- Institute for Water Research and Department of Microbiology, University of Granada, Granada, Spain
Desiccation-tolerant plants are able to survive for extended periods of time in the absence of water. The molecular understanding of the mechanisms used by these plants to resist droughts can be of great value for improving drought tolerance in crops. This understanding is especially relevant in an environment that tends to increase the number and intensity of droughts. The combination of certain microorganisms with drought-sensitive plants can improve their tolerance to water scarcity. One of these bacteria is Microbacterium sp. 3J1, an actinobacteria able to protect pepper plants from drought. In this study, we supplemented drought-tolerant and drought-sensitive plant rhizospheres with Microbacterium sp. 3J1 and analyzed their proteomes under drought to investigate the plant-microbe interaction. We also compare this root proteome with the proteome found in desiccation-tolerant plants. In addition, we studied the proteome of Microbacterium sp. 3J1 subjected to drought to analyze its contribution to the plant-microbe interaction. We describe those mechanisms shared by desiccation-tolerant plants and sensitive plants protected by microorganisms focusing on protection against oxidative stress, and production of compatible solutes, plant hormones, and other more specific proteins.
Introduction
Water scarcity is one of the most important limiting factors in agricultural production (Niles et al., 2015). Only a significant increase in food production can counterbalance the exponential growth of the population to supply enough food in the near future (Sapkota, 2019). To achieve this increase in food we have to ensure that crops are not lost due to more frequent droughts. Furthermore, we can recover previously abandoned farmland due to lack of water resources. We can use different approaches to grow plants for food purposes in these environments suffering from water limitation. One of these approaches consists of imitating the strategies followed by plants that naturally tolerate the lack of water, such as desiccation-tolerant plants and genetically modify the desiccation-sensitive plants (Lamaoui et al., 2018). Another approach that have arised recently is the use of desiccation-tolerant microorganisms with the capacity to protect plants that would otherwise be sensitive to drought (Nadeem et al., 2014; Enebe and Babalola, 2018).
Our group have identified a collection of desiccation-tolerant microorganisms, among them several actinobacteria stand out for their ability to tolerate desiccation due to the production of protective molecules called xeroprotectants (Narváez-Reinaldo et al., 2010; Julca et al., 2012; Santacruz-Calvo et al., 2013). In addition, some of these microorganisms have the ability to colonize plant roots and protect these plants, such as pepper and tomato, against drought (Manzanera et al., 2015a, b; Vílchez et al., 2016). A correlation between microorganisms with the largest capacity to protect plants from drought and their production of trehalose was found. Microbacterium sp. 3J1 was the microorganism with the highest production of trehalose and the one showing the highest protection of plants (Vílchez et al., 2016). By studying the metabolome of the interaction between the pepper plant root and Microbacterium sp. 3J1 under drought conditions, we have identified a change in the content of glutamine and α-ketoglutarate that resulted in the alteration of the C and N metabolism. As a result of this alteration, the concentration of sugars and amino acids was also modified due to the presence of the bacteria. In addition, antioxidant molecules, metabolites involved in the production of plant hormones such as ethylene, and substrates used for lignin production were altered in response to the presence of Microbacterium sp. 3J1 under drying conditions (Vílchez et al., 2018).
Similarly to desiccation-tolerant microorganisms there are some plants, such as Xerophyta viscosa, Selaginella tamariscina, Craterostigma plantagineum, and Boea hygrometrica, able to withstand desiccation by arresting their metabolism during drying conditions and resuming such metabolism once water becomes available again (Challabathula and Bartels, 2013). This process is termed anhydrobiosis and the plants are known as desiccation-tolerant plants or resurrection plants. To survive desiccation, these plants modulate the expression of a set of proteins involved in the decrease of photosynthesis, sugars accumulation, production of antioxidant molecules, or in the increase of the flexibility of cell walls and membranes (Challabathula and Bartels, 2013; Ambastha and Tiwari, 2015).
The study of proteomes is a versatile tool to understand the physiological processes involved in the tolerance of plants to absence of water. The study of proteomics instead of transcriptomics allows us to take into consideration transcript instability, post- transcriptional modifications and translational regulation of mRNA affecting gene expression. Changes in the quality and quantity of expressed proteins can be found with proteomic studies. To our knowledge, this is the first time the proteome of plants (Caspicum annuum) protected by a microorganism (Microbacterium sp. 3J1) under drought conditions is described and compared with the proteome of naturally desiccation-tolerant plants. The comparison of both types of proteomes may form the basis for the development of alternative strategies to protect desiccation-sensitive food plants frequently affected by droughts.
Results and Discussion
Experimental Set-Up
We identified the interaction between Microbacterium sp. 3J1 and pepper roots for the protection of the plant against drought. Therefore, we decided to focus on the analysis of C. annuum in the presence and absence of the microorganism, in both cases under drought conditions. In addition, a comparison of the proteome profile of the 3J1 strain between drought and non-stressing conditions was performed to find out a potential contribution of Microbacterium sp. 3J1 to the pepper plant.
We decided to sample root material from 28-day old plants previously exposed to drought for 14-days to compare the proteome resulting from different microbiome compositions. Microbacterium sp. 3J1 was added to the plant 14 days after germination (Figure 1A). Then we subjected this material to the mapping of the roots proteome. In addition, the relative water content (RWC) of the plants was recorded, and total soluble protein was extracted and analyzed by two-dimensional polyacrylamide gel electrophoresis (2D SDS–PAGE or 2-DE).
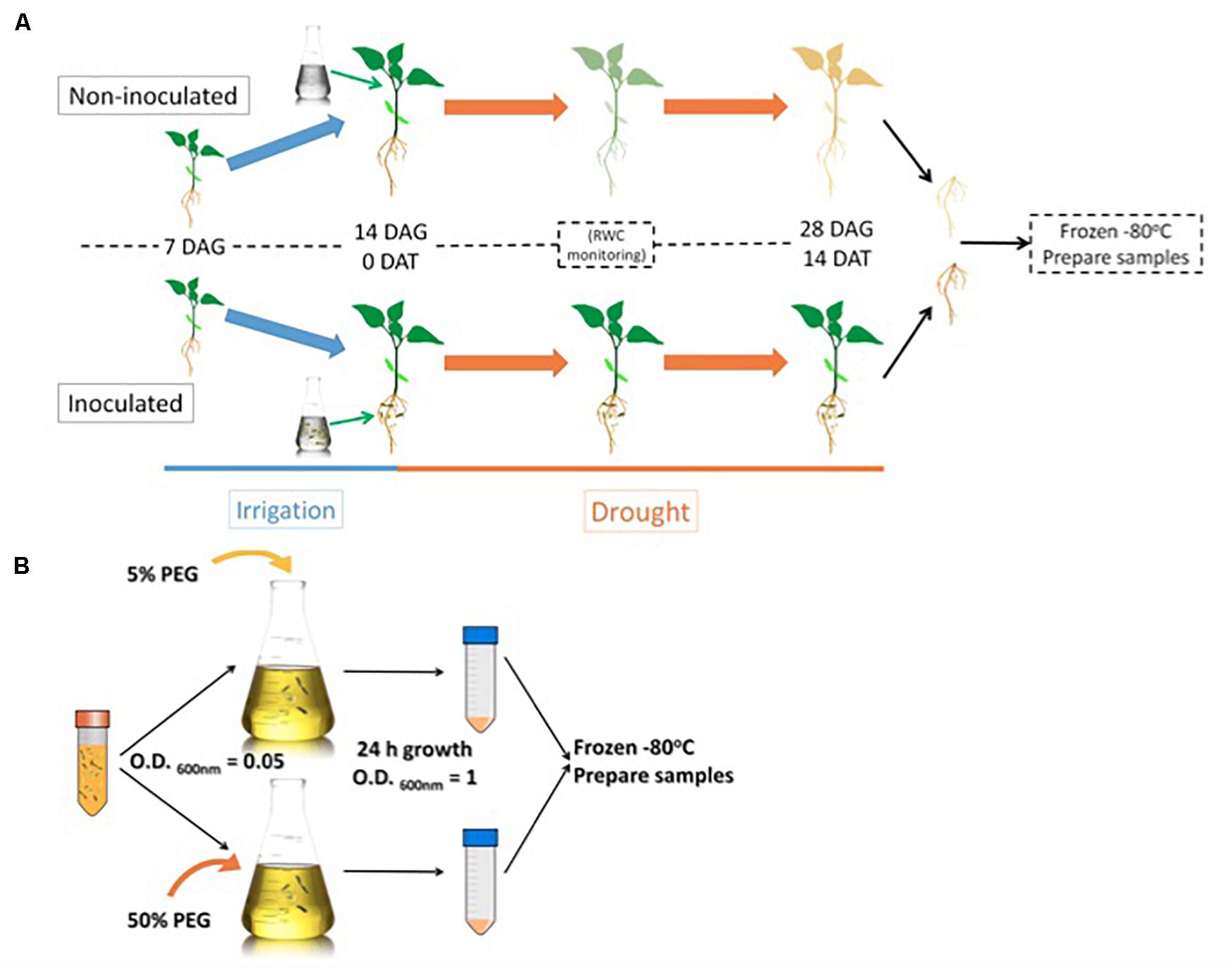
Figure 1. Root sample preparation workflow (A) and Microbacterium sp. 3J1 sample preparation workflow used in the proteomic study (B). DAG: days after germination; DAT: days after treatment. Microbacterium sp. 3J1-inoculated and non-inoculated pepper plant roots were sampled and preserved 14 DAT under drought conditions. Tests were performed with at least eight plants in triplicate. After freezing the samples, workflow continues with protein isolation and 2D-PAGE. Microbacterium sp. 3J1 cultures were grown until OD600 nm of approximately 1 in TSB supplemented with 5 or 50% PEG. Then samples from three different cultures were centrifuged and frozen until use. Protein isolation and 2D-PAGE (in triplicate) were performed using the frozen samples.
For the Microbacterium sp. 3J1 proteome, TSA cultures of the microorganism were supplemented with 5% and 50% polyethylene glycol (PEG), as indicated in the Materials and Methods section, to simulate the water activity of a non-stressed soil and of a soil subjected to drought-stress, respectively, (Figure 1B).
Analysis of Differential Proteins in Microbacterium sp. 3J1-Inoculated and Non-inoculated C. annuum Roots Under Drought
After 14 days in the absence of watering, the RWC of non-inoculated plants was 0.4, while the RWC for inoculated plants was 0.68. These results show that plants were protected by the presence of Microbacterium sp. 3J1 (Figure 2). Inoculated and non-inoculated plants were analyzed by 2-DE to understand the proteome response of pepper plants subjected to drought to the presence of Microbacterium sp. 3J1. The root protein maps produced from three independent protein extractions showed a high reproducibility based on the analysis using the PDQuest software.
