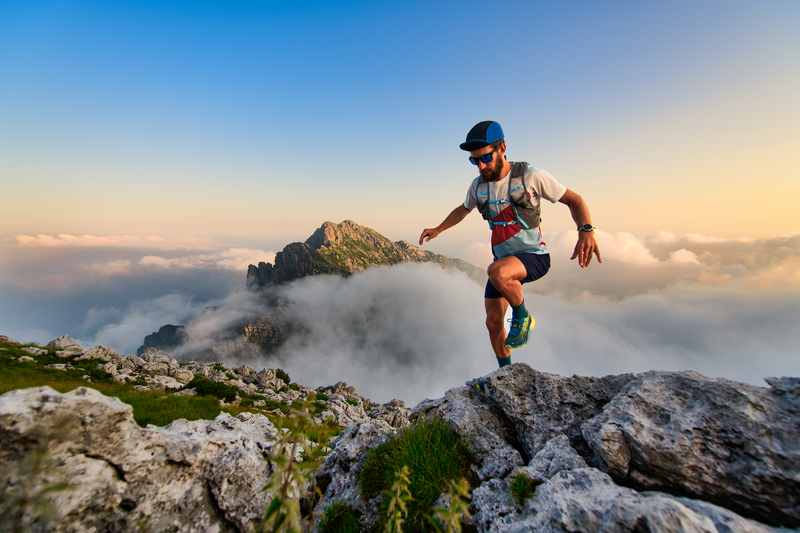
94% of researchers rate our articles as excellent or good
Learn more about the work of our research integrity team to safeguard the quality of each article we publish.
Find out more
ORIGINAL RESEARCH article
Front. Microbiol. , 26 June 2020
Sec. Antimicrobials, Resistance and Chemotherapy
Volume 11 - 2020 | https://doi.org/10.3389/fmicb.2020.01438
The spread of antibiotic resistance is a major public health concern worldwide. Commensal bacteria from the human genitourinary tract can act as reservoirs of resistance genes playing a role in their transfer to pathogens. In this study, the minimum inhibitory concentration of 16 antibiotics to 15 isolates from the human vagina, identified as Enterococcus faecalis, Streptococcus anginosus, and Streptococcus salivarius, was determined. Eight isolates were considered resistant to tetracycline, five to clindamycin and quinupristin-dalfopristin, and four to rifampicin. To investigate the presence of antimicrobial resistance genes, PCR analysis was performed in all isolates, and five were subjected to whole-genome sequencing analysis. PCR reactions identified tet(M) in all tetracycline-resistant E. faecalis isolates, while both tet(M) and tet(L) were found in tetracycline-resistant S. anginosus isolates. The tet(M) gene in E. faecalis VA02-2 was carried within an entire copy of the transposon Tn916. In S. anginosus VA01-10AN and VA01-14AN, the tet(M) and tet(L) genes were found contiguous with one another and flanked by genes encoding DNA mobilization and plasmid replication proteins. Amplification and sequencing suggested the lsaA gene to be complete in all E. faecalis isolates resistant to clindamycin and quinupristin-dalfopristin, while the gene contain mutations rendering to a non-functional LsaA in susceptible isolates. These results were subsequently confirmed by genome analysis of clindamycin and quinupristin-dalfopristin resistant and susceptible E. faecalis strains. Although a clinical breakpoint to kanamycin for S. salivarius has yet to be established, S. salivarius VA08-2AN showed an MIC to this antibiotic of 128 μg mL–1. However, genes involved in kanamycin resistance were not identified. Under the assayed conditions, neither tet(L) nor tet(M) from either E. faecalis or S. anginosus was transferred by conjugation to recipient strains of E. faecalis, Lactococcus lactis, or Lactobacillus plantarum. Nonetheless, the tet(L) gene from S. anginosus VA01-10AN was amplified by PCR, and cloned and expressed in Escherichia coli, to which it provided a resistance of 48–64 μg mL–1 to tetracycline. Our results expand the knowledge of the antibiotic resistance-susceptibility profiles of vaginal bacteria and provide the genetic basis of their intrinsic and acquired resistance.
