- 1CAS Key Laboratory of Experimental Marine Biology, CAS Center for Ocean Mega-Science, Institute of Oceanology, Chinese Academy of Sciences, Qingdao, China
- 2Laboratory for Marine Biology and Biotechnology, Pilot National Laboratory for Marine Science and Technology, Qingdao, China
- 3University of Chinese Academy of Sciences, Beijing, China
Translocation and assembly module (TAM) is a protein channel known to mediate the secretion of virulence factors during pathogen infection. Edwardsiella tarda is a Gram-negative bacterium that is pathogenic to a wide range of farmed fish and other hosts including humans. In this study, we examined the function of the two components of the TAM, TamA and TamB, of E. tarda (named tamAEt and tamBEt, respectively). TamAEt was found to localize on the surface of E. tarda and be recognizable by TamAEt antibody. Compared to the wild type, the tamA and tamB knockouts, TX01ΔtamA and TX01ΔtamB, respectively, were significantly reduced in motility, flagella formation, invasion into host cells, intracellular replication, dissemination in host tissues, and inducing host mortality. The lost virulence capacities of TX01ΔtamA and TX01ΔtamB were restored by complementation with the tamAEt and tamBEt genes, respectively. Furthermore, TX01ΔtamA and TX01ΔtamB were significantly impaired in the ability to survive under low pH and oxidizing conditions, and were unable to maintain their internal pH balance and cellular structures in acidic environments, which led to increased susceptibility to lysozyme destruction. Taken together, these results indicate that TamAEt and TamBEt are essential for the virulence of E. tarda and required for E. tarda to survive under stress conditions.
Introduction
Edwardsiella tarda is a Gram-negative bacterium of the family Enterobacteriaceae. It is an important pathogen that causes systemic infection in a wide variety of marine and freshwater fish as well as other hosts, including birds, reptiles, and mammals (Mohanty and Sahoo, 2007; Leung et al., 2012, 2019). In aquaculture, E. tarda-induced edwardsiellosis in many fish species has led to heavy economic losses in Asia, United States, and Europe (Castro et al., 2006; Nelson et al., 2009; Park et al., 2012). In humans, E. tarda has been reported to cause gastroinhastestinal and extraintestinal diseases in immunocompromised people (Abayneh et al., 2013; Shao et al., 2015).
Studies have indicated that E. tarda is an intracellular pathogen capable of invading and replicating in host phagocytes and non-phagocytes (Wang et al., 2013; Xie et al., 2014; Sui et al., 2017; Leung et al., 2019). E. tarda enters macrophages via both clathrin- and caveolin-mediated endocytosis (Sui et al., 2017). The type III (T3SS) and type VI (T6SS) secretion systems of E. tarda inject cytotoxic factors into host cells and facilitate invasion and intracellular replication of the pathogen (Zheng and Leung, 2007; Xie et al., 2010; Akeda et al., 2011). Other virulence factors, such as hemolysins, iron uptake and regulation systems, two-component systems, adhesins, invasins, and lysozyme inhibitors, are also involved in E. tarda infections (Wang et al., 2010; Chakraborty et al., 2011; Zheng et al., 2011; Li et al., 2012, 2015; Sun et al., 2012).
Bacterial virulence relies on membrane biogenesis pathways to assemble the outer membrane proteins (OMP) essential for the process of host-pathogen interactions, such as invasion and adhesion (Selkrig et al., 2015). In Gram-negative bacteria, the process of outer membrane assembly is dependent on the translocation and assembly module (TAM) and the β-barrel assembly machinery (BAM) complex (Selkrig et al., 2012; Gruss et al., 2013; Selkrig et al., 2014; Stubenrauch et al., 2016). TAM comprises two subunits: an integral OMP, TamA, and an inner membrane-anchored protein, TamB (Marani et al., 2006; Selkrig et al., 2012). Deletion of tamA or tamB reduces the virulence of Klebsiella pneumoniae, Proteus mirabilis, Citrobacter rodentium, Salmonella enterica, and Escherichia coli (Struve et al., 2003; Burall et al., 2004; Kelly et al., 2006; Selkrig et al., 2012). A recent study showed that the assembly of FimD, which is important for the deployment of fimbrial extensions from the surface of bacterial pathogens, and other usher proteins is mediated by the TAM complex (Stubenrauch et al., 2016).
In E. tarda, the tamA and tamB genes have been identified by genome sequencing; however, the role of TamA and TamB in E. tarda remains unknown. In the present work, we employed both in vitro and in vivo approaches to examine the function of E. tarda tamA and tamB (named tamAEt and tamBEt, respectively). Our results indicated that TamAEt and TamBEt were essential to the infectivity of E. tarda and to the survival of E. tarda under stress conditions, especially acidic conditions.
Materials and Methods
Ethics Statement
The experiments involving live animals in this study were approved by the Ethics Committee of Institute of Oceanology, Chinese Academy of Sciences. All methods were carried out in accordance with the relevant guidelines.
Fish
Clinically healthy tongue sole (Cynoglossus semilaevis) were purchased from a commercial fish farm in Shandong Province, China. Fish were maintained at 20°C in aerated seawater with 144% ± 4% dissolved oxygen (detected with a fast response DO sensor RINKO I; ARO-USB, JFE Advantech Co., Ltd., Japan; Air saturation range: 0–200%). The fish were fed with commercial feed purchased from Shandong Sheng-suo Fish Feed Research Center, Shandong, China. The content (%) of the feed was as follows: protein, ≥45; fat, ≥10; fiber, ≤4; calcium, ≥1.5; phosphate, ≥1.2; lysine, ≥2.2; ashes, ≤17. Before the experiment, the fish were verified to be clinically healthy by examining bacterial presence in spleen, kidney, and liver as reported previously (Zhou and Sun, 2015). Fish were euthanized by immersion in seawater containing 10 mg/L of tricaine methanesulfonate (Sigma, St. Louis, MO, United States) before tissue collection.
Bacterial Culture
Bacterial strains used in this study are listed in Table 1. E. tarda TX01, a pathogenic fish isolate, was cultured in Luria-Bertani broth (LB) at 28°C. The E. coli strains were cultured in LB medium at 37°C. Where indicated, polymyxin B, tetracycline, and chloramphenicol were supplemented at the concentrations of 50, 20, and 50 μg/ml, respectively.
Sequence Analysis
Sequence analysis was performed using the BLAST program at the National Center for Biotechnology Information (NCBI) and the Expert Protein Analysis System. Domain search was performed with the conserved domain search program of NCBI. Theoretical molecular mass and isoelectric point were predicted using EditSeq in the DNASTAR software package (Madison, WI, United States). Multiple sequence alignment was created with DNAMAN. Subcellular localization prediction was performed with the PSORTb v.3.0 server.
Plasmid Construction
To construct pETTamA, which expresses recombinant TamAEt (rTamAEt), tamAEt was amplified by PCR with primers TamA-F/TamA-R (Table 2). The PCR products were ligated with the T-A cloning vector T-Simple (TransGen Biotech., Beijing, China), and the recombinant plasmid was restriction digested with SmaI to retrieve the tamAEt-containing fragment, which was inserted into pET259 at the SwaI site, resulting in pETTamA. To construct pETTamB, which expresses the TamB domain of TamBEt (amino acid residues 780–1255) with a 6-histidine His-tag at the C-terminus, PCR was conducted with primers TamB-F/TamB-R (Table 2), and the PCR products were inserted into pET259 as above. To construct the low copy-number plasmids pJTTamA and pJTTamB that express tamAEt and tamBEt, respectively, tamAEt and tamBEt were amplified by PCR with primers TamA-F3/TamA-R and TamB-F3/TamB-R (Table 2), respectively; the PCR products were ligated with the TA cloning vector T-Simple, and the recombinant plasmids were digested with SmaI. The fragments containing tamAEt and tamBEt were retrieved and inserted into plasmid pBT3 at the EcoRV site, resulting in pBT3TamA and pBT3TamB, respectively. pBT3TamA and pBT3TamB were digested with SwaI, and the fragments carrying tamAEt and tamBEt were inserted into plasmid pJT at the SwaI site, resulting in pJTTamA and pJTTamB, respectively. All PCR products were verified by sequence analysis.
Construction of tamAEt and tamBEt Knockouts
To construct the mutant E. tarda with tamAEt knockout, i.e., TX01ΔtamA, in-frame deletion of a 1671 bp segment of tamAEt (residues 22–578) was performed by overlap extension PCR as follows: the first overlap PCR was performed with primers TamA-F1/TamA-R1, the second overlap PCR was performed with primers TamA-F2/TamA-R2, and the fusion PCR was performed with the primer pair TamA-F1/TamA-R2 (Table 2). The PCR products were ligated into the suicide plasmid pDM4 (Milton et al., 1996) at the BglII site, resulting in pDMTamA. S17-1 λpir was transformed with pDMTamA, and the transformants were mated with E. tarda TX01 via conjugation as previously described (Li et al., 2015). Briefly, the donor strain (resistant to chloramphenicol, sensitive to polymyxin B) and the recipient strain (resistant to polymyxin B, sensitive to chloramphenicol) were cultured in LB medium to OD600 of 0.8 and mixed at a ratio of 3:1. The mixture was spread onto a LB agar plate without antibiotics, and the plate was incubated at 28°C for 24 h. After incubation, the bacteria on the plate were resuspended in 2 ml LB, from which 100 μl was taken and plated on a LB agar plate supplemented with polymixin B and chloramphenicol to select for transconjugants. Transconjugants were cured of pDMTamA by incubation on LB agar plates supplemented with 10% sucrose (which induces sacB-mediated plasmid curing), and chloramphenicol-sensitive strains were subsequently analyzed by DNA sequencing to confirm in-frame deletion of tamAEt. This strain was named TX01ΔtamA. To construct the mutant E. tarda with tamBEt knockout, i.e., TX01ΔtamB, in-frame deletion of a 987 bp segment of tamB (corresponding to amino acid residues 919–1247) was performed by overlap extension PCR as follows: the first overlap PCR was performed with primers TamB-F1/TamB-R1, the second overlap PCR was performed with primers TamB-F2/TamB-R2, and the fusion PCR was performed with the primer pair TamB-F1/TamB-R2 (Table 2). S17-1 λpir was transformed with pDMTamB, and the transconjugants were selected as described above. One of the transconjugants was named TX01ΔtamB. To construct the tamAEt complement strain TX01ΔtamA/tamA, S17-1 λpir was transformed with pJTTamA, and the transformants were conjugated with TX01ΔtamA. The transconjugants were selected on LB agar plates supplemented with tetracycline (marker of pJT) and polymyxin B (marker of TX01 and its derivatives). One of the transformants was named TX01ΔtamA/tamA. To construct the tamBEt complement strain TX01ΔtamB/tamB, S17-1 λpir was transformed with pJTTamB, and the transformants were conjugated with TX01ΔtamB. The transconjugants were selected as described above. One of the transconjugants was named TX01ΔtamB/tamB.