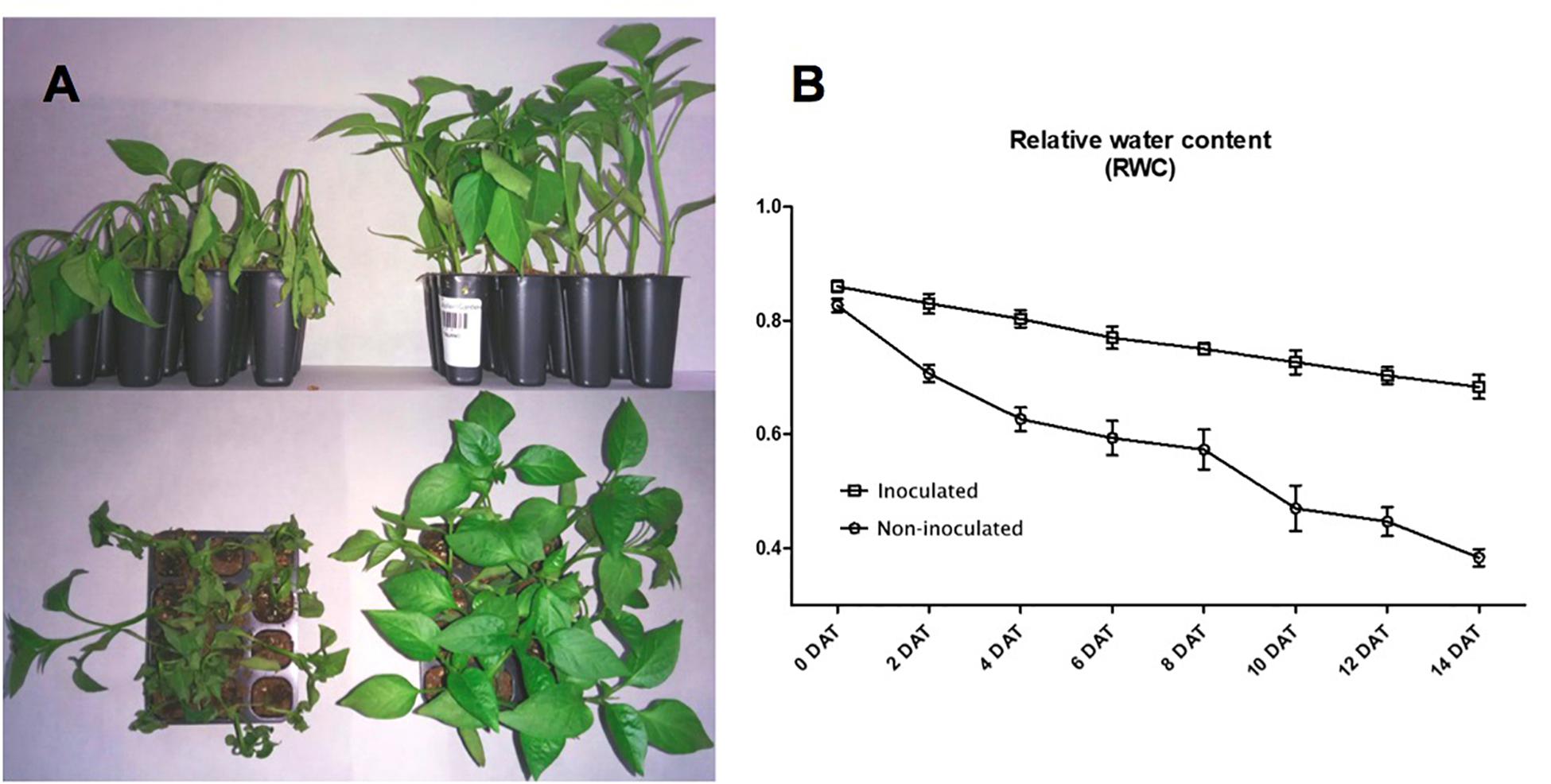
Figure 2. Microbacterium sp. 3J1-inoculated and non-inoculated C. annuum used in this study. (A) Phenotypes showed at 28 days after germination (DAG) of seedlings, at 14 days after treatment (DAT) with Microbacterium sp. 3J1 (left) and in absence of the microorganism as a non-inoculated condition (right), both in the absence of watering. (B) Drought critical point after treatment was determined by tracking the relative water content (RWC). Tests were performed with at least eight plants in triplicate. Population standard deviation (PSD) was used to determine the inner error bars. Statistic differences were found from 2 DAT. For statistic analysis ANOVA with post hoc Tukey’s test (P ≤ 0.05) was used for comparison of datasets obtained at each sampling time.
Figure 3 shows representative gels of proteins extracted from the non-inoculated and inoculated plants. In conjunction, a total of 749 protein spots were reproducibly detected using PDQuest software from the non-inoculated samples and from the inoculated samples (n = 3). From a spot-to-spot comparison and based on statistical analysis, a total of 66 spots exhibited at least 2-fold (p < 0.05) difference in abundance between the non-inoculated and the inoculated plants (Figure 3A). Among 66 differential proteins, 30 spots showed qualitative changes (3 qualitative spots corresponded to inoculated-roots, whereas 27 spots were identified in non-inoculated roots. The rest 36 spots were present in both conditions, showing quantitative changes. A total of 27 spots out of these 36 differentially expressed spots were identified by MALDI TOF/TOF (Figure 3B and Table 1). Therefore despite the time consuming and laborious involved in 2D-PAGE proteomics compared to shotgun proteomics we decided to use 2D-PAGE proteomics for a better comparison with most of the proteomic studies reported on desiccation-tolerant plants.
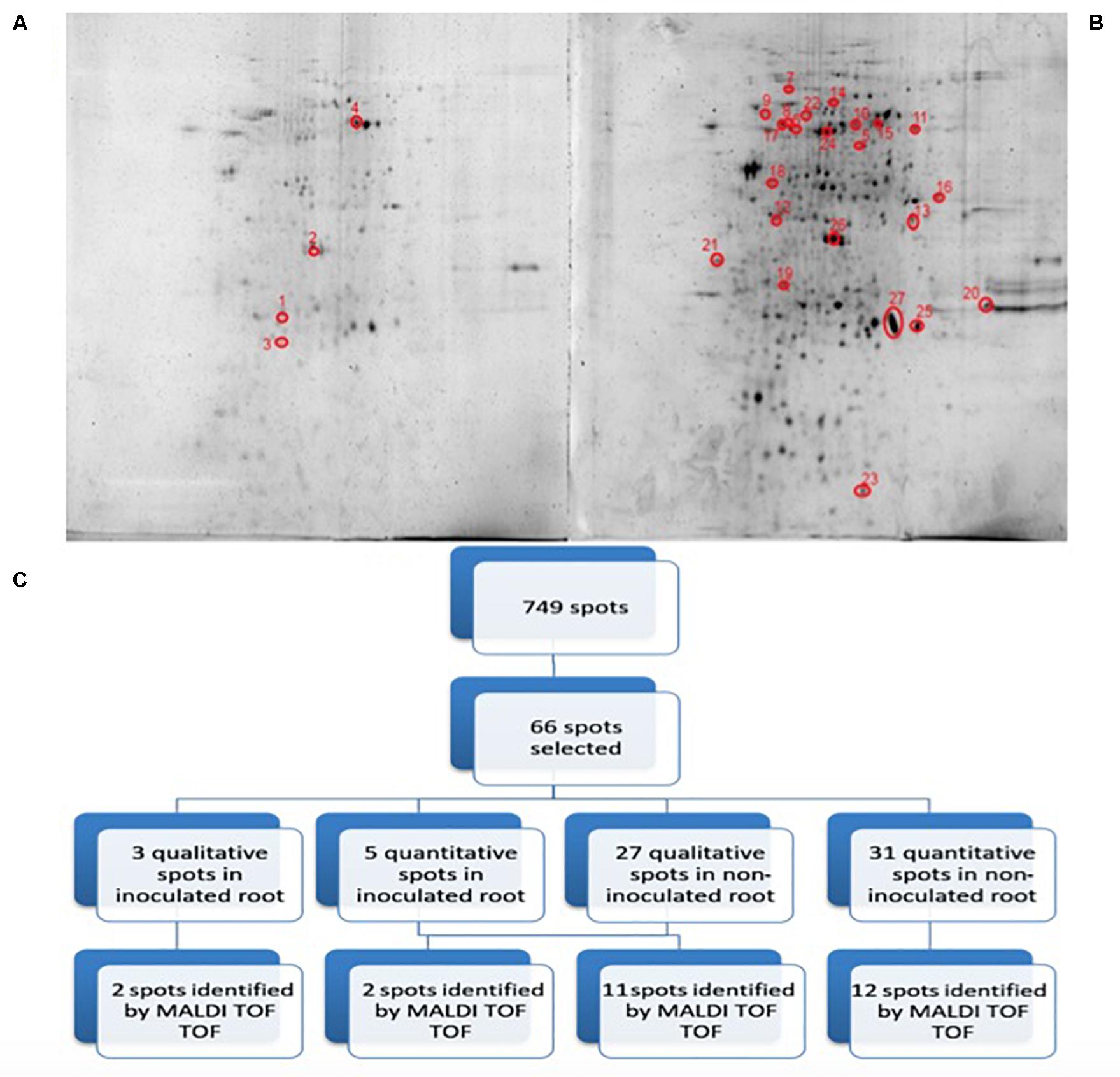
Figure 3. 2D-PAGE image analysis of non-inoculated and inoculated plants subjected to drought. Upper pictures show differences in pepper root proteomes of non-inoculated (A) and inoculated with Microbacterium sp. 3J1 (B) seedlings obtained under drought conditions. Three biological replicates (three different plants) were used. Lower diagram shows the spot selection procedure and the final identified ones (C). Spots detection and selection were performed with PDQuest software v8.0. Red circles and numbers correspond to selected protein spots that were finally identified by MALDI TOF/TOF and described in Table 1. Pictures were selected as the most representatives from at least three 2D-PAGE replicates performed for each condition.