The indiscriminate use of antibiotics in the treatment of infections and as prophylactic agents has potentiated the emergence and spread of different types of antibiotic resistance (Bengtsson-Palme et al., 2018). Antibiotic-resistant bacteria pose an increasingly important public health challenge worldwide. When resistance to an antibiotic is inherent to all strains of a bacterial species, it is generally referred to as intrinsic resistance. In contrast, when a strain of a typically susceptible species is resistant to a given antibiotic, it is considered to be acquired resistance (EFSA FEEDAP Panel, 2012). A bacterial species may have intrinsic resistance to an antibiotic due to the lack of target, the possession of low-affinity targets, cell impermeability to the antibiotic, or the existence of multidrug efflux mechanisms that excrete it (Cox and Wright, 2013). The acquisition of resistance occurs via genetic mutation or by antimicrobial resistance (AMR) gene gain via horizontal transfer. Transference is fueled by the presence of AMR genes in conjugative and mobilizable genetic elements, such as plasmids, transposons, or integrative and conjugative elements (van Hoek et al., 2011). Currently, there is a pressing need to limit the spread of such genes, which could be transferred to opportunistic and pathogenic bacteria (Peterson and Kaur, 2018).
The vagina represents a complex ecosystem in which many microbial species occur in varying numbers and proportions (Huang et al., 2014). Microorganisms exist in a finely tuned mutualistic relationship with the host, and provide the first line of defense against colonization and infection by pathogens (Smith and Ravel, 2017). The dominant bacterial species in human vagina belong to the genus Lactobacillus (Martin, 2012; Sirichoat et al., 2018). However, species of other genera, such as Gardnerella, Atopobium, Prevotella, Corynebacterium, Anaerococcus, Peptoniphilus, can, under certain conditions, constitute majority populations (Martin, 2012; Sirichoat et al., 2018). Species of Enterococcus and Streptococcus genera are frequently isolated as subdominant populations. Lactic acid-producing strains of these biotypes might possibly be used as vaginal probiotics to prevent or treat vaginal infections by inhibiting the development of pathogens (Nami et al., 2014). However, some strains of these genera are also known to be opportunistic pathogens causing occasional disease (Krzyściak et al., 2013; Ben Braïek and Smaoui, 2019). Further, enterococci are well-known reservoirs of antibiotic resistance genes, as they harbor abundant conjugative plasmids and transposons that might be broadly transferred to other bacteria (Miller et al., 2014). The intra- and inter-genus transfer of AMR genes from and to streptococci has been documented as well (Chajêcka-Wierzchowska et al., 2019). Indeed, it is known that the transfer of AMR genes between bacterial species does occur in the vagina (Nogacka et al., 2017). It is for these reasons that enterococci and streptococci -with the exception of Streptococcus thermophilus- have been refused Generally Regarded as Safety (GRAS) status by the US Food and Drug Administration (FDA, 2010), and are not recommended for the Qualified Presumption of Safety (QPS) by the European Food Safety Authority (EFSA) (EFSA BIOHAZ Panel, 2017).
While a number of studies have been conducted on antibiotic resistance in pathogens such as Gardnerella vaginalis, group B streptococci (Nagaraja, 2008; Bolukaoto et al., 2015), and lactic acid bacteria (LAB) from the human vagina (Martín et al., 2008; Fuochi et al., 2019), the antibiotic resistance-susceptibility profiles of other vaginal-dwelling cocci have yet to be determined. The aim of the present work was to examine the antibiotic resistance/susceptibility profiles of a set of cocci isolates recovered from the vagina of healthy Thai women, and to investigate by PCR and whole genome sequencing and analysis the genetic basis of the intrinsic and acquired resistances identified. The capacity of some resistances to undergo horizontal transfer in vitro was also assessed.
