- 1Department of Clinical Veterinary Medicine, College of Veterinary Medicine, China Agricultural University, Beijing, China
- 2Department of Production Animal Health, Faculty of Veterinary Medicine, University of Calgary, Calgary, AB, Canada
- 3Section of Microbiology, Department of Pathobiology, College of Veterinary Sciences and Animal Husbandry, Abdul Wali Khan University, Mardan, Pakistan
- 4College of Veterinary Medicine, Shandong Agricultural University, Tai’an, China
Inflammation is the hallmark of extended-spectrum β-lactamase (ESBL)-producing Escherichia coli-induced bovine mastitis. Organic selenium can activate pivotal proteins in immune responses and regulate the immune system. The present study aimed to investigate whether selenomethionine (SeMet) attenuates ESBL E. coli-induced inflammation in bovine mammary epithelial cells (bMECs) and macrophages. Cells were treated with 0, 5/10, 10/20, 20/40, or 40/60 μM SeMet for 12 h and/or inoculated with ESBL-E. coli [multiplicity of infection (MOI) = 5] for 4/6 h, respectively. We assessed inflammatory responses, including selenoprotein S (SeS), Toll-like receptor 4 (TLR4), Ikappa-B (IκB), phospho-NF-κB p65 (Ser536), interleukin-1β (IL-1β), tumor necrosis factor-α (TNF-α), and lactate dehydrogenase (LDH) activities. Treatment with 40/60 μM SeMet promoted cell viability and inhibited LDH activities in both bMECs and macrophages. Inoculation with ESBL-E. coli reduced cell viability, which was attenuated by SeMet treatment in bMECs and macrophages. SeMet increased ESBL E. coli-induced downregulation of SeS and decreased LDH activities, TLR4, IκB, phospho-NF-κB p65 (Ser536), IL-1β, and TNF-α protein expressions in bMECs and macrophages. In addition, knockdown of SeS promoted protein expression of TLR4-mediated nuclear factor-kappa (NF-κB) pathway and BAY 11-708 inhibited TNF-α and IL-1β protein levels in bMECs and macrophages after ESBL-E. coli treatment. Moreover, ESBL-E. coli inoculation increased monocyte chemoattractant protein 1 (MCP-1), C–C motif ligand 3 (CCL-3), and CCL-5 mRNA expressions in bMECs. In conclusion, ESBL-E. coli induced expression of MCP-1, CCL-3, and CCL-5 in bMECs and then recruited and activated macrophages, whereas SeMet attenuated ESBL E. coli-induced inflammation through activated SeS-mediated TLR4/NF-κB signaling pathway in bMECs and macrophages.
Introduction
Escherichia coli is an opportunistic environmental pathogen affecting bovine mammary glands, causing clinical mastitis, particularly during early lactation in dairy cows (Olde Riekerink et al., 2008; Gao et al., 2017). Prevalence of highly resistant extended-spectrum β-lactamase (ESBL)-producing E. coli is increasing in food-producing animals in various countries, including China (Liu et al., 2014; Ali et al., 2016; Zhuang et al., under review). The high incidence of multidrug-resistant E. coli strains is problematic, as treatment options are limited and severe complications are common (Mukherjee et al., 2013). Therefore, a better understanding of the pathogenesis of this major bacterial disease is needed.
Escherichia coli penetrates the lumen of the mammary gland, promoted by lactate dehydrogenase (LDH) release (Vangroenweghe et al., 2005) and incites distinct inflammatory and innate immune responses (Gilbert et al., 2013). When infection occurs, mammary epithelial cells and entry of macrophages into the mammary gland are initial responses against mastitis pathogens (Bougarn et al., 2011; Vural and Kehrl, 2014). Therefore, bovine mammary epithelial cells (bMECs), and macrophage lines are an ideal model to evaluate in vitro inflammation induced by E. coli. Furthermore, they are well characterized, readily available, and moderately priced. In addition, mammary epithelial cells and macrophages each have an array of germline-encoded pattern recognition receptors/sensors that recognize pathogen-associated molecular patterns and which activate the nuclear factor-kappa B (NF-κB) signaling pathway to mediate inflammatory and innate immune responses. In response to cell stimulation, NF-κB, which binds with Ikappa-B (IκB) protein in the cytoplasm, is released and translocates into the nucleus. Furthermore, the P65 subunit of NF-κB contains the transcriptional activation domain, which regulates transcription of inflammatory proteins during infection, e.g., tumor necrosis factor-α (TNF-α), and interleukin-1β (IL-1β; Lecoq et al., 2017).
Selenium (Se) is an essential trace element, with a Se-enriched diet being the major source of Se intake for mammals. To correct Se deficiency, recommended Se supplementation is 0.3, 0.15 to 0.2, and 0.1 mg/kg dry matter Se in the United States, Germany, and Britain, respectively (Salman et al., 2009). The major representative chemical forms of Se are inorganic Se (selenite and selenate) and organic Se [selenocysteine, selenomethionine (SeMet), methylselenocysteine, and Se-enriched yeast] (Gabel-Jensen and Gammelgaard, 2010). These Se forms are involved in various biochemical and physiological functions, including immunity, antioxidant function, and aging (Kubachka et al., 2017). In addition, Se can regulate the inflammatory process (Kaushal et al., 2014), and selenocompounds can influence macrophage functions (Shimohashi et al., 2000). Moreover, feeding Se-yeast increased percentage of E. coli killed by blood neutrophils (Cebra et al., 2003). However, protective effects and molecular mechanisms of SeMet on ESBL-E. coli infection in bMECs and macrophages remain unclear. Therefore, the present study established an in vitro experimental model of bMECs and macrophages exposed to ESBL-E. coli to determine whether SeMet conferred anti-inflammatory defense capacity via modulation of Toll-like receptor 4 (TLR4)/NF-κB signaling pathway.
Materials and Methods
Statement of Ethics
This study was conducted in accordance with ethical guidelines and standard biosecurity and institutional safety procedures of China Agricultural University (CAU; Beijing, China). Prior to the start of the study, ethical approval was granted by the Departmental Committee of the College of Veterinary Medicine, CAU.
Reagents and Antibodies
SeMet, dimethyl sulfoxide (DMSO), 3-(4,5-dimethyl-thiazol-2-yl)-2,5-diphenyl tetrazolium bromide (MTT), and N-acetyl-l-cysteine were acquired from Sigma-Aldrich Chemical (cat # 1217470-45-5, 298-93-1 and 616-91-1, Sigma, St. Louis, MO, United States). LDH activity assay kit was purchased from Beyotime Biotechnology (Shanghai, China). Opti-MEM (cat #SH30265.01) and fetal bovine serum (FBS, cat #10099-141) were purchased from Gibco (Grand Island, NY, United States). Lipofectamine 2000 reagent was from Thermo Fisher Scientific (Waltham, MA, United States). TransScript First-Strand complementary DNA (cDNA) Synthesis SuperMix and SYBR Green PCR Core Reagents were purchased from TransGen Biotech (TransGen Biotech, Beijing, China). Trizol Reagent was bought from Invitrogen (Carlsbad, CA, United States). The siRNA duplexes for bovine and murine Selenoprotein S (SeS) and two siRNA negative controls were purchased from Sangon Biotech (Guangdong, China). Primary antibodies to TLR4 (cat #19811-1-AP, 1:1000), NF-κB (cat #10745-1-AP, 1:1000), SeS (cat #15591-1-AP, 1:500), IKB (cat #10268-1-AP, 1:1000), TNF-α (cat # 17590-1-AP, 1:1000), IL-1β (cat #16806-1-AP, 1:1000), GAPDH (cat # 10494-1-AP, 1:2000), and PCNA (cat # 10205-2-AP, 1:2000) were obtained from Proteintech (Proteintech, Wuhan, China), and primary antibodies to the phospho-NF-κB p65 (Ser536; p-p65; cat # bs-0982R, 1:1000) were obtained from Bioss (Beijing, China). Horseradish peroxidase (HRP)-conjugated goat anti-rabbit (1:5000) IgG antibodies was from CW Bio (Beijing, China).
Bacterial Strains, Cell Culture, and Treatment
A strain of sequence type ESBL 410(4) E. coli, isolated from a milk sample of a dairy cow with clinical mastitis in Inner Mongolia (Ali et al., 2017), was used. This isolate induced the most profound changes in inflammation, oxidative stress, and apoptosis (Zhuang et al., under review). ST410(4) E. coli was re-established from frozen stocks by culturing on tryptose soya agar (Difco TM, Becton Dickinson, Sparks, MD, United States) supplemented with 5% defibrinated sheep blood and incubated at 37°C for 72 h, then sub-cultured on Lysogeny broth medium to mid-log phase. The bMECs line MAC-T (Shanghai Jingma Biological Technology, Shanghai, China) and murine macrophage cell line J774A.1 (Sigma-Aldrich, St. Louis, MO, United States) were cultured as previously described (Nakase et al., 2017; Shahid et al., 2017). The SeMet was solubilized in distilled water (concentration, 0.1 mol/L) for storage at −80°C and subsequently thawed and diluted with distilled water into working solutions of 200 and 400 μmol/L (μM). The bMECs and macrophages were cultured in Dulbecco’s modified eagle medium (DMEM) containing various concentrations of SeMet. After SeMet exposure for 12 h, bMECs and macrophages were infected with ESBL-producing ST410(4) E. coli at a MOI of 0 and 5 in 6-well plates for 4 and 6 h at 37°C, respectively. BAY 11-7082 (10/20 μM; Beyotime Biotechnology) was treated for 1 h before SeMet treatment. Furthermore, a blank control group with no E. coli or SeMet whatsoever was also included. Broth was centrifuged (10,000 × g for 10 min) and cells and supernatant were stored separately at −80°C until analyzed.
Cell Viability Assay
Cell viability was determined using an MTT reduction assay. The bMECs and macrophages were incubated with 0, 5, 10, 20, 40, 60, 80, and 100 μmol/L SeMet for 12 h. DMEM solution containing 10% MTT was added to the treated cells in each well. Cells were incubated at 37°C for 4 h, supernatants were removed, and formazan crystals were dissolved in 150 μl of DMSO. Absorbance was recorded at a wavelength of 490 nm and reference wavelength of 630 nm on a 318 MC microplate reader (Shanghai Sanco Instrument, Shanghai, China). Each group was assayed 20 times (10 replicates per group and each sample assayed in duplicate).