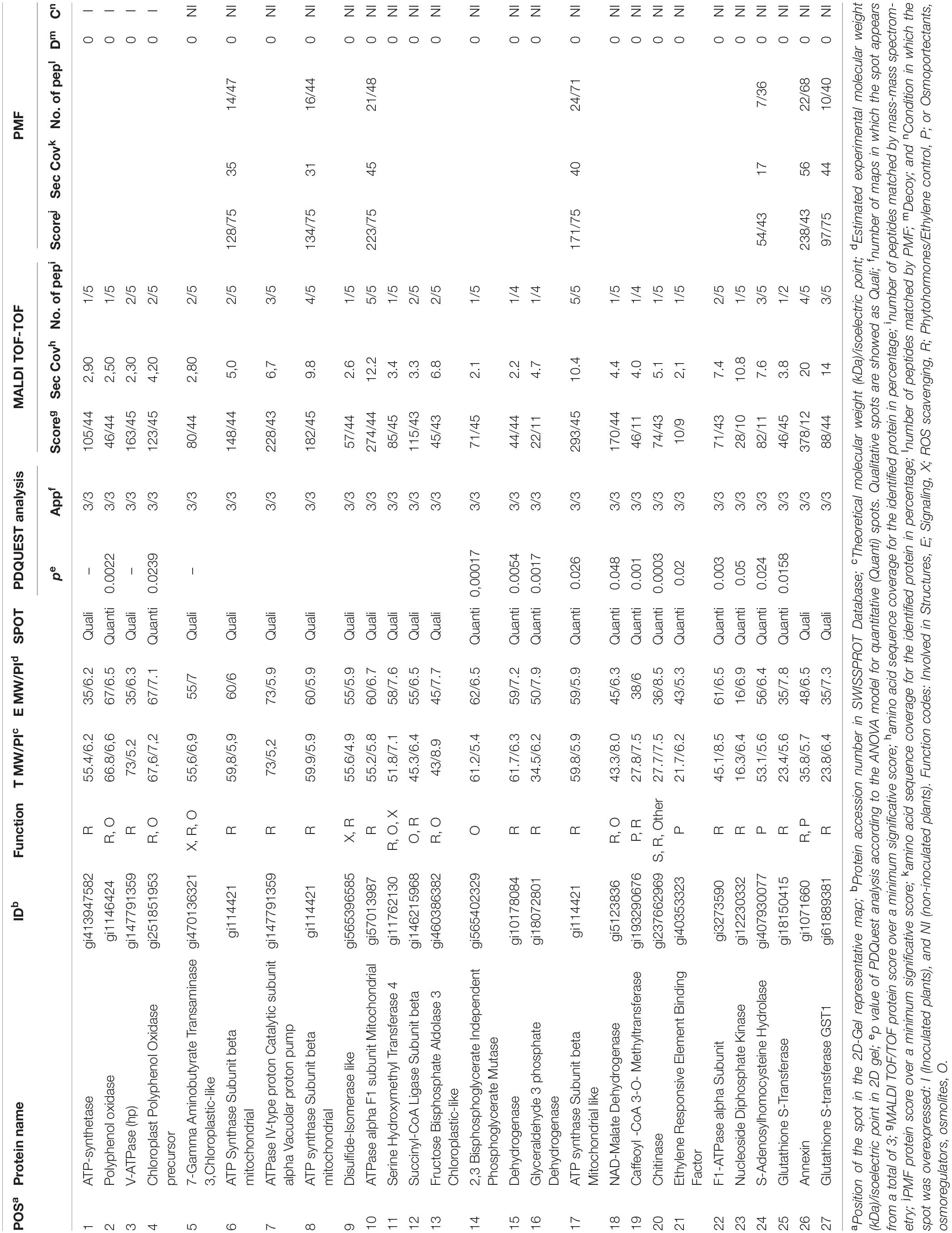
Table 1. List of proteins from the proteome analysis showing significantly different amounts between inoculated and non-inoculated plants.
The number of protein spots detected for the Microbacterium sp. 3J1 protection of C. annuum against drought was within the range of protein spots described in previous studies on desiccation-tolerant plants. These plants included Haberlea rhodopensis, which showed a similar number of protein spots (152 vs. 148) when comparing hydrated control plants with those dehydrated (with 16% water content), showing 33 proteins specific for the desiccated H. rhodopensis plants only (Mladenov et al., 2015). For X. viscosa, 428 (±52) protein spots were identified using also the same software, although a lower difference in water content, changing from 65% to 35% RWC, was tested (Ingle et al., 2007). In the case of B. hygrometrica, the change in RWC from 100% to 2.4% was more drastic and a total of 223 protein spots were reproducibly detected (Jiang et al., 2007).
Proteins Identified Using MALDI-TOF/TOF-MS
To understand the function of differentially expressed proteins in C. annuum roots exposed to drought in the presence of Microbacterium sp. 3J1, the 66 differentially expressed spot proteins were analyzed by gel excision followed by a trypsin digestion and MALDI TOF/TOF-MS analysis in order to obtain the peptide mass fingerprint (PMF) data. Only 27 out of the 66 analyzed proteins could be identified (Table 1). The reason for the low number of reported identified proteins is based on the combination of two facts. The first one is that there are only 1099 annotated proteins for Viridiplantae and C. annum in the NCBI database and 62 in SwissProt. Therefore it might be possible that some of the selected proteins are not reported in the databases and therefore are considered as non-identified. In addition we wanted to show only data corresponding to high confidence, therefore only proteins with high enough coverage, and some of the non-identified proteins corresponded to low coverage results. The low coverage of some of the non-identified proteins could be most likely due to difficulties associated with separating and detecting larger proteins or due to the co-migration of proteins to the same spot with proteins of similar isoelectric points and denatured molecular weight becoming focused at the same position of the gel.
These proteins were grouped in osmoprotectant producing proteins, reactive oxygen species (ROS) scavengers, plant-hormones, structural proteins, or signaling proteins. Similarly to the proteome of desiccation-tolerant plants exposed to dehydration, C. annum exposed to drought showed a diverse nature of differential proteins in the presence of Microbacterium sp. 3J1 compared to the non-inoculated plant. We consider this result to be indicative of the large impact of Microbacterium sp. 3J1 on plant cell function during dehydration. This is consistent with the diverse nature of differential proteins observed for desiccation-tolerant plants during dehydration processes.
Osmoprotectans
As a result of the proteome analysis, we have identified the decrease in fructose bisphosphate aldolase and NAD malate dehydrogenase in C. annuum plants exposed to drought in the presence of Microbacterium sp. 3J1 (spots 13 and 18, respectively) compared to non-inoculated plants. Theses enzymes are involved in the production of glyceraldehyde 3-phosphate and oxaloacetate, respectively. Fructose-1,6-bisphosphate aldolase is a key metabolic enzyme that reversibly catalyzes the aldol cleavage of fructose-1,6-bisphosphate into dihydroxyacetone phosphate and glyceraldehyde-3-phosphate, either during the glycolytic pathway, or gluconeogenesis and during the Calvin cycle (Berg et al., 2010). These reactions are involved in carbon fixation and sucrose metabolism and they are present in the chloroplast stroma and in the cytosol of green plants, allowing the plant to fix carbon dioxide into glyceraldehyde-3-phosphate, which can then be incorporated into other sugars (Anderson et al., 2005; Lv et al., 2017). The fact that the pepper plant produces increased amounts of these two enzymes points to the requirement of sucrose in the absence of Microbacterium sp. 3J1, whereas such requirement is no longer needed in the presence of this bacterial strain. We observed the accumulation of fructose (more than 8-fold), fructose-6-phosphate (approximately 18-fold increase), and trehalose (approximately 85-fold increase) in a recent metabolomic characterization of the pepper plant subjected to drought in the presence of Microbacterium sp. 3J1, compared to the non-inoculated plant. This result points to an alternative use of sugars as more efficient osmoprotectant (Vílchez et al., 2018). The concentration of other sugars was also increased in response to the microorganism when pepper plants were subjected to drought. Similarly, one of the mechanisms that desiccation-tolerant plants use to respond to drought consists of the accumulation of osmoprotectants, osmoregulators, and osmolyte substances. For instance X. viscosa, C. plantagineum, Sporobolus stapfianus, and other resurrection plants modulate their carbohydrate metabolism for the production of sucrose (Bianchi et al., 1991; Whittaker et al., 2001; Ingle et al., 2007; Yobi et al., 2013). However, Selaginella lepidophylla accumulates oxaloacetate, fumarate, succinate, and alpha-ketoglutarate (intermediates of the tricarboxylic acid cycle) during dehydration, therefore the importance of these metabolites for the metabolic flux through these pathways during dehydration or in preparation for rehydration is suggested (Yobi et al., 2013). Nevertheless, trehalose is a minor component in a few desiccation-tolerant angiosperm species despite its importance in desiccation tolerance among lower organisms (Ghasempour et al., 1998b). Despite the differences in the profile of osmoprotectants produced by desiccation-tolerant plants and by Microbacterium sp. 3J1-inoculated plants, Ingle et al. have described the increase in the abundance of proteins (phosphopyruvate hydratase) involved in the control of glycolysis-gluconeogenesis pathways in the desiccation-tolerant plant X. viscosa (Ingle et al., 2007). Oliver et al. also described the increase in enzymes associated with glycolysis including aldolases, phosphoglycerate kinase, and glyceraldehyde-3-phosphate dehydrogenase in S. stapfianus subjected to drying conditions (Oliver et al., 2011). All the increased proteins: phosphopyruvate hydratase (in X. vicosa), phosphoglycerate kinase and glyceraldehyde-3-phosphate dehydrogenase (in S. stapfianus), and fructose biphosphate aldolase and NAD malate dehydrogenase (in C. annuum plants exposed to drought in absence of Microbacterium sp. 3J1) would allow an increase in flux through the gluconeogenic route during drought, providing an increased pool of hexose phosphate substrates to deal with the higher demand of sucrose in both types of plants (natural desiccation-tolerant plants and 3J1-protected pepper plants) under drying conditions.
Energy Metabolism
The addition of Microbacterium sp. 3J1 to pepper plants subjected to drought resulted in the production of two proteins (spots 1, 3) not found in non-inoculated plants, the disappearance of three proteins (spots 6, 7, and 10) and the decreased in the amount of two proteins (spots 17, 22) related to the production of ATP. This change in the expression pattern of proteins involved in the production of ATP may produce a different cell concentration of ATP. The availability of ATP during drought is critical because this metabolite is needed for the production of osmoprotectants. The increased amount of certain proteins involved in the production of ATP substituting other ATP producing proteins has been reported for several resurrection plants such as B. hygrometrica (Jiang et al., 2007), S. stapfianus (Oliver et al., 2011), X. viscosa (Ingle et al., 2007), Tortula ruralis (Oliver et al., 2004), and for S. tamariscina (Wang et al., 2010), where the increased production of ATPases was suggested to play a role in protein folding or unfolding and protein degradation in response to drought stress.
