers, it is clear that the concentrations of nitrate and nitrite provided strongly influence the selection and activity of different key players. When similar concentrations of nitrate and nitrite were present as electron acceptors, both methanotrophic archaea, Methanoperedens spp., and NC10 bacteria (Rokubacteria) were enriched and active in methane oxidation (Raghoebarsing et al., 2006). Whereas nitrate alone supports the enrichment of Methanoperedens spp., nitrite favors the activity and growth of NC10 bacteria (Ettwig et al., 2008, 2016; Haroon et al., 2013). In fact, the NC10 bacterium “Candidatus Methylomirabilis oxyfera” is proposed to form its own intracellular oxygen during anaerobic growth with nitrite via NO-dismutation, a process termed oxygenic denitrification (Ettwig et al., 2010). Diverse oxygenic denitrifiers have also been reported from the BTEX-contaminated Siklós aquifer, however, whether they are involved in BTEX-biodegradation remains unclear (Zhu et al., 2017, 2019).
Nitrate is generally preferred in bioremediation, one reason is that by complete denitrification, the same molar amendment of nitrate has 67% more electron accepting capacity than nitrite, due to the different oxidation states of N (Kuypers et al., 2018). In addition, nitrite is very reactive and difficult to detect in the environment, and it may be also toxic to hydrocarbon-degrading microorganisms (Ulrich and Edwards, 2003). Nonetheless, in the environment, nitrate-driven hydrocarbon degradation hardly proceeds via the theoretical stoichiometry and is often incomplete. While oversupply of nitrate not only increases cost, but also can lead to other unwanted consequences, e.g., nitrite accumulation and N2O emissions. Thus, it is important to determine the optimal amount of nitrate needed for supporting in situ biodegradation and, at the same time, avoiding adverse effects of nitrite and nitrogen oxide accumulation. In this study, the influence of nitrate vs. nitrite availability as electron acceptors on the selection of active toluene degrading populations and the progression of nitrate reduction was investigated via a DNA-SIP experiment with 13C7-labeled toluene. Whether oxygenic denitrifiers were possibly involved in the process was also queried. The findings can help to better understand microbial responses to electron acceptor amendments and to optimize nitrate-dependent bioremediation strategies.
Materials and Methods
Sampling Site
Sediment samples were taken in April 2018 from the sump of a monitoring well (ST-2) of a BTEX-contaminated aquifer in Siklós, Hungary. Previous studies have shown that diverse hydrocarbon-degrading microorganisms are present and active in the aquifer (Tancsics et al., 2012, 2013). The sediment was retrieved as previously described (Tancsics et al., 2018).
Microcosm Incubations With 13C7-Toluene
For each microcosm incubation, 5 ml homogenously mixed sediment slurry was transferred into sterile 100 ml serum bottles containing 50 ml of autoclaved artificial groundwater medium (Winderl et al., 2010). Incubations containing about 0.8 mM 13C7-toluene and different electron acceptor combinations were set up in triplicates. To ensure complete toluene oxidation, the total electron-accepting potential of nitrate and nitrite provided was about 50 mmol l−1. According to nitrate/nitrite concentrations provided, the microcosms were designated as the follows: 8/0 microcosm with sole nitrate (8 mM), 5/7 microcosm with nitrate (5 mM) and nitrite (7 mM). 2/12 microcosm with both nitrate (2 mM) and nitrite (12 mM), and 0/18 microcosm with sole nitrite (18 mM). Control microcosms provided with 1 mM unlabeled 12C-toluene and without electron acceptor amendment were used to exclude aerobic toluene degradation. All serum bottles were sealed with air tight stoppers and sparged aseptically with N2/CO2 (80:20, v/v) for 5 min. Toluene was then added by injection with a glass syringe after headspace sparging. The initial pH of all incubations was ~7.3. The bottles were incubated at room temperature on a shaker at 100 rpm for 11 days. To avoid unspecific labeling via cross feeding, one replicate of each treatment was sacrificed for sediment sampling on the 7th day, when most toluene was consumed in nearly all incubations. The remaining duplicate serum bottles were further incubated for one more week.
Toluene, Nitrate, and Nitrite Quantification
Toluene concentrations were measured via headspace analysis on a Trace DSQ GC/MS (Thermo Electron, Germany) equipped with a Combi PAL autosampler (CTC Analytics, Switzerland) as previously described (Avramov et al., 2013). A DB5-MS capillary column (Agilent Technologies, Germany) and helium as carrier gas at a flow rate of 1 ml min−1 were used. Briefly, 1 ml of slurry was taken with glass syringe from each serum bottle and transferred to a 2 ml vial. Ethylbenzene was added with a glass syringe to a final concentration of 2.3 mg l−1 as internal standard, and then the vial was immediately capped. Standard vials containing different concentrations of toluene (0, 0.25, 0.5, 0.75, 1.0, and 1.5 mM) but with the same amount of internal standard were prepared in the same way. Both the sample and standard vials were shaken for 17 min at 70°C to reach toluene liquid-headspace equilibrium. Then, 100 μl of headspace gas was injected into the GC-MS. Toluene concentrations were calculated according to the calibration standard.
Liquid samples filtered through a 0.45 μm filter (Millex-GP; Merck Milipore, Germany) were used for nitrate and nitrite quantification with ion chromatography using a coupled Dionex ICS 1100 system, equipped with an AS4A 4 × 250 mm and a CS12 A 4 × 250 mm column for anions and cations, respectively.
DNA Extraction and Ultracentrifugation
In order to identify which microbes were involved in toluene degradation by DNA-SIP, one representative incubation from all, except the 2/12 microcosms, was sacrificed for DNA extraction at day 7, when most of added toluene was degraded. Since the 5/7 and the 2/12 microcosms showed similar pattern of activity, only the 5/7 microcosm was used for representing the condition of both nitrate and nitrite present as electron acceptor. Sediment DNA was isolated as previously described (Pilloni et al., 2012) with minor modifications. The final DNA precipitation was done at 4°C instead of 20°C. About 0.4 g sediment (wet weight) was used for each DNA extraction, and two extractions were performed from each sample. DNA was quantified with the Quant-iT PicoGreen dsDNA Assay Kit (Thermo Fisher, Waltham, USA) on an MX3000p cycler (Agilent, Santa Clara, USA), and checked by standard agarose gel electrophoresis. Two DNA extracts from the same sample were pooled and stored at −20°C until gradient centrifugation.