This study was approved by the Khon Kaen Ethics Committee in Human Research (Ref. HE581191). The women of this study were selected among patients attending the gynecological clinic of Srinagarind Hospital, Faculty of Medicine, Khon Kaen University (Khon Kaen, Thailand). To be eligible, women had to be 20–45 years old, not be pregnant, have regular menstruation, have no serious underlying disease (e.g., diabetes mellitus or systemic lupus erythematous) and no clinical genitourinary symptoms on examination, and had no antibiotic treatment during the last 6 months. Before sampling, volunteers signed a written informed consent. Vaginal exudates were taken by swabbing the lateral, anterior and posterior vaginal walls with sterile cotton-tipped applicators (performed at the clinic); these were then placed in sterile recipients containing reduced Amies transport medium (Oxoid, Basingstoke, United Kingdom) and stored at 4°C until culturing later on the same day.
Vaginal swabs were suspended and serially diluted in de Man, Rogosa and Sharpe (MRS) broth (Oxoid), and the dilutions spread on MRS agar plates containing 0.5% (w/v) CaCO3 (BDH, Poole, United Kingdom). Plates were incubated at 37°C for 48 h in aerobic or anaerobic (with Anaerocult; Merck, Darmstadt, Germany) conditions. Individual colonies surrounded by clear halos from both culture conditions were randomly selected and subcultured in MRS and incubated under the same conditions. Isolates were then screened by Gram staining, colony morphology, and the catalase test. Their hemolytic activity was assessed using sheep blood agar plates (Oxoid). Only Gram-positive, catalase negative, and γ-hemolytic cocci were selected. These isolates were routinely cultivated in MRS agar and incubated at 37°C for 48 h, and then stored in MRS broth supplemented with 15% (w/v) glycerol (Merck) at −80°C.
Genomic DNA extraction and purification from the isolates was performed using the GenElute Bacterial Genomic DNA kit (Sigma-Aldrich, St. Louis, Mo., United States) following the manufacturer’s instructions. Purified DNA was used as a template for amplifying the 16S rRNA genes using the universal primers 27F (5′-AGAGTTTGATCCTGGCTCAG-3′) and 1492R (5′-GGTTACCTTGTTACGACTT-3′) (Frank et al., 2008) and Taq polymerase (Ampliqon, Odense, Denmark). The PCR conditions were as follows; one cycle of 95°C for 5 min, followed by 35 cycles of denaturation at 94°C for 30 s, primer annealing at 55°C for 45 s, and extension at 72°C for 2 min. A final extension step at 72°C for 10 min was then performed. Amplified products were checked by electrophoresis using 1% agarose gels, visualized after 90 min, and photographed under UV light using a G Box equipment (SynGene, Cambridge, United Kingdm). The amplified products were purified using a GenElute PCR Clean-Up Kit (Sigma-Aldrich) column, and subjected to standard Sanger DNA sequencing at Macrogen (Madrid, Spain). Strains were identified at the species level by comparing their sequences to those in the NCBI database using the BLAST tool (Altschul et al., 1997). Sequences sharing 97% identity or higher were deemed to belong to the same species.
