- 1Biosciences Division, Oak Ridge National Laboratory, Oak Ridge, TN, United States
- 2Faculty of Life and Environmental Sciences, University of Iceland, Reykjavik, Iceland
Geothermal hot springs are a natural setting to study microbial adaptation to a wide range of temperatures reaching up to boiling. Temperature gradients lead to distinct microbial communities that inhabit their optimum niches. We sampled three alkaline, high temperature (80–100°C) hot springs in Yellowstone and Iceland that had cooling outflows and whose microbial communities had not been studied previously. The microbial composition in sediments and mats was determined by DNA sequencing of rRNA gene amplicons. Over three dozen phyla of Archaea and Bacteria were identified, representing over 1700 distinct organisms. We observed a significant non-linear reduction in the number of microbial taxa as the temperature increased from warm (38°C) to boiling. At high taxonomic levels, the community structure was similar between the Yellowstone and Iceland hot springs. We identified potential endemism at the genus level, especially in thermophilic phototrophs, which may have been potentially driven by distinct environmental conditions and dispersal limitations.
Introduction
Archaea and Bacteria inhabit nearly every environment on Earth, including many that are inhospitable to multicellular life, such as hot springs. As geothermal water cools, outflowing from the source, temperature, chemical and redox gradients form. Distinct microbial communities occupy the various niches of such gradients, based on individual species adaptation to different temperature and chemical optima (Stetter, 1999; Reysenbach and Shock, 2002). Hot springs around the world have been used for decades as natural laboratories to study the effect of environmental parameters on microbial evolution, diversity and physiology (Castenholz, 1969; Brock and Darland, 1970; Skirnisdottir et al., 2000; Purcell et al., 2007; Hamilton et al., 2012; Menzel et al., 2015; Alcorta et al., 2018). Extreme temperature and pH values have been shown to have the largest contribution in restricting microbial diversity, although the magnitude of their effects are dependent on the hot spring and were also influenced by geochemistry and other environmental factors (Meyer-Dombard et al., 2011; Hamilton et al., 2012; Cole et al., 2013; Wang et al., 2013; Sharp et al., 2014; Chiriac et al., 2017; Merkel et al., 2017; Power et al., 2018; Tang et al., 2018; Zhang et al., 2018).
Hot springs have also been used to test hypotheses on factors and mechanisms that lead to microbial diversification and biodiversity patterns (Ward and Cohan, 2005; Martiny et al., 2006). The hypothesis that microbes in general have a high dispersal rate, that would homogenize genetic variations that may arise as result of local ecological and evolutionary events, has been challenged by studies of microbes in geothermal hot springs (Whitaker et al., 2003; Papke and Ward, 2004). Because numerous thermophilic microbes that do not form endospores do not survive for extended periods desiccated in air (Castenholz, 1969; Beblo et al., 2009) their dispersal ability over large geographic distances is limited. Therefore, while microbial communities that inhabit geochemically similar hot springs on different continents are expected to be physiologically and taxonomically similar, some of the individual species may evolve as endemic populations, similar to plants and animals on distant islands. This has been demonstrated by comparing thermophilic Synechococcus (Bacteria) and Sulfolobus (Archaea) in hot springs from North America, Europe and Asia (Papke et al., 2003; Whitaker et al., 2003; Becraft et al., 2020).
Here we studied the microbial diversity across temperature gradients in three alkaline hot springs from Yellowstone National Park (YNP) and Iceland for which there was no prior microbial data available. We hypothesized that even though those individual hot springs are geographically isolated, they would share the same general microbial community composition at high taxonomic levels (and potentially physiological activities) at similar temperatures along the gradient. While multiple previous studies identified clear effects of temperature on community richness (Cole et al., 2013; Cuecas et al., 2014; Sharp et al., 2014; Power et al., 2018) some studies on springs with temperatures below 80°C did not (Wang et al., 2013). Selecting hot spring gradients that span a wide range of temperatures up to boiling, enabled us to test the degree of diversity variation across temperature intervals. At the same time, because of the large geographical distance separating the hot springs in North America from those in Iceland, we performed analyses for potential genetic variation between shared taxa at equivalent temperatures. As relatively few comparative studies of distant hot springs around the world have previously been conducted, we aimed to expand such microbial diversity comparisons and also enable future integrative studies.
Materials and Methods
Icelandic Hot Spring Samples
Microbial mats, sediments, and water samples were collected on June 9, 2016 at a hot springs field in the village of Flúðir, Iceland (GPS coordinates 64°08′13″ N 20°18′34″ W). The main hot spring, Vaðmálahver (Figure 1), is alkaline (pH ∼8.5) and the source water is 98°C (boiling). The outflow of Vaðmálahver gradually cools and the water discharges in a nearby river. Several hot spring sources from the same site discharge in Hverahólmi, the oldest public swimming lagoon in Iceland. The temperature in the main source as well as in the outflow, sediments and in the microbial mats was measured using a Fisherbrand Traceable Waterproof Thermometer (Fisher Scientific cat no. 02-402-0) that had a stainless steel temperature probe at the end of a long (10 ft) wire cable positioned either manually or with a telescopic pole. The pH was measured onsite using non-bleeding pH indicator strips (pH 5–10 range, EMD Millipore) on source water and along the outflow. Water and sediment gravel (approximately 90% water-10% gravel by volume) from the main source were collected for geochemical analysis using a stainless-steel cup (500 ml) at the end of a telescopic pole and immediately poured into sterile 100 mL Pyrex glass bottles, capped with no air headspace and secured using butyl rubber stoppers and aluminum crimps. The samples were left to cool naturally to room temperature and then stored and transported cold. A sulfide test done on site using lead acetate strips (Sigma-Aldrich) was negative (limit of detection 3–5 mg/l). Submerged microbial mats and sediments (∼1–2 grams) were collected using sterile syringes and stainless-steel spatulas and placed into plastic tubes containing ceramic beads and 750 μl XpeditionTM Lysis/Stabilization Solution (Zymo Research, Irvine, CA) and lysed by bead-beating for one minute with a battery-operated tube shaker. That ensured cellular lysis, inactivation of degradative enzymes and stabilization of the DNA until further processing. A total of seven different spots were sampled from and around the Vaðmálahver spring, ranging from 98 to 47°C (Supplementary Figure S1 and Table 1). The samples in the outflow were sequentially collected going upstream to higher temperatures, all the way to the source. This is an important consideration in sampling hot spring outflows, as collecting going downstream in the runoff would lead to contamination of lower temperature samples with sediments and mats disturbed upward. The main source of an adjacent spring (temperature of 92°C) that flows into Hverahólmi was also collected, as well as mat and water samples from the lagoon (38°C). The microbial community from the lagoon (250 ml water sample) was collected on a Millipore Sterivex 0.2 mm syringe filter and preserved by adding Xpedition solution into the filter cartridge and then capping. A microbial mat sample was collected from a submerged rock in the lagoon and processed as were the other mat samples. With the exception of the lagoon water (planktonic sample), all other samples that we collected for microbiological characterization from all the hot springs were mats and gravel/sediment. After reaching the laboratory the lysed samples were stored at −20°C until DNA extraction or at 4°C (samples for geochemical analyses).
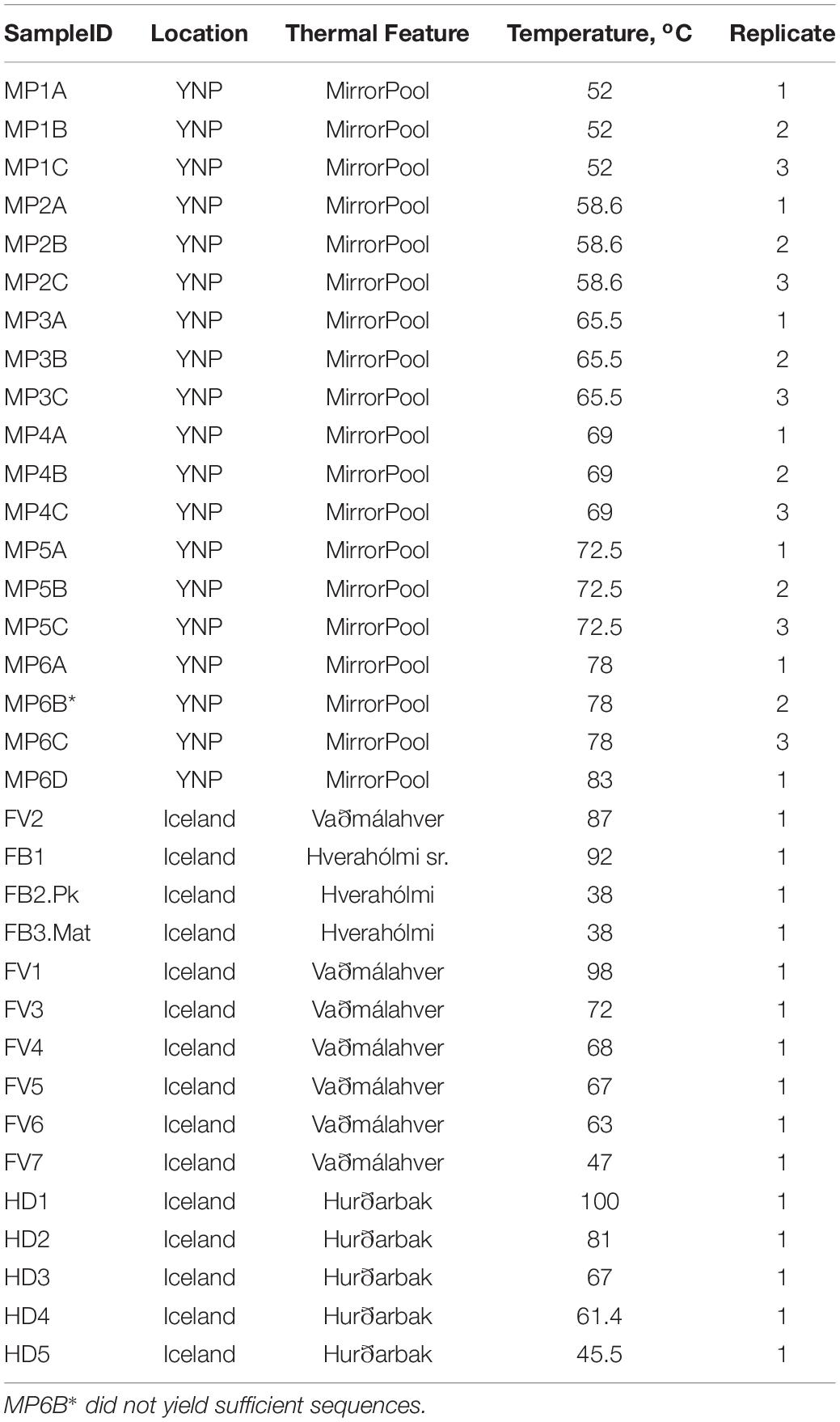
Table 1. Environmental samples used in the study. Except for FB2.Pk, which was a water sample, all others were mats/sediment.