Purification of Recombinant Proteins and Preparation of Antibodies
Escherichia coli BL21 (DE3) was transformed with pETTamA, pETTamB, or pET32a (which expresses the Trx tag). The transformants were cultured in LB medium at 37°C to mid-log phase, and the expression of rTamAEt, rTamBEt, and rTrx was induced by adding isopropyl-β-D-thiogalactopyranoside to a final concentration of 1 mM. After growth at 16°C for an additional 16 h, the cells were harvested by centrifugation, and recombinant proteins were purified using nickel-nitrilotriacetic acid columns (GE Healthcare, Piscataway, United States) as recommended by the manufacturer. The proteins were treated with Triton X-114 to remove endotoxin as reported previously (Zhang and Sun, 2015). The proteins were dialyzed for 24 h against phosphate buffered saline (PBS) and concentrated using PEG20000 (Solarbio, Beijing, China). The concentrations of the purified proteins were determined using the NanoPhotometer (Implen GmbH, Munich, Germany). Mouse antibodies against rTamAEt, rTamBEt, and rTrx were prepared as described previously (Li et al., 2016). The antibodies were purified using rProtein G Beads (Solarbio, Beijing, China). The specificity and titer of the antibodies were determined by Western blot and enzyme-linked immunosorbent assay (ELISA) as reported previously (Li et al., 2017).
Fluorescent Microscopy
Detecting of TamAEt on bacterial surface by fluorescence microscopy was performed as reported previously (Li et al., 2016). Briefly, E. tarda TX01 was cultured in LB medium to OD600 of 0.8 and resuspended in PBS (pH 7) to 108 CFU/ml. The bacterial suspension was dropped on a glass slide and incubated for 12 h at 28°C. The antibody against rTamAEt, rTamBEt, or rTrx was added to bacterial suspension. The cells were incubated at 37°C for 1 h and then washed three times with PBS (pH 7). Fluorescein isothiocyanate (FITC)-labeled goat anti-mouse IgG (Abcam, Cambridge, United Kingdom) was added to the bacteria, followed by incubation at 37°C for 1 h in the dark. After staining with 4, 6-diamino-2-phenyl indole (DAPI) (Invitrogen, Carlsbad, CA, United States), bacteria were visualized using a confocal microscope (Carl Zeiss, Oberkochen, Germany). To determine bacterial damage under acidic conditions, E. tarda TX01, TX01ΔtamA, TX01ΔtamB, TX01ΔtamA/tamA, and TX01ΔtamB/tamB were cultured as above and resuspended in PBS of pH 5 to 108 CFU/ml. The cells were incubated at 28°C for 2 h. After incubation, bacterial cells were treated with propidium iodide (PI) (Majorbio Biotech, Shanghai, China) and DAPI for 15 min in the dark according to the manufacturer’s instructions. The cells were then subjected to microscopy as above.
Western Blot
Edwardsiella tarda TX01, TX01ΔtamA, TX01ΔtamB, TX01ΔtamA/tamA, and TX01ΔtamB/tamB were cultured in LB medium to an OD600 of 0.8. Whole-cell proteins were prepared and subjected to Western blot as reported previously (Zhang M. et al., 2008) with mouse antibody against rTamBEt. RNA polymerase beta was used as an internal reference (Yang et al., 2019) and detected with anti-RNA polymerase beta antibody (Abcam, Cambridge, United Kingdom).
Electron Microscopy
To examine the flagella of E. tarda, E. tarda TX01, TX01ΔtamA, TX01ΔtamB, TX01ΔtamA/tamA, and TX01ΔtamB/tamB were statically cultured in LB medium at 28°C for 48 h and gently resuspended in PBS. Transmission electron microscope (TEM) examination was performed as previously reported (Givaudan and Lanois, 2000). Briefly, a drop of bacterial suspension was added to carbon-coated copper grids (200 mesh) and rinsed with ultrapure grade water, the bacteria were then negatively stained with 1% (wt/vol) phosphotungstic acid (5 s) and rinsed with ultrapure grade water. The grids were air dried and examined with a TEM (HT7700, Hitachi, Japan). To examine the structure of E. tarda under acidic conditions, E. tarda TX01, TX01ΔtamA, TX01ΔtamB, TX01ΔtamA/tamA, and TX01ΔtamB/tamB were cultured with shaking in LB medium to OD600 0.8, and resuspended in PBS buffer of pH 7 or pH 5 to 108 CFU/ml. The cells were incubated in PBS buffer of different pH at 28°C for 2 h. After incubation, the cells were observed with a TEM as above.
Motility Assay
Edwardsiella tarda TX01, TX01ΔtamA, TX01ΔtamB, TX01ΔtamA/tamA, and TX01ΔtamB/tamB were cultured with shaking in LB medium to OD600 0.8, and 10 μl cell suspension were spotted onto the center of fresh LB plates containing 0.3 or 0.5% (w/v) agar. The plates were incubated at 28°C for 2 days, and the motility of the bacteria was assessed by examining the diameter of the bacterial halo on the plate.
Bacterial Survival Under Acidic and Oxidizing Conditions
PBS buffer was adjusted to pH 7, pH 5, or pH 4.5 with hydrochloric acid. E. tarda TX01, TX01ΔtamA, TX01ΔtamB, TX01ΔtamA/tamA, and TX01ΔtamB/tamB were cultured as above and resuspended in PBS buffers of different pH to 105 CFU/ml. The cells were incubated at 28°C for 2 h. After incubation, the cells were diluted in PBS (pH 7) and plated on LB agar plates. The plates were incubated at 28°C for 24 h, and the colonies emerged on the plates were counted. The survival rate was calculated as follows: (number of survived cells in different pH/number of survived cells in pH 7) × 100%. To examine the effect of the acidic condition on the internal pH of E. tarda, above bacteria were suspended in PBS (pH 5) to 1010 CFU/ml and incubated at 28°C for 2 h. The cells were then pelleted by centrifugation and resuspended in 1 ml PBS (pH 7). The cells were boiled for 5 min at 100°C and subjected to sonication in an ice-water bath. The pH of the cell lysate was measured using a pH meter (Sartorius, Beijing, China). To examine the survival of E. tarda against lysozyme under acidic condition, TX01, TX01ΔtamA, TX01ΔtamB, TX01ΔtamA/tamA and TX01ΔtamB/tamB were suspended in PBS of different pH to 105 CFU/ml and incubated for 2 h. After incubation, the cells were treated with 100 μg/ml hen egg white lysozyme (HEWL) at 28°C for 1 h. The survival rate was calculated as follows: (number of survived cells after lysozyme treatment under different pH/number of survived cells without lysozyme treatment under the corresponding pH) × 100%. To examine the survival of E. tarda under oxidizing conditions, above bacteria (105 CFU/ml) were incubated in PBS (pH 7) containing 1 mM, 2 mM, or 3 mM H2O2 at 28°C for 2 h. The survival rate was calculated as follows: (number of survived cells after H2O2 treatment/number of cells surviving without H2O2 treatment) × 100%.
In vitro Infection
Bacterial Infection of Peripheral Blood Leukocytes (PBL)
Blood was collected from the caudal vein of tongue sole. PBL were isolated from the blood with 61% Percoll and collected as described previously (Li et al., 2017). The cells were cultured in L-15 medium (Thermo Scientific HyClone, Beijing, China) in 96-well culture plates (105 cells/well). E. tarda TX01, TX01ΔtamA, TX01ΔtamB, TX01ΔtamA/tamA, and TX01ΔtamB/tamB were prepared as above and added to PBL (105 CFU/well). The cells were incubated at 28°C for 0.5, 1, or 2 h. After incubation, the plates were washed with PBS (pH 7), and the cells were lysed with 100 μl PBS containing 1% Triton X-100. The cell lysate was diluted and plated in triplicate on LB agar plates. The plates were incubated at 28°C for 48 h, and the colonies that emerged on the plates were counted. The genetic identities of the colonies were verified by PCR with specific primers and sequence analysis of the PCR products. The experiment was performed three times.
Intracellular
Edwardsiella tarda TX01, TX01ΔtamA, TX01ΔtamB, TX01 ΔtamA/tamA, and TX01ΔtamB/tamB were prepared as above and added to tongue sole PBL (105 CFU/well). The cells were incubated at 28°C for 1 h and washed three times with PBS (pH 7). Fresh L-15 medium containing 100 μg/ml gentamicin (Solarbio, Beijing, China) was added to the cells, and the cells were incubated at 28°C for 1 h to kill extracellular bacteria. The plates were washed three times with PBS (pH 7) and incubated at 28°C for 0, 1, 2, 4, and 8 h. After incubation, the cells were lysed, and bacterial recovery was determined as above. The experiment was performed three times.
In vivo Infection
In vivo infection was performed as reported previously (Li et al., 2015). Briefly, E. tarda TX01, TX01ΔtamA, TX01ΔtamB, TX01ΔtamA/tamA, and TX01ΔtamB/tamB were cultured as above. The cells were washed with PBS (pH 7) and resuspended in PBS (pH 7) to 5 × 106CFU/ml. Tongue sole (average 15.7 g) were randomly divided into five groups (15 fish/group) and infected via intramuscular injection with 100 μl TX01, TX01ΔtamA, TX01ΔtamB, TX01ΔtamA/tamA or TX01ΔtamB/tamB. At 12, 24, and 48 h post-infection, kidney, spleen, and blood were collected from the fish (five at each time point). The tissues were homogenized in PBS (pH 7). The homogenates was serially diluted and plated in triplicate on LB agar plates. The plates were incubated at 28°C for 48 h, and the colonies that appeared on the plates were enumerated. The genetic identity of the colonies was verified as above. For mortality analysis, five groups (20 fish/group) of tongue sole were infected as above with TX01, TX01ΔtamA, TX01ΔtamB, TX01ΔtamA/tamA, or TX01ΔtamB/tamB, and the fish were monitored daily for mortality for 15 days.