Ultracentrifugation, fractionation and DNA recovery were performed as previously described (Lueders, 2015). Approximately 1 μg of DNA extract was loaded onto a gradient medium of CsCl (average density 1.72 g ml−1, Calbiochem, Darmstadt, Germany) in gradient buffer (0.1 M Tris-HCl at pH 8, 0.1 M KCl, and 1 mM EDTA). The sealed polyallomer tubes (QuickSeal, Beckman, California) were centrifuged at 44,500 rpm (184,000 g) for about 48 h as previously described (Lueders, 2015). A total of 12 fractions (each ca. 380–400 μl) from each gradient were collected from “heavy” to “light.” Refractory index of each fraction was measured with refractometer (DR201-95, Krüss, Hamburg, Germany), and buoyant density was calculated based on reference standards. DNA in gradient fractions were precipitated with two volume PEG, washed with 150 μl ice-cold 70% ethanol, and then resuspended in 25 μl elution buffer and stored at −20°C.
qPCR and Amplicon Sequencing
The distribution of density-resolved DNA over gradient fractions was quantified via 16S rRNA gene qPCR using bacterial primers Ba519F and Ba907R (Winderl et al., 2008). To test for the potential involvement of oxygenic denitrifiers in toluene degradation, putative nitric oxide dismutase (nod) genes were also quantified over gradient fractions using primer pair nod1446F and nod1706Rv2 (Zhu et al., 2017). qPCR was performed as previously described (Zhu et al., 2020).
For each gradient, DNA from representative light and heavy fractions with highest 16S rRNA and nod gene counts were selected for amplicon sequencing. 16S rRNA gene V4 amplicons were generated with the universal 16S primer pair 515F and 806R (Walters et al., 2016) from selected light and heavy gradient fractions. Sequencing libraries were prepared with the Nextera XT v2 kit and were sequenced on an Illumina iSeq-100. The sequencing was done in the Genomics and Bioinformatics Key Lab of Bayreuth University.
Sequencing Data Handling and Phylogenetic Analysis
Amplicon data were processed and analyzed with the DADA2 package in R, including quality filtering, denoizing, chimera removal, amplicon sequence variant (ASV) inference, and taxonomic assignment as previously described (Callahan et al., 2016, 2019). Abundant ASVs (≥2%) were aligned with reference 16S rRNA sequences with the ClustralW algorithm with default settings, and based on the alignment, a phylogenetic tree was constructed using the neighbor-joining method in MEGA-X. The robustness of the tree topology was tested by bootstrap analysis (1,000 replicates).
Nucleotide Sequence Deposition
Sequencing data are available at NCBI with SRA accession: PRJNA631140.
Results
Toluene Degradation and Nitrate, Nitrite Reduction
For the 8/0, 5/7, and 2/12 treatments, toluene was largely degraded within one week (Figure 1). In the 0/18 microcosms, the onset of toluene degradation was delayed, only a small part of toluene was degraded in the first week. No loss of toluene was observed in the non-electron acceptor control microcosms (data not shown). In the 8/0 microcosms, all nitrate appeared to be reduced to an equal molar amount of nitrite, which accumulated over the incubation period (Figure 1). In the 5/7 microcosms, where both nitrate and nitrite were provided, it seemed that nitrite rather than nitrate was first reduced. Nitrate reduction only started on the 3rd day, with a concomitant accumulation of nitrite, which then was further reduced. However, at the end of the incubation, nitrite was close to its initial concentration of ~7 mM (Figure 1). Similarly, in the 2/12 microcosms, nitrite seemed to be reduced first, and nitrate reduction started on the 3rd day, leading to a minor increase of nitrite concentration. Then both nitrate and nitrite were consumed simultaneously. In contrast, nitrite reduction in the 0/18 microcosm was insignificant in the 1st week, both toluene and nitrite were only markedly reduced afterwards.
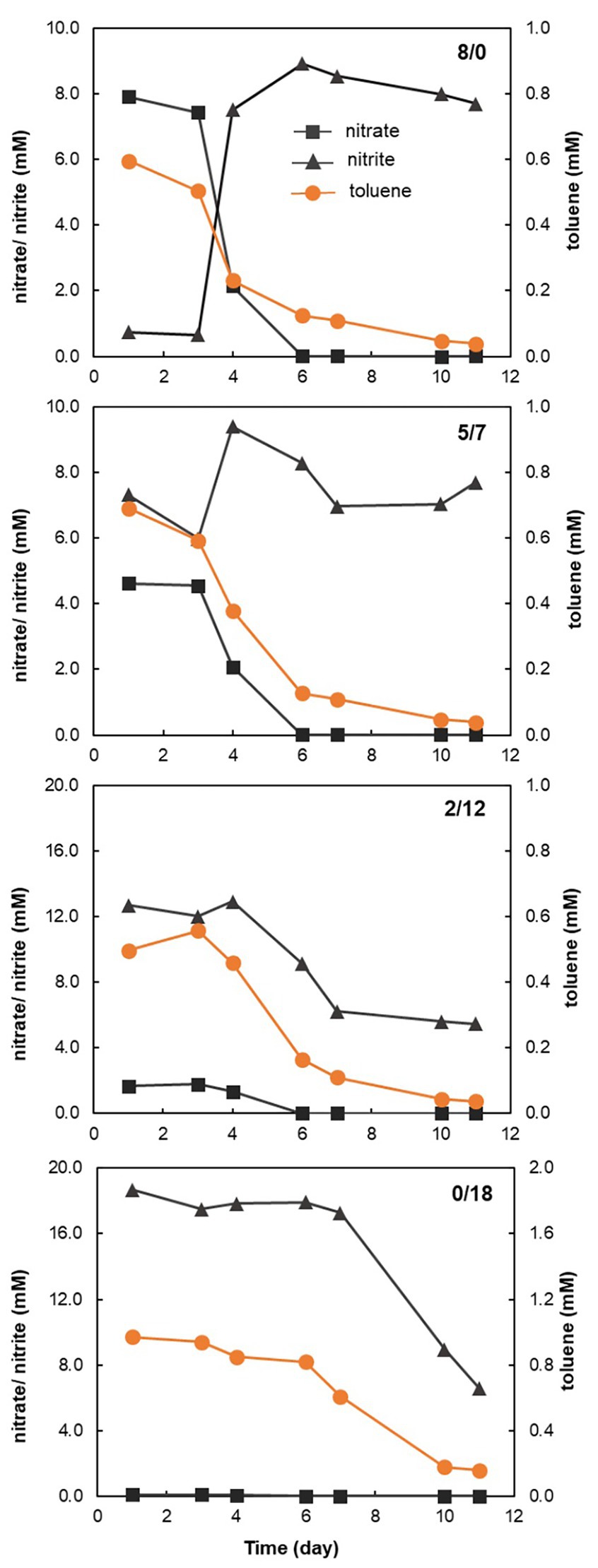
Figure 1. Activity of nitrate, nitrite reduction and toluene oxidation in all except the control treatment microcosms. The first 7 days data are the average of three replicate incubations, while the other data points are the average of the two rest incubations. The 8/0 panel represents the results of the microcosm contained 8/0 mM (nitrate/nitrite) as electron acceptors; the 5/7 panel represents the results of the microcosm contained 5/7 mM (nitrate/nitrite) as electron acceptors; the 2/12 panel represents the results of the microcosm contained 2/12 mM (nitrate/nitrite) as electron acceptors; and the 0/18 panel represents the results of the microcosm contained 0/18 mM (nitrate/nitrite) as electron acceptors.