Determination of LDH Activity
The bMECs and macrophages were cultured in 96-well plates and incubated with 0, 5, 10, 20, 40, 60, 80, or 100 μmol/L SeMet for 12 h. Thereafter, treated cells were infected with one ESBL-producing E. coli [ST410(4)] for 4 or 6 h. After infection, spent medium bMECs and macrophages was collected and centrifuged (10,000 × g for 10 min) to detect LDH activities, using an LDH activity assay kit (Beyotime Biotechnology), according to the manufacturer’s instructions. Adsorption values were determined at 490 nm with a 318 MC microplate reader (Shanghai Sanco Instrument). Assay sensitivity was 9.0–5,000 U/L and coefficient of variation was 1.5%. Each group was assayed 20 times (10 replicates per group and each sample assayed in duplicate).
MCP-1, C–C Motif Ligand 3 (CCL-3), and CCL-5 mRNA Expressions
Monocyte chemoattractant protein 1 (MCP-1), CCL-3, and CCL-5 mRNA expressions were analyzed by quantitative real-time reverse transcription-polymerase chain reaction (qRT-PCR). Total RNA was extracted from splenic lymphocytes using Trizol Reagent (Invitrogen) and total RNA was reverse-transcribed using TransScript First-Strand complementary DNA (cDNA) Synthesis SuperMix (TransGen Biotech), according to the manufacturer’s instructions. The PCR primers were designed according to the NCBI database (Table 1) and PCR was performed with a StepOnePlus Real-Time PCR System (Thermo Fisher Scientific) using SYBR Green PCR Core Reagents (TransGen Biotech). Amplification of β-actin mRNA was used as an endogenous control. There were 10 replicates per group and each sample was assayed in triplicate and a mean value was calculated. Data were analyzed according to the 2–ΔΔCt method and results were expressed as relative mRNA levels. Each group was assayed 18 times (three replicates per group and each sample assayed in sextuplicate).
Small Interfering RNA and Transfection of Cells
To knock down SeS, specific small interfering RNA (siRNA) duplexes targeting the bovine and murine SeS gene and two siRNA negative controls were purchased from Integrated DNA Technologies (Sangon Biotech). First, 2.5 nmol SeS siRNA and control siRNA were dissolved in 125 μl of DNase/RNase-free water and stored at −80°C. Then, SeS siRNA and control siRNA stock solution were diluted in Opti-MEM medium and then diluted siRNA was added to Lipofectamine 2000 reagent and incubated for 10 min in bMECs (1.25 μl of SeS siRNA and control siRNA, 30 μl of Opti-MEM medium, and 3 μl of Lipofectamine 2000 reagent) and macrophages (2.5 μl of SeS siRNA and control siRNA, 28.75 μl of Opti-MEM medium, and 3 μl of Lipofectamine 2000 reagent). Cells were treated with the siRNA directed against SeS and the scrambled control siRNA (final concentrations, 25 and 50 nM) using Lipofectamine 2000 reagent (Invitrogen) in Opti-MEM without serum, according to the manufacturer’s instructions, as previously described (Han et al., 2018). After 24 h, transfection medium was removed and 1 ml of DMEM with SeMet (20 and 40 μM) was added to recover cell growth for 12 h. Subsequently, cells were infected with ESBL-producing ST410(4) for 4 or 6 h and then cells were harvested and subjected to Western blotting.
Western Blotting Analysis
The bMECs and macrophages were lysed in buffer containing 10 mM Tris–buffer (pH 7.5), 1 mM PMSF, 1% Triton X–100, 1 mM protease inhibitor cocktail, and 1 mM phosphatase inhibitor. Nucleus extracts were prepared using a KeyGEN nucleus protein extraction kit and protein concentration was quantified using a BCA Protein Assay kit (Beyotime), according to the manufacturer’s instructions. Proteins (30–100 μg) were separated by 12% sodium dodecyl sulfate-polyacrylamide gels electrophoresis (SDS-PAGE) and electrotransferred to PVDF membranes (Millipore Corporation, St. Charles, MO, United States) by a wet transferor (BIO-RAD, United States). Then, PVDF membranes were blocked at room temperature for 2 h in 5% non-fat dry milk in 0.1% Tween-20–Tris buffered saline, pH 7.4 (TBST). After blocking, membranes were incubated overnight with specific primary antibodies at 4°C. Thereafter, membranes were probed with HRP-conjugated secondary antibody for 1 h at room temperature and fluorescence detected with an enhanced chemiluminescence system (ECL; Beyotime). Results were normalized to GAPDH, and band density was analyzed with Image J (National Institutes of Mental Health, Bethesda, MD, United States). Each group was assayed six times (three independent experiments, with two replicates per group).
Statistical Analyses
One-way ANOVA was used to determine effects of SeMet and/or E. coli on LDH activities, cell viability, and protein expression levels in bMECs and macrophages. For all analyses, a least-significant difference test was used to locate differences. All statistical analyses were done with SPSS 23.0 software (SPSS Inc., Chicago, IL, United States) and histograms were produced with GraphPad Prism 7.0 (GraphPad Software, Inc., San Diego, CA, United States). Data were expressed as mean ± standard deviation (SD), with p < 0.05, and p < 0.01 considered significant and highly significant, respectively.
Results
Cytotoxic Effects of Various Concentrations of SeMet on bMEC and Macrophages
To assess cytotoxic effects of selenium (Figure 1), bMECs and macrophages were cultured for 12 h with SeMet at various concentrations and cell viability and LDH activity were measured. Addition of 40 μM SeMet increased viability of bMECs (p < 0.01; Figure 1A) and suppressed LDH activities (p < 0.01; Figure 1B) compared to the blank control group. In addition, 40 and 60 μM SeMet increased macrophage viability (Figure 1C) and decreased LDH release (Figure 1D) without cytotoxicity (p < 0.01) compared to the blank control group. In contrast, 80 and 100 μM SeMet inhibited cell viability and increased LDH activities in both bMECs (p < 0.01; Figure 1A) and macrophages (p < 0.01; Figure 1B).
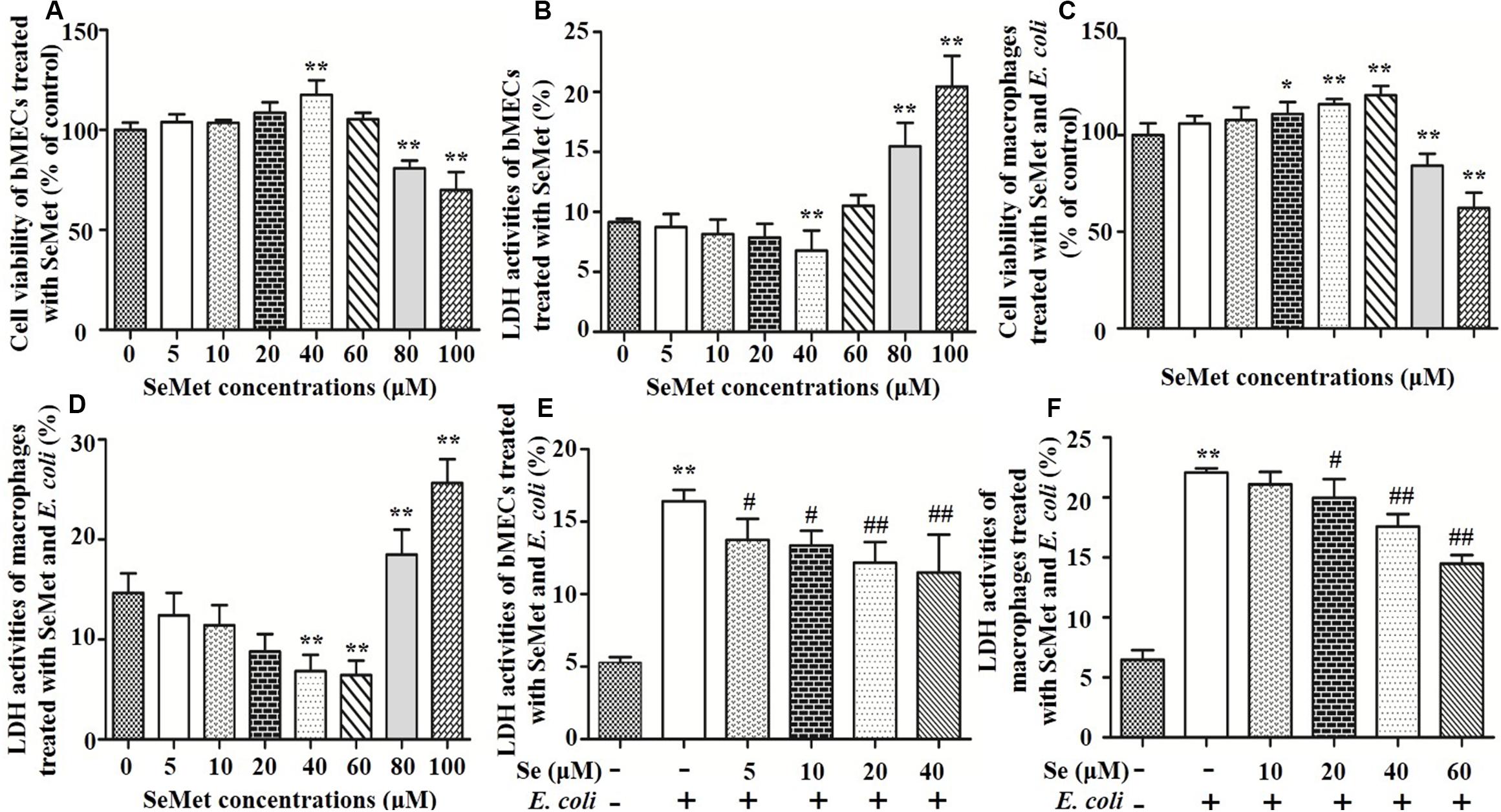
Figure 1. Effects of SeMet and/or ESBL E. coli on bMECs and macrophages. bMECs and macrophages were treated with various concentrations of SeMet for 12 h, followed by ESBL-E. coli for 4/6 h, respectively. (A) Cell viability was determined with an MTT assay in SeMet-treated bMECs. (B) LDH release was detected by LDH Assay Kit in SeMet-treated bMECs. (C) Cell viability was determined with an MTT assay in SeMet-treated macrophages. (D) LDH release was detected with an LDH Assay Kit in SeMet-treated macrophages. (E) LDH activities were determined with an LDH Assay Kit in SeMet and ESBL E. coli-treated bMECs. (F) LDH release was detected with an LDH Assay Kit in SeMet and ESBL E. coli-treated macrophages. Data represent means ± SD of five independent experiments. In each independent experiment, there were 10 replicates per group and each sample was assayed in duplicate with similar results. “–” after Se indicated that SeMet was not added. “–” and “+” after E. coli indicated that E. coli (MOI = 5) were not or were added, respectively. *p < 0.05 and **p < 0.01 were differences compared to the control group; #p < 0.05 and ##p < 0.01 were differences compared to ESBL E. coli-infected samples.