Statistical Analysis
All experiments were performed three times. Statistical analyses were carried out with SPSS 17.0 software (SPSS Inc., Chicago, IL, United States). Data were analyzed with analysis of variance (ANOVA), and statistical significance was defined as P < 0.05.
Results
Characterization of the Sequences of TamAEt and TamBEt
A search of the genome of E. tarda revealed the presence of tamA and tamB homologs (named tamAEt and tamBEt, respectively). TamAEt is composed of 578 amino acid residues, with a predicted molecular mass of 64.27 kDa and a predicted pI of 9.1. TamBEt is composed of 1255 amino acid residues, with a predicted molecular mass of 134.99 kDa and a predicted pI of 9.3. TamAEt possesses an outer membrane translocation and assembly module A domain; TamBEt possesses an outer membrane translocation and assembly module B domain (Supplementary Figure S1). TamAEt has 67.1–97.8% overall sequence identities with other bacterial OMP assembly factors, but only 12.8% sequence identity with E. tarda BamA. TamBEt has 59.8–96.4% overall sequence identities with other bacterial TamB (Supplementary Figure S2).
Localization of TamAEt Onto Bacterial Surface
Immunofluorescence microscopy showed that when E. tarda was treated with anti-rTamAEt antibody, the antibody was detected on the cells, whereas no cell-associated antibody was detected in E. tarda treated with anti-rTamBEt antibody or anti-rTrx antibody (Figure 1), suggesting that TamAEt is surface-exposed in E. tarda.
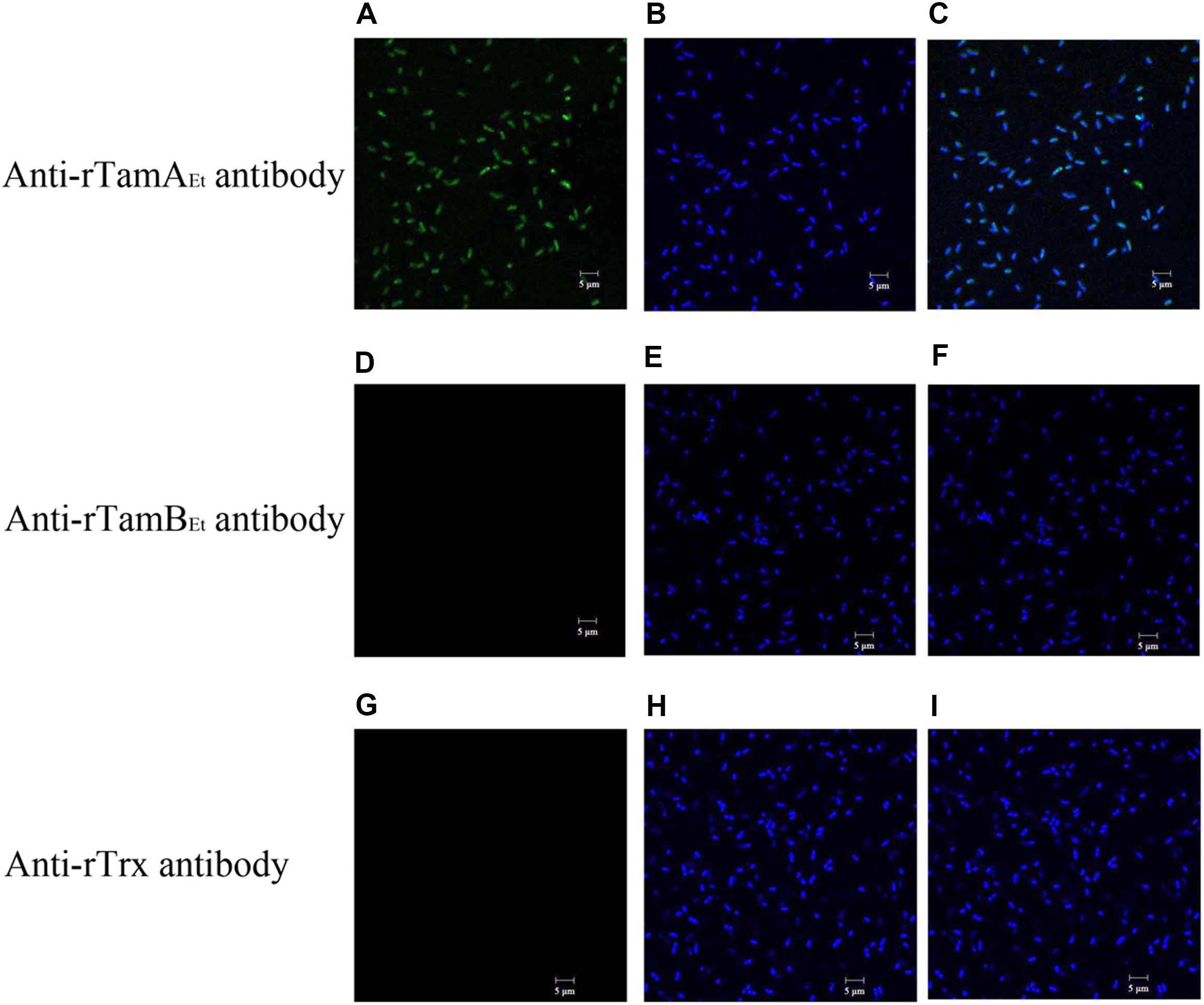
Figure 1. Detection of TamAEt on bacterial surface. Edwardsiella tarda TX01 was incubated with anti-rTamAEt antibody (A,B), anti-rTamBEt antibody (D,E), or anti-rTrx antibody (G,H) and then treated with FITC-labeled secondary antibody and stained with DAPI. The cells were subjected to microscopy with green (detecting FITC label; A,D,G) or blue (detecting DAPI stain; B,E,H) fluorescence light. (C) A merged image of (A,B); (F) a merged image of (D,E); (I) a merged image of (G,H).
Growth and Survival of tamAEt and tamBE Mutants Under Different Conditions
Growth and Motility
Two isogenetic mutants of E. tarda TX01, i.e., TX01ΔtamA and TX01ΔtamB, were constructed, which bear markerless deletions of tamAEt and tamBEt, respectively. The deletion of tamB in TX01ΔtamB was verified by Western blot, which detected no TamB production in TX01ΔtamB (Supplementary Figure S3). Growth analysis showed that when cultured in LB medium, TX01ΔtamA and TX01ΔtamB, displayed growth profiles similar to that of the parental strain TX01 (data not shown), which is consistent with previous observation in other bacterial species (Struve et al., 2003; Burall et al., 2004; Kelly et al., 2006; Selkrig et al., 2012). The swimming and swarming abilities of TX01ΔtamA and TX01ΔtamB were severely impaired in comparison to that of the wild type (Figure 2A and Table 3). In contrast, the swimming and swarming abilities of TX01ΔtamA/tamA and TX01ΔtamB/tamB, which have the tamAEt and tamBEt genes, respectively, introduced back to the mutant bacteria, were largely similar to that of the wild type (Figure 2A and Table 3). Electron microscopy showed that TX01, TX01ΔtamA/tamA, and TX01ΔtamB/tamB, but not TX01ΔtamA or TX01ΔtamB, possessed polar flagella (Figure 2B).
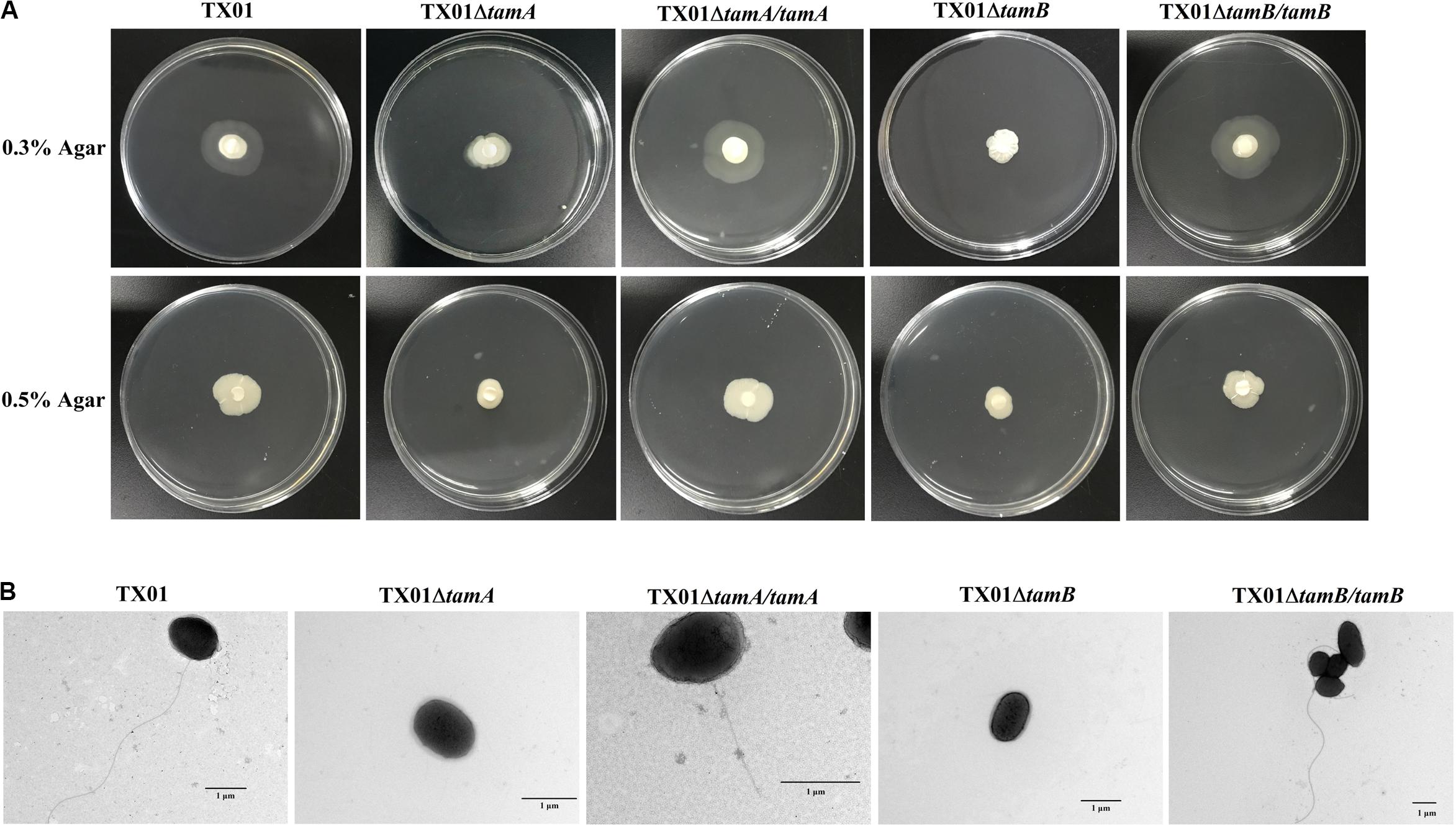
Figure 2. The motility and flagella of Edwardsiella tarda TX01 variants. (A) TX01, TX01ΔtamA, TX01ΔtamB, TX01ΔtamA/tamA, and TX01ΔtamB/tamB suspensions were spotted onto the center of LB plates containing 0.3 or 0.5% (w/v) agar. The plates were incubated at 28°C for 48 h before observation. (B) TX01, TX01ΔtamA, TX01ΔtamB, TX01ΔtamA/tamA, and TX01ΔtamB/tamB were cultured in LB medium and then examined with a transmission electron microscope.