Despite previous studies have reported that phosphorylation of high mobility group proteins reduced their binding to DNA, inhibiting replication and transcription in drought-sensitive plants such as maize (Zhao et al., 2009), we have described the potential role of DNA as xeroprotectant, i.e., as dehydration protectant (García-Fontana et al., 2016). Therefore we do not rule out a potential role of the over production of ATP in DNA production for DNA repair and, we do not discard that ATP can function as xeroprotectant by the production of DNA in the plant. The increase in proteins involved in DNA related processes have been reported for some desiccation-tolerant plants, such as S. stapfianus (Oliver et al., 2011). This increase suggests a shared mechanism between desiccation-tolerant plants and C. annuum protected by Microbacterium sp. 3J1.
Oxidative Metabolism
The proteome analysis of Microbacterium sp. 3J1-inoculated C. annuum plants subjected to drought is marked by the decrease in abundance of proteins associated with oxidative metabolism, and more specifically, with enzymes involved in the protection against ROS, pointing to a protection against ROS by alternative methods. ROS causes deleterious effects on the respiratory system, metabolic pathways, genomic stability, membranes, and organelles during drought events. The increased production of ATP occurs in conjunction with the protection against ROS, and in this sense, we have found increased production of certain ATP producing enzymes as above described. Glutathione protects against oxidative damage by quenching activated oxygen species and participates in the metabolism of hydrogen peroxide (Neubauer and Yamamoto, 1994). In addition to those proteins, we have identified an increased production of proteins involved in glutathione production such as glutathione-S-transferase and glutathione S-transferase GST1 (spots 25, 27). In the absence of Microbacterim sp. 3J1, the plant produces higher quantities of ROS scavengers, showing a special need to fight ROS, something that does not seem to be required in the presence of the 3J1 strain. The production of ROS scavengers is a common feature among desiccation-tolerant plants subjected to drying conditions (Kranner and Birtic, 2005; França et al., 2007; Oliver et al., 2011; Challabathula et al., 2018; Bentley et al., 2019). Jiang and coworkers identified increased production of glutathione S-transferase in response to dehydration in B. hygrometrica, in special during the early stages of dehydration using proteome assays (Jiang et al., 2007). The participation of glutathione to protect desiccation-tolerant plants has been described in many species, including H. rhodopensis (Georgieva et al., 2017), B. hygrometrica (Jiang et al., 2007), and S. stapfianus (Oliver et al., 2011), to name a few. Apart from glutathione-producing enzymes, other proteins such as ascorbate peroxidase (ascorbate acts as a scavenger of hydrogen peroxide), or superoxide dismutase have been reported as proteins involved in oxidative mechanisms in several desiccation-tolerant plants, such as X. viscosa (Sherwin and Farrant, 1998; Farrant, 2000). However, we have only found a decrease in the proteins needed for the production of glutathione for this type of antioxidant proteins in response to the presence of Microbacterim sp. 3J1.
Cell Signaling
An appropriate signaling system and the production of regulatory molecules, such as plant hormones, are needed for a coordinated response between the microorganism and the plant for its protection against drought. Therefore, we would expect the detection of proteins involved in the production of such molecules. A decrease in four proteins involved in the production of plant hormones was found in Microbacterium sp. 3J1-inoculated plants. These four proteins are caffeoyl-CoA 3-O-methyltransferase, ethylene responsive element binding factor, S-Adenosyl homocysteine hydrolase, and annexin (spots 19, 21, 24, and 26, respectively). Caffeoyl-CoA 3-O-methyltransferase (spot 19), catalyzes the conversion of S-adenosyl-L-methionine (SAM), and caffeoyl-CoA into S-adenosyl-L-homocysteine (SAH), and feruloyl-CoA, thus reducing the concentration of SAM. The production of ethylene in plants is mainly based in the presence of SAM, and therefore a decrease in caffeoyl-CoA 3-O-methyltransferase would translate into a reduction of ethylene and a concomitant release from senescence of the plant due to lack of water. The S-adenosyl homocysteine hydrolase (spot 24) is therefore involved in the reversible production of S-adenosyl homocysteine, contributing to the reduction of SAM and, consequently, reducing the ethylene production. The production of both enzymes has been described to increase in the drought-tolerant plant C. dactylon (Bohler et al., 2013). The SAM to SAH ratio is involved in the enhancement of drought tolerance where methylation is a key step (Espartero et al., 1994). In addition, we have previously described a reduction in the production of ethylene in pepper plants subjected to drought when inoculated with Microbacterium sp. 3J1 (Vílchez et al., 2018). This is of special relevance since we have observed the decrease in the ethylene responsive element-binding factor (spot 21) in pepper plants subjected to drought when Microbacterium sp. 3J1 was present. In resurrection plants, such as X. viscosa, it has been suggested that ethylene could be a signal for their tolerance to drought through the expression of XVT8 (a gene encoding a glycine-rich protein, with significant identity to dehydrins; Ndima et al., 2001). However, the abscisic acid (ABA) is a more common plant hormone for the response to drought in X. viscosa (Ingle et al., 2007). ABA controls the stomata closure and the accumulation of metabolites, increases the activity of antioxidant enzymes and the expression of genes encoding protective proteins in response to dehydration and it has been described as a major component in the hormonal control of the acquisition of desiccation tolerance in S. stapfianus (Gaff et al., 2009), and in other resurrection plants (Oliver et al., 2010; Oliver et al., 2011). The existence of a non-ABA signaling pathway has been proposed for S. stapfianus, where brassinosteroids, and methyl jasmonate could play a role (Ghasempour et al., 1998a, 2001).
Polyphenol Metabolism
We found an increase in the production of polyphenol oxidase (PPO) and the chloroplastid precursor of PPO (spots 2 and 4, respectively) in plants subjected to drought in the presence of Microbacterium sp. 3J1. An increase in PPO has been described to prevent of proteolytic activity for the conservation of the proteins during the desiccation state in Ramonda servica and B. hygrometrica (Veljovic-Jovanovic et al., 2006; Jiang et al., 2007). The PPO has also been described as ROS scavenger in H. rhodopensis, M. flabellifolia, and Ramonda serbica (Moore et al., 2005; Georgieva et al., 2007; Veljovic-Jovanovic et al., 2008). A potential role of PPO in Mehler-like reaction for detoxification of ROS has been suggested because most PPOs are localized in the chloroplast thylakoid lumen; in addition, the chemical structure of PPOs makes them ideal for free radical scavenging activities in the chloroplast (Sherman et al., 1995; Rice-Evans et al., 1997). Therefore, we suggest that the presence of Microbacterium sp. 3J1 in pepper plants subjected to drought use PPO instead of glutathione to prevent the damage produced by ROS (Figure 4).
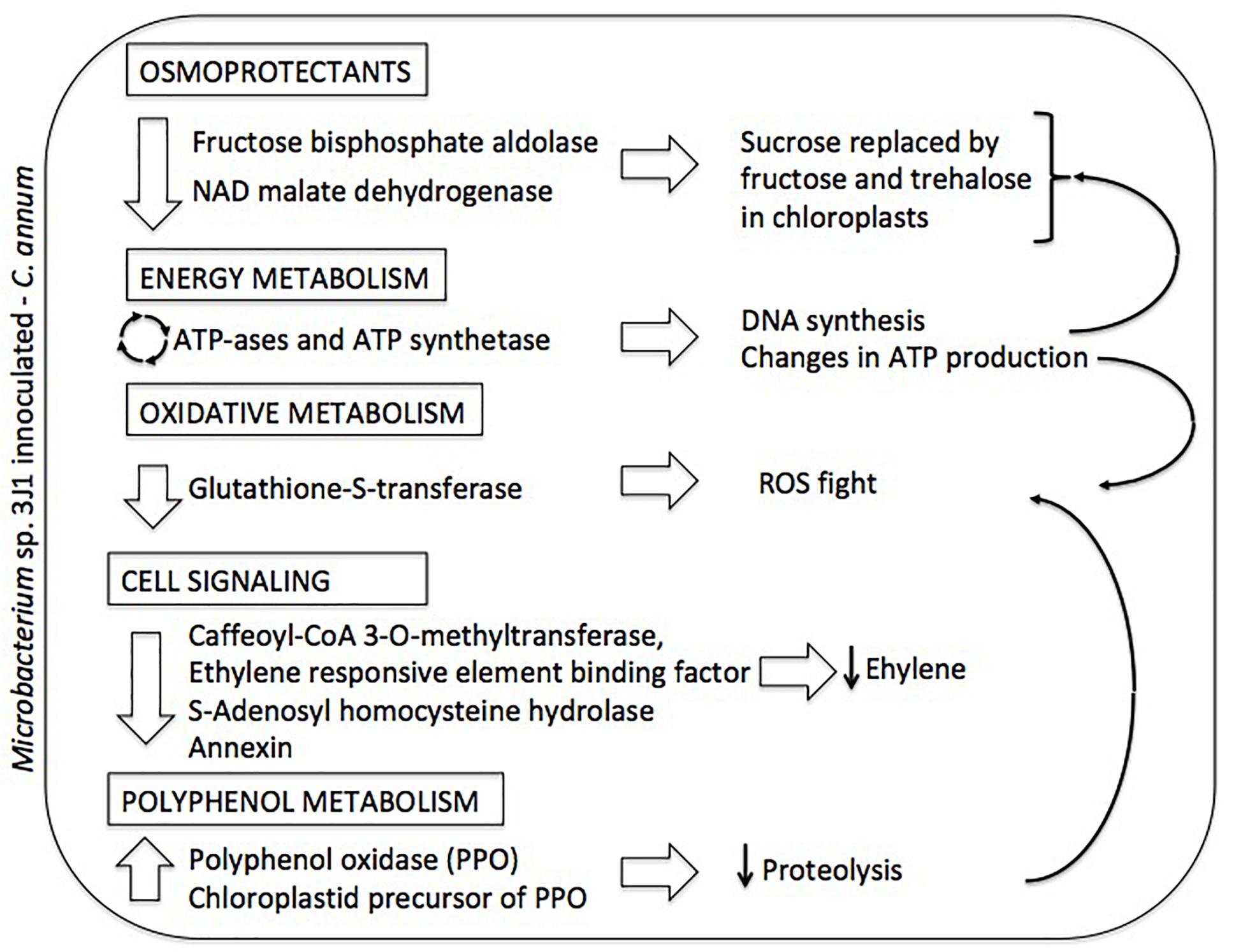
Figure 4. Summary diagram depicting the differential proteome of Microbacterium sp. 3J1-inoculated C. annuum leaves in response to dehydration. On the left column altered proteins and metabolic roles (in boxed) are described. On the right column proposed physiological responses and their interconnections (thin arrows) are depicted.