SeMet Reduced LDH Activity After ESBL-E. coli Exposure in bMECs and Macrophages
Exposure to ESBL-E. coli increased LDH release (p < 0.01) compared to the blank control group, which was attenuated (p < 0.05) by SeMet treatment in bMECs (5–40 μM; Figure 1E) and macrophages (20–60 μM; Figure 1F) compared to ESBL E. coli-infected samples.
SeMet Increased SeS and Inhibited Protein Expression Through TLR4-Mediated NF-κB Signaling Pathway Induced by ESBL-E. coli in bMECs and Macrophages
Western blotting analysis demonstrated that SeMet increased (p < 0.05) ESBL E. coli-induced downregulation of SeS in bMECs (5–40 μM; Figures 2A,B) and macrophages (20–60 μM; Figures 3A,B) compared to ESBL E. coli-infected samples. Regarding the TLR4-mediated NF-κB signaling pathway, incubation with ST410(4) for 4 or 6 h increased release of IL-1β and TNF-α (Supplementary Figure 1; p < 0.01), protein expression of TLR4 (Figures 2A,C, 3A,C), IκB (Figures 2A,D, 3A,D), p-p65 (Figures 2A,E, 3A,E; p < 0.01), TNF-α (Figures 2A,F, 3A,F,and Supplementary Figures 1B,D), and IL-1β (Figures 2A,G, 3A,G, and Supplementary Figures 1A,C; p < 0.01) and increased nuclear content of NF-κB (Figures 2A,E, 3A,E; p < 0.01) compared to the blank control group, whereas pre-treatment with SeMet antagonized this increase in bMECs (5–40 μM; Figure 2 and Supplementary Figure 2A) and macrophages (10–60 μM; Figure 3 and Supplementary Figure 2B) compared to ESBL E. coli-infected samples.
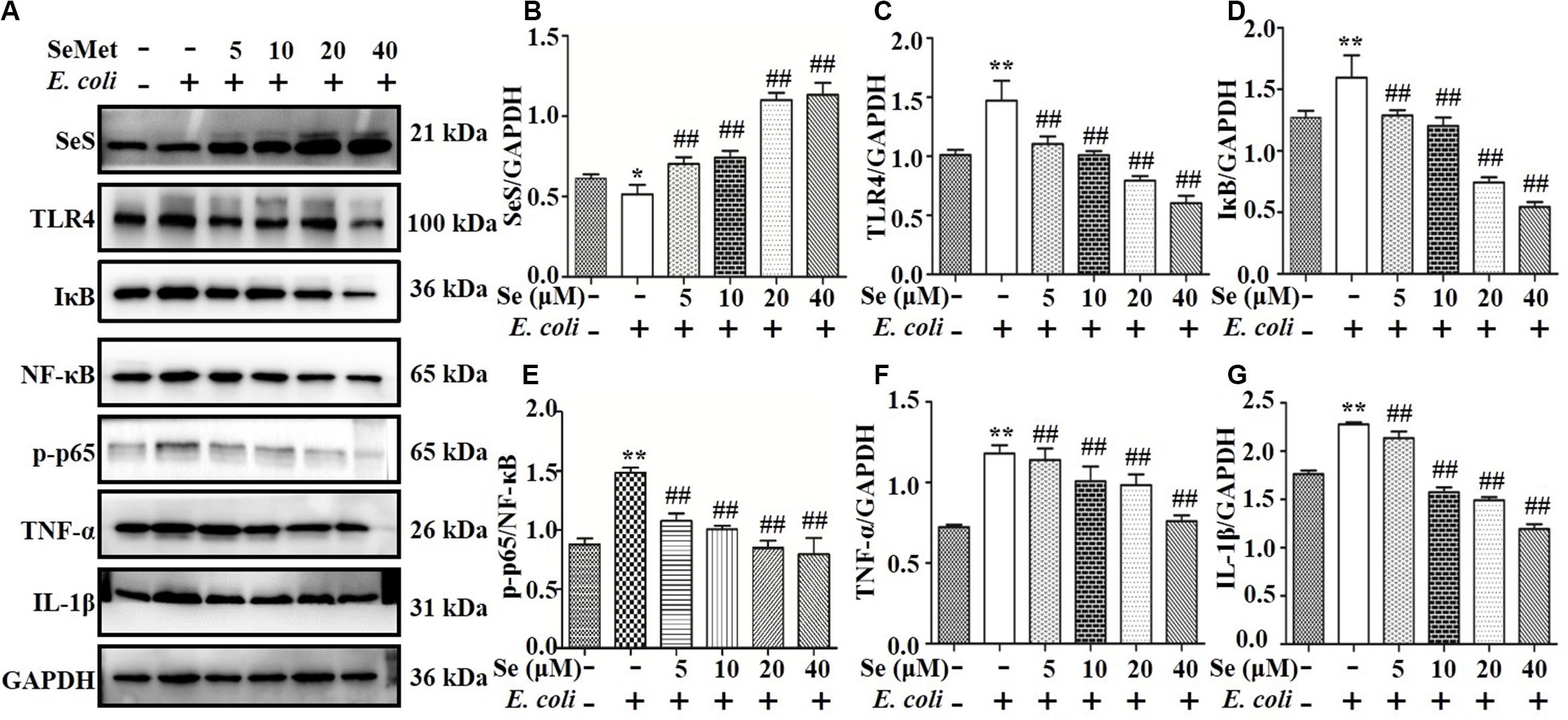
Figure 2. SeMet inhibited activation of TLR4/NF-κB pathway induced by ESBL-E. coli in bMECs. bMECs were pre-treated with various concentrations of SeMet for 12 h, followed by ESBL-E. coli for 4 h. (A–G) Protein levels of SeS, TLR4, IKB, NF-κB, p-p65 (Ser536), TNF-α, and IL-1β were determined by Western blotting. GAPDH was used as loading controls. Data represent means ± SD of three independent experiments. In each independent experiment, there were 10 replicates per group and each sample was assayed in duplicate with similar results. “–” after Se and SeMet indicated that SeMet was not added. “–” and “+” after E. coli indicated that E. coli (MOI = 5) were not or were added, respectively. *p < 0.05 and **p < 0.01, differences compared to control group; ##p < 0.01 differences compared to ESBL E. coli-infected samples.
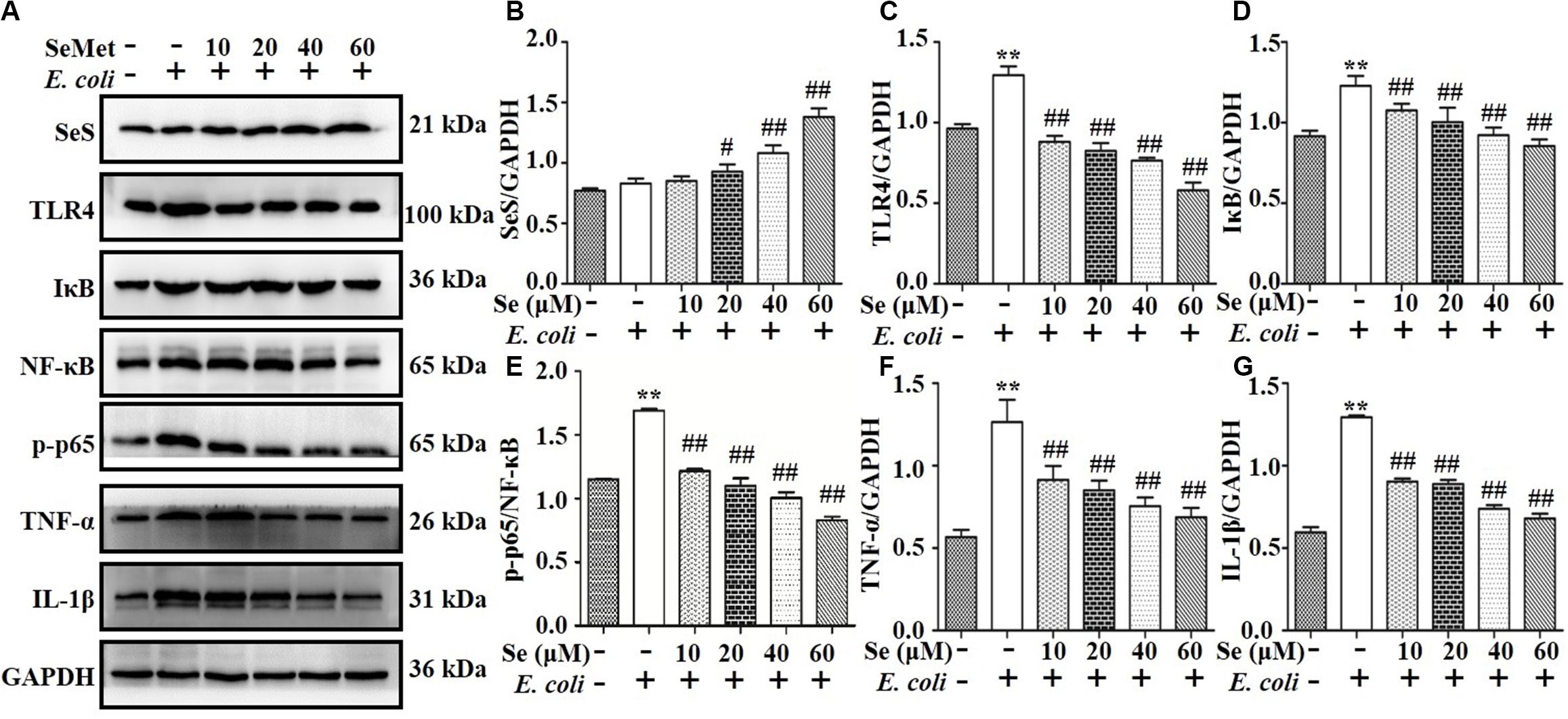
Figure 3. SeMet reversed activation of TLR4/NF-κB pathway induced by ESBL-E. coli in macrophages. Macrophages were pre-treated with various concentrations of SeMet for 12 h, followed by ESBL-E. coli for 6 h. (A–G) Protein levels of SeS, TLR4, IκB, NF-κB, p-p65 (Ser536), TNF-α, and IL-1β were determined by Western blotting. GAPDH was used as loading controls. Data represent means ± SD of three independent experiments. In each independent experiment, there were two replicates per group with similar results. “–” after Se and SeMet indicated that SeMet was not added. “–” and “+” after E. coli indicated that E. coli (MOI = 5) were not or were added, respectively. **p < 0.01, differences compared to the control group; #p < 0.05 and ##p < 0.01 differences compared to ESBL E. coli-infected samples.