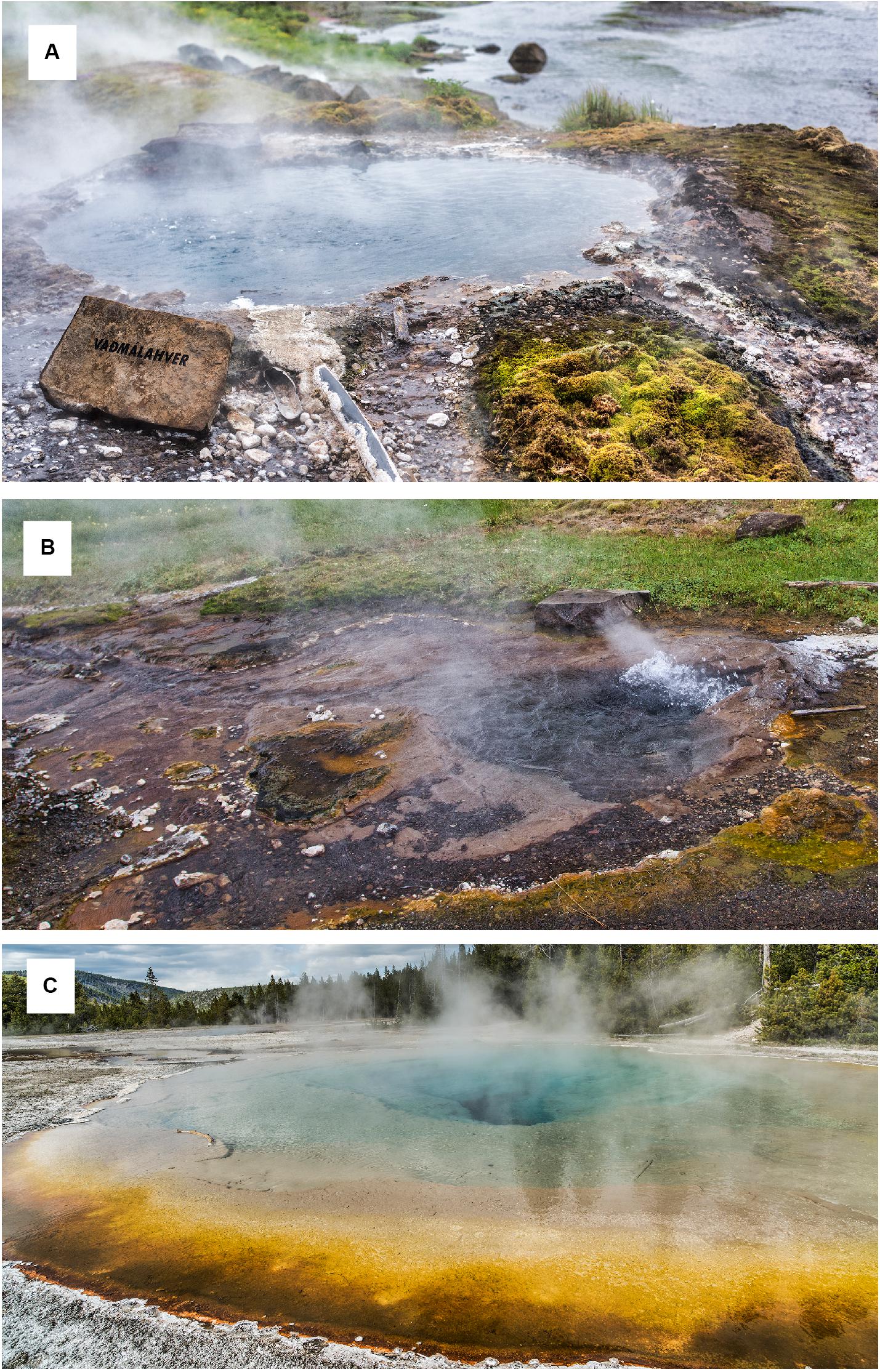
Figure 1. Overview of sampled thermal features. (A) Vaðmálahver, Flúðir (Iceland). (B) Hurðarbak, Borgarfjörður (Iceland). (C) Mirror Pool, Yellowstone National Park (United States). Actual sample collection points are presented in the Supplementary Figures S1–S3.
The second location in Iceland was also an alkaline spring (pH 8.0) at Hurðarbak (Figure 1), in the Borgarfjörður region [GPS coordinates 64°41′18″ N, 21°24′10″ W], approximately 80 km NW of Flúðir. Samples were collected on June 16, 2016. Five sampling spots were selected, with temperatures ranging from 99–102°C (the source spring) to 46°C in the outflow channel (Supplementary Figure S2 and Table 1). For geochemical analysis a water sample was collected from the source spring. Sample collection and processing were performed as described above.
Yellowstone National Park Hot Spring Samples
Microbial mats, sediment, and water samples were collected on December 31, 2016 at Mirror Pool, an alkaline (pH 8.0) thermal feature from the Upper Geyser Basin, in the Cascades Group [GPS coordinates 44°28′59″ N, 110°51′01″ W] (Figure 1). Nine sites were sampled along the main pool and gradient outflow of the spring, where temperatures ranged between 83°C–52°C (Supplementary Figure S3 and Table 1). Because the outflow of the pool was larger than for the hot springs in Iceland it was feasible to identify spots with the same temperature accessible for collection using spatulas. Therefore, three adjacent replicate samples (∼1 cm3 each) separated by less than 10 centimeters were collected for each temperature value, to determine the degree of diversity fluctuation across small scales. From the main pool, the collected samples were more distant and were scooped out from the stainless-steel canister. A water sample for geochemical analysis was also collected from the main pool. The samples were collected and processed as already described.
Water Chemistry Analysis
The chemical composition of the hot spring source water samples was performed at The University of Tennessee Knoxville Water Quality Core Facility. Carbon and nitrogen content were determined by thermal combustion and infrared detection with a Shimadzu carbon/nitrogen analyzer. The concentration of metals was measured by inductively coupled argon plasma (ICP) optical emission spectrometry using a Thermo-Scientific iCAP 7400 ICP spectrometer. Ions were measured by ion chromatography with a Thermo-Scientific®/Dionex ICS-2100 (anions) and ICS-1100 (cations), with background suppression for low detection limits.
DNA Extraction
Total genomic DNA from environmental samples was isolated using the ZR Soil Microbe DNA Kit (Zymo Research) following the manufacturer’s protocol. To isolate DNA from the high temperature sediment samples collected in the larger volume bottles, 25 mL of those samples subjected to centrifugation (12,000 × g for 20 min), the water carefully decanted, and the pellet suspended and lysed using the Zymo Lysis/Stabilization Solution following by processing as above. The concentration of DNA was determined using a Qubit dsDNA HS assay kit and fluorometer (Thermo Fisher Scientific).
Microbial SSU rRNA Gene Amplicon Sequencing
The V4 hypervariable region of the small subunit ribosomal RNA gene (SSU rRNA) was amplified using universal bacterial/archaeal 515F and 806R primers (Bates et al., 2011) fused to Illumina sequencing adapters, following the procedure developed by Lundberg et al. (Lundberg et al., 2013). To increase the coverage of archaeal groups not recognized effectively by the standard 515F and modified 806R primers (5′ GTGCCAGCMGCCGCGGTAA and 5′ GGACTACHVGGGTWTCTAA, respectively), we supplemented the reaction with further modified versions that included 20% 515FCren (5′ GTGKCAGCMGCCGCGGT AA, for Crenarchaeota), 5% 515FNano (5′ GTGGCAGYCG CCRCGGKAA, for Nanoarchaeota) and 5% 805RNano (5′ GGAMTACHGGGGTCTCTAAT, for Nanoarchaeota), similar to what was described in Liang et al. (2018). 12-nucleotide barcode sequences were incorporated into the second stage amplification reaction to enable sample multiplexing. The final amplicons were pooled, purified using Agencourt AMPure XP bead and quantified using Qubit. A diluted purified pooled amplicon sample (9 pM), containing 20% phiX DNA was denatured and sequenced (2 × 250 nt) on an Illumina MiSeq instrument (Illumina Inc., San Diego, CA) using a v2 500 cycle kit, according to manufacturer’s protocol.
Amplicon Sequence Analyses
The amplicon primer regions were trimmed from the raw FASTQ sequence files using cutadapt (Martin, 2016). The sequence reads were then de-multiplexed based on barcode sequences using the QIIME (Caporaso et al., 2010) python script split_libraries_fastq.py followed by splitting by individual samples using split_sequence_file_on_sample_ids.py. For one of the samples (MP6B) the number of sequences was very low (<500 sequences) and that sample was removed from analyses. Demultiplexed FASTQ paired reads were imported into QIIME2 v2019.7 (Bolyen et al., 2019) on a desktop computer. The reads were paired with VSEARCH (Rognes et al., 2016), quality filtered and denoised using Deblur (Amir et al., 2017). Resulting amplicon sequence variants (ASV) were aligned and used to generate a phylogenetic tree using the align-to-tree-mafft-fasttree pipeline from the q2-phylogeny plugin. To calculate alpha−diversity metrics [observed OTUs, Pielou’s eveness, Shannon’s index and Faith’s Phylogenetic Diversity (Faith, 1992)] beta diversity metrics [weighted UniFrac (Lozupone et al., 2007) and Bray−Curtis dissimilarity], and input the resulting matrices into principle coordinate analyses (PCoA) and visualization plots, we used the q2−diversity workflow, with rarefaction to 5000 sequences per sample (based on plateauing of the observed diversity and retaining of all samples). A general temperature classification of samples was generated by assigning each sample to groups separated by 5oC (from 40oC to 100oC). Environmental parameters that could impact alpha diversity were tested using Spearman correlation and analysis of variance (ANOVA), using q2 diversity alpha-correlation and q2 longitudinal (Bokulich et al., 2018b). To test for factors that contribute to microbial diversity differences between the samples (actual temperature, general temperature, location, hot spring) we used multi-way permutational multivariate analysis of variance (PERMANOVA) (q2 diversity adonis tests), comparing the variance explained by the various parameters singly or in combinations. Pairwise tests within metadata categories were performed by one-way PERMANOVA using the q2 diversity beta-group-significance.
To assign taxonomy to ASVs we used the q2−feature−classifier (Bokulich et al., 2018a) (classify−sklearn) against the Silva-132-99 SSU rRNA database (Pruesse et al., 2007). A table with the taxonomic classification of the reads for every sample is provided as a supplementary file (Supplementary Table S1). The raw FASTQ files are available in the NCBI SRA (accession numbers SRR11066910–SRR11066942, Bioproject PRJNA605860).
Phylogenetic Analyses
Phylogenetic trees to compare selected Yellowstone and Iceland ASVs with related organisms from GenBank were generated using PhyML in the software package Geneious1. BLASTN search algorithm was used to identify relatives of the Yellowstone and Iceland bacteria and archaea in public sequence databases followed by the phylogenetic reconstructions.