Microbacterium sp. 3J1 Proteome in Response to Drought
In order to assess the potential impact of the proteins produced by Microbacterium sp. 3J1 on the pepper plant during drought, we characterized the differential protein expression of this soil microorganism in response to severe drought by addition of PEG. The addition of PEG results in the sequestration of water molecules reducing the hydric potential of the media. To simulate the change from a non-stressed soil to a severe drought-stressed soil, liquid cultures of Microbacterium sp. 3J1 were supplemented with 5 and 50% (w/v) PEG 8000, respectively. The 2-DE was performed following the previously described conditions. A fold-change of at least 2 was considered as the minimum level of differentially expressed proteins when analyzing the 2-DE images. A total of 237 protein spots were analyzed as statistically significant (p-value < 0.05) and 43 protein spots were selected and analyzed by MALDI-TOF/TOF-MS- (Figure 5). A total of 40 quantitative (19 up- and 21 down-regulated) proteins and 3 qualitative spots were differentially expressed proteins between 5 and 50% PEG conditions. Among these 43 analyzed spots, 13 were successfully identified (Table 2). Similarly to the C. annuum’s proteome, Microbacterium’s proteome is poorly described and therefore it might be possible that some of the selected proteins are not reported in the databases and again are considered as non-identified. Also some of the non-identified proteins corresponded to low coverage results.
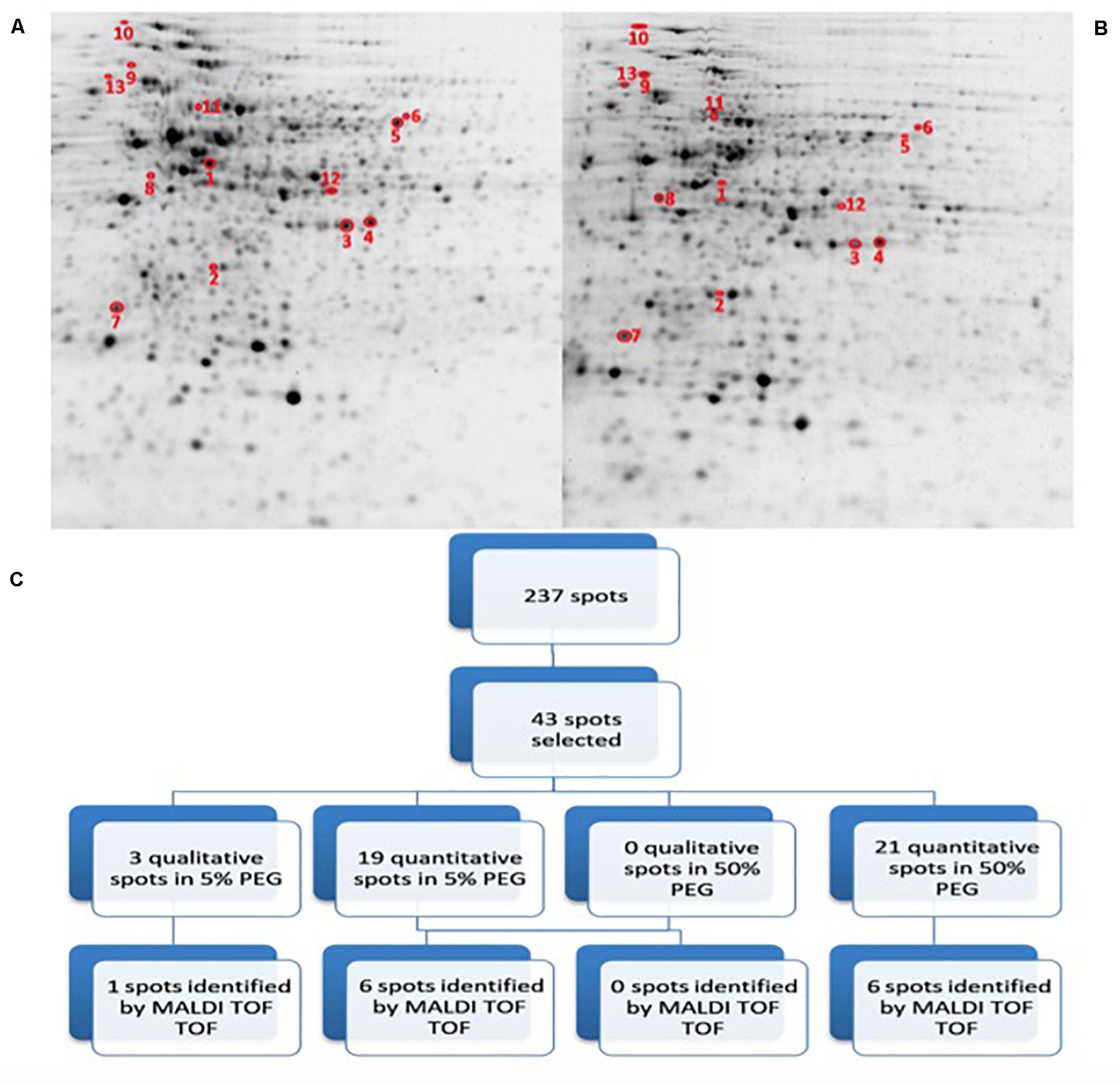
Figure 5. 2D-PAGE image analysis of drought-subjected Microbacterium sp. 3J1 cultures. Upper pictures show differences in Microbacterium sp. 3J1 proteome when 5% (A) or 50% PEG (B) were supplied to TSB growth medium Three biological replicates (three different cultures) were used. Lower diagram shows the spot selection procedure and the final identified ones (C). Spots detection and selection were performed with PDQuest software v8.0. Red circles and numbers correspond to selected protein spots that were finally identified by MALDI TOF/TOF and described in Table 2. Pictures were selected as the most representatives from at least three 2D-PAGE replicates performed for each condition.
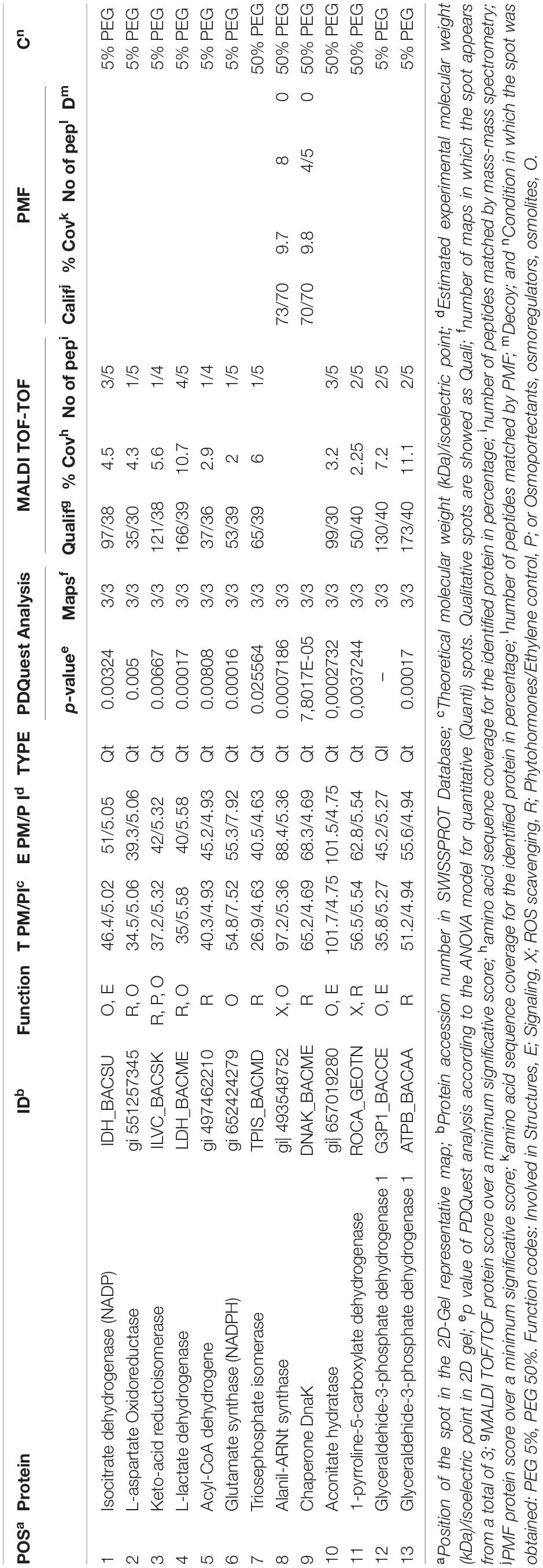
Table 2. List of proteins from the Microbacterium sp. 3J1 proteome analysis showing significantly different amounts unde hydric stress (50% PEG) and non-stressed conditions (5% PEG).
All differentially identified proteins were analyzed via the NCBI BLAST database and the function of these proteins was explained by inputting the Uniprot accession number into the Uniprot database as shown in Table 2 (Boutet et al., 2016; UniProt Consortium, 2019). The identified proteins were mainly involved in (a) osmotic protection, (b) energy metabolism, (c) antioxidant protection, and (d) cell signaling.
Regarding the proteins involved in osmotic protection, 8 proteins were differentially expressed (spots 1–4, 6, 8,10, and 12), including the decreased expression of L-aspartate oxidoreductase in media supplemented with 50% PEG (spot 2) compared to medium with 5% PEG. This enzyme is involved in the conversion of L-aspartate into oxaloacetate, resembling the catalytic step produced by the increased expression of malate dehydrogenase observed in C. annuum plants subjected to drought in the presence of Microbacterium sp. 3J1. In the presence of water stress conditions, we have also found a decreased production of NADPH-isocitrate dehydrogenase (spot 1) and an increased production of aconitase (spot 10), involved in the citric acid cycle, for the reversible catalytic conversion of citrate to isocitrate via cis-aconitate. The decreased production of NADPH-isocitrate dehydrogenase that catalyzes the irreversible conversion of isocitrate into α-ketoglutarate in conjunction with the increased production of aconitase in response to water stress conditions would be translated into a reduction of α-ketoglutarate by the partial inversion of the first steps of the citric acid cycle. The metabolomic analysis of the pepper plant subjected to drought also showed a dramatic decrease in α-ketoglutarate due to the presence of Microbacterium sp. 3J1. This decrease seems to respond to a decreased production of α-ketoglutarate and to the conversion of α-ketoglutarate into glutamine for the drastic change in the C and N metabolism of the plant due to the presence of the microorganism (Vílchez et al., 2018). In the inoculated plant subjected to drought, the concentration of glutamine is increased compared to the non-inoculated plant; however, no alteration in the glutamate was found. This might be explained by the observed decreased production of NADPH-glutamate synthase (spot 6) in Microbacterium sp. 3J1, when exposed to drying conditions (50% PEG). The produced glutamate from glutamine can be transformed into proline, a well described osmoprotectant, by enzymatic and non-enzymatic transformation. The last of the enzymatic steps requires the participation of the 1-pyrroline-5-carboxylate dehydrogenase, one of the increased proteins in Microbacterium sp. 3J1 in response to higher water stress (spot 11). The production of both proline and aspartate contribute to the production of osmoprotectants. Glutamate metabolism for the production of other amino acids such as alanine, valine, and isoleucine, could be reduced also by the observed decreased production of keto-acid reductoisomerase (spot 3) under water stress. An increased production of alanyl-tRNA synthetase (spot 8) was found. This is a key enzyme for the availability of amino acids also overproduced by Microbacterium sp. 3J1 in response to water stress. A disappearance of glyceraldehyde-3-phosphate dehydrogenase (spot 12) was also found in response to water stress in Microbacterium sp. 3J1. This enzyme catalyzes the reversible conversion of glyceraldehyde-3-phosphate into 1,3-bisphosphoglycerate, contributing to the metabolic flux of the glycolysis-gluconeogenesis pathways described in the inoculated peppers in presence of Microbacterium sp. 3J1. This could also be the case of the increased production of triose phosphate isomerase (spot 7) found in Microbacterium sp. 3J1 under water stress, since this enzyme catalyzes the reversible conversion of glyceraldehyde-3-phosphate into dihydroxyacetone phosphate, allowing the availability of free sugars for the production of osmoprotectant, as described for 3J1-inoculated pepper plants under drying conditions.