ESBL-E. coli Infection Promoted MCP-1, CCL-3, and CCL-5 mRNA Expressions in bMECs
To explore why SeMet promoted protein expressions of TLR4-mediated NF-κB signaling pathway in bMECs and macrophages for 4 and 6 h, respectively, MCP-1, CCL-3, and CCL-5 mRNA expressions were analyzed by qRT-PCR. The ESBL-producing ST410(4) E. coli increased MCP-1, CCL-3, and CCL-5 mRNA expressions compared to the blank control group (p < 0.01) in bMECs (Figure 4).
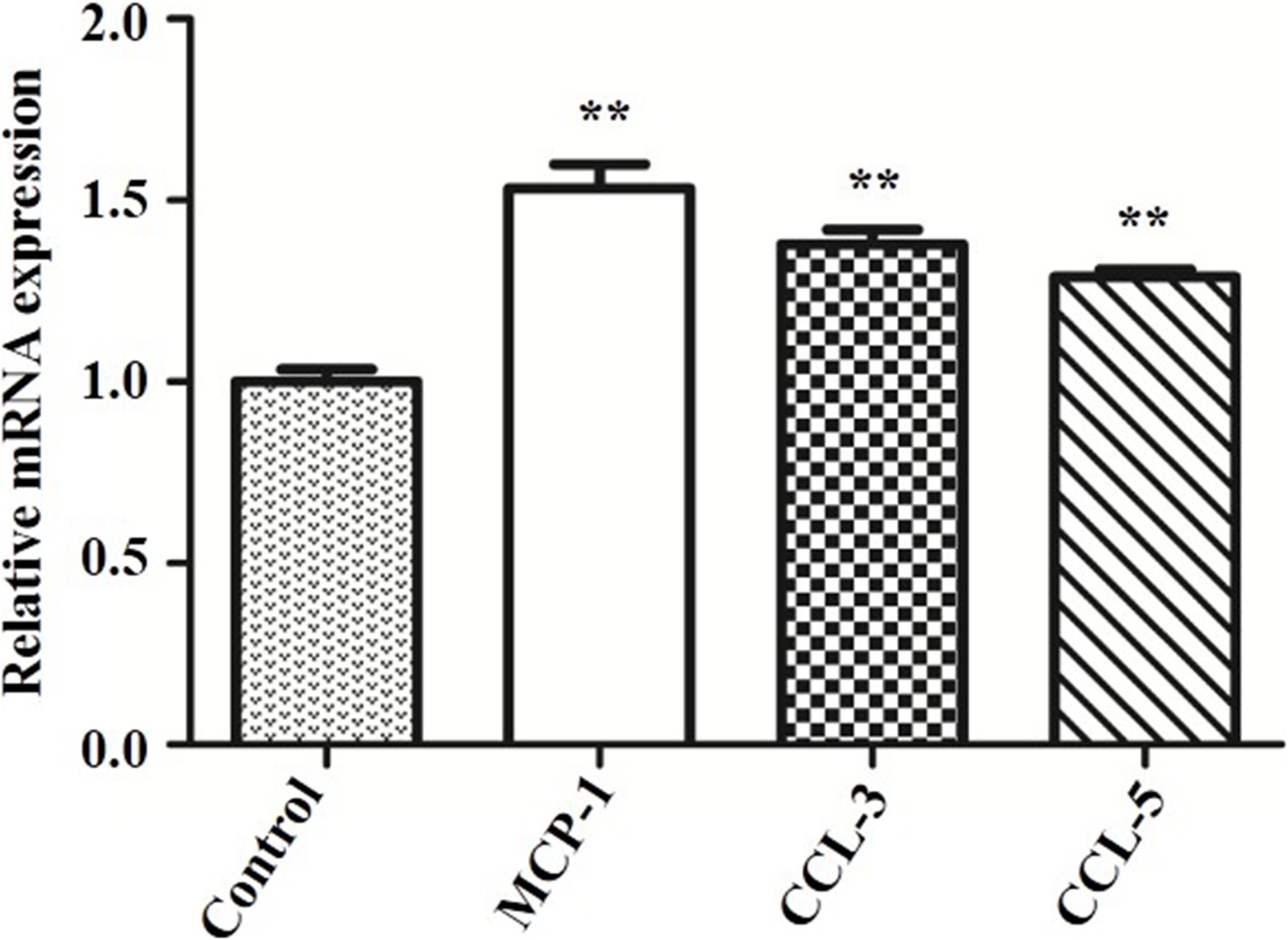
Figure 4. Effect of ESBL E. coli on MCP-1, CCL-3, and CCL-5 mRNA expressions in bMECs. bMECs were infected with ESBL E. coli (MOI = 5) for 4 h. Data represent means ± SD of three independent experiments. In each independent experiment, there were three replicates per group and each sample was assayed in sextuplicate with similar results. **p < 0.01, differences compared to control group.
SeS Depletion Reversed Effects of SeMet on TLR4-Mediated NF-κB Signaling Pathway in bMECs and Macrophages After ESBL-E. coli Infection
To clarify whether decreased activation of TLR4-mediated NF-κB signaling pathway induced by SeMet depended on SeS (Figures 5A,B, 6A,B), siRNA of SeS was introduced to bMECs and macrophages induced by ST410(4) and SeMet. Western blotting demonstrated that knockdown of SeS promoted TLR4 (Figures 5A,C, 6A,C), IKB (Figures 5A,D, 6A,D), TNF-α (Figures 5A,F, 6A,F), and IL-1β (Figures 5A,G, 6A,G) protein expressions, as well as nuclear expression of NF-κB (Figures 5A,E, 6A,E) inhibited by SeMet in bMECs (Figure 5) and macrophages (Figure 6) induced by ST410(4) stimulation (4 h).
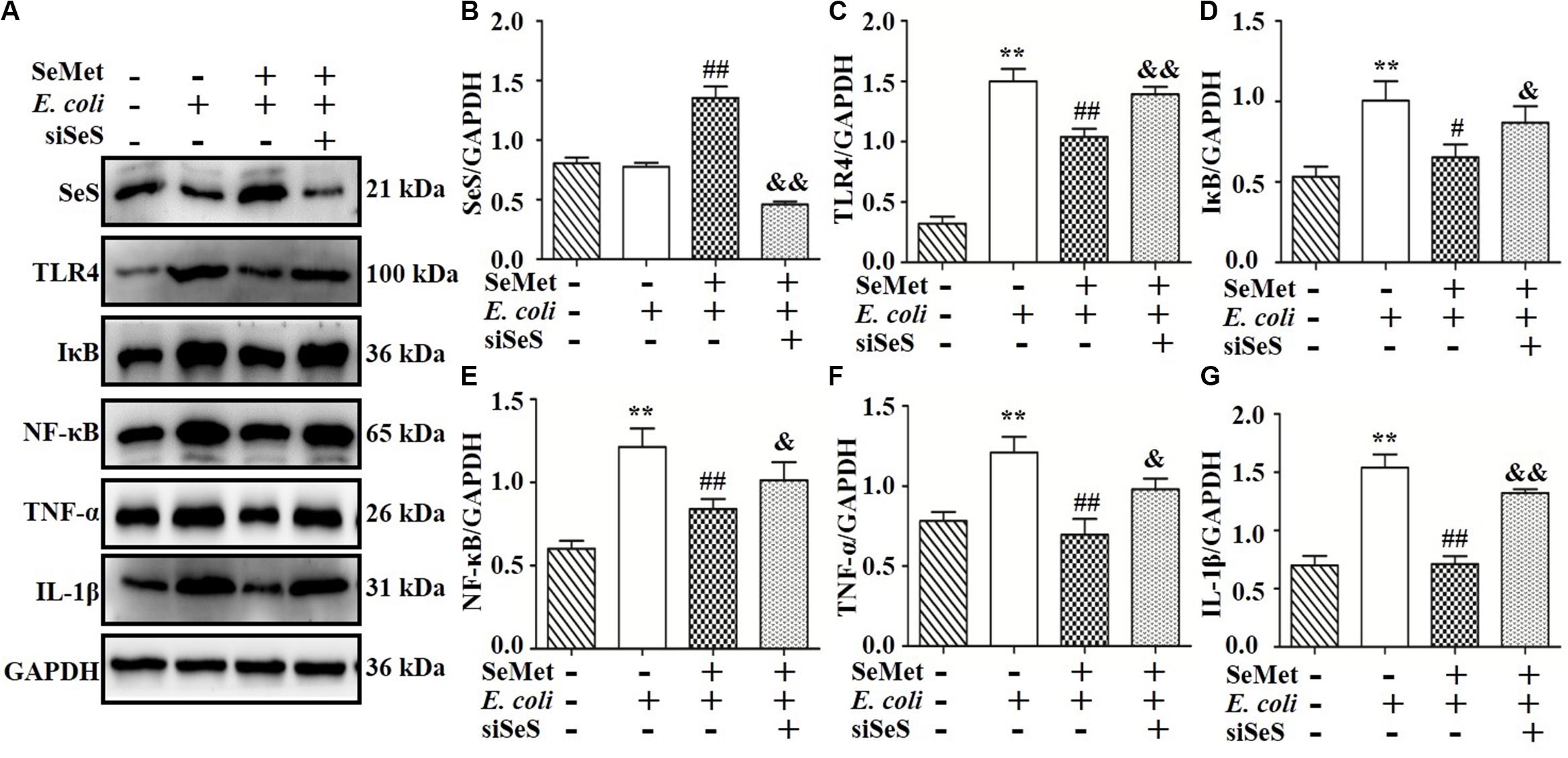
Figure 5. Depletion of SeS reversed effects of SeMet on TLR4/NF-κB pathway in ESBL E. coli-treated bMECs. Cells were transfected with control siRNA or SeS siRNA. Subsequently, transfected cells were treated with SeMet for 12 h, followed by ESBL-E. coli for 4 h. (A–G) Protein levels of SeS, TLR4, IκB, NF-κB, TNF-α, and IL-1β were determined by Western blotting. GAPDH was used as loading controls. Data represent means ± SD of three independent experiments. In each independent experiment, there were two replicates per group with similar results. “–” and “+” after SeMet indicated that SeMet (20 μM) were not or were added, respectively. “–” and “+” after E. coli indicated that E. coli (MOI = 5) were not or were added. “–” and “+” after siSeS indicated that siSeS (25 nM) were not or were added. **p < 0.01, difference compared to control siRNA transfected samples; #p < 0.05 and ##p < 0.01 differences compared to ESBL E. coli-infected control siRNA transfected samples, &p < 0.05 and &&p < 0.01 differences compared to SeMet and ESBL E. coli-treated control siRNA transfected samples.