Distribution of Total Bacteria and Oxygenic Denitrifiers in Density Gradients
One microcosm from each of the 8/0, 5/7, 0/18, and control treatments was sacrificed on day 7 for DNA-SIP analysis. To check how DNA was allocated along SIP density gradients, 16S rRNA targeted qPCR was performed on DNA recovered from all fractions of the 8/0, 5/7, 0/18, and control microcosms. Except the control, two 16S rRNA abundance peaks were present in light and heavy fractions, respectively (Figure 2). Especially abundant peaks of heavy DNA indicative of efficient 13C-labeling were detected in heavy fractions between 1.73 and 1.74 g ml−1, in the 8/0 and 5/7 gradients. The 0/18 microcosm also showed a peak of bacterial qPCR counts at 1.73 g ml−1, but it was an order of magnitude lower compared to the previous treatments. In the control treatment, the peak abundance of DNA was detected in light fractions at 1.70 g ml−1, with a minor peak also tailing into heavier fractions. This indicated that 13C-labeling and density-based DNA separation was successful.
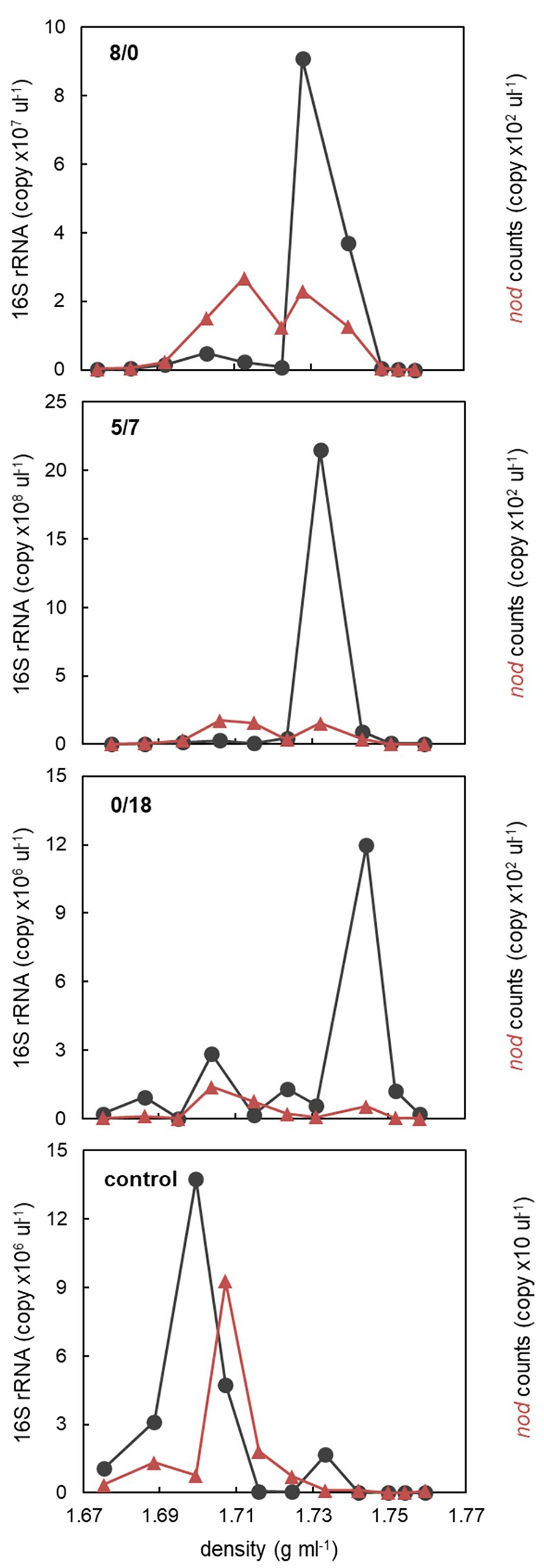
Figure 2. The abundance distribution of all bacteria (16S rRNA) and oxygenic denitrifiers (nod) along the density gradients of the 8/0, 5/7, 0/18, and the control microcosms. For each microcosm, DNA recovered from the fractions with highest 16S rRNA or nod counts at both light and heavy densities were used for amplicon sequencing analysis.
In order to check if oxygenic denitrifiers were also detectable in SIP gradients, putative nod genes were also quantified by qPCR in gradient fractions. In all 13C7-toluene microcosms, nod genes appeared to be distributed over two peaks across lighter and heavier fractions, but with a much lower absolute abundance (<2 × 102 copy μl−1) compared to that of 16S rRNA. Only one nod peak was found in the light fractions of the control SIP gradient (Figure 2). This indicates that some nod-carrying microbes, if not all, also assimilated 13C from amended toluene.
Identification of Labeled Bacteria by DNA-SIP
Representative light and heavy SIP fractions with high 16S rRNA gene counts were selected for amplicon sequencing. Eight amplicon libraries were sequenced, resulting in ≥50,000 reads for each library. After quality control and assembly, between ~34,000 and 54,000 nonchimeric reads remained. From these, 686 amplicon sequence variants (ASVs) were inferred in total. Only 34 of these ASVs were detected at a relative abundance ≥2% in at least one library.
For each microcosm, more ASVs were detected in the light fractions than in the heavy ones. In the 8/0 and 5/7 microcosms, ASVs associated with members of the Zoogloeaceae were clearly enriched in the heavy DNA, accounting for up to 68 and 41% of the total community, respectively (Figure 3). Seven ASVs were found within the Zoogloeaceae, with five (ASV2, 4, 13, 24, and 38) representing Azoarcus spp., a well-known nitrate-reducing toluene degrader. The other two (ASV45 and 50) were from unclassified Zoogloeaceae. A phylogenetic tree was inferred for abundant ASVs, showing that the five Azoarcus ASVs were closely clustered, while ASV45 and 50 branched slightly more deeply (Figure 4). Azoarcus ASV4 was of very low abundance (0.1–0.4%) in all light fractions of 13C-toluene treatments. However, it accounted for about 25 and 12% in the heavy DNA of the 8/0 and 5/7 microcosms, respectively, but it was nearly absent (<0.1%) in the heavy DNA of the 0/18 microcosm with only nitrite as electron acceptor.