Results and Discussion
Geochemical Comparisons of the Three Hot Springs
The three hot springs were selected because of their high temperature (80–100°C at the source), similarities in pH and the presence of discharge channels with gradual cooling that harbor distinct microbial mats. Mirror Pool is a large (∼15 × 20 meters) non-erupting deep pool, in the Upper Geyser Basin thermal region (Cascade Group complex) of Yellowstone National Park. Abundant silica deposits are present both in the pool and on its edges and the outflow channel. The temperature and pH we recorded are similar to those reported in the YNP Research Coordination Network database2, 76–80°C and pH 8, measured in 1999 although we could not find previous geochemical data. Similar to other alkaline-siliceous chloride-type springs in that thermal region (Fournier, 1989), Mirror Pool has high levels of chloride, sodium, silica and arsenic but is low on sulfur or sulfate, calcium and magnesium (Table 2). While the concentration of dissolved sulfide was below the limit of detection using lead acetate test strips (∼5 mg/l), other hot springs in the Cascade Group, which share the same overall chemistry, were shown to be very low in sulfide (0.02 mg/l) (Thompson and DeMonge, 1996). Its relatively low flow rate and close proximity to the forest line are probably linked to the relatively high dissolved organic carbon (∼100 mg/l). Unlike Mirror Pool, the two sampled hot springs in Iceland were much smaller (<2 m in diameter), boiling and actively discharging, likely explaining their lower dissolved organic carbon content (10–20 mg/l). The overall mineral content of both springs was also lower than that of Mirror Pool, although they had higher levels of sulfate, calcium, iron and sulfide. Sulfide concentration is also higher in the two Icelandic hot springs (1.34 mg/l in Vaðmálahver and 1.2 mg/l at Hurðarbak), based on published measurements (Arnorsson and Gunnlaugsson, 1983; Ali, 1997). We could not find matched data in the literature on other dissolved gasses that we could not measure onsite (O2, H2, CO2, CO, CH4). We recognize therefore that, as the water flows from the source and cools, there may be changes in the water chemistry that we have not accounted for, such as dissolved gases, precipitation of minerals, microbial metabolic products.
Temperature Differentially Influences the Microbial Alpha Diversity
The combined sequencing of the SSU rRNA amplicons from all samples resulted in over 5.7 million sequences. After de-multiplexing, quality-based filtering, denoising, chimera and singleton removal, the number of sequences for individual samples ranged from 5,315 to 332,920, with an average of ∼115,000 sequences per sample and a total of 1729 amplicon sequence variants (ASVs) (unique taxa). Because the type of clustering algorithm and selection of similarity level impacts the number of traditional operational taxonomic units (OTUs), we only used ASVs for calculation of diversity indices. Depending on the degree of sequence variability in the V4 region for the various taxa, we expect ASVs to provide resolution to genera, species and below, based on comparisons of know species of Archaea and Bacteria [e.g (Shakya et al., 2013)].
For studying the microbial diversity within each sample (alpha diversity) we used both direct counts of the number of ASVs as well as metrics that take into account the evenness of diversity (Pielou’s evenness), abundance and distribution of the taxa (Shannon’s index) or the phylogenetic diversity (Faith’s diversity). In Mirror Pool, where we were able to take spatially separated samples at the same temperature, there were differences in alpha diversity between environmental replicates, with the coefficient of variation ranging from 0.2%–12%. The higher deviations were for high temperature samples. Those differences were, however, minor compared to the temperature-linked differences. At the Icelandic hot springs, temperature had the largest impact and was inversely correlated with the number of detected taxa (ASVs) and phylogenetic diversity (Spearman p = 0.006 and p = 0.000, respectively) (Figure 2). The decline in number and diversity of microbial taxa with temperature does not appear to be linear and is steeper in the ranges corresponding to the transition between mesophily and thermophily (35–45oC) and between thermophily and hyperthermophily (>80°C). Such non-linear relationships have been previously reported for hot spring communities in Canada, New Zealand, United States (Nevada) and Thailand where wide temperature ranges were present within individual thermal systems (Cole et al., 2013; Cuecas et al., 2014; Sharp et al., 2014). ANOVA tests of potential multiple effects on the alpha diversity confirmed that, while the temperature value had the largest influence (P-Value from F-Ratio = 3.4e-05), the individual thermal feature was significant too (P-Value from F-Ratio = 3.6e-03, passing pairwise T-tests with BH-FDR). In Mirror Pool, where the available temperature range was narrower, its effect on alpha diversity was minor. When only strict thermophilic temperature values were analyzed for the Icelandic features as well (50–80°C, the range sampled in Mirror Pool), the temperature effect on alpha diversity was absent, which may explain reports of no temperature effect on species richness (Wang et al., 2013). Also, when analyzing the temperature distribution of non-phylogenetic alpha diversity evenness indices (Pielou’s, Shannon’s), we observed that samples spanning the 67–80°C were sharply higher relative to what appears to be relatively linear distribution across the other two temperature ranges (Figure 2). While we cannot provide a definitive explanation for these differences, one possibility is that in the 67–80°C range there are major shifts in the microbial communities, with the stratified microbial mats dominated by photosynthetic groups (Cyanobacteria, Chloroflexi) being replaced by various extreme thermophilic taxa (Thermi, Aquificae, Crenarchaeota) that are not spatially organized, with no dominating members. As the temperature further increases, the number of organisms that can survive decreases and certain taxa dominate (Pyrobaculum, Ignisphaera, Thermocrinis), leading to a reduced evenness. We further investigated this by calculating the Shannon’s index at different sequence similarity clustering levels (between 97% and 75% similarity levels). Interestingly, the effect is maintained even at distances corresponding to family order levels OTUs (∼90% level OTUs), and the diversity index begins to somewhat flatten for the thermophilic range in the class-phylum categories (80-75% level OTUs) but is followed by the abrupt drop at the extreme temperatures (>80°C). This suggests that the non-linear distribution of Shannon’s evenness index is linked to major shifts on how the communities are structured across the temperature gradient. These results and observations highlight the importance of sampling multiple temperature ranges in hot springs and the choice of diversity indices in studying such environments.
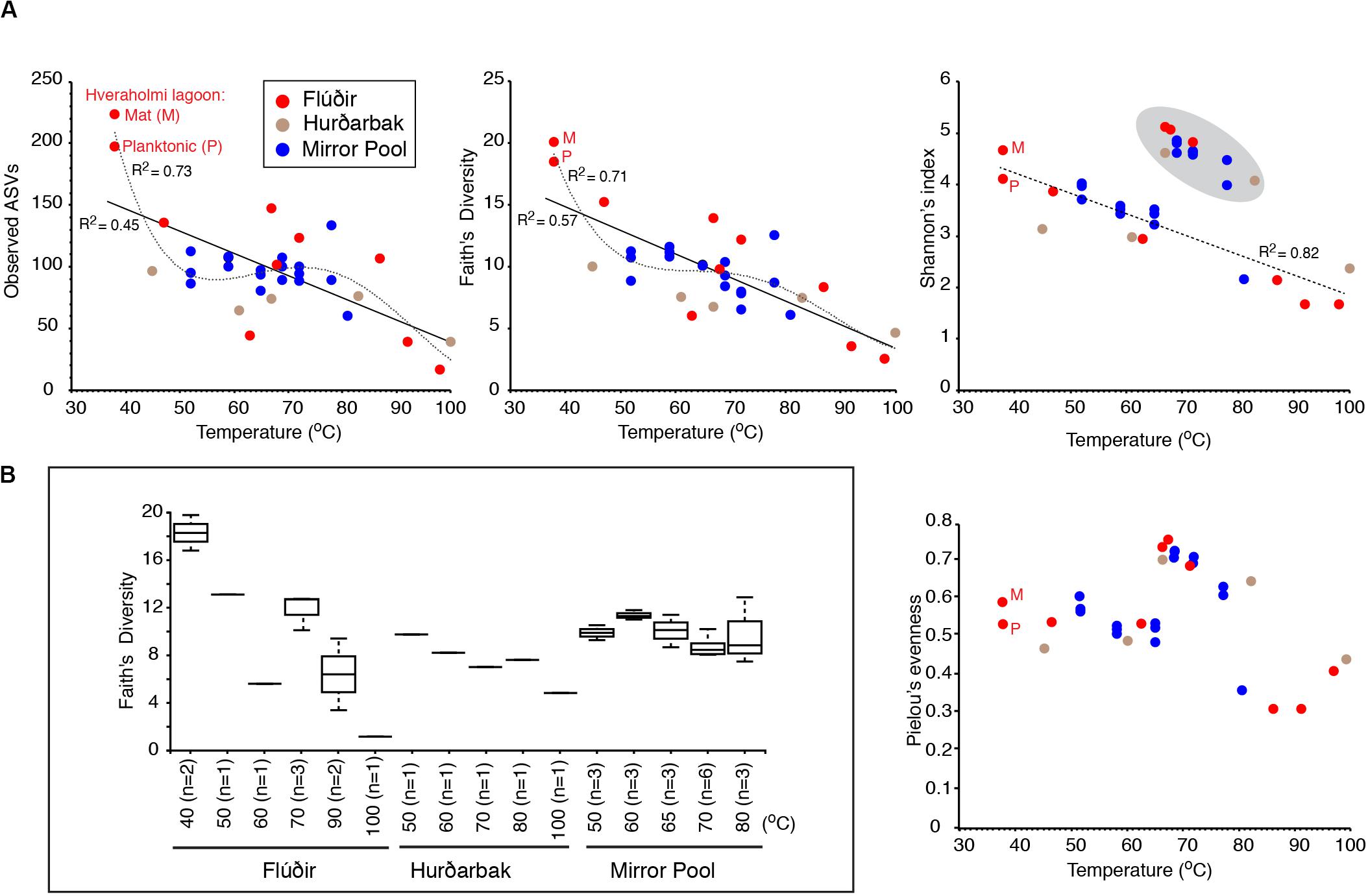
Figure 2. Microbial alpha diversity versus temperature. (A) Scatterplots of various diversity indices versus temperature. Linear or polynomial regression and goodness of fit are shown. For the Shannon’s index, the effect of excluding the upper cluster values (circled) on linear regression fit is shown. (B) Scatterplots of diversity indices vs. temperature after excluding the Cyanobacteria and Chloroflexi sequences.
Temperature and Biogeography Effects on Beta Diversity
While alpha diversity analyses revealed the microbial community structure within individual samples collected across temperatures and locations, it does not enable a direct comparison of communities between samples (beta diversity) (Lozupone and Knight, 2008). Because some individual ASVs may represent closely related ecotypes, species or genera, we also aimed to compare the community structure across samples, temperature and locations taking into account taxonomic (and potential physiological) relatedness of the various organisms. We therefore used weighted UniFrac as a quantitative beta diversity metric that incorporates both the phylogenetic distance between ASVs and their relative abundance (Lozupone and Knight, 2005, 2008). The multi-dimensional UniFrac distance matrices were condensed through a principal coordinate analysis (PCoA) into a three-dimensional space, in which the microbial diversity characteristics of each sample is represented by a discrete data point. Communities that have similar types and abundance of species are closer to each other in that space than those that consist of different types of microbes. The PCoA plot shows that most samples from close temperature values cluster together (are similar) regardless of the hot spring or location (Iceland or Yellowstone) (Figure 3), with most of the variation (∼77%) explained in the three main coordinates. A test of the combined effect of temperature and location (which includes underlying chemical differences) to beta diversity revealed over 80% of the variation explained by those two factors (ADONIS R2 = 0.877, p = 0.001). Temperature was, however, the most important driving factor (PERMANOVA pseudoF = 10.4, p = 0.001, ANOSIM R = 0.77, p = 0.001) (Figure 4), which is also evident based on the PCoA plot (Figure 3), where samples are distributed primarily by temperature rather than location or thermal feature. Most sample replicates from Yellowstone grouped tightly together, except for some at higher temperatures that display a larger dissimilarity. Therefore, even though there are distinct differences in some of the measured chemical composition of the springs at the source, these appear to be secondary factors in shaping of the microbial communities when we compared these alkaline hot springs. Shaping of the microbial communities by other physical and chemical factors have nevertheless been documented in comparisons of thermal environments, for example hydrogen concentration (Spear et al., 2005) pH (Power et al., 2018; Colman et al., 2019) and minerals (Mathur et al., 2007). We also recognize that, even though temperature was the dominant factor measured here, changes in temperature along the outflows may be driving specific changes in water chemistry that we did not measure. Such temperature-associated changes also could impact the microbial communities, but we cannot distinguish them here. For example, it has been shown that in acidic geothermal springs, water cooling results in precipitation of metals, changes in dissolved gasses, generating chemical energy gradients that in turn shape the microbial communities along the gradient (Macur et al., 2004). To our knowledge, such chemical gradients have not been measured in thermal alkaline outflows.