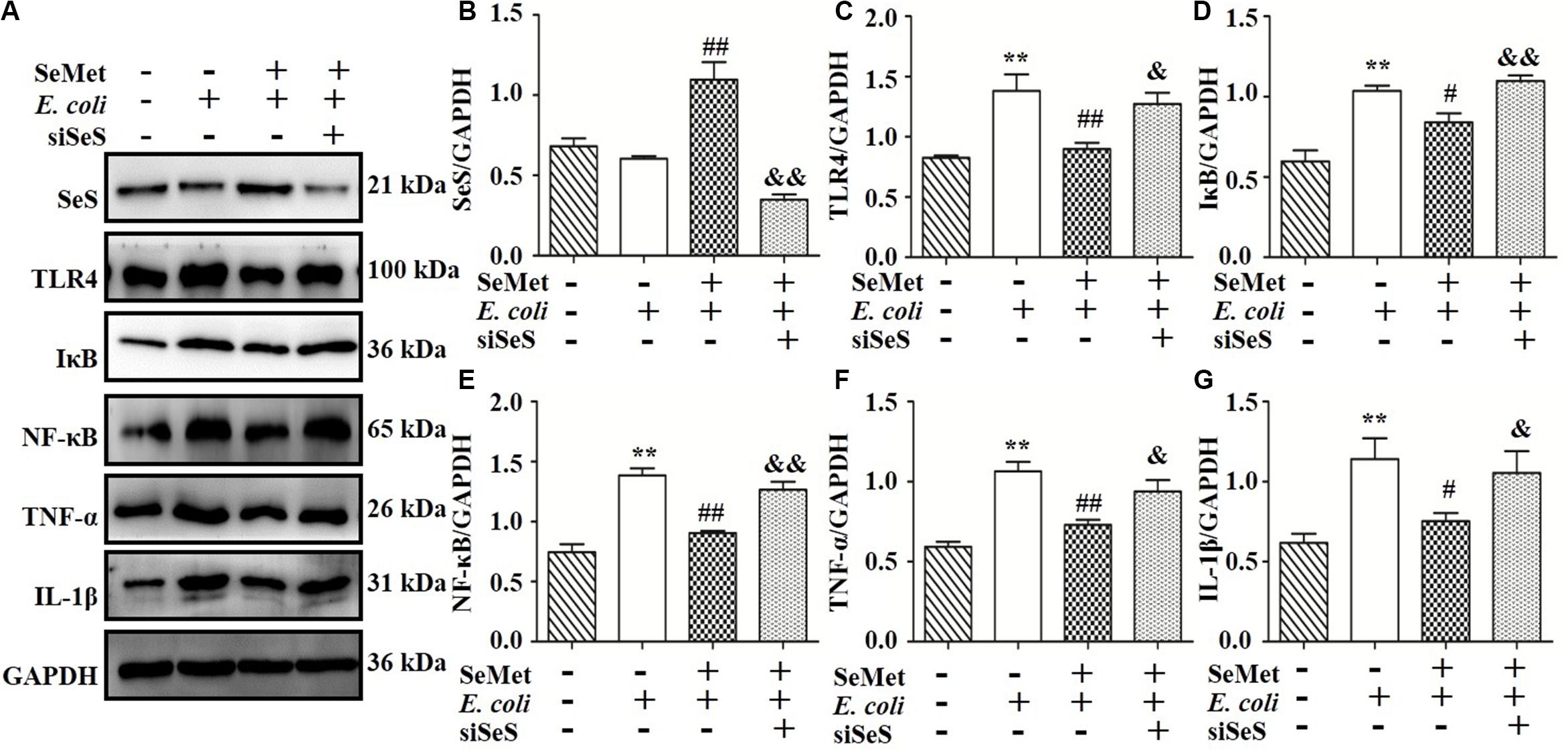
Figure 6. Knockdown of SeS suppressed the modulation of SeMet on TLR4/NF-κB pathway in macrophages infected with ESBL-E. coli. Macrophages were transfected with control siRNA or SeS siRNA. Subsequently, transfected cells were treated with CGA for 12 h, followed by ESBL-E. coli for 6 h. (A–G) Protein levels of SeS, TLR4, IκB, nuclear NF-κB, TNF-α, and IL-1β were determined by Western blotting. GAPDH was used as loading control. Data represent means ± SD of three independent experiments. In each independent experiment, there were two replicates per group with similar results. “–” and “+” after SeMet indicated that SeMet (40 μM) were not or were added, respectively. “–” and “+” after E. coli indicated that E. coli (MOI = 5) were not or were added. “–” and “+” after siSeS indicated that siSeS (50 nM) were not or were added. **p < 0.01, difference compared to control siRNA transfected samples; #p < 0.05 and ##p < 0.01 differences compared to ESBL E. coli-infected control siRNA transfected samples; &p < 0.05 and &&p < 0.01 differences compared to SeMet and ESBL E. coli-treated control siRNA transfected samples.
NF-κB Involved in SeMet-Regulated Inflammation Induced by ESBL-E. coli in bMECs and Macrophages
To further investigate whether NF-κB pathway is involved in regulation of SeMet on inflammation in ST410(4)-treated bMECs and macrophages, BAY 11-708 (a specific NF-κB inhibitor) was used to suppress NF-κB activation (Figures 7A,C, 8A,C). BAY 11-708 inhibited (p < 0.05) TNF-α (Figures 7A,D, 8A,D) and IL-1β (Figures 7A,E, 8A,E) protein levels in bMECs (Figure 7) and macrophages (Figure 8) after ST410(4) treatment. In addition, decreased NF-κB had no effect on SeS protein expression in bMECs or macrophages.
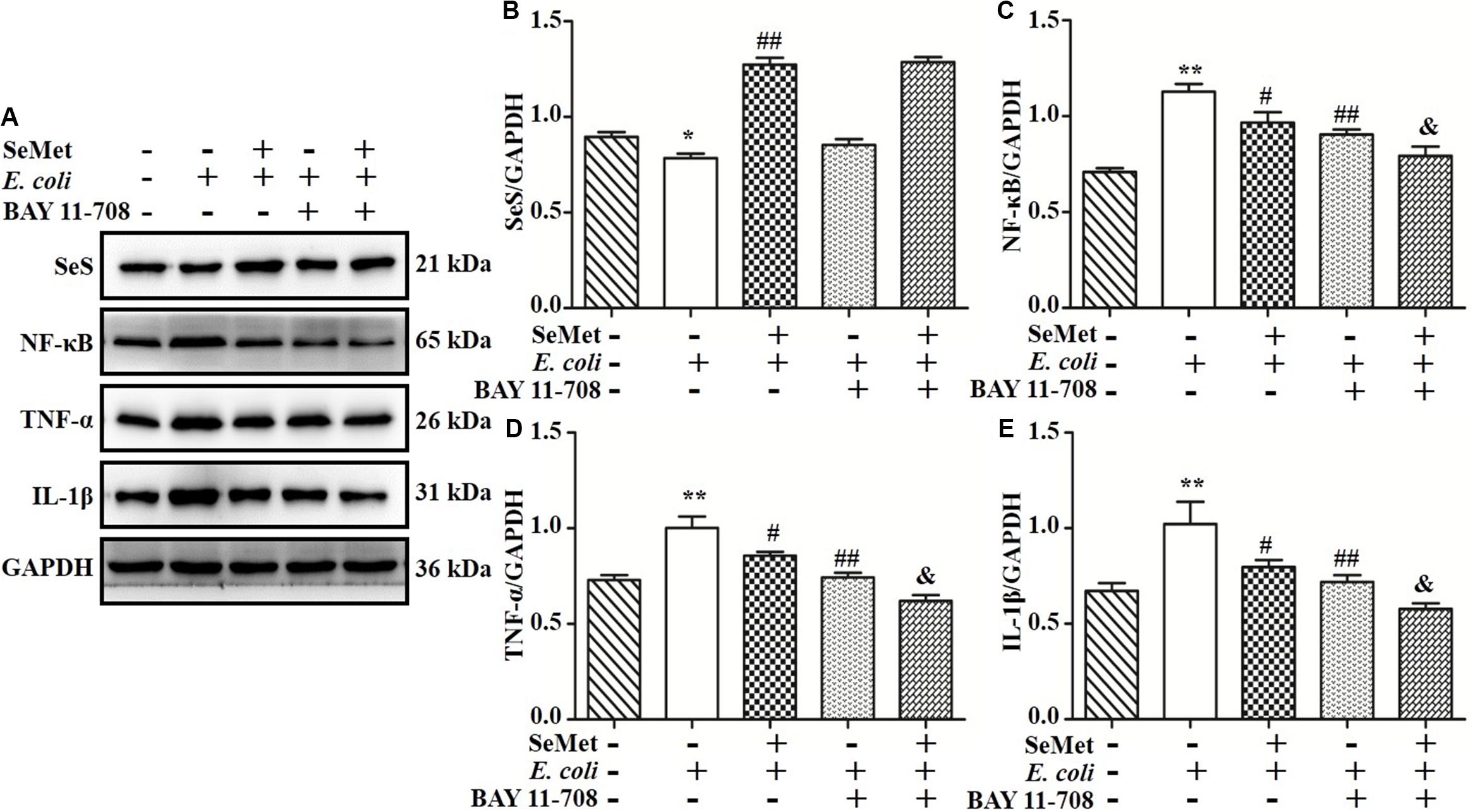
Figure 7. NF-κB mediated regulation of SeMet on inflammation induced by ESBL-E. coli in bMECs. The bMECs were incubated with BAY11-708 (10 μM) for 1 h. Subsequently, treated cells were exposed with SeMet for 12 h, followed by ESBL-E. coli for 4 h. (A–E) Protein levels of SeS, TNF-α, and IL-1β were determined by Western blotting. GAPDH was used as loading control. Data represent means ± SD of three independent experiments. In each independent experiment, there were two replicates per group with similar results. “–” and “+” after SeMet indicated that SeMet (20 μM) were not or were added, respectively. “–” and “+” after E. coli indicated that E. coli (MOI = 5) were not or were added. “–” and “+” after BAY 11-708 indicated that BAY 11-708 (10 μM) were not or were added. *p < 0.05 and **p < 0.01, differences compared to the control group; #p < 0.05 and ##p < 0.01 differences compared to ESBL E. coli-infected samples; &p < 0.05 differences compared to SeMet and ESBL E. coli-treated samples.