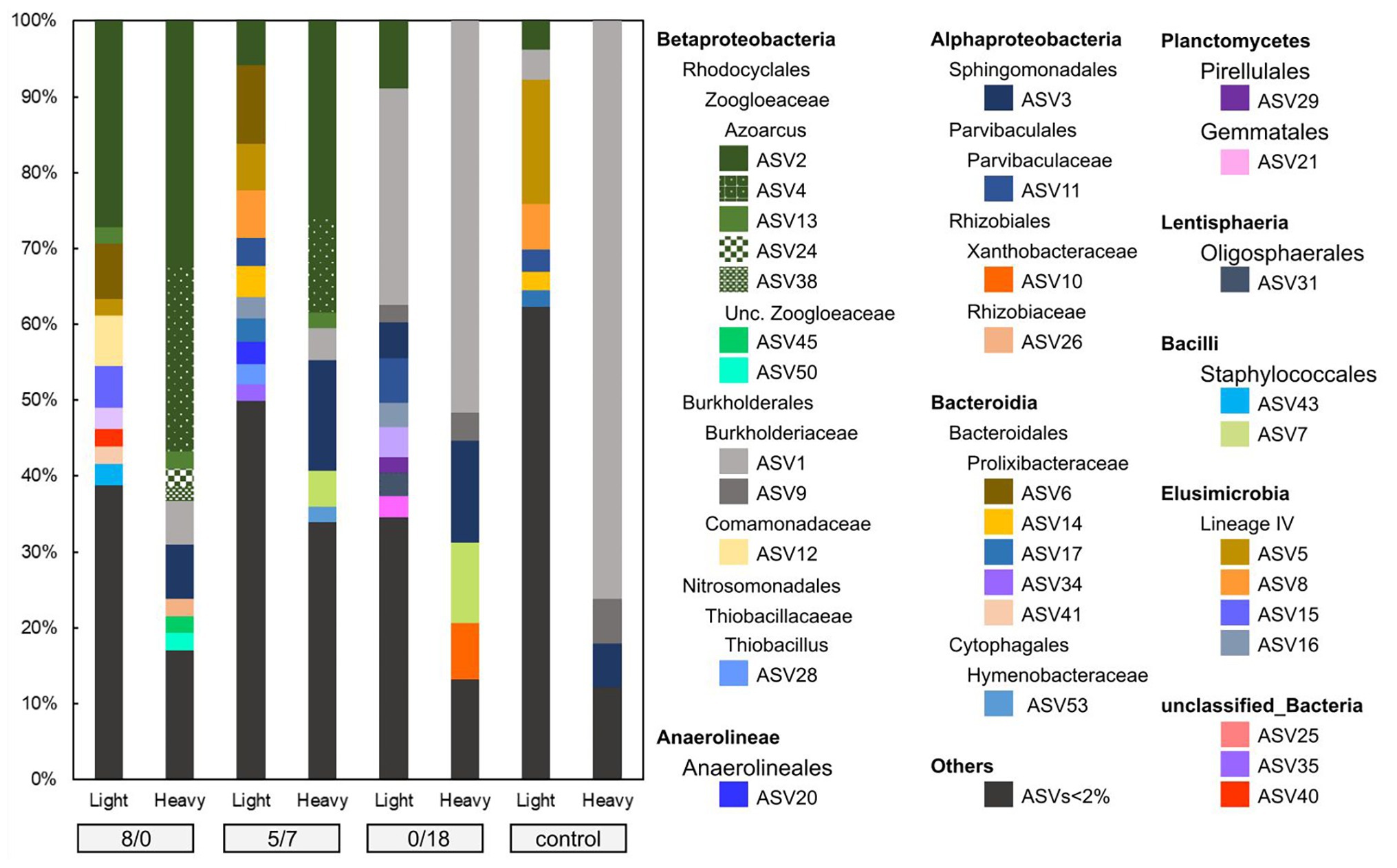
Figure 3. ASVs (amplicon sequencing variants) diversity in light and heavy fractions of the 8/0, 5/7, 0/18, and the control microcosms. ASVs with relative abundance <2% were not individually displayed.
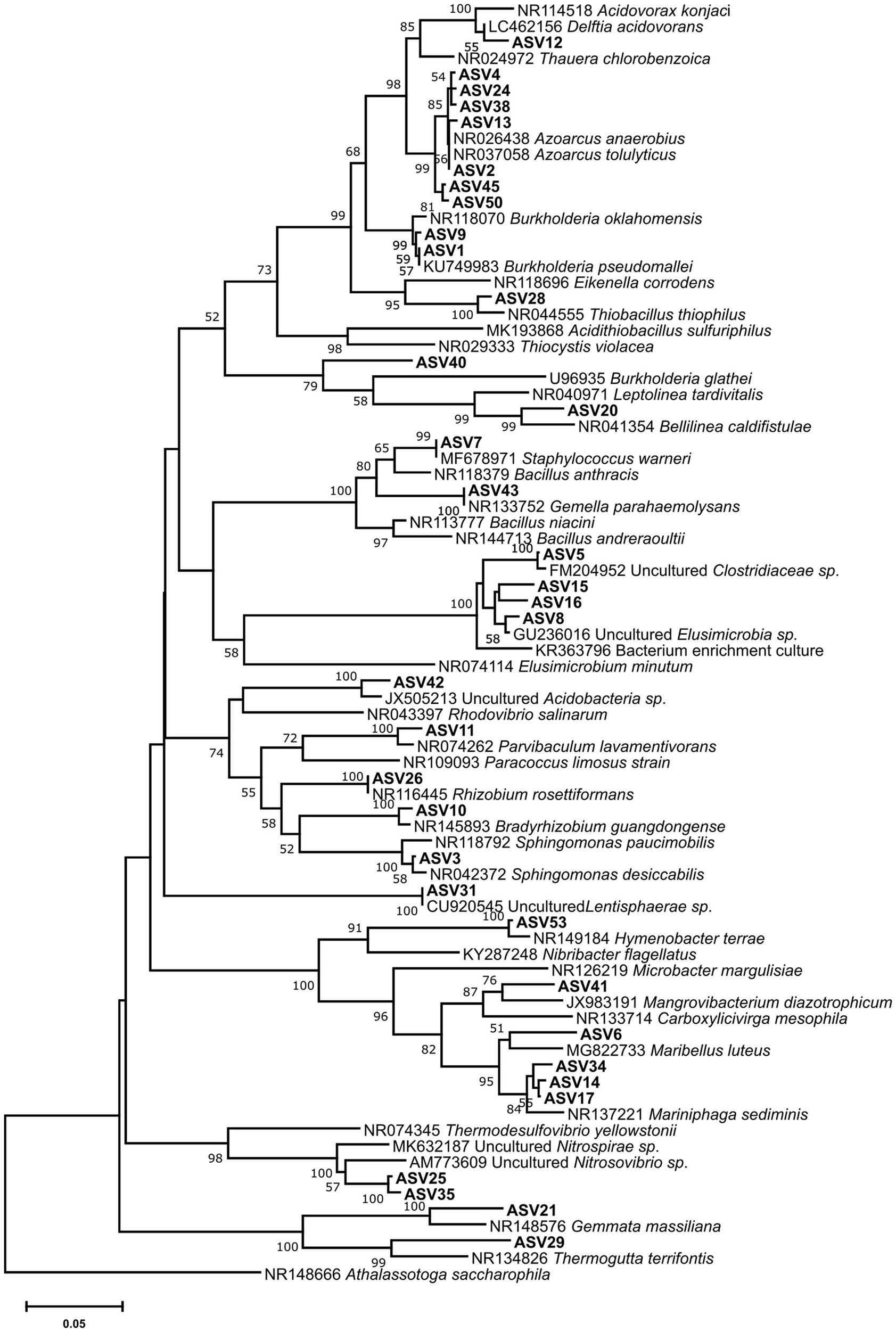
Figure 4. Bootstrapped neighbor-joining phylogeny of abundant (≥2%) ASVs and reference 16S rRNA sequences. Bootstrap support (1,000 replicates) >50% is indicated at the nodes. The scale bar represents 5% nucleotide sequence divergence. The phylogeny was calculated in MEGA-X based on the V4 region alignment using default parameters.