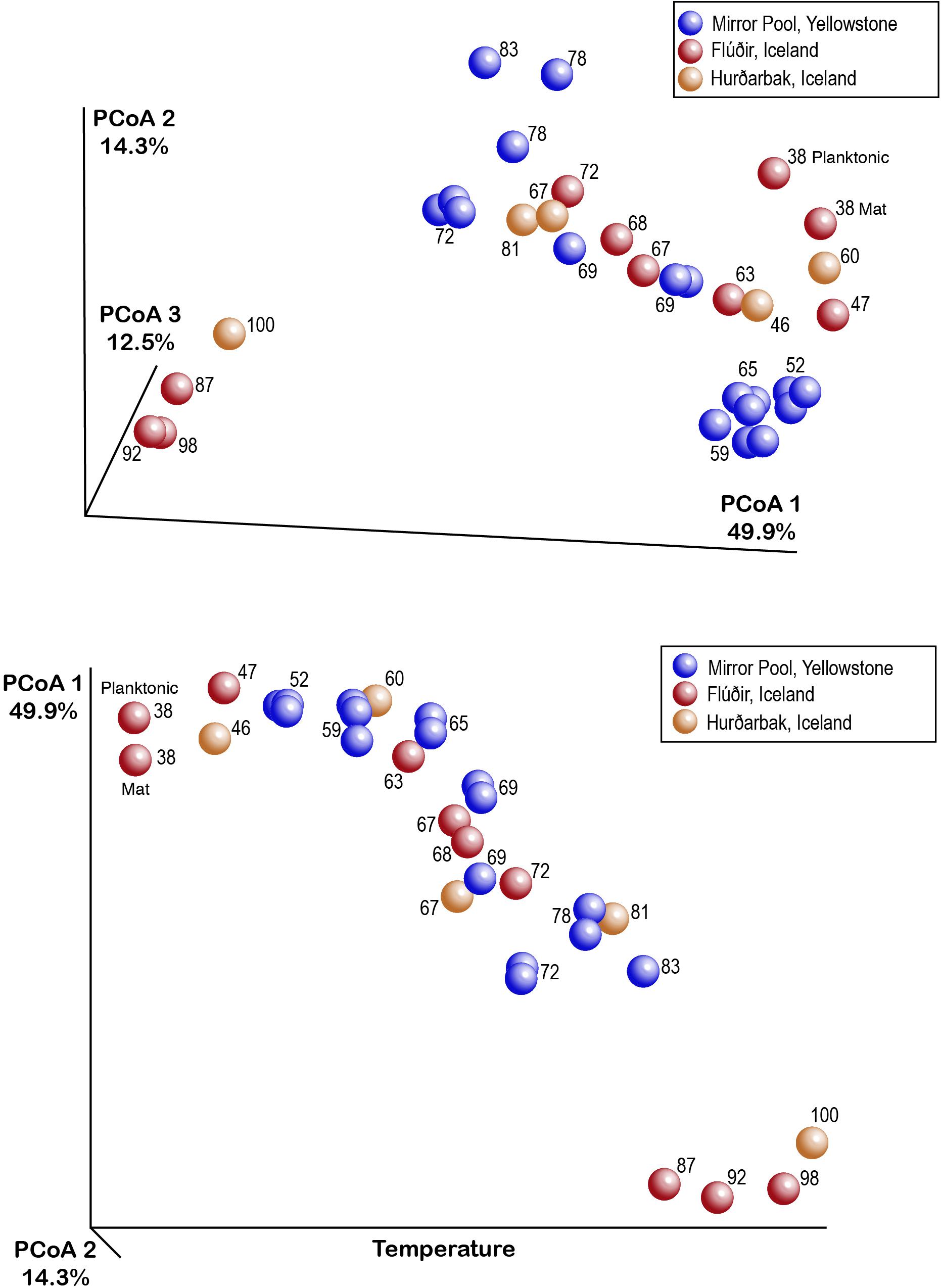
Figure 3. Representation of hot springs microbial beta diversity through EMPeror plots of the principal coordinates analysis output for weighted UniFrac distances.
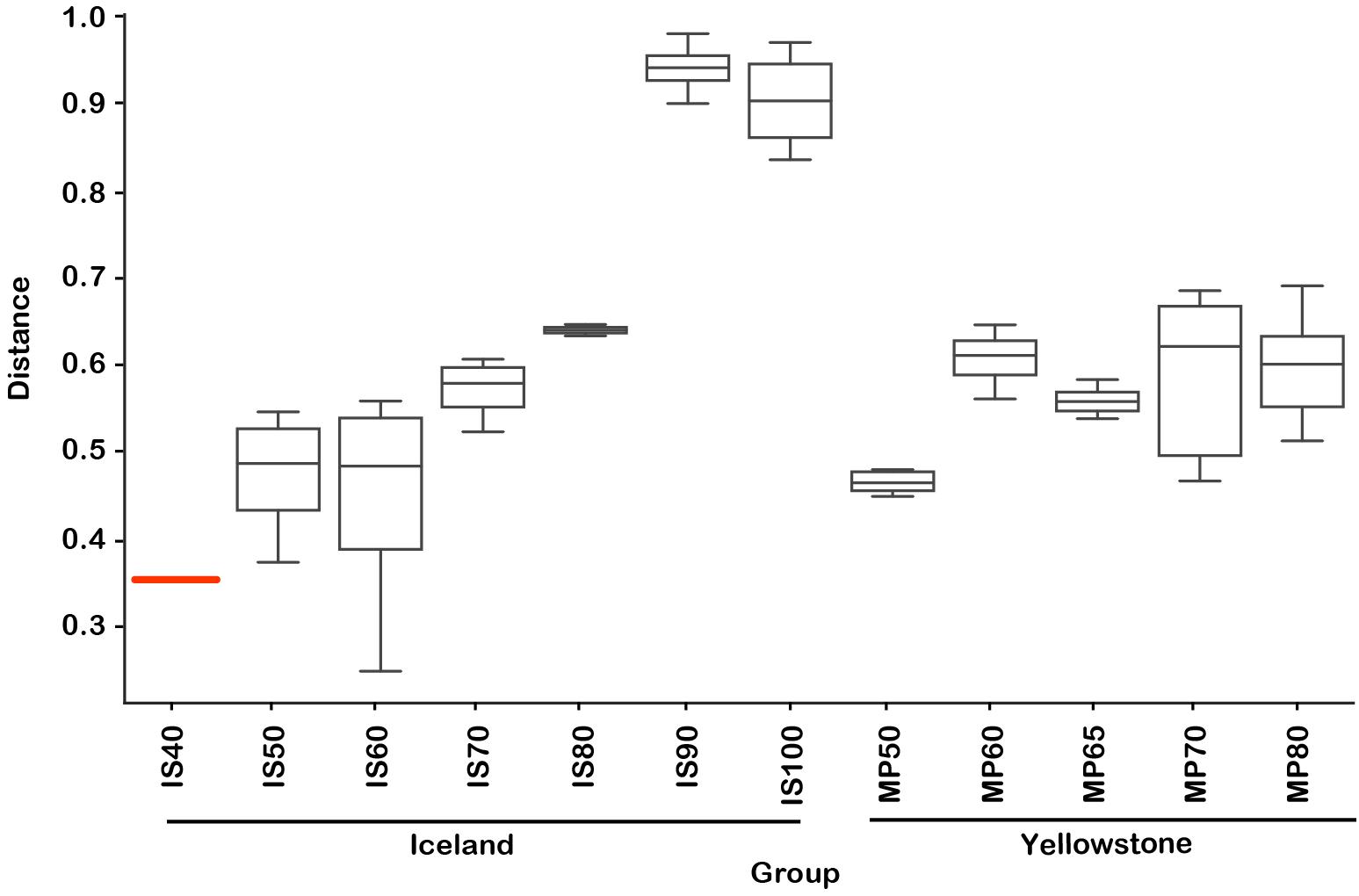
Figure 4. Weighted UniFrac location-temperature group significance plot. Distances are relative to the lowest temperature group (Flúðir Hverahólmi, Iceland, 40°C). PERMANOVA F-test significance p = 0.001. IS, Iceland.
Microbial Taxonomy Across Temperature Gradients and Hot Springs
The 1729 unique sequence variants were assigned to 182 genera, corresponding to 5 phyla of Archaea (9 classes) and 40 phyla of Bacteria (86 classes) (Figures 5, 6). The temperature gradient that forms in the three distinct hot spring systems creates distinct niches where organisms that are best adapted to those conditions thrive. In all those systems, such niches can be distinguished even macroscopically, based on the morphology and color of the mats (Supplementary Figures S1–S3). The deep amplicon sequencing that we achieved revealed the presence and relative abundance of numerous groups of organisms including the rare taxa. At the lowest temperature (38°C), the water and microbial mats of the Hverahólmi lagoon are dominated by a large diversity of mesophilic and mildly thermophilic heterotrophic as well as photosynthetic bacteria, including Alpha and Betaproteobacteria (Roseomonas, Rhodobacter, Tepidimonas), Bacteroidetes (Chitinophaga, Saprospira) and Cyanobacteria (Cyanobium, Leptolyngbya) (Figure 6). The overall diversity is slightly higher in the mat than in the water column although the overall community structure and taxonomic composition are the same (Figures 2, 3). Some differences may, however, be due to the difference in collection and processing between the two sample types (filtration versus complete mat biomass). As the lagoon receives a constant stream of high temperature hot spring water, we also detected numerous extreme thermophilic and hyperthermophilic archaea and bacteria in the lagoon (e.g., Pyrobaculum, Aquificae, Thermi) at <0.1% of total sequences. While those organisms increase the alpha diversity, the lagoon being the most diverse of the sampled niches, at tens of degrees below their optimum they most likely represent a physiologically inactive, non-dividing component of the community (Stetter, 2006). Some, depending on their capacity to survive low temperatures and exposure to oxygen, may have the potential to colonize other hot springs by different dispersal mechanisms (water, wind).