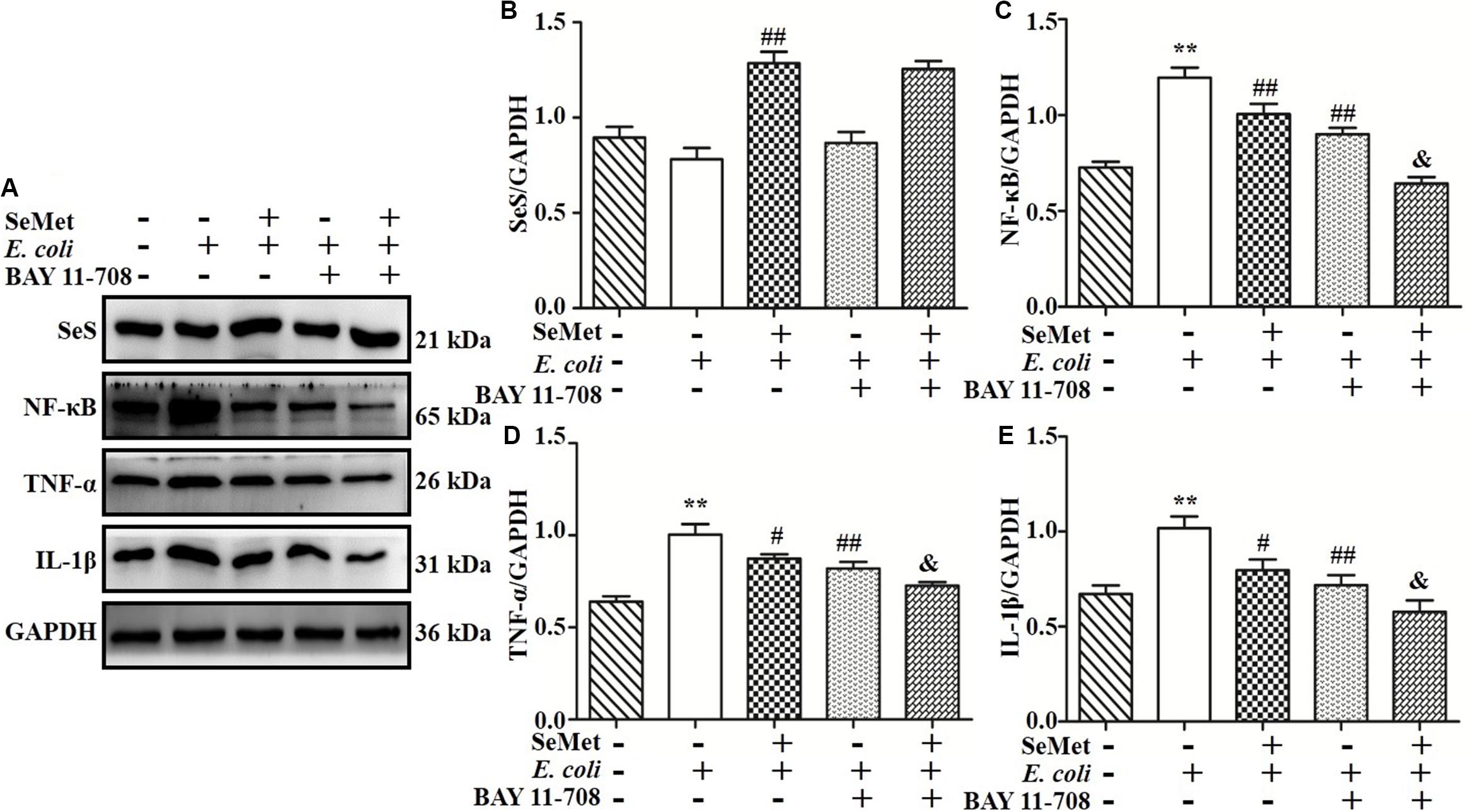
Figure 8. NF-κB mediated decrease of SeMet-induced inflammation in macrophages infected by ESBL-E. coli. Macrophages were incubated with BAY11-708 (20 μM) for 1 h. Subsequently, treated cells were exposed with SeMet for 12 h, followed by ESBL-E. coli for 4 h. (A–E) Protein levels of SeS, TNF-α, and IL-1β were determined by Western blotting. GAPDH was used as loading control. Data represent means ± SD of three independent experiments. In each independent experiment, there were two replicates per group with similar results. “–” and “+” after SeMet indicated that SeMet (40 μM) were not or were added, respectively. “–” and “+” after E. coli indicated that E. coli (MOI = 5) were not or were added. “–” and “+” after BAY 11-708 indicated that BAY 11-708 (20 μM) were not or were added. **p < 0.01, differences compared to control group; #p < 0.05 and ##p < 0.01 differences compared to ESBL E. coli-infected samples; and &p < 0.05 difference compared to SeMet and ESBL E. coli-treated samples.
Discussion
Our aim was to characterize how SeMet protected bMECs and macrophages against inflammation induced by ESBL-E. coli infection through modulation of the TLR4/NF-κB signaling pathway. The ESBL-E. coli strain ST410(4) isolated from a cow with mastitis enhanced LDH release and protein expression of TLR4, IκB, NF-κB, TNF-α, and IL-1β, whereas SeMet attenuated inflammation in ESBL E. coli-treated bMECs and macrophages. In addition, siSeS and BAY 11-708, a NF-κB inhibitor, suppressed NF-κB, TNF-α, and IL-1β expression.
In this study, 40, and/or 60 μM SeMet significantly increased cell viability and inhibited LDH release in bMECs and macrophages, respectively. In contrast, 80 and 100 μM SeMet significantly damaged bMECs and macrophages at 12 h. Therefore, we inferred that although SeMet had protective effects on bMECs (up to 40 μM), and macrophages (up to 60 μM), higher concentrations (i.e., 80 and 100 μM SeMet) had toxic effects on bMECs and macrophages, consistent with another report (Kiełczykowska et al., 2018). In addition, Bi et al. (2016) reported that 4 to 128 μM sodium selenite decreased cell viability in RAW264.7 macrophages at 12 h. Pretreatment of bMECs with 10 μM selenite methionine or 1 μM sodium selenite best rescued cell viability in heat shock-treated bMECs (83.1 and 81.4%, respectively; Zou et al., 2019). This discrepancy might be due to absorptive mechanisms, bioavailability, and toxicity of various Se. In that regard, organic Se has higher bioavailability and less toxicity to animals than inorganic Se (Kim and Mahan, 2001; Weiss and Hogan, 2005). In the current study, SeMet supplementation in advance decreased LDH activities induced by ESBL-producing E. coli in bMECs and macrophages. LDH, as a glycolytic enzyme, is abundant and present in most types of mammalian cells; therefore, increased LDH release indicates that cell membranes were damaged (Jin et al., 2013). Thus, we inferred that ESBL-E. coli damaged cell membranes, whereas SeMet prevented membrane damage in bMECs and macrophages.
Infection of bMECs and macrophages with E. coli incited a rapid inflammatory response, characterized by release of a large number of pro-inflammatory cytokines. Such cytokines activate immune effector cells to eliminate invading pathogen during the first stage (Porcherie et al., 2012; Aribi et al., 2015). Significant increases in TNF-α and IL-1β, important pro-inflammatory cytokines, were induced by E. coli in the current study, indicating a profound inflammatory response in bMECs and macrophages. However, expressions of pro-inflammatory cytokines must be tightly regulated and compartmentalized to prevent overexpression of these molecules, with potential for chronic inflammation and tissue injury (Scheibel et al., 2010; Awada et al., 2014). In this study, SeMet inhibited E. coli-induced protein expression of TNF-α and IL-1β, indicating that SeMet suppressed inflammation induced by E. coli infection in bMECs and macrophages and inhibited the cytokine storm.
Activation of signaling pathways of inflammation has an important role in regulating immune responses (Bi et al., 2016). For example, E. coli activated the TLR4/NF-κB signaling pathway in the udder (Yang et al., 2008). Based on significantly increased TLR4, IκB, TNF-α, IL-1β protein expressions, and NF-κB nuclear protein expression induced by E. coli, we inferred that it activated TLR4/NF-κB signaling pathways in bMECs and macrophages, consistent with E. coli causing mastitis through the TLR4/NF-κB pathway (Ma et al., 2019). Various concentrations of SeMet treatment enhanced SeS protein expression, whereas decreased TLR4, IκB, TNF-α, IL-1β protein expressions, and NF-κB nuclear protein expression in bMECs and macrophages were consistent with a selenium supplement inhibiting LPS-induced inflammatory cytokines through the NF-κB signaling pathway in murine mammary epithelial cells (Zhang et al., 2014). In addition, Se deficiency facilitates inflammation following Staphylococcus aureus infection by regulating TLR2/NF-κB pathway in the murine mammary gland and macrophages (Gao et al., 2016; Xu et al., 2020). Thus, we inferred that SeMet promoted SeS expression and relieved E. coli-inflammatory responses through TLR4-mediated NF-κB signaling pathway in bMECs and macrophages in this study.
Selenoprotein S, a member of the selenoprotein family, is expressed in multiple tissues and is involved in cellular stress responses and immune and inflammatory processes (Santos et al., 2019). In this study, knockdown of SeS by siRNA partially reversed E. coli-induced increases in TLR4, IKB, NF-κB, TNF-α, and IL-1β. Therefore, we concluded that knockdown of SeS inhibited TNF-α and IL-1β expression by mediation of TLR4/NF-κB signaling pathway. Interestingly, the present study clarified that SeMet did not suppress TLR4, IκB, NF-κB, TNF-α, and IL-1β expressions in bMECs and macrophages with SeS depletion. Thus, SeS has an important role in SeMet mediation of the TLR4/NF-κB pathway and in E. coli-induced inflammation in bMECs and macrophages. Furthermore, BAY11-708, a NF-κB inhibitor, did not affect SeS expression, and suppressed TNF-α and IL-1β expression in the presence of E. coli and/or SeMet, indicating that both SeS and inhibited NF-κB reduced E. coli-induced inflammatory response. In addition, although SeS is a target gene of NF-κB (Gao et al., 2006), additional supplemental Se further enhanced SeS expression and the increased SeS had a negative feedback effect on NF-κB activation. Thus, SeMet promoted SeS expression and inhibited E. coli-induced inflammation through the TLR4/NF-κB signaling pathway.