In the control and the 0/18 microcosms, ASV1 appeared most abundant in heavy DNA, accounting for up to ca. 76 and 52% of their total community, respectively (Figure 3). ASV1 and ASV9 both belonged to the Burkholderiaceae family and were closely related to Burkholderia pseudomallei and Burkholderia oklahomensis (Figure 4), both of which are not known for a capacity to degrade aromatic hydrocarbons (Limmathurotsakul et al., 2016). At the same time, ASV3, representing a species in the Sphingomonadales, was especially abundant in heavy fractions of the 5/7 and 0/18 13C-microcosms, while was of very low abundance (<1%) in the corresponding light fractions. This lineage is known to host many toluene degrading bacteria, albeit mostly aerobic degraders (Kertesz et al., 2017).
Discussion
Toluene can be oxidized to CO2 by many denitrifiers. Full oxidation of 1 mmol of toluene to CO2 yields 36 mmol of electrons, while the full reduction of 1 mmol of nitrate or nitrite to N2 requires 5 and 3 mmol electrons, respectively. Within the 1st week, about 0.5 mM toluene was oxidized in the 8/0 microcosm, while about 8 mM nitrate was reduced to nitrite, which was not further reduced during prolonged incubation (Figure 1). This can be explained by the fact that Azoarcus spp. were the dominant toluene-degraders in these microcosms (Figure 3), which have been reported to accumulate nitrite from nitrate under toluene limited conditions (Chee-Sanford et al., 1996). Nevertheless, based on the electron balance, toluene was fully oxidized to CO2 in these treatments.
In the 8/0 treatment, where no nitrite was provided, nitrate reduction did not proceed beyond nitrite. Intriguingly, when nitrate and nitrite were provided simultaneously (the 5/7 and 2/12 microcosms), nitrate appeared to be fully reduced within 6 days (Figure 1). In the 5/7 microcosm, nitrite but not nitrate appeared to be consumed initially, while nitrate reduction to nitrite started after the 3rd day, concurrent with a transiently increased nitrite concentration. However, in the end of incubation, nitrite concentration was similar to the initial value, indicating there was no net nitrite reduction. Possibly, this was observed since 5 mM of nitrate (by reduction to N2) is sufficient to fully oxidize 0.7 mM of toluene to CO2 (Figure 1). While in the 2/12 microcosm, nitrate alone was not enough to oxidize the toluene, so nitrite was also consumed along with nitrate depletion (Figure 1). Previous studies have indicated that nitrite vs. nitrate concentration and C/N ratio are important controls on nitrate/nitrite reduction pathways (Dong et al., 2009; Kraft et al., 2014). Although the end product of nitrate and nitrite reduction in these microcosms remained unclear, the progression of nitrate reduction was clearly influenced by the presence of nitrite. When nitrite was provided as the sole electron acceptor (the 0/18 microcosm), both nitrite reduction and toluene oxidation showed a long lag phase and the activity seemed to pick up only after day 6 (Figure 1). Presumably, the toxicity of nitrite in high concentrations could have caused this delay (Chayabutra and Ju, 2000; Ulrich and Edwards, 2003). Denitrifying hydrocarbon-degrading cultures have mostly been studied under nitrate reducing conditions to date (Widdel et al., 2010). The influence of nitrite availability, an obligate intermediate of nitrate reduction, on hydrocarbon degradation rates and degrader selection has hardly been investigated. Here, we demonstrate that degradation activities are clearly affected by nitrite. In further studies, more detailed and fine-tuned nitrite amendments could be tested to determine optimal combinations of nitrate and nitrite, which can improve total electron acceptor usage efficiency while avoiding nitrite toxicity.
Stable isotope probing is a powerful tool for dissecting active pollutant degraders in complex communities. Due to assimilation of heavier stable isotopes, genomes of the active degraders become enriched in heavy fractions of DNA gradients (Vogt et al., 2016). In our study, the community composition of ASVs in the heavy fractions of different treatments were clearly distinct (Figure 3), indicating that different degrader lineages were active in our treatments. In the 8/0 microcosm, Azoarcus spp. of the Zoogloeaceae within Betaproteobacteria were dominant and identified as the key toluene degraders (Figure 3). While in the 5/7 microcosm, with both nitrate and nitrite as electron acceptors, Zoogloeaceae as well as Sphingomonadales (ASV3) dominated the heavy fractions, both likely representing active key populations. Some members of the Sphingomonadales are known as aerobic, but not anaerobic aromatic hydrocarbon degraders, e.g., the recently described Sphingobium aquiterrae and Sphingobium terrigena (Revesz et al., 2018; Park et al., 2019). However, Sphingomonas mucosissima, which closely relate to ASV3 (Figure 4), has not been demonstrated for toluene degradation (Reddy and Garcia-Pichel, 2007). In our study, ASV3 microbes were among the most significantly labeled groups, suggesting an important role in denitrifying toluene oxidation. However, isolation and cultivation of an ASV3 bacterium will be needed to confirm its function in hydrocarbon degradation.
In both the 8/0 and the 5/7 microcosms, Azoarcus spp. were the dominant toluene degraders. However, the Azoarcus-related ASVs (ASV2, 4, 12, 24, and 38) were heterogeneously distributed. Relative abundance of Azoarcus ASV4, rather than ASV2, shifted strongly with changing electron acceptors. These ASVs are phylogenetically closely clustered together (Figure 4), and they would have been classified as one OTU when using the common 97% similarity cutoff. Thus, within-genus response to electron acceptor availability was only apparent via ASVs resolution. This further illustrates the advantage of using ASVs for environmental sequencing data analysis (Callahan et al., 2017).
Although ASV1, belonging to the Burkholderiaceae, was extremely abundant in the 0/18 microcosm heavy DNA, we do not assume it to be involved in toluene degradation. ASV1 also dominated heavy DNA of the control microcosm (Figure 3), where only 12C-toluene was provided. The genome of B. pseudomallei, closely related to ASV1 (Figure 4), is known to have a high G + C content of 68% (Holden et al., 2004). According to the linear relationship between DNA buoyant density (g ml−1) and G + C content (Buckley et al., 2007), ASV1 and B. pseudomallei DNA with a 68% G + C content, could have an intrinsic buoyant density of >1.72 g ml−1. This was similar to the density of heavy fractions, where ASV1 was found in the control microcosm. Thus likely, the appearance of ASV1 in heavy DNA was related to a G + C effect, rather than 13C-labeling. Very likely, 13C-labeling of active degraders would have been more clearly evident in SIP gradients loaded with 0/18 DNA extracted after 2 weeks of incubation. Unfortunately, this analysis was not conducted in the present work.