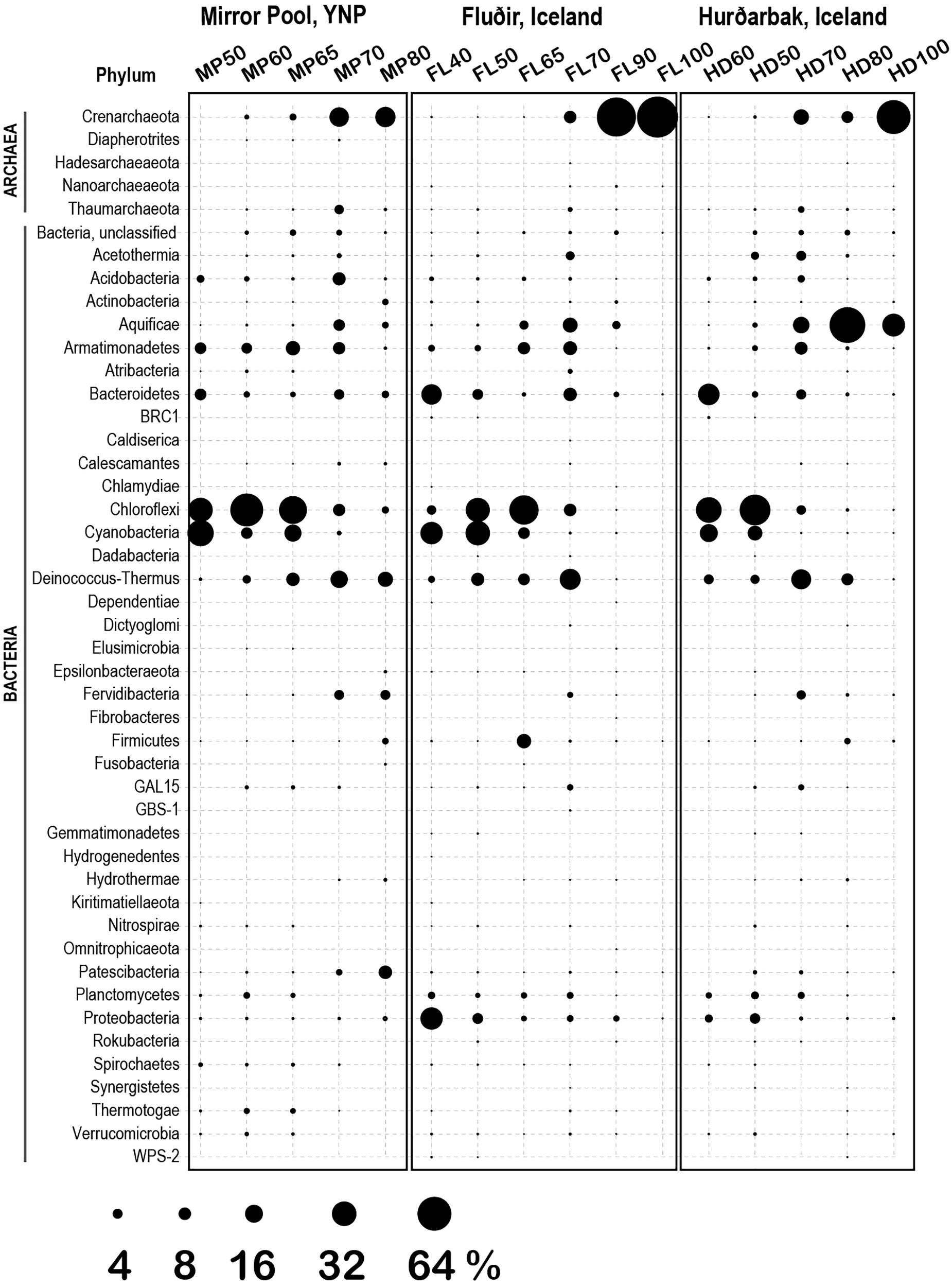
Figure 5. Microbial diversity (phylum level) at the three thermal springs depending on general temperature (40–100°C). Circle size indicates the inferred relative abundance, based on amplicon data (in %).
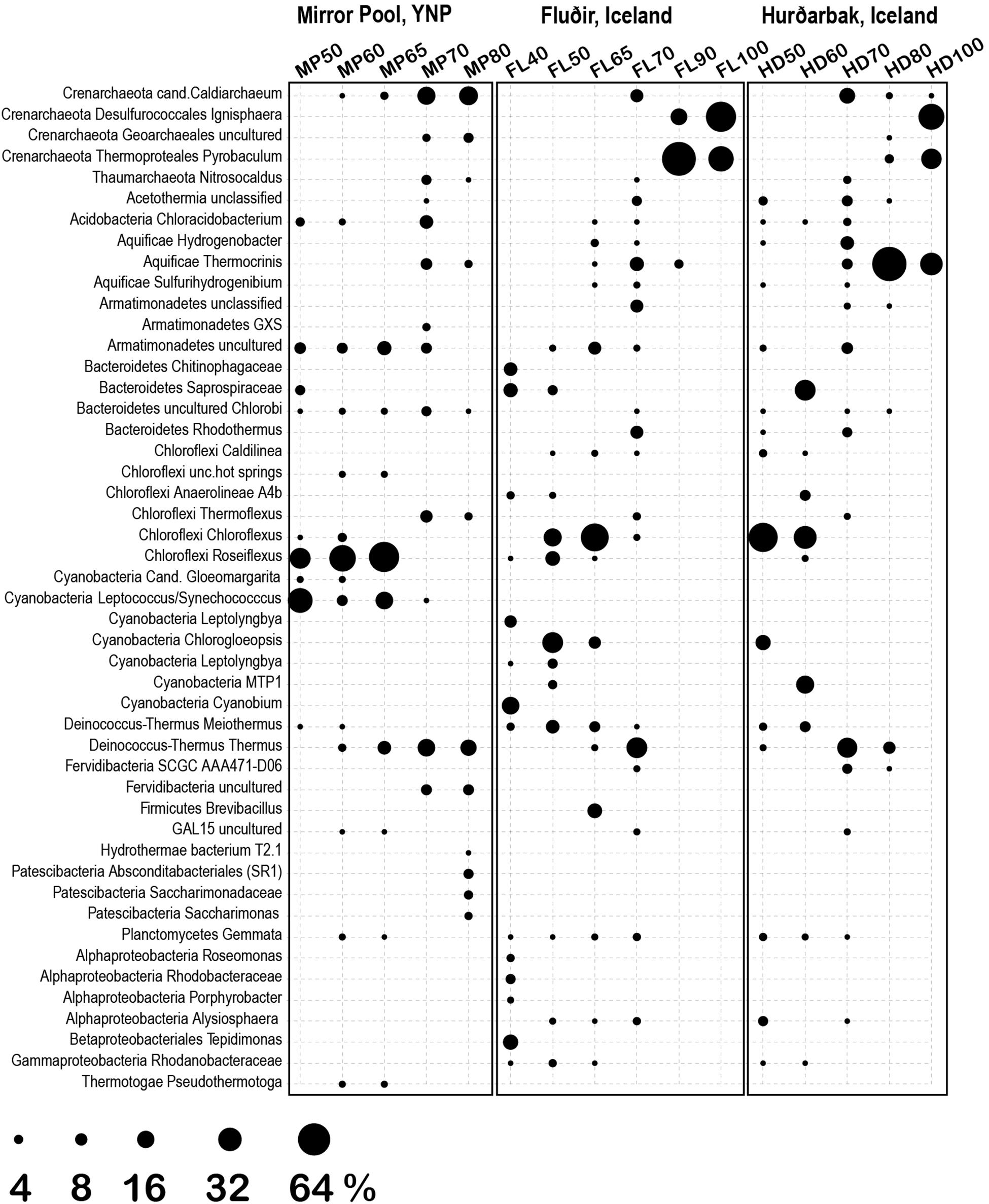
Figure 6. Most abundant genera/families at the three thermal springs depending on general temperature. Circle size indicates the inferred relative abundance based on amplicon data (in %).
The thermal areas (50–80°C) of all three hot springs share a variety of common organisms including Armatimonadetes, Chlorobi, Planctomycetes at the lower range, and Thaumarchaeota, Aquificae (Thermocrinis) and Thermi, at the upper temperature range. There are though some specific differences, including in the distribution of Aigarchaeota (Crenarchaeota). In Mirror Pool we observed two distinct types of Aigarchaeota. The most abundant phylotype is related to Candidatus Caldiarchaeaum subterraneum, an uncultured, likely heterotrophic archaeon identified in subsurface geothermal aquifers (Nunoura et al., 2011; Takami et al., 2015). The Mirror Pool lineage was also identified in other Yellowstone alkaline hot springs (Queens Laundry) (Meyer-Dombard et al., 2011) and, based on relative abundance, appears to prefer temperatures around 70°C although was present also at lower temperatures. The Yellowstone lineage is absent in the two Icelandic hot springs that we sampled, which, however, hosts a related archaeon, more closely related to Candidatus Caldiarchaeum subterraneum, over a similar temperature range (Supplementary Table S1, Figure S5). The other major group of Aigarchaeota was represented by several phylotypes related to the more recently described Candidatus Calditenuis aerorheumensis from Octopus Spring (Yellowstone), an organism proposed to potentially chemoautotrophically use oxygen as a terminal electron acceptor (Beam et al., 2016). Related lineages have been found in a variety of other alkaline and circumneutral hot spring including the Great Boiling Spring in Nevada (Rinke et al., 2013) Belcher Spring (Yellowstone) (Colman et al., 2015) Iceland (Mirete et al., 2011) and even in a shallow marine hydrothermal vent from Papua New Guinea (GenBank EF100632, Meyer-Dombard D.R., unpublished) (Supplementary Figure S5). In Mirror Pool these organisms inhabit the higher temperature range, unlike the Caldiarchaeum-type, presumably as the very low sulfide level allows sufficient dissolved oxygen. In the two Icelandic hot springs the Calditenuis-type lineages were present at much lower relative abundance. We could not test redox levels in the different niches of the hot spring runoff therefore these potential associations to oxygen and sulfide content remain speculative.
The dominant community members between 50–65°C were oxygenic and anoxygenic chlorophototrophs (diverse Cyanobacteria, Chloroflexi and Chloracidobacterium), forming characteristic green, orange and red mats depending on the site and temperature, as it has been shown in many other hot springs around the world (Miller et al., 2009; Klatt et al., 2013; Wang et al., 2013; Cuecas et al., 2014; Thiel et al., 2016). There were, however, some notable differences between Mirror Pool and the Icelandic features (Figure 6). Synechococcus/Leptococcus, an abundant member of the mats in Yellowstone, as well as Gloeomargarita, were absent in the two Icelandic hot springs. The striking absence of Synechococcus in Icelandic hot springs has been documented decades ago along with an overall lower diversity of thermophilic Cyanobacteria (Castenholz, 1969; Hreggvidsson et al., 2017). Two potential explanations have been proposed. As the light levels in Iceland severely decrease during the winter months, that could limit the survival of phototrophs that require a higher energy level. In addition, during the winter months at high latitudes, the temperature gradient changes, which would require upstream outflow colonization, a double challenge with the low light levels (Castenholz, 1969). Alternatively, it has been hypothesized that thermophilic Synechococcus and other species that are sensitive to freezing and desiccation are less likely to survive the time required for dispersal from sources in North America or Eurasia (Miller and Castenholz, 2000). As it has been well documented that some Cyanobacteria are highly sensitive to sulfide (Castenholz, 1977; Cohen et al., 1986; Skirnisdottir et al., 2000; Hamilton et al., 2012) that may also contribute to differences between the springs, as the levels in Vaðmálahver and Hurðarbak reach those toxic levels (∼1 mg/l). The Icelandic mats harbored, however, diverse other Cyanobacteria, primarily at 50°C and below, including Chlorogloeopsis and Leptolyngbya. We also observed important differences across Chloroflexi between the thermal regions. Chloroflexi are more tolerable to higher levels of sulfide and can detoxify it using a type II sulfide:quinone oxidoreductase or, some species, can use it as an electron donor for photoautotrophy (Bryant et al., 2012). The Mirror Pool mats are dominated by Roseiflexus, while the Icelandic mats are primarily composed of Chloroflexus. Mats with different abundance of Chloroflexus versus Roseiflexus have been found across hot springs in YNP and, because they were not correlated with the concentration of sulfide, unidentified environmental factors appear to drive those differences (Klatt et al., 2013). There are complex physiological interactions between photosynthetic autotrophs and heterotrophs in the mats as well as microbial partitioning driven by light levels, chemical gradients and competition (Ramsing et al., 2000; Ward and Cohan, 2005; Klatt et al., 2013; Cuecas et al., 2014; Nowack et al., 2015). Distinguishing the contribution of biogeography versus geochemistry in the composition of Icelandic versus Yellowstone mats clearly requires additional studies.