Interestingly, SeMet significantly promoted protein expressions of TLR4-mediated NF-κB signaling pathway in bMECs for 4 h, whereas those protein expressions significantly increased in macrophages for 6 h. Perhaps this discrepancy was due to E. coli first invading bMECs and subsequently promoting expression of chemotactic factors. Furthermore, those chemotactic factors recruited, and activated macrophages (Swamydas et al., 2015) that phagocytose pathogenic bacteria (e.g., E. coli) and mount a partial inflammatory response (Feng et al., 2008). In addition, under physiological conditions, NF-κB is present in the cytoplasm as an inactive heterotrimer consisting of p50, p65, and IκB. However, when activated by various carcinogens, tumor promoters, or proinflammatory agents, ubiquitination and degradation of IκBα promoted NF-κB (p65 subunit) release from the cytoplasm to the nucleus (Alok and Bharat, 2002). However, E. coli significantly increased IκB and NF-κB protein expressions in this study. This apparent discrepancy may have been due to increased nuclear NF-κB driving IκB expression, generating a negative feedback loop (Hayden and Ghosh, 2008). Moreover, SeMet treatment significantly decreased IκB and NF-κB protein expressions in this study, indicating that SeMet may have enhanced the negative feedback effect of NF-κB activation on IκB expression. However, the mechanism by which SeMet inhibited NF-κB expression with decreased IκB expression remains unclear and warrants further study.
Conclusion
Extended-spectrum β-lactamase E. coli induced expressions of chemotactic factors in bMECs and then recruited and activated macrophages. In addition, SeMet attenuated ESBL E. coli-induced inflammation through activated SeS-mediated TLR4/NF-κB signaling pathway in bMECs and macrophages (Figure 9), which provided experimental evidence for cytoprotective roles of Se.
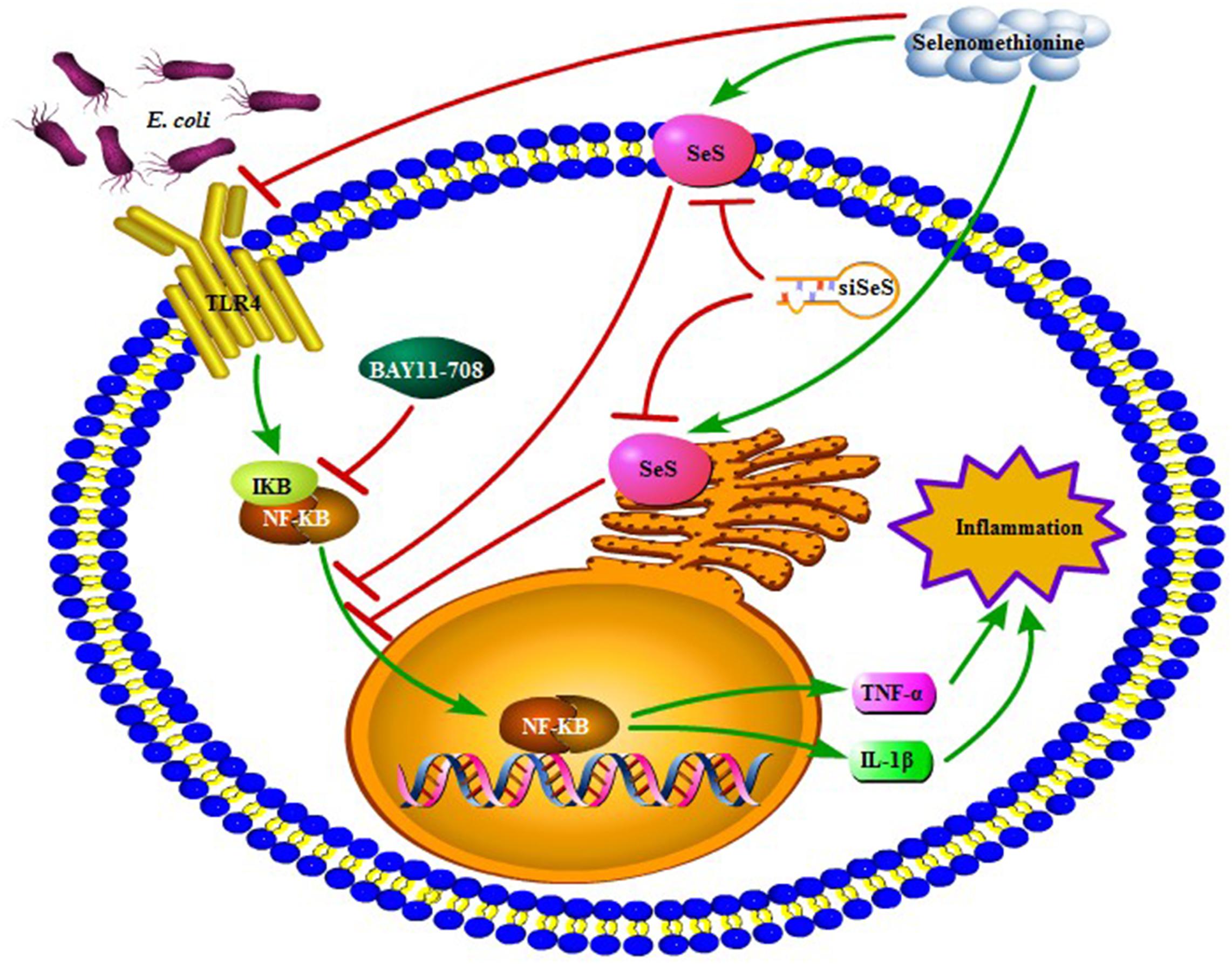
Figure 9. A proposed signaling pathway involved in SeMet against ESBL E. coli-induced inflammation. Note that SeMet protects bMECs and macrophages against inflammation by inhibition of TLR4/NF-κB signaling pathway. Meanwhile, SeMet suppressed NF-κB pathway through SeS and inhibited NF-κB-reduced inflammation. Green arrow and red bar indicate stimulation and inhibition, respectively.
Data Availability Statement
The raw data supporting the conclusions of this article will be made available by the authors, without undue reservation, to any qualified researcher.
Author Contributions
BH conceived and designed the experiments. CZ, GL, MZ, SX, and JG performed the experiments, analyzed the data, and contributed to drafting the manuscript. SR, YL, and GL analyzed the data, participated in the preparation of the manuscript, and analyzed the results. CZ, HB, and JK wrote, reviewed, and edited the manuscript. All authors approved the final version of the manuscript.
Funding
This study was financially supported by the National Natural Science Foundation of China (Nos. 31550110200 and 31772813), the National Key R&D Project (No. 2016YFD0501203), the High-end Foreign Experts Recruitment Program (No. GDT20171100013), the National Dairy Industry and Technology System (CARS-36), Hebei Key R&D Project (19226607D), and Ningxia Key R&D Project (No. 2019BBF02027).
Conflict of Interest
The authors declare that the research was conducted in the absence of any commercial or financial relationships that could be construed as a potential conflict of interest.
Supplementary Material
The Supplementary Material for this article can be found online at: https://www.frontiersin.org/articles/10.3389/fmicb.2020.01461/full#supplementary-material
References
Ali, T., Rahman, S., Zhang, L., Shahid, M., Han, D., Gao, J., et al. (2017). Characteristics and genetic diversity of multi-drug resistant extended-spectrum beta-lactamase (ESBL)-producing Escherichia coli isolated from bovine mastitis. Oncotarget 8:90144. doi: 10.18632/oncotarget.21496
Ali, T., Rahman, S., Zhang, L., Shahid, M., Zhang, S., Liu, G., et al. (2016). ESBL-producing Escherichia coli from cows suffering mastitis in China contain clinical class 1 integrons with CTX-M linked to ISCR1. Front. Microbiol. 7:1931. doi: 10.3389/fmicb.2016.01931
Alok, C. B., and Bharat, B. A. (2002). Nuclear factor-kappa B and cancer: its role in prevention and therapy. Biochem. Pharmacol. 64, 883–888. doi: 10.1016/s0006-2952(02)01154-1
Aribi, M., Meziane, W., Habi, S., Boulatika, Y., Marchandin, H., and Aymeric, J. L. (2015). Macrophage bactericidal activities against Staphylococcus aureus are enhanced in vivo by selenium supplementation in a dose-dependent manner. PLoS One 10:e0135515. doi: 10.1371/journal.pone.0135515
Awada, R., Saulnier-Blache, J. S., Grès, S., Bourdon, E., Rondeau, P., Parimisetty, A., et al. (2014). Autotaxin downregulates LPS-induced microglia activation and pro-inflammatory cytokines production. J. Cell Biochem. 115, 2123–2132. doi: 10.1002/jcb.24889
Bi, C. L., Wang, H., Wang, Y. J., Sun, J., Dong, J. S., Meng, X., et al. (2016). Selenium inhibits Staphylococcus aureus-induced inflammation by suppressing the activation of the NF-κB and MAPK signalling pathways in RAW264.7 macrophages. Eur. J. Pharmacol. 780, 159–165. doi: 10.1016/j.ejphar.2016.03.044
Bougarn, S., Cunha, P., Gilbert, F. B., Meurens, F., and Rainard, P. (2011). Technical note: validation of candidate reference genes for normalization of quantitative PCR in bovine mammary epithelial cells responding to inflammatory stimuli. J. Dairy Sci. 94, 2425–2430. doi: 10.3168/jds.2010-3859
Cebra, C. K., Heidel, J. R., Crisman, R. O., and Stang, B. V. (2003). The relationship between endogenous cortisol, blood micronutrients, and neutrophil function in postparturient holstein cows. J. Vet. Intern. Med. 17, 902–907. doi: 10.1111/j.1939-1676.2003.tb02531.x
Feng, R., Desbordes, S. C., Xie, H., Tillo, E. S., Pixley, F., Stanley, E. R., et al. (2008). PU.1 and C/EBPalpha/beta convert fibroblasts into macrophage-like cells. Proc. Natl. Acad. Sci. USA. 105, 57–62. doi: 10.1073/pnas.0711961105
Gabel-Jensen, C., and Gammelgaard, B. (2010). Selenium metabolism in hepatocytes incubated with selenite, selenate, selenomethionine, Se-methylselenocysteine and methylseleninc acid and analysed by LC-ICP-MS. J. Anal. Atom. Spectrom. 25:414. doi: 10.1039/b921365a
Gao, J., Barkema, H. W., Zhang, L., Liu, G., Deng, Z., Cai, L., et al. (2017). Incidence of clinical mastitis and distribution of pathogens on large Chinese dairy farms. J. Dairy Sci. 100, 4797–4806. doi: 10.3168/jds.2016-12334
Gao, X., Zhang, Z., Li, Y., Shen, P., Hu, X., Cao, Y., et al. (2016). Selenium deficiency facilitates inflammation following S. aureus infection by regulating TLR2-related pathways in the mouse mammary gland. Biol. Trace Elem. Res. 172, 49–57. doi: 10.1007/s12011-015-0614-y
Gao, Y., Hannan, N. R., Wanyonyi, S., Konstantopolous, N., Pagnon, J., Feng, H. C., et al. (2006). Activation of the selenoprotein SEPS1 gene expression by pro-inflammatory cytokines in HepG2 cells. Cytokine 33, 246–251. doi: 10.1016/j.cyto.2006.02.005
Gilbert, F. B., Cunha, P., Jensen, K., Glass, E. J., Foucras, G., Robert-Granié, C., et al. (2013). Differential response of bovine mammary epithelial cells to Staphylococcus aureus or Escherichia coli agonists of the innate immune system. Vet. Res. 44:40. doi: 10.1016/j.domaniend.2005.02.016
Han, D., Gao, J., Gu, X., Hengstler, J. G., Zhang, L., Shahid, M., et al. (2018). P21Waf1/Cip1 depletion promotes dexamethasone-induced apoptosis in osteoblastic MC3T3-E1 cells by inhibiting the Nrf2/HO-1 pathway. Arch. Toxicol. 92, 679–692. doi: 10.1007/s00204-017-2070-2