A decreased production of the L-lactate dehydrogenase (spot 4) was observed in response to water stress. This protein reversibly catalyzes the inter-conversion of pyruvate and lactate, which may serve for the connection of the altered glycolysis-gluconeogenesis pathways with the altered citric acid cycle, therefore allowing an increased production of glucose.
Finally, an increased production of the chaperone protein DnaK (spot 9) was found in response to water stress by increasing the PEG concentration in the media from 5% to 50%. The DnaK protein actively participates in the response to hyperosmotic shock and it is also involved in chromosomal DNA replication. As previously described by our group, an increased production of DNA by Microbacterium sp. 3J1 was found in response to drought, where the DNA molecules seem to have a role as osmoprotectact as proved in vitro for the protection of proteins against desiccation (García-Fontana et al., 2016).
An increased production of the β subunit of the ATP synthetase (spot 13) was found in response to water stress. We associate this increased production with the energy metabolism of Microbacterium sp. 3J1 in an analogous manner to the need of ATP during droughts in plants. Another finding was the decreased production of acyl-CoA dehydrogenase in response to water stress (spot 5). Acyl-CoA dehydrogenases are enzymes catalyzing the α, β-dehydrogenation of acyl-CoA esters in fatty acid and amino acid catabolism. We associate this reduced production of the acyl-CoA dehydrogenase under drying conditions with being part of the shut down of the lipid metabolism within the general arrest of metabolism found in anhydrobionts during the desiccated state. A reduced production of the acyl-CoA dehydrogenase was observed in Bradyrhizobium japonicum, a desiccation-tolerant microorganism, in response to other stressors, such as acidic pH (Puranamaneewiwat et al., 2006), or in Acinetobacter baumannii and in the cyanobacteria Leptolyngbya ohadii among other desiccation-tolerant microorganisms in response to desiccation (Gayoso et al., 2014; Oren et al., 2017).
Conclusion
The desiccation-tolerant bacteria Microbacterium sp. 3J1 has been characterized at biochemical and metabolomic levels due to its response to drought. Despite the ability of Microbacterium sp. 3J1 to protect a diverse range of plants from drought, its response to drought was not characterized at the proteomics level to date. The ability to tolerate desiccation has been described in several microorganisms, and many of them have been described as able to protect plants from drought. However, the information available on the proteomics of desiccation-tolerant microorganisms and their role in protecting plants from drought is scarce. In the present study, we observe a parallel pattern between the protection of plants from drought conducted by Microbacterium sp. 3J1 and the mechanisms that naturally desiccation-tolerant plants use to survive desiccation. Despite of the large variety of mechanisms used by plants, a common strategy consisting of increasing the production of osmotic protectants, increasing energy metabolism, improving the antioxidant protection and reshaping the cell signaling in the plant via plant hormone production was found. We describe a similar pattern in pepper plants protected from drought by Microbacterium sp. 3J1, where all these molecular strategies are altered by an altered expression of proteins. The presence of Microbacterium sp. 3J1 in pepper plants subjected to drought seems to respond to the mechanism that the bacterium itself uses to survive drought, where the microorganism seems to take control of the plant metabolism for a higher chance of survival. We believe that this knowledge may form the basis for the development of alternative strategies to protect desiccation-sensitive food plants such as C. annum, which are affected by droughts frequently.
Materials and Methods
Bacteria and Plant Growth Conditions
Green Pepper Capsicum annuum L. cv. Maor plants were produced by the specialist grower SaliPlant S.L. (Granada, Spain). Plants were grown in green houses under constant relative humidity (50–60%). The plants were illuminated with a 12-h light/dark cycle. Once plants were purchased, they were incubated in the lab under similar humidity and light/dark cycle. For the light cycle, 200 μmol photons⋅m–2 s–1 were used. In addition, dawn–dusk cycles were included with 150 μmol photons⋅m–2 s–1. The temperature ranged from 18 to 20°C during the dark period and from 20 to 25°C during the light period.
For inoculation, half of the plants were treated with 4 mL of bacterial suspension (108–109 CFU/mL) per plant in sterile saline solution, while the other half of the plants were used as non-inoculated controls and watered with the same volume of sterile saline solution.
Plants were regularly watered and allowed to acclimate for at least 2 weeks after inoculation before simulation of drought was implemented.
For inoculation, Microbacterium sp. 3J1 was used as a rhizobacteria drought-tolerance enhancer. These bacteria were grown in tryptic soy broth (TSB) at 30°C and 180 rpm (Manzanera et al., 2004).
Dehydration Treatment and Determination of the Relative Water Content (RWC)
Three groups of C. annuum plants, each consisting of three individual plants, were dried down by withholding water. A control group of plants was watered with an equivalent volume of saline solution in the absence of inoculant. Plants of each condition (with and without inoculant) were extracted from the substrate and completely cleaned of soil by several washes in distilled water. Roots tissues were cut and preserved until sample preparation and protein extraction by wrapping in aluminum foil and storing at −80°C after treatment with liquid nitrogen.
Fresh weight (FW), dry weight (DW), and fully turgid weight (FTW) of the whole plants free from soil were recorded. The RWC was calculated according to Vílchez et al. (2016) and to Mayak et al. (2004) using the following equation RWC = (FW–DW) × (FTW–DW)–1. In addition, root length (RL) and stem length (SL) were measured.
Polyethylene glycol 8000 was added to the microorganism culture to simulate dehydration. Microbacterium 3J1 were grown in TSB supplemented with 5% (w/v) PEG 8000, at an initial absorbance of 0.05 at 600 nm wavelength. The cultures were incubated for 24 h and then split into two. Half of cultures were supplemented with 50% PEG 8000 to simulate severe drought. The cells were further incubated at 30°C and 180 rpm until stationary phase was reached and then, harvested by centrifugation at 15,000 g for 30 min. The cell pellets were stored at −80°C until use.
Isolation of Total Protein
Frozen pellets, consisting of 0.5 g of plant tissue or cells collected from 10 ml of Microbacterium sp. 3J1 cultures were resuspended in 1 mL lysis buffer containing 500 mM Tris–HCl pH 6.8, 4% sodium docecyl sulfate (SDS; w/v), 30% glicerol (v/v), 1 mM EDTA, 5 mM phenylmethanesulfonyl fluoride (PMSF), 200 mM dithiothreitol (DTT), 0,01% Benzonase® Nuclease (v/v; Roche). Three biological replicates (three different plants and three different bacterial cultures) and technical replicates (three different 2D-PAGE) were used.
For the plants and bacterial pellets, cells were broken using FastPrep (three cycles of 40 s at 6.5 m/s). The lysate was centrifuged for 15 min at 20,000 g and 4°C, and the supernatant was recovered for soluble protein quantification by Bradford Method. Soluble proteins (150 μg) were precipitated. Protein precipitation was performed by adding 50% (v/v) ice cold trichloroacetic acid (TCA) to obtain a final concentration of 10% TCA, which was then incubated on ice for 15 min. The samples were centrifuged for 15 min at 20,000 g and 4°C, and then the protein pellet was washed three times with 1 ml of chilled acetone and it was centrifuged at 4°C for 5 min at 20,000 g. Finally, the dry samples were stored at −20°C until use.
Two-Dimensional Polyacrylamide Gel Electrophoresis (2D-PGAGE)
Total soluble protein was dissolved in 300 μL of rehydration buffer (RH) 8 M Urea, 2 M Thiourea, 2% (w/v) Chaps, 0.5 mM PMSF, 20 mM DTT, 0.5% (v/v) Bio-Lyte Ampholyte (BioRad), and trace amounts of bromophenol blue, at 15°C 2 h and 200 rpm. The protein samples were then centrifuged for 15 min at 20,000 g and 4°C.
Supernatants, containing 150 μg soluble protein fraction, were separated by isoelectric focusing (IEF) using a 17-cm non-linear pH 3.0–10.0 immobilized pH gradient (IPG) for plant proteins and a non-linear pH 4.0–7.0 IPG strips (ReadyStrips, BioRad) for bacterial proteins in the first dimension. IEF was performed at 20°C. Strips were first actively rehydrated at 50 V for 14 h which accumulated to 288 V/h. IEF was performed using a Protean i12 IEF System (BioRad) with the following program: and initial step of 100 V for 5 h, followed by four step gradients of 500 V for 30 min, 500 V for 7 h, 1,000 V to 500 V/h, and 8000 V to 13,500 V/h. At the end, a step of 8,000 V to 45,000 V/h was reached. A total of 64,076 V/h were accumulated at the end.
For the second dimension, IPG strips were equilibrated for 15 min in 5 mL of reducing equilibration buffer [75 mM Tris–HCl pH 8.8, 6 M urea, 30% (v/v) glycerol, 2% (w/v) SDS, 10 mg/mL of DTT, and traces of bromophenol blue] followed by 15 min incubation in 5 mL of alkylating equilibration buffer [75 mM Tris–HCl pH 8.8, 6 M urea, 30% (v/v) glycerol, 2% (w/v) SDS, 25 mg/mL of iodoacetamide and traces of bromophenol blue]. The second dimension SDS-PAGE was performed on a PROTEAN II xi Basic Electrophoresis System (BioRad) at 1 mA/gel and 100 V at 15°C, overnight until the bromophenol blue reached the bottom of the gel. The entire electrophoresis unit was protected from direct light during the run.
After electrophoresis, gels were stained for 2 h with fluorescent Oriole Solution (BioRad), protected from direct light and under constant shaking.