Survival Under Acidic and Oxidizing Conditions
When incubated in PBS buffer of pH 7, the survival rates of TX01ΔtamA and TX01ΔtamB were comparable to that of the wild type TX01. However, at pH 5 and pH 4.5, the survival rates of TX01ΔtamA and TX01ΔtamB were significantly lower than that of TX01 (Figure 3A). In contrast, the survival rates of TX01ΔtamA/tamA and TX01ΔtamB/tamB were largely similar to that of the wild type (Figure 3A). Furthermore, following incubation in the pH 5 buffer, the cell lysates of TX01ΔtamA and TX01ΔtamB exhibited pH values of 6.25 and 6.27, respectively, which were significantly lower than that of the cell lysates of the wild type (pH 7.2), TX01ΔtamA/tamA (pH 7.1) and TX01ΔtamB/tamB (pH 7.05) (Figure 3B). Pre-incubation in the pH 6 or pH 5 buffer, but not in the pH 7 buffer, significantly reduced the survival rates of TX01ΔtamA and TX01ΔtamB against lysozyme treatment (Figure 3C). In contrast, pre-incubation in low pH buffers had no significant effects on the survival of TX01ΔtamA/tamA or TX01ΔtamB/tamB against lysozyme treatment (Figure 3C). Similarly, the survival rates of TX01ΔtamA and TX01ΔtamB, but not TX01ΔtamA/tamA and TX01ΔtamB/tamB, in 1 mM, 2 mM, and 3 mM H2O2 were significantly reduced compared to that of the wild type (Figure 3D).
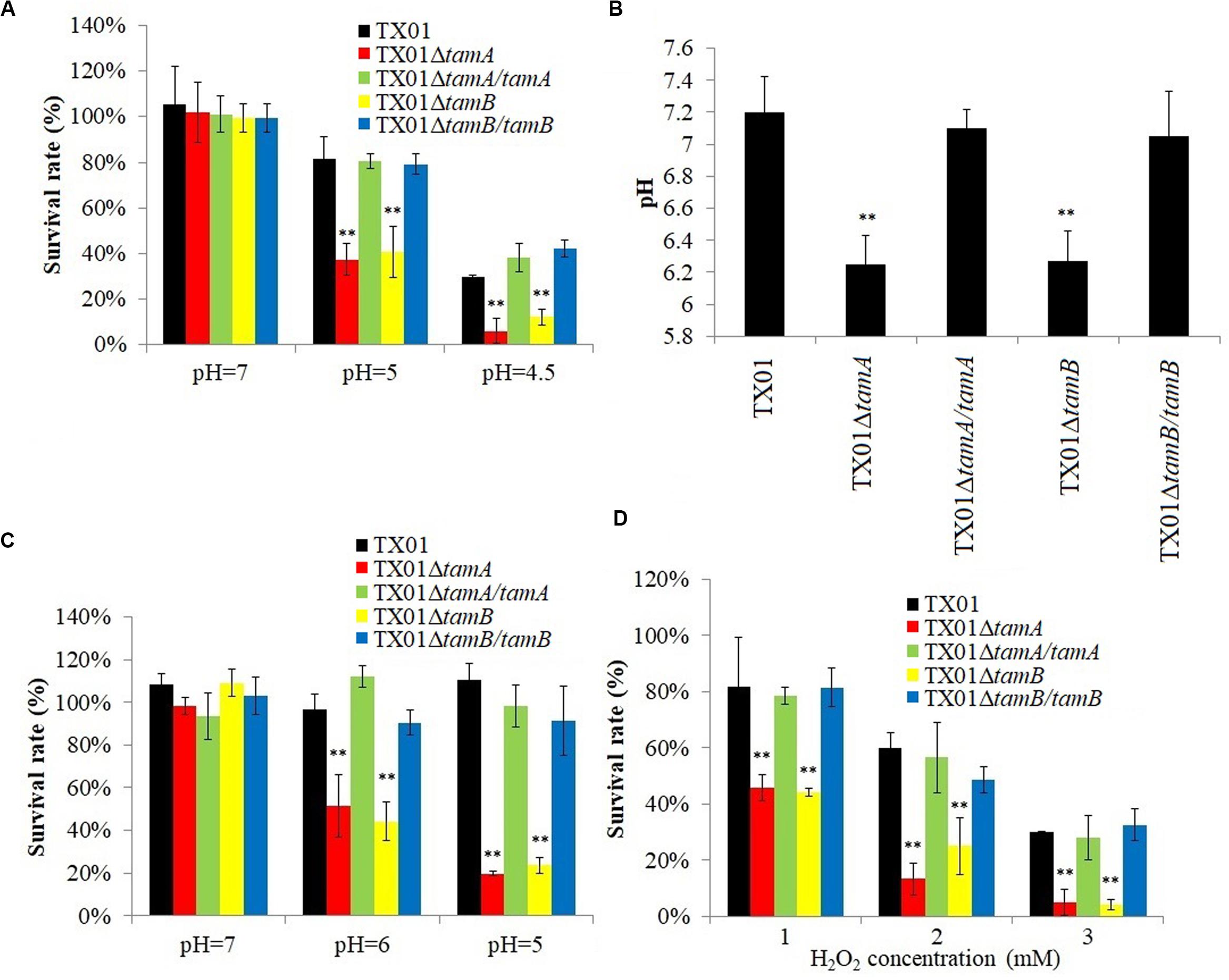
Figure 3. Effects of pH and H2O2 on the survival of Edwardsiella tarda variants. (A) TX01, TX01ΔtamA, TX01ΔtamB, TX01ΔtamA/tamA, and TX01ΔtamB/tamB were incubated in PBS of different pH for 2 h, and bacterial survival was determined. (B) TX01, TX01ΔtamA, TX01ΔtamB, TX01ΔtamA/tamA, and TX01ΔtamB/tamB were incubated in PBS of pH 5 for 2 h; after incubation, the pH of the cell lysate was determined. (C) TX01, TX01ΔtamA, TX01ΔtamB, TX01ΔtamA/tamA, and TX01ΔtamB/tamB were incubated in PBS of different pH for 2 h; after incubation, the cells were treated with lysozyme, and bacterial survival was determined. (D) TX01, TX01ΔtamA, TX01ΔtamB, TX01ΔtamA/tamA, and TX01ΔtamB/tamB were incubated with different concentrations of H2O2 for 1 h, and bacterial survival was determined. Data are the means of three independent assays and presented as means ± SEM. **P < 0.01.
Membrane Integrity of TX01ΔtamA and TX01ΔtamB Under Acidic Condition
Fluorescence microscopy showed that when incubated in PBS buffer of pH 5, TX01ΔtamA and TX01ΔtamB, but not TX01, TX01ΔtamA/tamA, or TX01ΔtamB/tamB, were markedly labeled by PI (Figure 4A), which can only penetrate into dead or damaged cells. TEM showed that compared to pH 7, pH 5 induced severe damages to the cellular structures of TX01ΔtamA and TX01ΔtamB, with the bacteria cells exhibiting swelling and formation of bubble-like protrusion structures (Figure 4B). In contrast, no apparent change in the cellular structures of the wild type or the complemented strains was observed (Figure 4B).