Distinct toluene-degrading microbial communities thus were labeled in microcosms with different electron acceptor amendments. The labeled groups were different to a previous DNA-SIP incubation probing toluene-degraders under hypoxic condition, albeit using sediments from the same site (Tancsics et al., 2018). Quatrionicoccus spp. were dominantly labeled under hypoxia, accounting for about 56% of all the bacterial community in the heavy fraction, followed by Zoogloea spp. (~14%) and Rhodoferax (~11%), while Azoarcus spp. were hardly labeled (Tancsics et al., 2018). This indicates that the Siklós aquifer harbors diverse respiratory groups of degraders, capable of functioning under various electron acceptor availability. Various microbes capable of denitrifying toluene degradation were isolated over the last decades, many of them affiliated to Azoarcus (Weelink et al., 2010). Recently, the genus Azoarcus has been reclassified from belonging to the previous Rhodocyclaceae to the new Zoogloeaceae family (Boden et al., 2017). The Azoarcus ASVs identified in our study closely relate to Azoarcus anaerobius and Azoarcus tolulyticus (Figure 4). However, based on the relatively short 16S rRNA reads from Illumina sequencing, a robust phylogenetic placement of these ASVs is not possible. Nevertheless, the differential pattern of nitrate and nitrite reduction observed in our microcosms could largely relate to different active degrader communities being stimulated and selected (Figure 3). The ratios of C/N and electron donors to acceptors could also have been important (Dong et al., 2009) and future studies should take this into account. Nonetheless, a small amendment of nitrite, in addition to nitrate, could activate indigenous microbes to more readily conduct full denitrification, resulting in a community capable of more efficiently using available electron acceptors. Therefore, providing both nitrate and nitrite to the contaminant degrading microbes could possibly increase bioremediation efficacy. Which ratios work the best, however, still requires further investigation.
Oxygenic denitrifiers are microbes proposed to form intracellular oxygen via nitric oxide dismutation. Previously, oxygenic denitrifiers have been suggested to be able to oxidize methane and long-chain alkanes, however, whether they can be involved in aromatic hydrocarbon degradation is still unknown (Zhu et al., 2017). It has been shown that oxygenic denitrifiers were present in the Siklós aquifer, accounting for a few percent of total bacterial gene counts in the sediment (Zhu et al., 2017, 2019). Transcripts of the nitric oxide dismutase (nod) gene, a functional marker for oxygenic denitrifiers were detected, albeit in extremely low numbers, in the labeled mRNA pool from a hypoxic microcosm, using Siklós aquifer sediment as inoculum and 13C7-toluene as substrate (Bradford et al., 2018). In the present work, the distribution of nod genes in SIP gradients indicated that oxygenic denitrifiers were also partially 13C-labeled. However, whether they directly degraded toluene or just assimilated metabolites from dominating degraders is not clear. Furthermore, nod abundance was very low, 5–6 orders of magnitude lower than that of 16S rRNA genes in respective DNA fractions. This indicates that oxygenic denitrifiers were not important in toluene degradation in these microcosms. Unlike the methane-driven oxygenic denitrifying NC10 bacteria, which have a strong preference for nitrite over nitrate (Ettwig et al., 2010), the abundance of labeled nod genes in our study did not show such a pattern. Further research for demonstrating the catabolic capacities of putative oxygenic denitrifiers is thus still needed.
Conclusions
In this study, we demonstrated that nitrate and nitrite as electron acceptors influenced active toluene-degrading microbial populations in aquifer sediments. Members of the Zoogloeaceae were identified as key toluene degraders in microcosms with the presence of nitrate, while members of the Sphingomonadales were likely to be more important with increasing nitrite availability. Intra-genus variability of Azoarcus ASVs as a response to nitrate and nitrite was also demonstrated for the first time. The availability of nitrite also affected the outcomes of nitrate reduction. Our findings suggest a possibly overlooked beneficial role of nitrite in bioremediation. Via modulating active microbial communities, nitrite can enhance overall electron acceptor usage in sites, where nitrate is amended for pollutant degradation. This could offer new routes for optimizing future bioremediation strategies.
Data Availability Statement
Sequencing data are available at NCBI with SRA accession: PRJNA631140.
Author Contributions
BZ and TL designed the project. SF, BZ, and ZW performed the experiment. BZ analyzed the data with help from SF, ZW, AT, and TL. BZ wrote the manuscript with inputs from all authors. All authors contributed to the article and approved the submitted version.
Funding
This research was funded by the European Research Council (ERC) under the European Union’s Seventh Framework Program (FP7/2007-2013), grant agreement 616644 (POLLOX) to TL. ZW was supported by the Collaborative Research Center 1253 CAMPOS (P2: Sub-Catchments) and funded by the German Research Foundation (DFG, grant agreement SFB 1253/1).
Conflict of Interest
The authors declare that the research was conducted in the absence of any commercial or financial relationships that could be construed as a potential conflict of interest.
Acknowledgments
We greatly acknowledge the support of the Genomics Key Lab of the University of Bayreuth in NGS analysis.
References
Avramov, M., Schmidt, S. I., and Griebler, C. (2013). A new bioassay for the ecotoxicological testing of VOCs on groundwater invertebrates and the effects of toluene on Niphargus inopinatus. Aquat. Toxicol. 130, 1–8. doi: 10.1016/j.aquatox.2012.12.023
Boden, R., Hutt, L. P., and Rae, A. W. (2017). Reclassification of Thiobacillus aquaesulis (Wood & Kelly, 1995) as Annwoodia aquaesulis gen. nov., comb. nov., transfer of Thiobacillus (Beijerinck, 1904) from the Hydrogenophilales to the Nitrosomonadales, proposal of Hydrogenophilalia class. nov. within the ‘Proteobacteria’, and four new families within the orders Nitrosomonadales and Rhodocyclales. Int. J. Syst. Evol. Microbiol. 67, 1191–1205. doi: 10.1099/ijsem.0.001927