Above 70–73°C, the upper temperature limit for photosynthesis, the microbial mats disappeared sharply, and all communities were composed primarily of Archaea, Aquificae, Armatimonadetes and Thermi, as well as a variety of other phyla including uncultured lineages (Figure 5). As the highest temperature in Mirror Spring was 82°C, we could not directly compare strict hyperthermophilic communities (>85°C) between the Yellowstone and Iceland springs. Both sources of Hurðarbak and Vaðmálahver springs (90–100°C) were dominated by the strictly hyperthermophilic Crenarchaeota Pyrobaculum and Ignisphaera but also contained low levels of Nanoarchaeota. Pyrobaculum (ord. Thermoproteales) are predominantly anaerobes, with one species isolated form Iceland (P. islandicus) being a strict anaerobe, facultative lithoautotrophs (Huber et al., 2006). That may explain why they are abundant in the more reduced, sulfate rich and organic poor Hurðarbak and Vaðmálahver. Ignisphaera, an anaerobic heterotroph, a less characterized member of the Desulfurococcales, with one species isolated from New Zealand being very sensitive to sodium chloride (Niederberger et al., 2006) which may explain its absence from Mirror Pool. Hurðarbak also had a significant population of Thermocrinis, an autotrophic member of the Aquificae that dominated the community at 80 °C but was a minor component in both Vaðmálahver and Mirror Pool. As Thermocrinis have been isolated both from YNP and Iceland (Huber et al., 1998; Eder and Huber, 2002), it is not clear what causes their differential relative abundance between the springs we studied. Another chemical characteristic that we cannot clearly link to a microbial differential feature is the significantly more abundant organic carbon in Mirror Pool, although that may result in overall higher microbial productivity. The relatively few studies that have correlated chemical gradients of springs with microbial composition and productivity revealed limiting energetic and nutritional factors that impact the communities in different microenvironments (Macur et al., 2004; Colman et al., 2016; Lindsay et al., 2018; Havig and Hamilton, 2019). Future combined analyses of datasets generated from multiple studies and locations should better define environmental parameters that shape the composition of those communities and the abundance of specific organisms.
Potential Endemism at Icelandic and Yellowstone Springs
Inspection of unique sequence variants revealed potential lineages that may be endemic to hot springs in Iceland or Yellowstone. Geographic separation of thermophilic communities or Archaea and Cyanobacteria between multiple sites across three continents has previously been shown to result in divergence of local populations that would ultimately lead to speciation (Castenholz, 1969; Papke et al., 2003; Whitaker et al., 2003). Therefore, phylogenetic analysis was used to characterize several closely related sequences between Icelandic and Yellowstone hot springs, to test whether short amplicon sequences many provide sufficient resolution. The included taxa were Archaea, Chloroflexi and Cyanobacteria lineages (Figure 7), taxa that have previously analyzed in the context of biogeography. The genetic distance between those sequences suggests indeed they represent related but distinct species or subspecies. Some of the sequences from Mirror Pool are very closely related or identical to prior sequences reported from other Yellowstone springs. Because we did not find close homologs from Icelandic hot springs in rRNA sequence databases, the analyzed Vaðmálahver and Hurðarbak amplicons had their closest relatives in hot springs from China. The analysis of the Aigarchaeota phylotypes (Supplementary Figure S5), discussed in the previous section, may also point to endemism, potentially driven by a combination of environmental factors (temperature and redox levels) and geographic separation. Across the entire dataset, a large fraction of ASVs were specific to either Yellowstone or Iceland. Specifically, out of the 654 ASVs with at least 100 sequences between all samples (>0.003% of the 3.8 million sequences assigned to ASVs), 56% were only identified in the Icelandic springs, 37% only in Yellowstone, while only 7% of the ASVs were common between YNP and Iceland. Some of unique ASVs were high abundance taxa (e.g., a Ca. Caldiarchaeum ASV was represented by over 50,000 sequences from Iceland and no sequence from YNP while a Chloroflexus ASV had ∼240,000 sequences from YNP and no sequence from Iceland). The distribution of all ASVs in the Icelandic and Yellowstone springs and their taxonomic classification is provided in the Supplementary Table S2. Because the resolution enabled by ASVs varies across microbial taxa, it is not possible to conclude that certain species or subspecies are unique to one of another location, as multiple ASVs were classified to the same taxonomic rank.
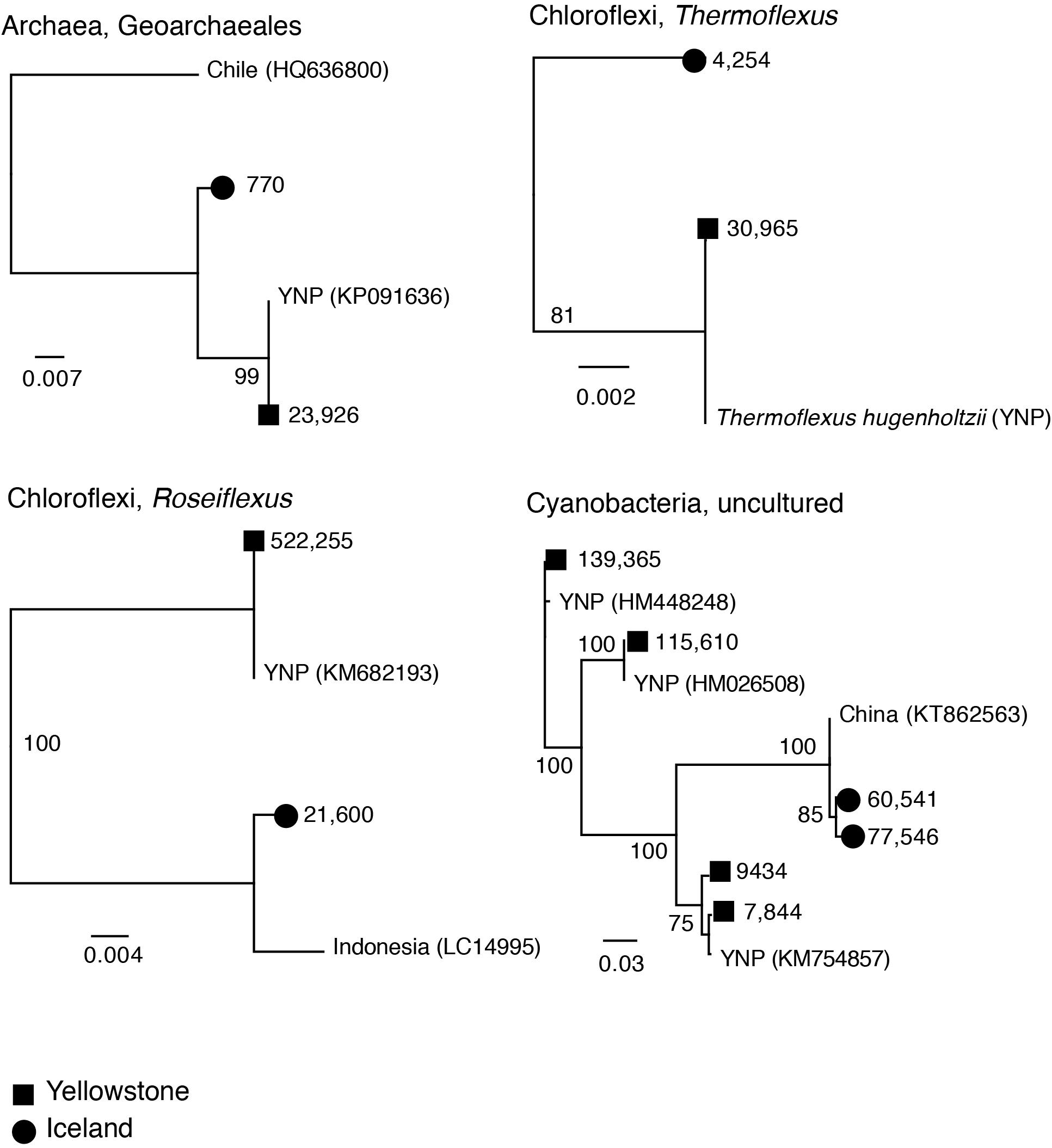
Figure 7. Neighbor joining trees (JC corrected distances) of potential endemic amplicon sequence variants (ASVs) from Iceland and Yelowstone (Mirror Pool). Numbers on branch tips indicate abundance across the entire dataset. Reference sequences and accession numbers from public rRNA databases and location were included. The numbers at nodes indicate bootstrap support. Scale bar indicates inferred number of substitutions per site.
While preliminary, based on few sampled sites and short sequences, these observations support the hypothesis that endemic lineages evolved in those hot springs and are in line with the previous studies comparing Icelandic geothermal systems with those from North America, continental Europe and Asia. The taxon differences could also be due to differences in chemical composition of the hot springs, which may have selected for related but globally distributed species. Nevertheless, the studies by Papke and colleagues (Papke et al., 2003; Papke and Ward, 2004) that included more locations and deeper phylogenetic analyses, concluded that chemical differences did not explain observed differences in species distribution across continents. Independent analyses of hot springs across the world should therefore enrich our understanding of the links between the ecological diversity and evolutionary history of thermophilic organisms, at local and global scales.
Author’s Note
This manuscript has been released as a pre-print at bioRxiv (https://doi.org/10.1101/841700), Podar P.T. et al., “Comparative analysis of microbial diversity across temperature gradients in hot springs from Yellowstone and Iceland”, Dec. 11, 2019 (Podar et al., 2019).
Data Availability Statement
The raw FASTQ files are available in the NCBI SRA (accession numbers SRR11066910–SRR11066942, Bioproject PRJNA605860).
Author Contributions
PP and MP designed the study analyzed the data and wrote the manuscript with input from SB and ZY. PP, SB, and MP collected the environmental samples. PP and ZY performed the DNA extraction, amplification, and sequencing. All authors contributed to the article and approved the submitted version.
Funding
This research was funded in part by grants from the National Science Foundation (DEB1134877) and the National Aeronautics and Space Administration (NNX16AJ66G). Oak Ridge National Laboratory is managed by UT-Battelle, LLC, for the United States Department of Energy under contract DE-AC05-00OR22725.
Conflict of Interest
The authors declare that the research was conducted in the absence of any commercial or financial relationships that could be construed as a potential conflict of interest.