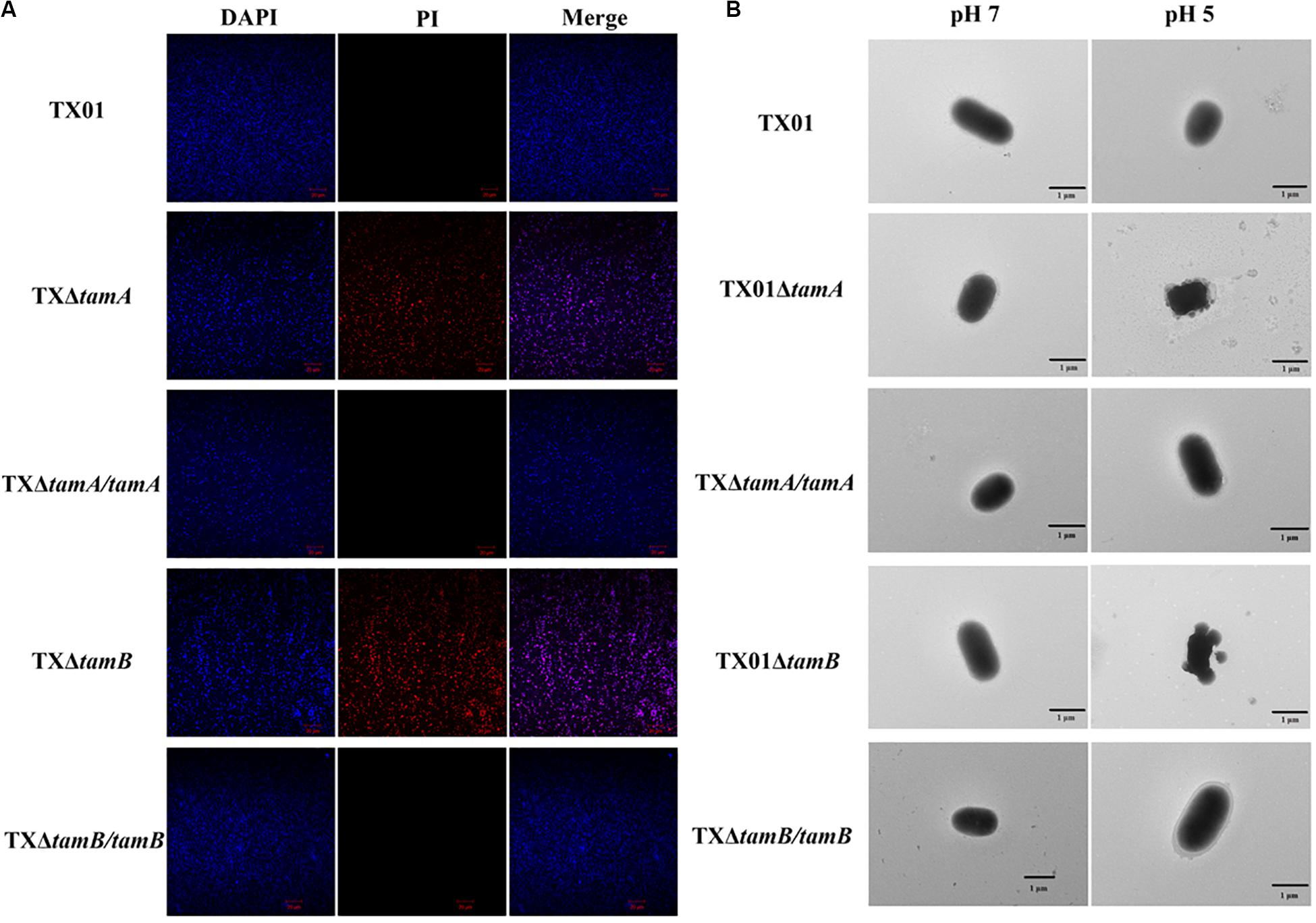
Figure 4. The structures of Edwardsiella tarda variants under acidic condition. (A) TX01, TX01ΔtamA, TX01ΔtamB, TX01ΔtamA/tamA, and TX01ΔtamB/tamB were incubated at pH 5 for 2 h and stained with DAPI and PI. The cells were subjected to microscopy with red or blue fluorescence light. (B) TX01, TX01ΔtamA, TX01ΔtamB, TX01ΔtamA/tamA, and TX01ΔtamB/tamB were incubated at pH 5 or pH 7 for 2 h and examined with a transmission electron microscope.
In vitro Infectivity of TX01ΔtamA and TX01ΔtamB
In vitro study showed that when tongue sole PBLs were infected with E. tarda mutants or wild type for 0.5, 1, and 2 h, the numbers of TX01ΔtamA and TX01ΔtamB recovered from the cells were significantly lower than that of the wild type TX01, whereas the numbers of recovered TX01ΔtamA/tamA and TX01ΔtamB/tamB were comparable to that of the wild type (Figure 5A). When the extracellular bacteria were removed by killing, the intracellular TX01, TX01ΔtamA/tamA, and TX01ΔtamB/tamB were found to continue to replicate and increase in number, whereas the intracellular TX01ΔtamA and TX01ΔtamB exhibited no detectable increase in number (Figure 5B).
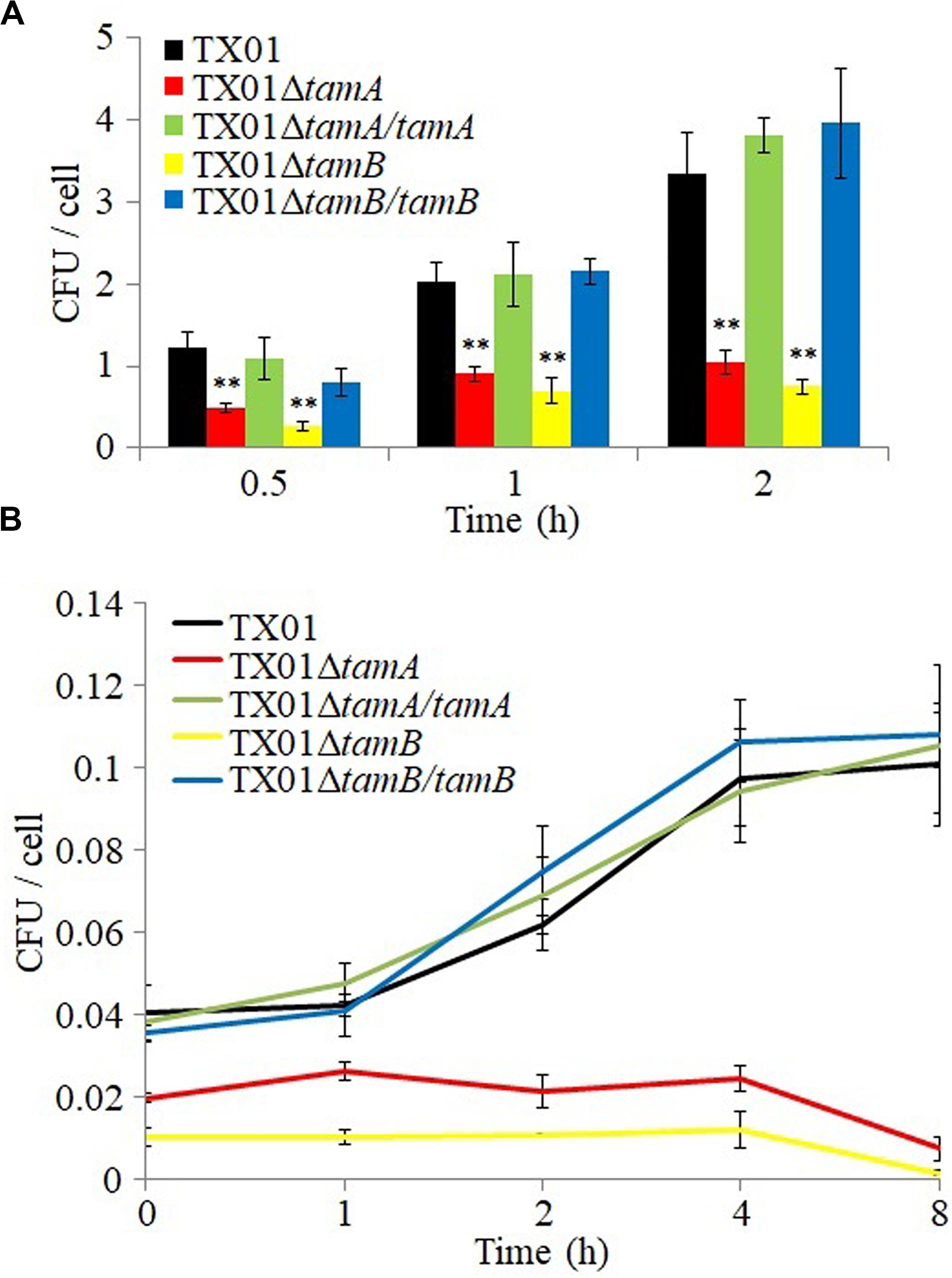
Figure 5. Cellular infectivity of Edwardsiella tarda variants. (A) Tongue sole peripheral blood leukocytes (PBL) were infected with TX01, TX01ΔtamA, TX01ΔtamB, TX01ΔtamA/tamA, or TX01ΔtamB/tamB for 0.5, 1, and 2 h, attached bacterial number was determined. (B) PBLs were infected as above for 1 h; the extracellular bacteria were killed with antibiotic, and the intracellular bacterial number was determined at different time points. Data are the means of three independent assays and presented as means ± SEM. **P < 0.01.
In vivo Infectivity and Lethality of TX01ΔtamA and TX01ΔtamB
In vivo study showed that when inoculated into tongue sole, TX01ΔtamA and TX01ΔtamB exhibited dramatically reduced bacterial disseminations in kidney, spleen, and blood in comparison to the wild type TX01, whereas the tissue dissemination capacities of TX01ΔtamA/tamA and TX01ΔtamB/tamB were similar to that of the wild type (Figure 6). Consistently, fish mortalities induced by TX01ΔtamA and TX01ΔtamB were significantly lower than that induced by TX01, TX01ΔtamA/tamA, or TX01ΔtamB/tamB (Figure 7).