Bradford, L. M., Vestergaard, G., Táncsics, A., Zhu, B., Schloter, M., and Lueders, T. (2018). Transcriptome-stable isotope probing provides targeted functional and taxonomic insights into microaerobic pollutant-degrading aquifer microbiota. Front. Microbiol. 9:2696. doi: 10.3389/fmicb.2018.02696
Buckley, D. H., Huangyutitham, V., Hsu, S. F., and Nelson, T. A. (2007). Stable isotope probing with 15N achieved by disentangling the effects of genome G+C content and isotope enrichment on DNA density. Appl. Environ. Microbiol. 73, 3189–3195. doi: 10.1128/AEM.02609-06
Callahan, B. J., McMurdie, P. J., and Holmes, S. P. (2017). Exact sequence variants should replace operational taxonomic units in marker-gene data analysis. ISME J. 11, 2639–2643. doi: 10.1038/ismej.2017.119
Callahan, B. J., McMurdie, P. J., Rosen, M. J., Han, A. W., Johnson, A. J., and Holmes, S. P. (2016). DADA2: high-resolution sample inference from Illumina amplicon data. Nat. Methods 13, 581–583. doi: 10.1038/nmeth.3869
Callahan, B. J., Wong, J., Heiner, C., Oh, S., Theriot, C. M., Gulati, A. S., et al. (2019). High-throughput amplicon sequencing of the full-length 16S rRNA gene with single-nucleotide resolution. Nucleic Acids Res. 47:e103. doi: 10.1093/nar/gkz569
Chayabutra, C., and Ju, L. K. (2000). Degradation of n-hexadecane and its metabolites by Pseudomonas aeruginosa under microaerobic and anaerobic denitrifying conditions. Appl. Environ. Microbiol. 66, 493–498. doi: 10.1128/AEM.66.2.493-498.2000
Chee-Sanford, J. C., Frost, J. W., Fries, M. R., Zhou, J., and Tiedje, J. M. (1996). Evidence for acetyl coenzyme a and cinnamoyl coenzyme a in the anaerobic toluene mineralization pathway in Azoarcus tolulyticus Tol-4. Appl. Environ. Microbiol. 62, 964–973. doi: 10.1128/AEM.62.3.964-973.1996
Dong, L. F., Smith, C. J., Papaspyrou, S., Stott, A., Osborn, A. M., and Nedwell, D. B. (2009). Changes in benthic denitrification, nitrate ammonification, and anammox process rates and nitrate and nitrite reductase gene abundances along an estuarine nutrient gradient (the Colne estuary, United Kingdom). Appl. Environ. Microbiol. 75, 3171–3179. doi: 10.1128/AEM.02511-08
Ettwig, K. F., Butler, M. K., Le Paslier, D., Pelletier, E., Mangenot, S., Kuypers, M. M., et al. (2010). Nitrite-driven anaerobic methane oxidation by oxygenic bacteria. Nature 464, 543–548. doi: 10.1038/nature08883
Ettwig, K. F., Shima, S., van de Pas-Schoonen, K. T., Kahnt, J., Medema, M. H., Op den Camp, H. J., et al. (2008). Denitrifying bacteria anaerobically oxidize methane in the absence of Archaea. Environ. Microbiol. 10, 3164–3173. doi: 10.1111/j.1462-2920.2008.01724.x
Ettwig, K. F., Zhu, B. L., Speth, D., Keltjens, J. T., Jetten, M. S. M., and Kartal, B. (2016). Archaea catalyze iron-dependent anaerobic oxidation of methane. Proc. Natl. Acad. Sci. U. S. A. 113, 12792–12796. doi: 10.1073/pnas.1609534113
Grishchenkov, V. G., Slepen’kin, A. V., and Boronin, A. M. (2002). Anaerobic degradation of biphenyl by the facultative anaerobic strain Citrobacter freundii BS2211. Appl. Biochem. Microbiol. 38, 125–128. doi: 10.1023/A:1014350230764
Haroon, M. F., Hu, S., Shi, Y., Imelfort, M., Keller, J., Hugenholtz, P., et al. (2013). Anaerobic oxidation of methane coupled to nitrate reduction in a novel archaeal lineage. Nature 500, 567–570. doi: 10.1038/nature12375
Holden, M. T., Titball, R. W., Peacock, S. J., Cerdeno-Tarraga, A. M., Atkins, T., Crossman, L. C., et al. (2004). Genomic plasticity of the causative agent of melioidosis, Burkholderia pseudomallei. Proc. Natl. Acad. Sci. U. S. A. 101, 14240–14245. doi: 10.1073/pnas.0403302101
Kelso, B. H. L., Smith, R. V., and Laughlin, R. J. (1999). Effects of carbon substrates on nitrite accumulation in freshwater sediments. Appl. Environ. Microbiol. 65, 61–66. doi: 10.1128/AEM.65.1.61-66.1999
Kertesz, M. A., Kawasaki, A., and Stolz, A. (2017). “Aerobic hydrocarbon-degrading Alphaproteobacteria: Sphingomonadales” in Taxonomy, genomics and ecophysiology of hydrocarbon-degrading microbes. Vol. 2017. ed. T. J. McGenity (Cham: Springer International Publishing), 1–21.
Kraft, B., Tegetmeyer, H. E., Sharma, R., Klotz, M. G., Ferdelman, T. G., Hettich, R. L., et al. (2014). The environmental controls that govern the end product of bacterial nitrate respiration. Science 345, 676–679. doi: 10.1126/science.1254070
Kuypers, M. M. M., Marchant, H. K., and Kartal, B. (2018). The microbial nitrogen-cycling network. Nat. Rev. Microbiol. 16, 263–276. doi: 10.1038/nrmicro.2018.9
Limmathurotsakul, D., Golding, N., Dance, D. A., Messina, J. P., Pigott, D. M., Moyes, C. L., et al. (2016). Predicted global distribution of Burkholderia pseudomallei and burden of melioidosis. Nat. Microbiol. 1:15008. doi: 10.1038/nmicrobiol.2015.8
Lovley, D. R., Giovannoni, S. J., White, D. C., Champine, J. E., Phillips, E. J., Gorby, Y. A., et al. (1993). Geobacter metallireducens gen. nov. sp. nov., a microorganism capable of coupling the complete oxidation of organic compounds to the reduction of iron and other metals. Arch. Microbiol. 159, 336–344. doi: 10.1007/BF00290916
Lueders, T. (2015). “DNA‐ and RNA-based stable isotope probing of hydrocarbon degraders” in Hydrocarbon and lipid microbiology protocols. Springer protocols handbooks. eds. T. J. McGenity, K. N. Timmis, and B. Nogales (Berlin, Heidelberg: Springer).
Lueders, T. (2017). The ecology of anaerobic degraders of BTEX hydrocarbons in aquifers. FEMS Microbiol. Ecol. 93:fiw220. doi: 10.1093/femsec/fiw220
Park, Y. J., Kim, K. H., Han, D. M., Lee, D. H., and Jeon, C. O. (2019). Sphingobium terrigena sp. nov., isolated from gasoline-contaminated soil. Int. J. Syst. Evol. Microbiol. 69, 2459–2464. doi: 10.1099/ijsem.0.003514
Pilloni, G., Granitsiotis, M. S., Engel, M., and Lueders, T. (2012). Testing the limits of 454 Pyrotag sequencing: reproducibility, quantitative assessment and comparison to T-RFLP fingerprinting of aquifer microbes. PLoS One 7:e40467. doi: 10.1371/journal.pone.0040467
Ponsin, V., Coulomb, B., Guelorget, Y., Maier, J., and Hohener, P. (2014). In situ biostimulation of petroleum hydrocarbon degradation by nitrate and phosphate injection using a dipole well configuration. J. Contam. Hydrol. 171, 22–31. doi: 10.1016/j.jconhyd.2014.10.003
Rabus, R., Boll, M., Heider, J., Meckenstock, R. U., Buckel, W., Einsle, O., et al. (2016). Anaerobic microbial degradation of hydrocarbons: from enzymatic reactions to the environment. J. Mol. Microbiol. Biotechnol. 26, 5–28. doi: 10.1159/000443997