Hayden, M. S., and Ghosh, S. (2008). Shared principles in NF-κB signaling. Cell 132:362. doi: 10.1016/j.cell.2008.01.020
Jin, Y., Ye, X., Shao, L., Lin, B. C., He, C. X., Zhang, B. B., et al. (2013). Serum lactic dehydrogenase strongly predicts survival in metastatic nasopharyngeal carcinoma treated with palliative chemotherapy. Eur. J. Cancer 49, 1619–1626. doi: 10.1016/j.ejca.2012.11.032
Kaushal, N., Kudva, A. K., Patterson, A. D., Chiaro, C., Kennett, M. J., Desal, D., et al. (2014). Crucial role of macrophage selenoproteins in experimental colitis. J. Immunol. 193, 3683–3692. doi: 10.4049/jimmunol.1400347
Kiełczykowska, M., Kocot, J., Paździor, M., and Musik, I. (2018). Selenium - a fascinating antioxidant of protective properties. Adv. Clin. Exp. Med. 27, 245–255. doi: 10.17219/acem/67222
Kim, Y. Y., and Mahan, D. C. (2001). Comparative effects of high dietary levels of organic and inorganic selenium on selenium toxicity of growing-finishing pigs. J. Anim. Sci. 79, 942–948. doi: 10.2527/2001.794942x
Kubachka, K. M., Hanley, T., Mantha, M., Wilson, R. A., Falconer, T. M., Kassa, Z., et al. (2017). Evaluation of selenium in dietary supplements using elemental speciation. Food Chem. 218, 313–320. doi: 10.1016/j.foodchem.2016.08.086
Lecoq, L., Raiola, L., Chabot, P. R., Cyr, N., Arseneault, G., Legault, P., et al. (2017). Structural characterization of interactions between transactivation domain 1 of the p65 subunit of NF-κB and transcription regulatory factors. Nucleic Acids Res. 45, 5564–5576. doi: 10.1093/nar/gkx146
Liu, Y., Liu, G., Liu, W., Liu, Y., Ali, T., Chen, W., et al. (2014). Phylogenetic group, virulence factors and antimicrobial resistance of Escherichia coli associated with bovine mastitis. Res. Microbiol. 165, 273–277. doi: 10.1016/j.resmic.2014.03.007
Ma, N., Chang, G., Huang, J., Wang, Y., Gao, Q., and Cheng, X. (2019). cis-9, trans-11-conjugated linoleic acid exerts an anti-inflammatory effect in bovine mammary epithelial cells after Escherichia coli stimulation through NF-κB signaling pathway. J. Agric. Food Chem. 67, 193–200. doi: 10.1021/acs.jafc.8b05500
Mukherjee, M., Basu, S., Mukherjee, S. K., and Majumder, M. (2013). Multidrug-resistance and extended spectrum beta-lactamase production in uropathogenic E. coli which were isolated from hospitalized patients in Kolkata, India. J. Clin. Diagn. Res. 7, 449–453. doi: 10.7860/JCDR/2013/4990.2796
Nakase, J., Ukawa, Y., Takemoto, S., Kubo, T., Sagesaka, Y. M., Aoki-Yoshida, A., et al. (2017). RNA and a cell wall component of Enterococcus faecalis IC-1 are required for phagocytosis and interleukin 12 production by the mouse macrophage cell line J774.1. Biosci. Biotechnol. Biochem. 81, 1099–1105. doi: 10.1080/09168451.2017
Olde Riekerink, R. G., Barkema, H. W., Kelton, D. F., and Scholl, D. T. (2008). Incidence rate of clinical mastitis on Canadian dairy farms. J. Dairy Sci. 91, 1366–1377. doi: 10.3168/jds.2007-0757
Porcherie, A., Cunha, P., Trorereau, A., Roussel, P., Gilbert, F. B., and Rainard, P. (2012). Repertoire of Escherichia coli agonists sensed by innate immunity receptors of the bovine udder and mammary epithelial cells. Vet. Res. 43, 14–21. doi: 10.1186/1297-9716-43-14
Salman, S., Khol-Parisini, A., Schafft, H., Lahrssen-Wiederholt, M., Hulan, H. W., Dinse, D., et al. (2009). The role of dietary selenium in bovine mammary gland health and immune function. Anim. Health Res. Rev. 10, 21–34. doi: 10.1017/S1466252308001588
Santos, L. R., Durães, C., Ziros, P. G., Pestana, A., Esteves, C., Neves, C., et al. (2019). Interaction of genetic variations in NFE2L2 and SELENOS modulates the risk of Hashimoto’s thyroiditis. Thyroid 29, 1302–1315. doi: 10.1089/thy.2018.0480
Scheibel, M., Klein, B., Merkle, H., Schulz, M., Fritsch, R., Greten, F. R., et al. (2010). IκBβ is an essential co-activator for LPS-induced IL-1β transcription in vivo. J. Exp. Med. 207, 2621–2630. doi: 10.1084/jem.20100864
Shahid, M., Gao, J., Zhou, Y., Liu, G., Ali, T., Deng, Y., et al. (2017). Prototheca zopfii isolated from bovine mastitis induced oxidative stress and apoptosis in bovine mammary epithelial cells. Oncotarget 8:31938. doi: 10.18632/oncotarget.16653
Shimohashi, N., Nakamuta, M., Uchimura, K., Sugimoto, R., Lwamoto, H., Enjoji, M., et al. (2000). Selenoorganic compound, ebselen, inhibits nitric oxide and tumor necrosis factor- α production by the modulation of jun-N-terminal kinase and NF-κB signaling pathway in rat kupffer cells. J. Cell. Biochem. 78, 595–606. doi: 10.1002/1097-4644(20000915)78:43.0.CO;2-B
Swamydas, M., Break, T. J., and Lionakis, M. S. (2015). Mononuclear phagocyte-mediated antifungal immunity: the role of chemotactic receptors and ligands. Cell. Mol. Life Sci. 72, 2157–2175. doi: 10.1007/s00018-015-1858-6
Vangroenweghe, F., Lamote, I., and Burvenich, C. (2005). Physiology of the periparturient period and its relation to severity of clinical mastitis. Domest. Anim. Endocrinol. 29, 283–293.
Vural, A., and Kehrl, J. H. (2014). Autophagy in macrophages: impacting inflammation and bacterial infection. Scientifica 2014:825463. doi: 10.1155/2014/825463
Weiss, W. P., and Hogan, J. S. (2005). Effect of selenium source on selenium status, neutrophil function, and response to intramammary endotoxin challenge of dairy cows. J. Dairy Sci. 88, 4366–4374. doi: 10.3168/jds.S0022-0302(05)73123-4
Xu, J., Gong, Y., Sun, Y., Cai, J., Liu, Q., Bao, J., et al. (2020). Impact of selenium deficiency on inflammation, oxidative stress, and phagocytosis in mouse macrophages. Biol. Trace Elem. Res. 194, 237–243. doi: 10.1007/s12011-019-01775-7
Yang, W., Zerbe, H., Petzl, W., Brunner, R. M., Günther, J., Draing, C., et al. (2008). Bovine TLR2 and TLR4 properly transduce signals from Staphylococcus aureus and E. coli, but S. aureus fails to both activate NF-kappaB in mammary epithelial cells and to quickly induce TNFalpha and interleukin-8 (CXCL8) expression in the udder. Mol. Immunol. 45, 1385–1397. doi: 10.1016/j.molimm.2007.09.004
Zhang, W., Zhang, R. X., Wang, T. C., Jiang, H. C., Guo, M. Y., Zhou, E. S., et al. (2014). Selenium inhibits LPS-induced pro-inflammatory gene expression by modulating MAPK and NF-κB signaling pathways in mouse mammary epithelial cells in primary culture. Inflammation 37, 478–485. doi: 10.1007/s10753-013-9761-5
Keywords: selenomethionine, ESBL-Escherichia coli, bovine mastitis, inflammation, selenoprotein S, TLR4/NF-κB pathway
Citation: Zhuang C, Liu G, Barkema HW, Zhou M, Xu S, ur Rahman S, Liu Y, Kastelic JP, Gao J and Han B (2020) Selenomethionine Suppressed TLR4/NF-κB Pathway by Activating Selenoprotein S to Alleviate ESBL Escherichia coli-Induced Inflammation in Bovine Mammary Epithelial Cells and Macrophages. Front. Microbiol. 11:1461. doi: 10.3389/fmicb.2020.01461
Received: 08 February 2020; Accepted: 04 June 2020;
Published: 08 July 2020.
Edited by:
Esaki M. Shankar, Central University of Tamil Nadu, IndiaReviewed by:
Chaoxin Man, Northeast Agricultural University, ChinaSridhar Muthusami, Karpagam Academy of Higher Education, India
Vignesh Ramachandran, University of Kuala Lumpur, Malaysia
Copyright © 2020 Zhuang, Liu, Barkema, Zhou, Xu, ur Rahman, Liu, Kastelic, Gao and Han. This is an open-access article distributed under the terms of the Creative Commons Attribution License (CC BY). The use, distribution or reproduction in other forums is permitted, provided the original author(s) and the copyright owner(s) are credited and that the original publication in this journal is cited, in accordance with accepted academic practice. No use, distribution or reproduction is permitted which does not comply with these terms.
*Correspondence: Jian Gao, gaojian2016@cau.edu.cn; Bo Han, hanbo@cau.edu.cn