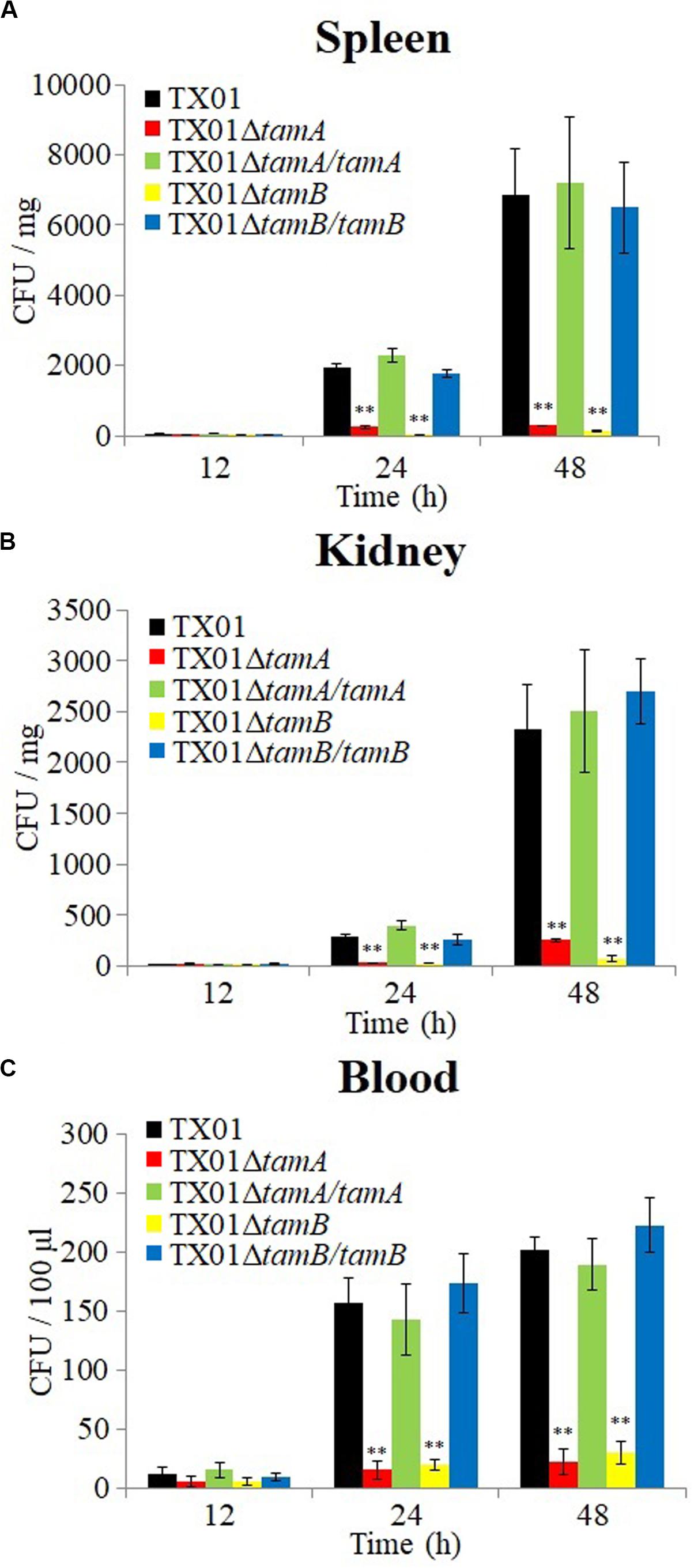
Figure 6. The tissue dissemination capacities of Edwardsiella tarda variants. Tongue sole were infected with TX01, TX01ΔtamA, TX01ΔtamB, TX01ΔtamA/tamA, or TX01ΔtamB/tamB for different hours, and bacterial recoveries from spleen (A), kidney (B), and blood (C) were determined. Data are the means of three independent assays and presented as means ± SEM. **P < 0.01.
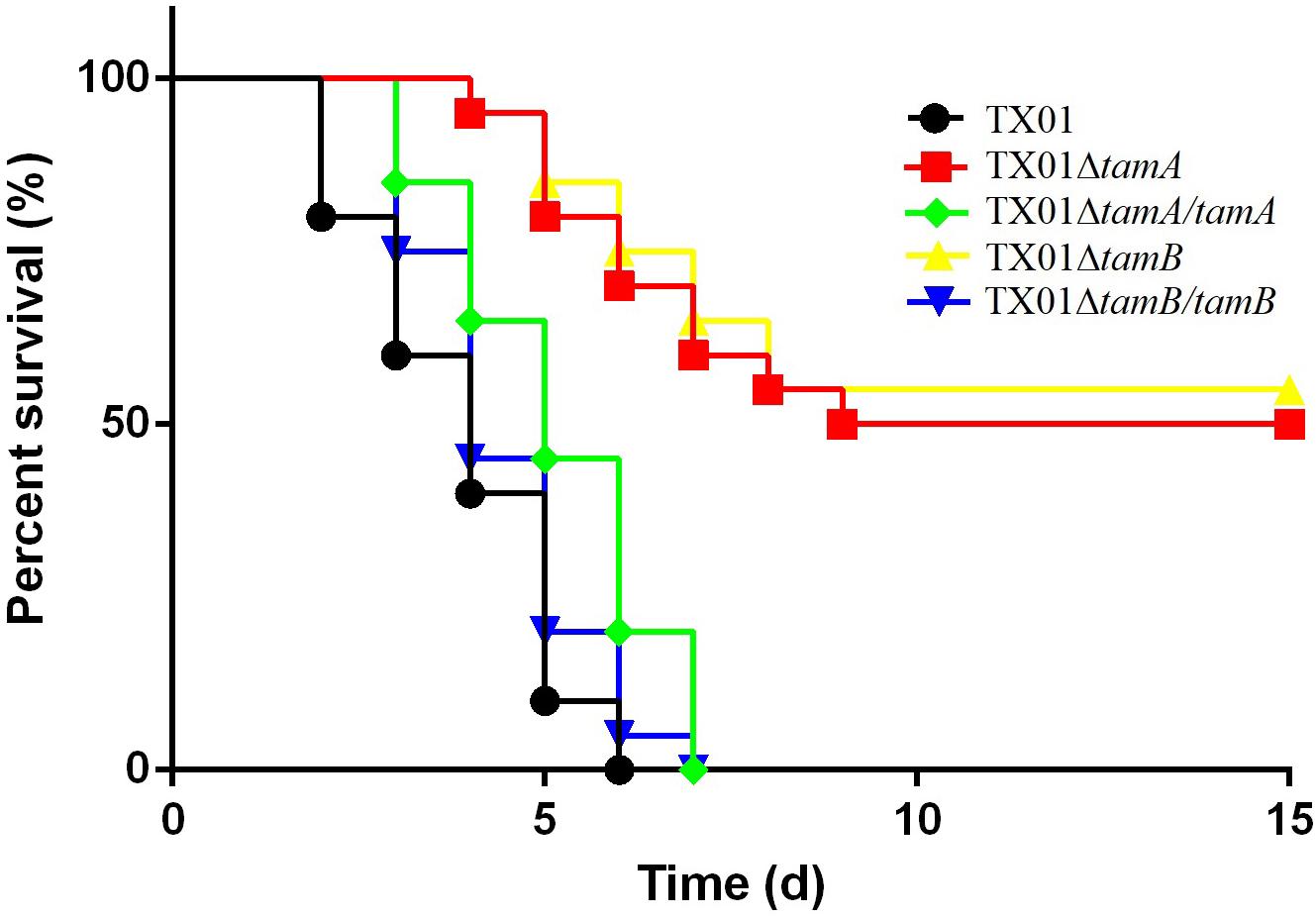
Figure 7. Survivals of tongue sole infected with Edwardsiella tarda variants. Tongue sole were infected with TX01, TX01ΔtamA, TX01ΔtamB, TX01ΔtamA/tamA, or TX01ΔtamB/tamB. The mortality and survival of the fish were monitored daily for 15 days. The experiment was performed three times, and the mean survival rates are shown.
Discussion
In Gram-negative bacteria, the process of outer membrane assembly is dependent on the Omp85-family protein β-barrel assembly machinery (Hagan et al., 2011), and some membrane structures require a distinct subgroup of the Omp85 family protein, TamA (Selkrig et al., 2012; Gruss et al., 2013; Stubenrauch et al., 2016). TamA and BamA have similar domain structures, and both are Omp85-family proteins that function in parallel pathways for OMP assembly in bacteria (Selkrig et al., 2015). It has been postulated that in the evolution of some bacteria, duplicate copies of BamA form the origin of TamA (Heinz et al., 2015). In our study, we found that TamAEt and TamBEt possess conserved domains of TamA and TamB, respectively. TamAEt has high levels of sequence identities with other bacterial OMP assembly factors but a low identity with the BamA of E. tarda, suggesting that the BAM and TAM complexes may have evolved to have different functions in E. tarda.
Gram-negative bacteria are separated from the external environment by the inner and outer membranes, which are set apart by the periplasm and the peptidoglycan layer (Albenne and Ieva, 2017). In Proteobacteria, TAM is composed of two membrane proteins, TamA and TamB. TamA is integrated in the outer membrane, while TamB has a signal-anchor sequence embedded in the inner membrane, and the interaction of these two subunits depends on TamB penetrating through the peptidoglycan layer (Selkrig et al., 2012; Shen et al., 2014). Consistently, we found that TamAEt, but not TamBEt, was exposed on the surface of E. tarda, suggesting that, like the TAM complex of other bacterial species (Selkrig et al., 2012), TamAEt probably associates with the inner membrane protein TamBEt and functions as a complex with the latter.
In uropathogenic E. coli, TAM catalyzes the assembly of the OMP FimD, which is essential for the ordered assembly of type 1 fimbriae (Munera et al., 2007; Stubenrauch et al., 2016). In Proteus mirabilis, transposon mutants of TamA and TamB show no defect in swarming activity (Burall et al., 2004). In our study, TX01ΔtamA and TX01ΔtamB exhibited no apparent flagella, implying a requirement of TamAEt and TamBEt in the biosynthesis/transport of E. tarda flagella. Consistently, TX01ΔtamA and TX01ΔtamB displayed very little swimming and swarming capacities. These observations indicated that tamAEt and tamBEt mutations affected flagella formation, resulting in impaired motility.
pH is known to regulate the expression of membrane proteins, such as porins, that are required for bacterial survival under stress conditions associated with low pH, oxidation, osmotic pressure, and high temperature (Begic and Worobec, 2006; Klimentova et al., 2019). Low pH has been shown to induce conformational changes in the extracellular loop reign of OmpG and influence the transmembrane pore formation (Korkmaz-Ozkan et al., 2010). At pH 5, OmpF increased fluoroquinolone antibiotic permeability and accumulation, resulting in increased antibiotic sensitivity (Cama et al., 2015). In Mycobacterium bovis, the transcription of the ompA gene was increased at pH 5.5 (Singh and Verma, 2008). In many pathogenic Gram-negative bacteria, the type III secretion system, which consists of the inner and OMP s and a needle, is also regulated by low pH (Markham et al., 2008). In our study, the survivals of TX01ΔtamA and TX01ΔtamB were significantly decreased under acidic and oxidative conditions, and the internal pH of these mutants were significantly affected by environmental pH, implying that the mutants were unable to maintain pH homeostasis inside the bacteria. Furthermore, low pH rendered TX01ΔtamA and TX01ΔtamB susceptible to lysozyme damage. Consistently, low pH severely damaged the cellular structures of TX01ΔtamA and TX01ΔtamB, which likely accounted for the observed lysozyme sensitivity under acidic conditions. These results suggested that TamAEt and TamBEt likely play an important role in the regulation of the proteins that form the normal membrane structure and determine the membrane integrity and permeability of E. tarda. It is also likely that TamAEt and TamBEt, by forming a complex, are directly involved in the formation of membrane integrity by constituting a membrane channel with selective permeability. E. tarda is an intracellular pathogen and can survive in host macrophages (Okuda et al., 2006; Liu et al., 2017). As a strategy of intracellular survival, E. tarda is able to detoxify the reactive oxygen species (ROS) generated by host phagocytes through the production of catalase and superoxide dismutases (Srinivasa Rao et al., 2003; Cheng et al., 2010). In our study, the survival rates of the TX01ΔtamA and TX01ΔtamB mutants after treatment with H2O2, which produces ROS, were significantly lower than that of the wild type, suggesting that the defective cellular structure caused by TamAEt and TamBEt mutation facilitated the H2O2-derived ROS to penetrate into the bacterial cells and kill the cells more effectively. This observation further supports the above conclusion that TamAEt and TamBEt are vital to the structural integrity of E. tarda.