Raghoebarsing, A. A., Pol, A., van de Pas-Schoonen, K. T., Smolders, A. J., Ettwig, K. F., Rijpstra, W. I., et al. (2006). A microbial consortium couples anaerobic methane oxidation to denitrification. Nature 440, 918–921. doi: 10.1038/nature04617
Reddy, G. S., and Garcia-Pichel, F. (2007). Sphingomonas mucosissima sp. nov. and Sphingomonas desiccabilis sp. nov., from biological soil crusts in the Colorado Plateau, USA. Int. J. Syst. Evol. Microbiol. 57, 1028–1034. doi: 10.1099/ijs.0.64331-0
Revesz, F., Toth, E. M., Kriszt, B., Boka, K., Benedek, T., Sarkany, O., et al. (2018). Sphingobium aquiterrae sp. nov., a toluene, meta‐ and para-xylene-degrading bacterium isolated from petroleum hydrocarbon-contaminated groundwater. Int. J. Syst. Evol. Microbiol. 68, 2807–2812. doi: 10.1099/ijsem.0.002898
Rockne, K. J., Chee-Sanford, J. C., Sanford, R. A., Hedlund, B. P., Staley, J. T., and Strand, S. E. (2000). Anaerobic naphthalene degradation by microbial pure cultures under nitrate-reducing conditions. Appl. Environ. Microbiol. 66, 1595–1601. doi: 10.1128/AEM.66.4.1595-1601.2000
Tancsics, A., Farkas, M., Szoboszlay, S., Szabo, I., Kukolya, J., Vajna, B., et al. (2013). One-year monitoring of meta-cleavage dioxygenase gene expression and microbial community dynamics reveals the relevance of subfamily I.2.C extradiol dioxygenases in hypoxic, BTEX-contaminated groundwater. Syst. Appl. Microbiol. 36, 339–350. doi: 10.1016/j.syapm.2013.03.008
Tancsics, A., Szalay, A. R., Farkas, M., Benedek, T., Szoboszlay, S., Szabo, I., et al. (2018). Stable isotope probing of hypoxic toluene degradation at the Siklos aquifer reveals prominent role of Rhodocyclaceae. FEMS Microbiol. Ecol. 94:fiy088. doi: 10.1093/femsec/fiy088
Tancsics, A., Szoboszlay, S., Szabo, I., Farkas, M., Kovacs, B., Kukolya, J., et al. (2012). Quantification of subfamily I.2.C catechol 2,3-dioxygenase mRNA transcripts in groundwater samples of an oxygen-limited BTEX-contaminated site. Environ. Sci. Technol. 46, 232–240. doi: 10.1021/es201842h
Tang, Y. J., Carpenter, S., Deming, J., and Krieger-Brockett, B. (2005). Controlled release of nitrate and sulfate to enhance anaerobic bioremediation of phenanthrene in marine sediments. Environ. Sci. Technol. 39, 3368–3373. doi: 10.1021/es040427w
Ulrich, A. C., and Edwards, E. A. (2003). Physiological and molecular characterization of anaerobic benzene-degrading mixed cultures. Environ. Microbiol. 5, 92–102. doi: 10.1046/j.1462-2920.2003.00390.x
Vogt, C., Lueders, T., Richnow, H. H., Kruger, M., von Bergen, M., and Seifert, J. (2016). Stable isotope probing approaches to study anaerobic hydrocarbon degradation and degraders. J. Mol. Microbiol. Biotechnol. 26, 195–210. doi: 10.1159/000440806
Walters, W., Hyde, E. R., Berg-Lyons, D., Ackermann, G., Humphrey, G., Parada, A., et al. (2016). Improved bacterial 16S rRNA gene (V4 and V4-5) and fungal internal transcribed spacer marker gene primers for microbial community surveys. mSystems 1, e00009–e00015. doi: 10.1128/mSystems.00009-15
Weelink, S. A. B., van Eekert, M. H. A., and Stams, A. J. M. (2010). Degradation of BTEX by anaerobic bacteria: physiology and application. Rev. Environ. Sci. Biotechnol. 9, 359–385. doi: 10.1007/s11157-010-9219-2
Widdel, F., Knittel, K., and Galushko, A. (2010). “Anaerobic hydrocarbon-degrading microorganisms: an overview” in Handbook of hydrocarbon and lipid microbiology. ed. K. N. Timmis (Berlin, Heidelberg: Springer), 1997–2021.
Winderl, C., Anneser, B., Griebler, C., Meckenstock, R. U., and Lueders, T. (2008). Depth-resolved quantification of anaerobic toluene degraders and aquifer microbial community patterns in distinct redox zones of a tar oil contaminant plume. Appl. Environ. Microbiol. 74, 792–801. doi: 10.1128/AEM.01951-07
Winderl, C., Penning, H., von Netzer, F., Meckenstock, R. U., and Lueders, T. (2010). DNA-SIP identifies sulfate-reducing Clostridia as important toluene degraders in tar-oil-contaminated aquifer sediment. ISME J. 4, 1314–1325. doi: 10.1038/ismej.2010.54
Xu, M. Y., Zhang, Q., Xia, C. Y., Zhong, Y. M., Sun, G. P., Guo, J., et al. (2014). Elevated nitrate enriches microbial functional genes for potential bioremediation of complexly contaminated sediments. ISME J. 8, 1932–1944. doi: 10.1038/ismej.2014.42
Yang, S. C., Song, Y., Wang, D., Wei, W. X., Yang, Y., Men, B., et al. (2016). Application of nitrate to enhance biodegradation of gasoline components in soil by indigenous microorganisms under anoxic condition. Environ. Technol. 37, 1045–1053. doi: 10.1080/09593330.2015.1098731
Zhu, B., Bradford, L., Huang, S., Szalay, A., Leix, C., Weissbach, M., et al. (2017). Unexpected diversity and high abundance of putative nitric oxide dismutase (nod) genes in contaminated aquifers and wastewater treatment systems. Appl. Environ. Microbiol. 83, e02750–e02716. doi: 10.1128/AEM.02750-16
Zhu, B., Wang, J., Bradford, L. M., Ettwig, K., Hu, B., and Lueders, T. (2019). Nitric oxide dismutase (nod) genes as a functional marker for the diversity and phylogeny of methane-driven oxygenic denitrifiers. Front. Microbiol. 10:1577. doi: 10.3389/fmicb.2019.01577
Keywords: toluene degradation, bioremediation, stable isotope probing, oxygenic denitrification, Azoarcus
Citation: Zhu B, Friedrich S, Wang Z, Táncsics A and Lueders T (2020) Availability of Nitrite and Nitrate as Electron Acceptors Modulates Anaerobic Toluene-Degrading Communities in Aquifer Sediments. Front. Microbiol. 11:1867. doi: 10.3389/fmicb.2020.01867
Edited by:
Qiaoyun Huang, Huazhong Agricultural University, ChinaReviewed by:
Annika Vaksmaa, Royal Netherlands Institute for Sea Research (NIOZ), NetherlandsAng Li, Harbin Institute of Technology, China
Copyright © 2020 Zhu, Friedrich, Wang, Táncsics and Lueders. This is an open-access article distributed under the terms of the Creative Commons Attribution License (CC BY). The use, distribution or reproduction in other forums is permitted, provided the original author(s) and the copyright owner(s) are credited and that the original publication in this journal is cited, in accordance with accepted academic practice. No use, distribution or reproduction is permitted which does not comply with these terms.
*Correspondence: Baoli Zhu, YmFvbGkuemh1QHVuaS1iYXlyZXV0aC5kZQ==; YmFvbGl6aHVAZ21haWwuY29t Tillmann Lueders, dGlsbG1hbm4ubHVlZGVyc0B1bmktYmF5cmV1dGguZGU=
†Present address: Sebastian Friedrich, Chair of Hydrology and River Basin Management, Technical University of Munich, Munich, Germany