Image Acquisition and Image Analysis
Stained gels were immediately observed at excitation/emission wavelengths of 270/604 nm using ChemiDoc MP Imaging System (BioRad). The gel images were captured using ImageLab software, using the automatic exposure and 24.5°cm width.
Images were analyzed with PDQuest Basic Software (Bio-Rad, Hercules, CA, United States). Spots differentially expressed were selected by two different criteria: Qualitative spots (presence/absence), and quantitative spots (p = ≤ 0.05) with a fold difference of 2 compared to the control.
Gel Excision and In-Gel Digestion of Proteins
The spots containing the proteins of interest were cut using the EXQuest Spot Cutter (Bio-Rad).
Gel pieces containing the proteins of interest were digested using the DigestPro MS (Intavis) instrument. Proteins were reduced with DTT 10 mM in BCA 50 mM (56°C 1 h), alkylated with iodoacetamide (IA) 55 mM in BCA 50 mM (30 min), and enzymatically digested with Trypsin Gold Mass Spectrometry Grade (Promega) for 10 h at 37°C. Peptides were eluted with trifluoroacetic acid (TFA) 0.2% and acetonitrile (AcN) 30%.
Mass Spectrometry (MS) Identification of Proteins
Tryptic digests of each spots were desalted with Zip Tip μC18 Pipette Tips (Millipore, Bedford, CA, United Kingdom) according to the recommended protocol and eluted directly on the AnchorChip target plate (Bruker-Daltonics, Hamburg, Germany) with α-cyano-4-hydroxycinnamic acid (CHCA) matrix. The MS and tandem MS (MS/MS) spectra were obtained using an UltrafleXtreme MALDI-TOF/TOF mass spectrometer (Bruker-Daltonics) in auto-mode using Flex Control v3.4 (Bruker-Daltonics) and processed using ProteinScape v3.1.3 (Bruker-Daltonics). Peptide spectra were acquired in reflectron mode with 2,000 laser shots per spectrum. Spectra were externally calibrated using peptide calibration standard (Bruker). A total of 2,500 laser shots were accumulated for MS/MS data. The PMF and MS/MS search were performed on NCBI database for Viridiplantae and Capsicum annuum searching for plant proteins. Bacterial proteins of Microbacterium sp. 3J1 were searched in databases for Microbacteriae, Micrococci, and Actinobacteria. SwissProt database was used for all organisms, using MASCOT 2.4.0 software integrated together with ProteinScape software (Bruker-Daltonics). The parameters used for the search engine were: monoisotopic peptide mass accuracy of 50 ppm, fragment mass accuracy to ±0.5 Da; maximum of only one missed cleavage; carbamidomethylation of cysteine as a fixed modification and partial oxidation of methionine as a variable modification. There were no restrictions regarding the molecular weight (MW) and isoelectric point (pI) of the proteins. Filtering of peaks was done for known autocatalytic trypsin peaks; the signal to noise threshold was set to 2. The significance threshold was set at p < 0.05.
Author’s Note
This manuscript has been released as a pre-print at BioRxiv (García-Fontana et al., 2020).
Data Availability Statement
The original contributions presented in the study are publicly available. This data can be found here: https://datadryad.org/stash/share/weamto_bvAkLYgWdvB6gSpKLQIXpM7EolKZ2LR0PARk.
Author Contributions
MM designed the experimental procedures, wrote the manuscript, and analyzed the results. CG-F and JV performed the experiments, did the statistical analysis, and revised the manuscript. All authors contributed to the article and approved the submitted version.
Funding
This work was funded by the Spanish Ministry for Economy and Competitiveness and the European Union, within the context of the research projects CTM2017-84332-R, and CGL2017-91737-EXP and by the Andalusian Regional Government and the European Union under the aegis of research project P11-RNM-7844 and P18-RT-976. JV was funded by the Spanish Ministry of Economy with a FPU fellowships.
Conflict of Interest
The authors declare that the research was conducted in the absence of any commercial or financial relationships that could be construed as a potential conflict of interest.
References
Ambastha, V., and Tiwari, B. S. (2015). Cellular water and Anhydrobiosis in plants. J. Plant Growth Regul. 34, 665–671. doi: 10.1007/s00344-015-9497-6
Anderson, L. E., Ringenberg, M. R., Brown, V. K., and Carol, A. A. (2005). Both chloroplastic and cytosolic phosphofructoaldolase isozymes are present in the pea leaf nucleus. Protoplasma 225, 235–242. doi: 10.1007/s00709-005-0099-1
Bentley, J., Moore, J. P., and Farrant, J. M. (2019). Metabolomics as a complement to phylogenetics for assessing intraspecific boundaries in the desiccation-tolerant medicinal shrub Myrothamnus flabellifolia (Myrothamnaceae). Phytochemistry 159, 127–136. doi: 10.1016/j.phytochem.2018.12.016
Berg, I. A., Kockelkorn, D., Ramos-Vera, W. H., Say, R. F., Zarzycki, J., Hügler, M., et al. (2010). Autotrophic carbon fixation in archaea. Nat. Rev. Microbiol. 8, 447–460.
Bianchi, G., Gamba, A., Murelli, C., Salamini, F., and Bartels, D. (1991). Novel carbohydrate metabolism in the resurrection plant Craterostigma plantagineum. Plant J. 1, 355–359. doi: 10.1046/j.1365-313x.1991.t01-11-00999.x
Bohler, S., Sergeant, K., Jolivet, Y., Hoffmann, L., Hausman, J.-F., Dizengremel, P., et al. (2013). A physiological and proteomic study of poplar leaves during ozone exposure combined with mild drought. Proteomics 13, 1737–1754.
Boutet, E., Lieberherr, D., Tognolli, M., Schneider, M., Bansal, P., Bridge, A. J., et al. (2016). “UniProtKB/swiss-prot, the manually annotated section of the uniprot knowledgebase: how to use the entry view,” in Plant Bioinformatics: Methods and Protocols, ed. D. Edwards, (New York, NY: Springer), 23–54.
Challabathula, D., and Bartels, D. (2013). Desiccation tolerance in resurrection plants: new insights from transcriptome, proteome and metabolome analysis. Front. Plant Sci. 4:482. doi: 10.3389/fpls.2013.00482
Challabathula, D., Zhang, Q., and Bartels, D. (2018). Protection of photosynthesis in desiccation-tolerant resurrection plants. J. Plant Physiol. 227, 84–92. doi: 10.1016/j.jplph.2018.05.002
Enebe, M. C., and Babalola, O. O. (2018). The influence of plant growth-promoting rhizobacteria in plant tolerance to abiotic stress: a survival strategy. Appl. Microbiol. Biotechnol. 102, 7821–7835. doi: 10.1007/s00253-018-9214-z
Espartero, J., Pintor-Toro, J. A., and Pardo, J. M. (1994). Differential accumulation of S-adenosylmethionine synthetase transcripts in response to salt stress. Plant Mol. Biol. 25, 217–227. doi: 10.1007/bf00023239
Farrant, J. M. (2000). A comparison of mechanisms of desiccation tolerance among three angiosperm resurrection plant species. Plant Ecol. 151, 29–39.
França, M. B., Panek, A. D., and Eleutherio, E. C. A. (2007). Oxidative stress and its effects during dehydration. Comp. Biochem. Physiol. Part A Mol. Integr. Physiol. 146, 621–631. doi: 10.1016/j.cbpa.2006.02.030
Gaff, D. F., Blomstedt, C. K., Neale, A. D., Le, T. N., Hamill, J. D., and Ghasempour, H. R. (2009). Sporobolus stapfianus, a model desiccation-tolerant grass. Funct. Plant Biol. 36, 589–599.
García-Fontana, C., Narváez-Reinaldo, J. J., Castillo, F., González-López, J., Luque, I., and Manzanera, M. (2016). A new physiological role for the DNA molecule as a protector against drying stress in desiccation-tolerant microorganisms. Front. Microbiol. 7:2066. doi: 10.3389/fchem.2018.0002066
García-Fontana, C., Vilchez, J. I., and Manzanera, M. (2020). Proteome comparison between natural desiccation-tolerant plants and drought-protected Caspicum annuum plants by Microbacterium sp. 3J1. bioRxiv [Preprint], doi: 10.1101/2020.03.23.004705
Gayoso, C. M., Mateos, J., Méndez, J. A., Fernández-Puente, P., Rumbo, C., Tomás, M., et al. (2014). Molecular mechanisms involved in the response to desiccation stress and persistence in Acinetobacter baumannii. J. Proteom. Res. 13, 460–476. doi: 10.1021/pr400603f
Georgieva, K., Dagnon, S., Gesheva, E., Bojilov, D., Mihailova, G., and Doncheva, S. (2017). Antioxidant defense during desiccation of the resurrection plant Haberlea rhodopensis. Plant Physiol. Biochem. 114, 51–59. doi: 10.1016/j.plaphy.2017.02.021
Georgieva, K., Szigeti, Z., Sarvari, E., Gaspar, L., Maslenkova, L., Peeva, V., et al. (2007). Photosynthetic activity of homoiochlorophyllous desiccation tolerant plant Haberlea rhodopensis during dehydration and rehydration. Planta 225, 955–964.
Ghasempour, H. R., Anderson, E. M., and Gaff, D. F. (2001). Effects of growth substances on the protoplasmic drought tolerance of leaf cells of the resurrection grass, Sporobolus stapfianus. Funct. Plant Biol. 28, 1115–1120.
Ghasempour, H. R., Anderson, E. M., Gianello, R. D., and Gaff, D. F. (1998a). Growth inhibitor effects on protoplasmic drought tolerance and protein synthesis in leaf cells of the resurrection grass, Sporobolus stapfianus. Plant Growth Regul. 24, 179–183.
Ghasempour, H. R., Gaff, D. F., Williams, R. P. W., and Gianello, R. D. (1998b). Contents of sugars in leaves of drying desiccation tolerant flowering plants, particularly grasses. Plant Growth Regul. 24, 185–191.