The outer membrane of Gram-negative bacteria protects cells from external aggressions and mediates the secretion of virulence factors, and intact OMPs can promote host adhesion (Henderson and Nataro, 2001; Ranava et al., 2018). Efficient and correct assembly of integral OMPs requires the TAM complex (Heinz et al., 2015; Ranava et al., 2018). In Citrobacter rodentium, Salmonella enterica and E. coli, TAM mutation eliminated the virulence of the bacteria (Selkrig et al., 2012, 2014; Stubenrauch et al., 2016). In our study, we found that TX01ΔtamA and TX01ΔtamB exhibited significantly decreased ability to invade into and replicate in fish cells, and were significantly attenuated in the ability of tissue dissemination and inducing mortality in the host. These results indicated that TamAEt and TamBEt were required for optimal bacterial virulence. It is possible that mutation of tamAEt and tamBEt affects not only the membrane structure and permeability of the bacteria, but also the secretion of some virulence factors, which together lead to decreased survival in the stressing environment of the host.
Conclusion
Our study demonstrated that TamAEt and TamBEt are required for motility, flagella development, stress survival, and infectivity of E. tarda. TamAEt and TamBEt exert a significant impact on membrane integrity, which affects both the physiology and the pathogenicity of the bacteria. These results add new insights into the function of bacterial TAM and the survival mechanism of E. tarda.
Data Availability Statement
The datasets generated for this study can be found in the WP_012847252.1 of GenBank, WP_012847253.1 of GenBank.
Ethics Statement
The animal study was reviewed and approved by the Ethics Committee of Institute of Oceanology, Chinese Academy of Sciences.
Author Contributions
ML and LS conceived and designed the experiments. ML, BJ, and YS performed the experiments and analyzed the data. ML wrote the manuscript. LS edited the manuscript. All the authors contributed to the article and approved the submitted version.
Funding
This work was supported by the grants from the National Key Research and Development Program of China (2018YFD0900501), the Marine S&T Fund of Shandong Province for Pilot National Laboratory for Marine Science and Technology (Qingdao) (2018SDKJ0302-2), the National Natural Science Foundation of China (31972831), the Youth Innovation Promotion Association of the Chinese Academy of Sciences (2017249), the Huiquan Young Scholar Program of Institute of Oceanology, CAS, and the Taishan Scholar Program of Shandong Province.
Conflict of Interest
The authors declare that the research was conducted in the absence of any commercial or financial relationships that could be construed as a potential conflict of interest.
Supplementary Material
The Supplementary Material for this article can be found online at: https://www.frontiersin.org/articles/10.3389/fmicb.2020.01743/full#supplementary-material
References
Abayneh, T., Colquhoun, D. J., and Sørum, H. (2013). Edwardsiella piscicida sp. Nov., a novel species pathogenic to fish. J. Appl. Microbiol. 114, 644–654. doi: 10.1111/jam.12080
Akeda, Y., Kodama, T., Saito, K., Iida, T., Oishi, K., and Honda, T. (2011). Identification of the vibrio parahaemolyticus type III secretion system 2-associated chaperone VocC for the T3SS2-specific effector VopC. FEMS Microbiol. Lett. 324, 156–164. doi: 10.1111/j.1574-6968.2011.02399.x
Albenne, C., and Ieva, R. (2017). Job contenders: roles of the ß-barrel assembly machinery and the translocation and assembly module in autotransporter secretion. Mol. Microbiol. 106, 505–517. doi: 10.1111/mmi.13832
Begic, S., and Worobec, E. A. (2006). Regulation of Serratia marcescens ompF and ompC porin genes in response to osmotic stress, salicylate, temperature and pH. Microbiology 152, 485–491. doi: 10.1099/mic.0.28428-0
Burall, L. S., Harro, J. M., Li, X., Lockatell, C. V., Himpsl, S. D., Hebel, J. R., et al. (2004). Proteus mirabilis genes that contribute to pathogenesis of urinary tract infection: identification of 25 signature-tagged mutants attenuated at least 100-fold. Infect. Immun. 72, 2922–2938. doi: 10.1128/iai.72.5.2922-2938.2004
Cama, J., Bajaj, H., Pagliara, S., Maier, T., Braun, Y., Winterhalter, M., et al. (2015). Quantification of fluoroquinolone uptake through the outer membrane channel ompF of Escherichia coli. J. Am. Chem. Soc. 137, 13836–13843. doi: 10.1021/jacs.5b08960
Castro, N., Toranzo, A., Barja, J. L., Núñez, S., and Magariños, B. (2006). Characterization of Edwardsiella tarda strains isolated from turbot. Psetta maxima (L.). J. Fish Dis. 29, 541–547. doi: 10.1111/j.1365-2761.2006.00750.x
Chakraborty, S., Sivaraman, J., Leung, K. Y., and Mok, Y. K. (2011). Two-component PhoB-PhoR regulatory system and ferric uptake regulator sense phosphate and iron to control virulence genes in type III and VI secretion systems of Edwardsiella tarda. J. Biol. Chem. 286, 39417–39430. doi: 10.1074/jbc.M111.295188
Cheng, S., Zhang, M., and Sun, L. (2010). The iron-cofactored superoxide dismutase of Edwardsiella tarda inhibits macrophage-mediated innate immune response. Fish Shellfish Immunol. 29, 972–978. doi: 10.1016/j.fsi.2010.08.004
Givaudan, A., and Lanois, A. (2000). flhDC, the flagellar master operon of Xenorhabdus Nematophilus: requirement for motility, lipolysis, extracellular hemolysis, and full virulence in insects. J. Bacteriol. 182, 107–115. doi: 10.1128/jb.182.1.107-115.2000
Gruss, F., Zahringer, F., Jakob, R. P., Burmann, B. M., Hiller, S., and Maier, T. (2013). The structural basis of autotransporter translocation by TamA. Nat. Struct. Mol. Biol. 20, 1318–1320. doi: 10.1038/nsmb.2689
Hagan, C. L., Silhavy, T. J., and Kahne, D. (2011). ß-Barrel membrane protein assembly by the Bam complex. Annu. Rev. Biochem. 80, 189–210. doi: 10.1146/annurev-biochem-061408-144611
Heinz, E., Selkrig, J., Belousoff, M. J., and Lithgow, T. (2015). Evolution of the translocation and assembly module (TAM). Genome Biol. Evol. 7, 1628–1643. doi: 10.1093/gbe/evv097
Henderson, I. R., and Nataro, J. P. (2001). Virulence functions of autotransporter proteins. Infect. immun. 69, 1231–1243. doi: 10.1128/IAI.69.3.1231-1243.2001
Kelly, M., Hart, E., Mundy, R., Marches, O., Wiles, S., Badea, L., et al. (2006). Essential role of the type III secretion system effector NleB in colonization of mice by Citrobacter rodentium. Infect. Immun. 74, 2328–2337. doi: 10.1128/iai.74.4.2328-2337.2006
Klimentova, J., Pavkova, I., Horcickova, L., Bavlovic, J., Kofronova, O., Benada, O., et al. (2019). Francisella tularensis subsp. holarctica releases differentially loaded outer membrane vesicles under various stress conditions. Front. Microbiol. 10:2304. doi: 10.3389/fmicb.2019.02304
Korkmaz-Ozkan, F., Koster, S., Kuhlbrandt, W., Mantele, W., and Yildiz, O. (2010). Correlation between the OmpG secondary structure and its pH-dependent alterations monitored by FTIR. J. Mol. Biol. 401, 56–67. doi: 10.1016/j.jmb.2010.06.015
Leung, K. Y., Siame, B. A., Tenkink, B. J., Noort, R. J., and Mok, Y. K. (2012). Edwardsiella tarda - virulence mechanisms of an emerging gastroenteritis pathogen. Microbes Infect. 14, 26–34. doi: 10.1016/j.micinf.2011.08.005
Leung, K. Y., Wang, Q., Yang, Z., and Siame, B. A. (2019). Edwardsiella piscicida: a versatile emerging pathogen of fish. Virulence 10, 555–567. doi: 10.1080/21505594.2019.1621648
Li, M. F., Hu, Y. H., Zheng, W. J., Sun, B. G., Wang, C. L., and Sun, L. (2012). Inv1: an Edwardsiella tarda invasin and a protective immunogen that is required for host infection. Fish Shellfish Immunol. 32, 586–592. doi: 10.1016/j.fsi.2012.01.016
Li, M. F., Li, J., and Sun, L. (2016). CsMAP34, a teleost MAP with dual role: a promoter of MASP-assisted complement activation and a regulator of immune cell activity. Sci. Rep. 6:39287. doi: 10.1038/srep39287
Li, M. F., Sui, Z. H., and Sun, L. (2017). A teleost CD46 is involved in the regulation of complement activation and pathogen infection. Sci. Rep. 7:15028. doi: 10.1038/s41598-017-15124-y
Li, M. F., Wang, C., and Sun, L. (2015). Edwardsiella tarda Mlic, a lysozyme inhibitor that participates in pathogenesis in a manner that parallels Ivy. Infect. Immun. 83, 583–590. doi: 10.1128/iai.02473-14
Liu, L. Y., Nie, P., Yu, H. B., and Xie, H. X. (2017). Regulation of type III secretion of translocon and effector proteins by the EsaB/EsaL/EsaM complex in Edwardsiella tarda. Infect. Immun. 85:e00322-17. doi: 10.1128/IAI.00322-17