Acknowledgments
Environmental sampling in Iceland was under permits issued by Iceland’s National Energy Authority (Orkustofnun) to MP and SB. We thank Dr. Jakob Kristjánsson for help with sampling and permits. Sampling in Yellowstone National Park was under permit YELL-SCI-5714 and we thank Stacey Gunther for help with sampling coordinating. We thank Adrian Gonzalez from The University of Tennessee Knoxville Water Quality Core Facility for chemical analysis of the water samples.
Supplementary Material
The Supplementary Material for this article can be found online at: https://www.frontiersin.org/articles/10.3389/fmicb.2020.01625/full#supplementary-material
Footnotes
References
Alcorta, J., Espinoza, T., Viver, M. E., Alcaman-Arias, N., Trefault, R., Rossello-Mora, R., et al. (2018). Temperature modulates Fischerella thermalis ecotypes in porcelana hot spring. Syst. Appl. Microbiol. 41, 531–543. doi: 10.1016/j.syapm.2018.05.006
Ali, I. (1997). Exploration of Geothermal Fluids in the Reykholt Area, Borgarfjordur, W-Iceland. Geothermal Training Programme. Reykjavik: The United Nations University, 195–220.
Amir, A., McDonald, J. A., Navas-Molina, E., Kopylova, J. T., Morton, Z., Zech, E. P., et al. (2017). Deblur rapidly resolves single-nucleotide community sequence patterns. mSystems 2:e00191-16.
Arnorsson, S., and Gunnlaugsson, E. (1983). The chemistry of geothermal waters in Iceland. II. Mineral equilibria and independent variables controlling water compositions. Geochim. Cosmochim. Acta 47, 547–566. doi: 10.1016/0016-7037(83)90277-6
Bates, S. T., Berg-Lyons, J. G., Caporaso, W. A., Walters, R., Knight, P., and Fierer, N. (2011). Examining the global distribution of dominant archaeal populations in soil. ISME J. 5, 908–917. doi: 10.1038/ismej.2010.171
Beam, J. P., Jay, M. C., Schmid, D. B., Rusch, M. F., Romine, M., Jennings, M. A., et al. (2016). Ecophysiology of an uncultivated lineage of Aigarchaeota from an oxic, hot spring filamentous ‘streamer’ community. ISME J. 10, 210–224. doi: 10.1038/ismej.2015.83
Beblo, K., Rabbow, R., Rachel, H., Huber, E., and Rettberg, P. (2009). Tolerance of thermophilic and hyperthermophilic microorganisms to desiccation. Extremophiles 13, 521–531. doi: 10.1007/s00792-009-0239-1
Becraft, E. D., Wood, F. M., Cohan, M., and Ward, D. M. (2020). Biogeography of American Northwest hot spring a/b’-lineage synechococcus populations. Front. Microbiol. 11:77. doi: 10.3389/fmicb.2020.00077
Bokulich, N. A., Dillon, Y., Zhang, J. R., Rideout, E., Bolyen, H., Li, P. S., et al. (2018a). q2-longitudinal: longitudinal and paired-sample analyses of microbiome data. mSystems 3:e00219-18.
Bokulich, N. A., Kaehler, J. R., Rideout, M., Dillon, E., Bolyen, R., Knight, G. A., et al. (2018b). Optimizing taxonomic classification of marker-gene amplicon sequences with QIIME 2’s q2-feature-classifier plugin. Microbiome 6:90.
Bolyen, E., Rideout, M. R., Dillon, N. A., Bokulich, C. C., Abnet, G. A., Al-Ghalith, H., et al. (2019). Reproducible, interactive, scalable and extensible microbiome data science using QIIME 2. Nat. Biotechnol. 37, 852–857.
Brock, T. D., and Darland, G. K. (1970). Limits of microbial existence: temperature and pH. Science 169, 1316–1318. doi: 10.1126/science.169.3952.1316
Bryant, D., Liu, T., Li, F., Zhao, A. M., Garcia Costas, C. G., Klatt, D. M., et al. (2012). “Comparative and functional genomics of anoxygenic green bacteria from the Taxa Chlorobi, Chloroflexi, and Acidobacteria,” in Functional Genomics and Evolution of Photosynthetic Systems, eds R. L. Burnap and W. F. J. Vermaas (Dordrecht: Springer), 47–102. doi: 10.1007/978-94-007-1533-2_3
Caporaso, J., Kuczynski, J., Stombaugh, J., and Bittinger, K. (2010). QIIME allows analysis of high-throughput community sequencing data. Nat. Methods 7, 335–336.
Castenholz, R. (1969). The thermophilic cyanophytes of Iceland and the upper temperature limit. J. Phycol. 5, 360–368. doi: 10.1111/j.1529-8817.1969.tb02626.x
Castenholz, R. W. (1977). The effect of sulfide on the blue-green algae of hot springs II. Yellowstone National Park. Microb. Ecol. 3, 79–105. doi: 10.1007/bf02010399
Chiriac, C. M., Szekeres, K., Rudi, A., Baricz, A., Hegedus, N., Dragos, N., et al. (2017). Differences in temperature and water chemistry shape distinct diversity patterns in thermophilic microbial communities. Appl. Environ. Microbiol. 83:e01363-17.
Cohen, Y., Jorgensen, N. P., Revsbech, P., and Poplawski, R. (1986). Adaptation to hydrogen sulfide of oxygenic and anoxygenic photosynthesis among Cyanobacteria. Appl. Environ. Microbiol. 51, 398–407. doi: 10.1128/aem.51.2.398-407.1986
Cole, J. K., Peacock, J. A., Dodsworth, A. J., Williams, D. B., Thompson, H., Dong, G., et al. (2013). Sediment microbial communities in Great Boiling Spring are controlled by temperature and distinct from water communities. ISME J. 7, 718–729. doi: 10.1038/ismej.2012.157
Colman, D. R., Feyhl-Buska, K. J., Robinson, K. M., Fecteau, H., Xu, E. L., Shock, K., et al. (2016). Ecological differentiation in planktonic and sediment-associated chemotrophic microbial populations in Yellowstone hot springs. FEMS Microbiol. Ecol. 92:fiw137. doi: 10.1093/femsec/fiw137
Colman, D. R., Lindsay, M. J., Amenabar, R., and Boyd, E. S. (2019). The intersection of geology, geochemistry, and microbiology in continental hydrothermal systems. Astrobiology 19, 1505–1522. doi: 10.1089/ast.2018.2016
Colman, D. R., Thomas, K. R., Maas, R., and Takacs-Vesbach, C. D. (2015). Detection and analysis of elusive members of a novel and diverse archaeal community within a thermal spring streamer consortium. Extremophiles 19, 307–313. doi: 10.1007/s00792-014-0715-0
Cuecas, A., Portillo, W., Kanoksilapatham, M., and Gonzalez, J. M. (2014). Bacterial distribution along a 50 degrees C temperature gradient reveals a parceled out hot spring environment. Microb. Ecol. 68, 729–739. doi: 10.1007/s00248-014-0437-y
Eder, W., and Huber, D. (2002). New isolates and physiological properties of the Aquificales and description of Thermocrinis albus sp. nov. Extremophiles 6, 309–318. doi: 10.1007/s00792-001-0259-y
Faith, D. P. (1992). Conservation evaluation and phylogenetic diversity. Biol. Cons. 61, 1–10. doi: 10.1016/0006-3207(92)91201-3
Fournier, R. O. (1989). Geochemistry and dynamics of the Yellowstone National Park hydrothermal system. Ann. Rev. Earth Planet. Sci. 17, 13–53. doi: 10.1146/annurev.ea.17.050189.000305
Hamilton, T. L., Vogl, D. A., Bryant, E. S., Boyd, S., and Peters, J. W. (2012). Environmental constraints defining the distribution, composition, and evolution of chlorophototrophs in thermal features of Yellowstone National Park. Geobiology 10, 236–249. doi: 10.1111/j.1472-4669.2011.00296.x
Havig, J. R., and Hamilton, R. L. (2019). Productivity and community composition of low biomass/high silica precipitation hot springs: a possible window to earth’s early biosphere? Life 9:64. doi: 10.3390/life9030064
Hreggvidsson, G. O., Petursdottir, S. K., Stefansson, S. H., Bjorndottir, O. H., and Fridjonsson, P. (2017). “Divergence of species in the geothermal environment,” in Adaptation of Microbial Life to Environmental Extremes, eds H. Stan-Lotter and S. Fendrihan (Cham: Springer), 41–74. doi: 10.1007/978-3-319-48327-6_3
Huber, H., Huber, R., and Stetter, K. O. (2006). “Thermoproteales,” in The Prokaryotes, eds M. Dworkin, S. Falkow, E. Rosenberg, K. H. Schleifer, and E. Stackebrandt (New York, NY: Springer), 10–22. doi: 10.1007/0-387-30743-5_2
Huber, R., Eder, S., Heldwein, G., Wanner, H., Huber, R., Rachel, K., et al. (1998). Thermocrinis ruber gen. nov., sp. nov., A pink-filament-forming hyperthermophilic bacterium isolated from yellowstone national park. Appl. Environ. Microbiol. 64, 3576–3583. doi: 10.1128/aem.64.10.3576-3583.1998
Klatt, C. G., Inskeep, M. J., Herrgard, Z. J., Jay, D. B., Rusch, S. G., Tringe, M., et al. (2013). Community structure and function of high-temperature chlorophototrophic microbial mats inhabiting diverse geothermal environments. Front. Microbiol. 4:106. doi: 10.3389/fmicb.2013.00106
Liang, X., Whitham, E. K., Holwerda, X., Shao, L., Tian, Y. W., Wu, V., et al. (2018). Development and characterization of stable anaerobic thermophilic methanogenic microbiomes fermenting switchgrass at decreasing residence times. Biotechnol. Biofuels 11:243.
Lindsay, M. R., Amenabar, K. M., Fecteau, R. V., Debes, M. C., Fernandes, K. E., Fristad, H., et al. (2018). Subsurface processes influence oxidant availability and chemoautotrophic hydrogen metabolism in Yellowstone hot springs. Geobiology 16, 674–692. doi: 10.1111/gbi.12308
Lozupone, C., and Knight, R. (2005). UniFrac: a new phylogenetic method for comparing microbial communities. Appl. Environ. Microbiol. 71, 8228–8235. doi: 10.1128/aem.71.12.8228-8235.2005
Lozupone, C. A., Hamady, S. T., Kelley, T., and Knight, R. (2007). Quantitative and qualitative beta diversity measures lead to different insights into factors that structure microbial communities. Appl. Environ. Microbiol. 73, 1576–1585. doi: 10.1128/aem.01996-06
Lozupone, C. A., and Knight, R. (2008). Species divergence and the measurement of microbial diversity. FEMS Microbiol. Rev. 32, 557–578. doi: 10.1111/j.1574-6976.2008.00111.x
Lundberg, D. S., Yourstone, P., Mieczkowski, C. D., Jones, D., and Dangl, J. L. (2013). Practical innovations for high-throughput amplicon sequencing. Nat. Methods 10, 999–1002. doi: 10.1038/nmeth.2634
Macur, R. E., Langner, H., and Inskeep, W. (2004). Linking geochemical processes with microbial community analysis: successional dynamics in an arsenic-rich, acid-sulphate-chloride geothermal spring. Geobiology 2, 163–177. doi: 10.1111/j.1472-4677.2004.00032.x
Martin, M. (2016). Cutadapt removes adapter sequences from high-throughput sequencing reads. EMBnet J. 17, 10–12.