Ingle, R. A., Schmidt, U. G., Farrant, J. M., Thomson, J. A., and Mundree, S. G. (2007). Proteomic analysis of leaf proteins during dehydration of the resurrection plant Xerophyta viscosa. Plant Cell Environ. 30, 435–446. doi: 10.1111/j.1365-3040.2006.01631.x
Jiang, G., Wang, Z., Shang, H., Yang, W., Hu, Z., Phillips, J., et al. (2007). Proteome analysis of leaves from the resurrection plant Boea hygrometrica in response to dehydration and rehydration. Planta 225, 1405–1420. doi: 10.1007/s00425-006-0449-z
Julca, I., Alaminos, M., González-López, J., and Manzanera, M. (2012). Xeroprotectants for the stabilization of biomaterials. Biotechnol. Adv. 30, 1641–1654. doi: 10.1016/j.biotechadv.2012.07.002
Kranner, I., and Birtic, S. (2005). A modulating role for antioxidants in desiccation tolerance. Integr. Comp. Biol. 45, 734–740. doi: 10.1093/icb/45.5.734
Lamaoui, M., Jemo, M., Datla, R., and Bekkaoui, F. (2018). Heat and drought stresses in crops and approaches for their mitigation. Front. Chem. 6:26. doi: 10.3389/fchem.2018.00026
Lv, G.-Y., Guo, X.-G., Xie, L.-P., Xie, C.-G., Zhang, X.-H., Yang, Y., et al. (2017). Molecular characterization, gene evolution, and expression analysis of the Fructose-1, 6-bisphosphate Aldolase (FBA) gene family in wheat (Triticum aestivum L.). Front. Plant Sci. 8:1030. doi: 10.3389/fchem.2018.0001030
Manzanera, M., García-Fontana, C., Vílchez, J. I., Narváez-Reinaldo, J. J., and González-López, J. (2015a). Genome sequence of Microbacterium sp. Strain 3J1, a highly desiccation-tolerant bacterium that promotes plant growth. Genome Announc. 3:e00713-15.
Manzanera, M., Narváez-Reinaldo, J. J., García-Fontana, C., Vílchez, J. I., and González-López, J. (2015b). Genome sequence of Arthrobacter koreensis 5J12A, a plant growth-promoting and desiccation-tolerant strain. Genome Announc. 3:e0648-15.
Manzanera, M., Vilchez, S., and Tunnacliffe, A. (2004). High survival and stability rates of Escherichia coli dried in hydroxyectoine. FEMS Microbiol. Lett. 233, 347–352. doi: 10.1111/j.1574-6968.2004.tb09502.x
Mayak, S., Tirosh, T., and Glick, B. R. (2004). Plant growth-promoting bacteria confer resistance in tomato plants to salt stress. Plant Physiol. Biochem. 42, 565–572. doi: 10.1016/j.plaphy.2004.05.009
Mladenov, P., Zasheva, D., Djilianov, D., and Tchorbadjieva, M. (2015). Towards proteomics of desiccation tolerance in the resurrection plant Haberlea rhodopensis. Cr. Acad. Bulg. Sci. 68, 59–64.
Moore, J. P., Westall, K. L., Ravenscroft, N., Farrant, J. M., Lindsey, G. G., and Brandt, W. F. (2005). The predominant polyphenol in the leaves of the resurrection plant Myrothamnus flabellifolius, 3,4,5 tri-O-galloylquinic acid, protects membranes against desiccation and free radical-induced oxidation. Biochem. J. 385, 301–308. doi: 10.1042/bj20040499
Nadeem, S. M., Ahmad, M., Zahir, Z. A., Javaid, A., and Ashraf, M. (2014). The role of mycorrhizae and plant growth promoting rhizobacteria (PGPR) in improving crop productivity under stressful environments. Biotechnol. Adv. 32, 429–448. doi: 10.1016/j.biotechadv.2013.12.005
Narváez-Reinaldo, J. J., Barba, I., González-López, J., Tunnacliffe, A., and Manzanera, M. (2010). Rapid method for isolation of desiccation-tolerant strains and xeroprotectants. Appl. Environ. Microbiol. 76, 5254–5262. doi: 10.1128/aem.00855-10
Ndima, T., Farrant, J., Thomson, J., and Mundree, S. (2001). Molecular characterization of XVT8, a stress-responsive gene from the resurrection plant Xerophyta viscosa Baker. Plant Growth Regul. 35, 137–145.
Neubauer, C., and Yamamoto, H. Y. (1994). Membrane barriers and Mehler-peroxidase reaction limit the ascorbate available for violaxanthin de-epoxidase activity in intact chloroplasts. Photosyn. Res. 39, 137–147. doi: 10.1007/bf00029381
Niles, M. T., Lubell, M., and Brown, M. (2015). How limiting factors drive agricultural adaptation to climate change. Agric. Ecosyst. Environ. 200, 178–185. doi: 10.1016/j.agee.2014.11.010
Oliver, M. J., Cushman, J. C., and Koster, K. L. (2010). “Dehydration tolerance in plants,” in Plant Stress Tolerance: Methods and Protocols, ed. R. Sunkar, (Totowa, NJ: Humana Press), 3–24. doi: 10.1007/978-1-60761-702-0_1
Oliver, M. J., Dowd, S. E., Zaragoza, J., Mauget, S. A., and Payton, P. R. (2004). The rehydration transcriptome of the desiccation-tolerant bryophyte Tortula ruralis: transcript classification and analysis. BMC Genom. 5:89. doi: 10.1186/1471-2164-5-89
Oliver, M. J., Jain, R., Balbuena, T. S., Agrawal, G., Gasulla, F., and Thelen, J. J. (2011). Proteome analysis of leaves of the desiccation-tolerant grass, Sporobolus stapfianus, in response to dehydration. Phytochemistry 72, 1273–1284. doi: 10.1016/j.phytochem.2010.10.020
Oren, N., Raanan, H., Murik, O., Keren, N., and Kaplan, A. (2017). Dawn illumination prepares desert cyanobacteria for dehydration. Curr. Biol. 27, R1056–R1057.
Puranamaneewiwat, N., Tajima, S., and Niamsup, H. (2006). Proteomic analysis of Bradyrhizobium japonicum USDA110 in acidic condition. Chiang Mai J. Sci. 13, 335–345.
Rice-Evans, C., Miller, N., and Paganga, G. (1997). Antioxidant properties of phenolic compounds. Trends Plant Sci. 2, 152–159. doi: 10.1016/s1360-1385(97)01018-2
Santacruz-Calvo, L., González-López, J., and Manzanera, M. (2013). Arthrobacter siccitolerans sp. nov., a highly desiccation-tolerant, xeroprotectant-producing strain isolated from dry soil. Int. J. Syst. Evol. Microbiol. 63, 4174–4180. doi: 10.1099/ijs.0.052902-0
Sapkota, A. R. (2019). Water reuse, food production and public health: adopting transdisciplinary, systems-based approaches to achieve water and food security in a changing climate. Environ. Res. 171, 576–580. doi: 10.1016/j.envres.2018.11.003
Sherman, T. D., Le Gardeur, T., and Lax, A. R. (1995). Implications of the phylogenetic distribution of polyphenol oxidase in plants. Enzym. Brow. Prevent. 600, 103–119. doi: 10.1021/bk-1995-0600.ch008
Sherwin, H. W., and Farrant, J. M. (1998). Protection mechanisms against excess light in the resurrection plants Craterostigma wilmsii and Xerophyta viscosa. Plant Growth Regul. 24, 203–210.
UniProt Consortium (2019). UniProt: a worldwide hub of protein knowledge. Nucleic Acids Res. 47, D506–D515.
Veljovic-Jovanovic, S., Kukavica, B., and Navari-Izzo, F. (2008). Characterization of polyphenol oxidase changes induced by desiccation of Ramonda serbica leaves. Physiol. Plant 132, 407–416. doi: 10.1111/j.1399-3054.2007.01040.x
Veljovic-Jovanovic, S., Kukavica, B., Stevanovic, B., and Navari-Izzo, F. (2006). Senescence- and drought-related changes in peroxidase and superoxide dismutase isoforms in leaves of Ramonda serbica. J. Exp. Bot. 57, 1759–1768. doi: 10.1093/jxb/erl007
Vílchez, J. I., García-Fontana, C., Román-Naranjo, D., González-López, J., and Manzanera, M. (2016). Plant drought tolerance enhancement by trehalose production of desiccation-tolerant microorganisms. Front. Microbiol. 7:1577. doi: 10.3389/fchem.2018.0001577
Vílchez, J. I., Niehaus, K., Dowling, D. N., González-López, J., and Manzanera, M. (2018). Protection of pepper plants from drought by Microbacterium sp. 3J1 by modulation of the plant’s glutamine and α-ketoglutarate content: a comparative metabolomics approach. Front. Microbiol. 9:284. doi: 10.3389/fchem.2018.000284
Wang, X., Chen, S., Zhang, H., Shi, L., Cao, F., Guo, L., et al. (2010). Desiccation tolerance mechanism in resurrection fern-ally Selaginella tamariscina revealed by physiological and proteomic analysis. J. Proteome Res. 9, 6561–6577. doi: 10.1021/pr100767k
Whittaker, A., Bochicchio, A., Vazzana, C., Lindsey, G., and Farrant, J. (2001). Changes in leaf hexokinase activity and metabolite levels in response to drying in the desiccation-tolerant species Sporobolus stapfianus and Xerophyta viscosa. J. Exp. Bot. 52, 961–969. doi: 10.1093/jexbot/52.358.961
Yobi, A., Wone, B. W. M., Xu, W., Alexander, D. C., Guo, L., Ryals, J. A., et al. (2013). Metabolomic profiling in Selaginella lepidophylla at various hydration states provides new insights into the mechanistic basis of desiccation tolerance. Mol. Plant 6, 369–385. doi: 10.1093/mp/sss155
Keywords: drought tolerance, Microbacterium sp. 3J1, Capsicum annum, comparative proteomics, actinobacteria
Citation: García-Fontana C, Vilchez JI and Manzanera M (2020) Proteome Comparison Between Natural Desiccation-Tolerant Plants and Drought-Protected Caspicum annuum Plants by Microbacterium sp. 3J1. Front. Microbiol. 11:1537. doi: 10.3389/fmicb.2020.01537
Received: 21 April 2020; Accepted: 12 June 2020;
Published: 10 July 2020.
Edited by:
Davide Neri, Marche Polytechnic University, ItalyReviewed by:
Jean Armengaud, Commissariat à l’Energie Atomique et aux Energies Alternatives (CEA), FranceSang-Wook Han, Chung-Ang University, South Korea
Copyright © 2020 García-Fontana, Vilchez and Manzanera. This is an open-access article distributed under the terms of the Creative Commons Attribution License (CC BY). The use, distribution or reproduction in other forums is permitted, provided the original author(s) and the copyright owner(s) are credited and that the original publication in this journal is cited, in accordance with accepted academic practice. No use, distribution or reproduction is permitted which does not comply with these terms.
*Correspondence: Maximino Manzanera, bWFuemFuZXJhQHVnci5lcw==
†These authors have contributed equally to this study
‡Present address: Cristina García-Fontana, Biomedical Research Institute of Granada (Ibs.GRANADA), Fundación para la Investigación Biosanitaria de Andalucía Oriental (FIBAO), Granada, Spain Juan Ignacio Vilchez, Shanghai Center for Plant Stress Biology (PSC), Chinese Academy of Sciences, Shanghai, China