Marani, P., Wagner, S., Baars, L., Genevaux, P., de Gier, J. W., Nilsson, I., et al. (2006). New Escherichia coli outer membrane proteins identified through prediction and experimental verification. Protein Sci. 15, 884–889. doi: 10.1110/ps.051889506
Markham, A. P., Birket, S. E., Picking, W. D., Picking, W. L., and Middaugh, C. R. (2008). pH sensitivity of type III secretion system tip proteins. Proteins 71, 1830–1842. doi: 10.1002/prot.21864
Milton, D. L., O’Toole, R., Horstedt, P., and Wolf-Watz, H. (1996). Flagellin A is essential for the virulence of Vibrio anguillarum. J. Bacteriol. 178, 1310–1319. doi: 10.1128/jb.178.5.1310-1319.1996
Mohanty, B. R., and Sahoo, P. K. (2007). Edwardsiellosis in fish: a brief review. J. Biosci. 32, 1331–1344. doi: 10.1007/s12038-007-0143-8
Munera, D., Hultgren, S., and Fernandez, L. A. (2007). Recognition of the N-terminal lectin domain of FimH adhesin by the usher FimD is required for type 1 pilus biogenesis. Mol. Microbiol. 64, 333–346. doi: 10.1111/j.1365-2958.2007.05657.x
Nelson, J. J., Nelson, C. A., and Carter, J. E. (2009). Extraintestinal manifestations of Edwardsiella tarda infection: a 10-year retrospective review. J. La. State Med. Soc. 161, 103–106. doi: 10.1111/j.1742-1241.2005.00527.x
Okuda, J., Arikawa, Y., Takeuchi, Y., Mahmoud, M. M., Suzaki, E., Kataoka, K., et al. (2006). Intracellular replication of Edwardsiella tarda in murine macrophage is dependent on the type III secretion system and induces an up-regulation of anti-apoptotic NF-κB target genes protecting the macrophage from staurosporine-induced apoptosis. Microb. Pathog. 41, 226–240. doi: 10.1016/j.micpath.2006
Park, S. B., Aoki, T., and Jung, T. S. (2012). Pathogenesis of and strategies for preventing Edwardsiella tarda infection in fish. Vet. Res. 43:67. doi: 10.1186/1297-9716-43-67
Ranava, D., Caumont-Sarcos, A., Albenne, C., and Ieva, R. (2018). Bacterial machineries for the assembly of membrane-embedded ß-barrel proteins. FEMS Microbiol. Lett. 365:fny087. doi: 10.1093/femsle/fny087
Selkrig, J., Belousoff, M. J., Headey, S. J., Heinz, E., Shiota, T., Shen, H. H., et al. (2015). Conserved features in TamA enable interaction with TamB to drive the activity of the translocation and assembly module. Sci. Rep. 5:12905. doi: 10.1038/srep12905
Selkrig, J., Leyton, D. L., Webb, C. T., and Lithgow, T. (2014). Assembly of ß-barrel proteins into bacterial outer membranes. Biochim. Biophys. Acta 1843, 1542–1550. doi: 10.1016/j.bbamcr.2013.10.009
Selkrig, J., Mosbahi, K., Webb, C. T., Belousoff, M. J., Perry, A. J., Wells, T. J., et al. (2012). Discovery of an archetypal protein transport system in bacterial outer membranes. Nat. Struct. Mol. Biol. 19, 506–510. doi: 10.1038/nsmb.2261
Shao, S., Lai, Q., Liu, Q., Wu, H., Xiao, J., Shao, Z., et al. (2015). Phylogenomics characterization of a highly virulent Edwardsiella strain ET080813T encoding two distinct T3SS and three T6SS gene clusters: propose a novel species as Edwardsiella anguillarum sp.nov. Syst. Appl. Microbiol. 38, 36–47. doi: 10.1016/j.syapm.2014.10.008
Shen, H. H., Leyton, D. L., Shiota, T., Belousoff, M. J., Noinaj, N., Lu, J., et al. (2014). Reconstitution of a nanomachine driving the assembly of proteins into bacterial outer membranes. Nat. Commun. 5:5078. doi: 10.1038/ncomms6078
Singh, J. P., and Verma, R. (2008). Cloning and molecular characterization of outer membrane protein a (ompA) gene from Mycobacterium bovis. Microbiol. Immunol. 52, 410–417. doi: 10.1111/j.1348-0421.2008.00049.x
Srinivasa Rao, P. S., Yamada, Y., and Leung, K. Y. (2003). A major catalase (KatB) that is required for resistance to H2O2 and phagocyte-mediated killing in Edwardsiella tarda. Microbiology 149, 2635–2644. doi: 10.1099/mic.0.26478-0
Struve, C., Forestier, C., and Krogfelt, K. A. (2003). Application of a novel multi-screening signature-tagged mutagenesis assay for identification of Klebsiella pneumoniae genes essential in colonization and infection. Microbiology 149, 167–176. doi: 10.1099/mic.0.25833-0
Stubenrauch, C., Belousoff, M. J., Hay, I. D., Shen, H. H., Lillington, J., Tuck, K. L., et al. (2016). Effective assembly of fimbriae in Escherichia coli depends on the translocation assembly module nanomachine. Nat. Microbiol. 1:16064. doi: 10.1038/nmicrobiol.2016.64
Sui, Z. H., Xu, H., Wang, H., Jiang, S., Chi, H., and Sun, L. (2017). Intracellular trafficking pathways of Edwardsiella tarda: from clathrin- and caveolin-mediated endocytosis to endosome and lysosome. Front. Cell. Infect. Microbiol. 7:400. doi: 10.3389/fcimb.2017.00400
Sun, K., Zhang, W. W., Hou, J. H., and Sun, L. (2009). Immunoprotective analysis of VhhP2, a Vibrio harveyi vaccine candidate. Vaccine 27, 2733–2740. doi: 10.1016/j.vaccine.2009.03.012
Sun, Y., Zheng, W. J., Hu, Y. H., Sun, B. G., and Sun, L. (2012). Edwardsiella tarda eta1, an in vivo-induced antigen that is involved in host infection. Infect. Immun. 80, 2948–2955. doi: 10.1128/iai.00063-12
Wang, B., Yu, T., Dong, X., Zhang, Z., Song, L., Xu, Y., et al. (2013). Edwardsiella tarda invasion of fish cell lines and the activation of divergent cell death pathways. Vet. Microbiol. 163, 282–289. doi: 10.1016/j.vetmic.2012.12.027
Wang, X., Wang, Q., Xiao, J., Liu, Q., Wu, H., and Zhang, Y. (2010). Hemolysin EthA in Edwardsiella tarda is essential for fish invasion in vivo and in vitro and regulated by two-component system EsrA-EsrB and nucleoid protein HhaEt. Fish Shellfish Immunol. 29, 1082–1091. doi: 10.1016/j.fsi.2010.08.025
Xie, H. X., Lu, J. F., Rolhion, N., Holden, D. W., Nie, P., Zhou, Y., et al. (2014). Edwardsiella tarda-induced cytotoxicity depends on its type III secretion system and flagellin. Infect. Immun. 82, 3436–3445. doi: 10.1128/iai.01065-13
Xie, H. X., Yu, H. B., Zheng, J., Nie, P., Foster, L. J., Mok, Y. K., et al. (2010). EseG, an effector of the type III secretion system of Edwardsiella tarda, triggers microtubule destabilization. Infect. Immun. 78, 5011–5021. doi: 10.1128/iai.00152-10
Yang, D. H., Liu, X. H., Xu, W. T., Gu, Z. Y., Yang, C. T., Zhang, L. Z., et al. (2019). The Edwardsiella piscicida thioredoxin-like protein inhibits ASK1-MAPKs signaling cascades to promote pathogenesis during infection. PLoS Pathog. 15:e1007917. doi: 10.1371/journal.ppat.1007917
Zhang, B. C., and Sun, L. (2015). Tongue sole (Cynoglossus semilaevis) prothymosin alpha: cytokine-like activities associated with the intact protein and the C-terminal region that lead to antiviral immunity via Myd88-dependent and -independent pathways respectively. Dev. Comp. Immunol. 53, 96–104. doi: 10.1016/j.dci.2015.07.004
Zhang, M., Sun, K., and Sun, L. (2008). Regulation of autoinducer 2 production and luxS expression in a pathogenic Edwardsiella tarda strain. Microbiology 154, 2060–2069. doi: 10.1099/mic.0.2008/017343-0
Zhang, W. W., Sun, K., Cheng, S., and Sun, L. (2008). Characterization of DegQVh, a serine protease and a protective immunogen from a pathogenic Vibrio harveyi strain. Appl. Environ. Microbiol. 74, 6254–6262. doi: 10.1128/aem.00109-08
Zheng, J., and Leung, K. Y. (2007). Dissection of a type VI secretion system in Edwardsiella tarda. Mol. Microbiol. 66, 1192–1206. doi: 10.1111/j.1365-2958.2007.05993.x
Zheng, W. J., Hu, Y. H., and Sun, L. (2011). The two Dps of Edwardsiella tarda are involved in resistance against oxidative stress and host infection. Fish Shellfish Immunol. 31, 985–992. doi: 10.1016/j.fsi.2011.08.018
Keywords: Edwardsiella tarda, translocation and assembly module, acid tolerance, membrane integrity, virulence
Citation: Li M, Jia B, Sun Y and Sun L (2020) The Translocation and Assembly Module (TAM) of Edwardsiella tarda Is Essential for Stress Resistance and Host Infection. Front. Microbiol. 11:1743. doi: 10.3389/fmicb.2020.01743
Received: 24 February 2020; Accepted: 03 July 2020;
Published: 24 July 2020.
Edited by:
Lisa Sedger, University of Technology Sydney, AustraliaReviewed by:
Christopher Stubenrauch, Monash University, AustraliaJavier Santander, Memorial University of Newfoundland, Canada
Copyright © 2020 Li, Jia, Sun and Sun. This is an open-access article distributed under the terms of the Creative Commons Attribution License (CC BY). The use, distribution or reproduction in other forums is permitted, provided the original author(s) and the copyright owner(s) are credited and that the original publication in this journal is cited, in accordance with accepted academic practice. No use, distribution or reproduction is permitted which does not comply with these terms.
*Correspondence: Li Sun, bHN1bkBxZGlvLmFjLmNu