Martiny, J. B., Bohannan, J. H., Brown, R. K., Colwell, J. A., Fuhrman, J. L., Green, M. C., et al. (2006). Microbial biogeography: putting microorganisms on the map. Nat. Rev. Microbiol. 4, 102–112. doi: 10.1038/nrmicro1341
Mathur, J., Bizzoco, D. G., Ellis, D. A., Lipson, A. W., Poole, R., Levine, R., et al. (2007). Effects of abiotic factors on the phylogenetic diversity of bacterial communities in acidic thermal springs. Appl. Environ. Microbiol. 73, 2612–2623. doi: 10.1128/aem.02567-06
Menzel, P., Gudbergsdottir, A. G., Rike, L., Lin, Q., Zhang, P., Contursi, M., et al. (2015). Comparative metagenomics of eight geographically remote terrestrial hot springs. Microb. Ecol. 70, 411–424. doi: 10.1007/s00248-015-0576-9
Merkel, A. Y., Pimenov, A. I., Slobodkin, G. B., Slobodkina, I. Y., Tarnovetckii, E. N., Frolov, A. V., et al. (2017). Microbial diversity and autotrophic activity in Kamchatka hot springs. Extremophiles 21, 307–317. doi: 10.1007/s00792-016-0903-1
Meyer-Dombard, D. R., Swingley, J., Raymond, J., Havig, E. L., Shock, L., and Summons, R. E. (2011). Hydrothermal ecotones and streamer biofilm communities in the Lower Geyser Basin, Yellowstone National Park. Environ. Microbiol. 13, 2216–2231. doi: 10.1111/j.1462-2920.2011.02476.x
Miller, S. R., and Castenholz, R. W. (2000). Evolution of thermotolerance in hot spring cyanobacteria of the genus Synechococcus. Appl. Environ. Microbiol. 66, 4222–4229. doi: 10.1128/aem.66.10.4222-4229.2000
Miller, S. R., Strong, K. L., Jones, H., and Ungerer, M. (2009). Bar-coded pyrosequencing reveals shared bacterial community properties along the temperature gradients of two alkaline hot springs in Yellowstone National Park. Appl. Environ. Microbiol. 75, 4565–4572. doi: 10.1128/aem.02792-08
Mirete, S., de Figueras, C. G., and Gonzalez-Pastor, J. E. (2011). Diversity of Archaea in Icelandic hot springs based on 16S rRNA and chaperonin genes. FEMS Microbiol. Ecol. 77, 165–175. doi: 10.1111/j.1574-6941.2011.01095.x
Niederberger, T. D., Gotz, I. R., McDonald, R. S., Ronimus, S., and Morgan, H. W. (2006). Ignisphaera aggregans gen. nov., sp. nov., a novel hyperthermophilic crenarchaeote isolated from hot springs in Rotorua and Tokaanu, New Zealand. Int. J. Syst. Evol. Microbiol. 56(Pt 5), 965–971. doi: 10.1099/ijs.0.63899-0
Nowack, S., Olsen, G. A., Schaible, E. D., Becraft, G., Shen, I., Klapper, D. A., et al. (2015). The molecular dimension of microbial species: 2. Synechococcus strains representative of putative ecotypes inhabiting different depths in the Mushroom Spring microbial mat exhibit different adaptive and acclimative responses to light. Front. Microbiol. 6:626. doi: 10.3389/fmicb.2015.00626
Nunoura, T., Takaki, J., Kakuta, S., Nishi, J., Sugahara, H., Kazama, G. J., et al. (2011). Insights into the evolution of Archaea and eukaryotic protein modifier systems revealed by the genome of a novel archaeal group. Nucleic Acids Res. 39, 3204–3223. doi: 10.1093/nar/gkq1228
Papke, R. T., Ramsing, M. M., Bateson, N., and Ward, D. M. (2003). Geographical isolation in hot spring cyanobacteria. Environ. Microbiol. 5, 650–659. doi: 10.1046/j.1462-2920.2003.00460.x
Papke, R. T., and Ward, D. M. (2004). The importance of physical isolation to microbial diversification. FEMS Microbiol. Ecol. 48, 293–303. doi: 10.1016/j.femsec.2004.03.013
Podar, P. T., Yang, S. H., Bjorndottir, H., and Podar, M. (2019). Comparative analysis of microbial diversity across temperature gradients in hot springs from Yellowstone and Iceland. bioRxiv [Preprint]. doi: 10.1101/841700
Power, J. F., Carere, C. K., Lee, G. L. J., Wakerley, D. W., Evans, M., Button, D., et al. (2018). Microbial biogeography of 925 geothermal springs in New Zealand. Nat. Commun. 9:2876.
Pruesse, E., Quast, K., Knittel, B. M., Fuchs, W., Ludwig, J., Peplies, R., et al. (2007). SILVA: a comprehensive online resource for quality checked and aligned ribosomal RNA sequence data compatible with ARB. Nucleic Acids Res. 35, 7188–7196. doi: 10.1093/nar/gkm864
Purcell, D., Sompong, L. C., Yim, T. G., Barraclough, Y., Peerapornpisal, L., and Pointing, D. S. (2007). The effects of temperature, pH and sulphide on the community structure of hyperthermophilic streamers in hot springs of northern Thailand. FEMS Microbiol. Ecol. 60, 456–466. doi: 10.1111/j.1574-6941.2007.00302.x
Ramsing, N. B., Ferris, M., and Ward, D. M. (2000). Highly ordered vertical structure of Synechococcus populations within the one-millimeter-thick photic zone of a hot spring cyanobacterial mat. Appl. Environ. Microbiol. 66, 1038–1049. doi: 10.1128/aem.66.3.1038-1049.2000
Reysenbach, A. L., and Shock, E. (2002). Merging genomes with geochemistry in hydrothermal ecosystems. Science 296, 1077–1082. doi: 10.1126/science.1072483
Rinke, C., Schwientek, A., Sczyrba, N. N., Ivanova, I. J., Anderson, J. F., Cheng, A., et al. (2013). Insights into the phylogeny and coding potential of microbial dark matter. Nature 499, 431–437. doi: 10.1038/nature12352
Rognes, T., Flouri, B., Nichols, C., Quince, C., and Mahe, F. (2016). VSEARCH: a versatile open source tool for metagenomics. PeerJ 4:e2584. doi: 10.7717/peerj.2584
Shakya, M., Quince, J. H., Campbell, Z. K., Yang, C. W., Schadt, W., and Podar, M. (2013). Comparative metagenomic and rRNA microbial diversity characterization using archaeal and bacterial synthetic communities. Environ. Microbiol. 15, 1882–1899. doi: 10.1111/1462-2920.12086
Sharp, C. E., Brady, G. H., Sharp, S. E., Grasby, M. B., Stott, M., and Dunfield, P. F. (2014). Humboldt’s spa: microbial diversity is controlled by temperature in geothermal environments. ISME J. 8, 1166–1174. doi: 10.1038/ismej.2013.237
Skirnisdottir, S., Hreggvidsson, S., Hjorleifsdottir, V. T., Marteinsson, S. K., Petursdottir, O., Holst, P., et al. (2000). Influence of sulfide and temperature on species composition and community structure of hot spring microbial mats. Appl. Environ. Microbiol. 66, 2835–2841. doi: 10.1128/aem.66.7.2835-2841.2000
Spear, J. R., Walker, T. M., McCollom, R., and Pace, N. R. (2005). Hydrogen and bioenergetics in the Yellowstone geothermal ecosystem. Proc. Natl. Acad. Sci. U.S.A. 102, 2555–2560. doi: 10.1073/pnas.0409574102
Stetter, K. O. (1999). Extremophiles and their adaptation to hot environments. FEBS Lett. 452, 22–25. doi: 10.1016/s0014-5793(99)00663-8
Stetter, K. O. (2006). Hyperthermophiles in the history of life. Philos. Trans. R. Soc. Lond. B Biol. Sci. 361, 1837–1842.
Takami, H., Arai, K., Takemoto, I., Uchiyama, Y., and Taniguchi, T. (2015). Functional classification of uncultured candidatus caldiarchaeum subterraneum using the maple system. PLoS One 10:e0132994. doi: 10.1371/journal.pone.0132994
Tang, J., Liang, D., Jiang, L., Li, Y., Luo, M. M. R., and Daroch, M. (2018). Temperature-controlled thermophilic bacterial communities in hot springs of western Sichuan, China. BMC Microbiol. 18:134. doi: 10.1186/s12866-018-1271-z
Thiel, V., Wood, M. T., Olsen, M., Tank, C. G., Klatt, D. M., Ward, D. A., et al. (2016). The dark side of the mushroom spring microbial mat: life in the shadow of chlorophototrophs. I. microbial diversity based on 16S rRNA gene amplicons and metagenomic sequencing. Front Microbiol 7:919. doi: 10.3389/fmicb.2016.00919
Thompson, J. S., and DeMonge, J. M. (1996). Chemical Analyses of Hot Springs, Pools, and Geysers from Yellowstone National Park, Wyoming and Vicinity, 1980-1993. Denver: Denver Federal Center.
Wang, S., Hou, H., Dong, H., Jiang, L., Huang, G., Wu, C., et al. (2013). Control of temperature on microbial community structure in hot springs of the Tibetan Plateau. PLoS One 8:e62901. doi: 10.1371/journal.pone.0062901
Ward, D. M., and Cohan, F. M. (2005). “Microbial diversity in hot spring cyanobacterial mats: patterns and prediction,” in Geothermal Biology and Geochemistry in Yellowstone National Park, eds W. Inskeep and J. E. McDermott (Bozeman, MT: Thermal Biology Institute), 186–203.
Whitaker, R. J., Grogan, D. W., and Taylor, J. W. (2003). Geographic barriers isolate endemic populations of hyperthermophilic archaea. Science 301, 976–978. doi: 10.1126/science.1086909
Keywords: hot springs, thermophiles, microbial ecology, rRNA amplicons, biogeography
Citation: Podar PT, Yang Z, Björnsdóttir SH and Podar M (2020) Comparative Analysis of Microbial Diversity Across Temperature Gradients in Hot Springs From Yellowstone and Iceland. Front. Microbiol. 11:1625. doi: 10.3389/fmicb.2020.01625
Received: 09 February 2020; Accepted: 22 June 2020;
Published: 14 July 2020.
Edited by:
Peter Dunfield, University of Calgary, CanadaReviewed by:
Jeremy Dodsworth, California State University, San Bernardino, United StatesAllyson Lee Brady, McMaster University, Canada
Copyright © 2020 Podar, Yang, Björnsdóttir and Podar. This is an open-access article distributed under the terms of the Creative Commons Attribution License (CC BY). The use, distribution or reproduction in other forums is permitted, provided the original author(s) and the copyright owner(s) are credited and that the original publication in this journal is cited, in accordance with accepted academic practice. No use, distribution or reproduction is permitted which does not comply with these terms.
*Correspondence: Mircea Podar, cG9kYXJtQG9ybmwuZ292
†ORCID: Mircea Podar, orcid.org/0000-0003-2776-0205