Isolates were typed using random amplified polymorphic DNA-PCR (RAPD-PCR) and repetitive element-PCR (rep-PCR) fingerprinting methods, using primer M13 (5′-GAGGGTGGCGGTTCT-3′) as described by Rossetti and Giraffa (2005), primer BoxA2R (5′-ACGTGGTTTGAAGAGATTTTCG-3′) as described by Koeuth et al. (1995), and primer OPA18 (5′-AGGTGACCGT-3′) as described by Mättö et al. (2004). PCR reaction mixtures contained 2 μL of each purified genomic DNA (≈100 ng), 12.5 μL of 2 × Master Mix RED (Ampliqon), 5 μL of either primer (10 μM) and molecular biology grade water (Sigma-Aldrich) in a total volume of 25 μL. The PCR conditions were as follows; one cycle of 95°C for 7 min, followed by 40 cycles of denaturation at 95°C for 30 s, primer annealing at 42°C (M13), 40°C (BoxA2R) or 32°C (OPA18) for 1 min, and extension at 72°C for 4 min. A final extension step at 72°C for 10 min was then performed. Amplification products were separated by electrophoresis using 2.5% agarose gel and visualized as above. Pattern profiles were clustered and compared using the unweighted pair group method with arithmetic mean (UPGMA), and their similarity expressed via the simple matching (SM) coefficient in GeneTools v.4.03 (SynGene). Triplicate analysis by RAPD-PCR and rep-PCR techniques with all three primers identified a combined repeatability of the fingerprinting of 94%; consequently, profiles with <94% similarity were considered different strains.
The minimum inhibitory concentration (MIC) of 16 antibiotics was determined according to ISO standard 10932:2010 (IDF, 2010) using VetMIC plates (National Veterinary Institute of Sweden, Uppsala, Sweden). The plates contained twofold serial dilutions of the antibiotics gentamicin, kanamycin, streptomycin, neomycin, tetracycline, erythromycin, clindamycin, chloramphenicol, ampicillin, penicillin, vancomycin, quinupristin-dalfopristin, linezolid, trimethoprim, ciprofloxacin, or rifampicin. Individual colonies grown on Muller-Hinton agar plates (Oxoid) were suspended in 2 mL sterile saline (0.9% NaCl solution) to obtain a density corresponding to McFarland standard 1 (≈3 × 108 cfu mL–1). This suspension was further diluted 1:1000 in Muller-Hinton broth to achieve a final cell concentration of approximately 3 × 105 cfu mL–1. One-hundred microliters of this inoculum were then added to each well of the VetMIC plates. Following incubation for 48 h at 37°C, MICs were visually determined as the lowest antibiotic concentration at which growth was inhibited. The concentration range for clindamycin in the VetMIC plates was insufficient to determine the actual MIC for some isolates; this was determined using the MICE system (Oxoid) following the manufacturer’s recommendations. A strain was considered phenotypically resistant to an antibiotic when it was not inhibited by a concentration higher than the established clinical breakpoint retrieved from the CLSI guidelines (CLSI, 2019). When not covered, the microbiological cut-off values of the European Committee on Antimicrobial Susceptibility Testing (EUCAST) (EUCAST, 2019) were adopted.
Genes involved in tetracycline resistance were searched for by PCR using the degenerate primer pairs DI-DII and Tet1-Tet2 targeting genes encoding ribosomal protection proteins (RPPs). Specific primers were used for the detection of genes coding for resistance to tetracycline [tet(M), tet(O), tet(S), tet(W), tet(K), and tet(L)], erythromycin and clindamycin [erm(A), erm(B), erm(C), erm(F), and mef(A)], chloramphenicol (cat), β-lactam antibiotics (bla), aminoglycosides [aac(6’)-aph(2″) and aad(E)], clindamycin (lsaA), and vancomycin (vanA) (Supplementary Table 1). The reaction mixtures (50 μL) contained 25 μL of Taq 2 × Master Mix RED, 1.5 μL of each primer (10 μM), 2 μL of each purified genomic DNA (≈100 ng) and 20 μL of molecular biology grade water. The PCR conditions were as follows; initial denaturation at 94°C for 5 min, 35 cycles of 94°C for 1 min, an appropriate annealing temperature (Supplementary Table 1) for 1 min, 72°C for 2 min, and a final extension step at 72°C for 10 min. The amplified products were then electrophoresed, visualized, and recorded. Selected amplicons were purified and sequenced, and their sequences compared as above.
A library of 0.5 kbp was constructed from the total genomic DNA belonging to five vaginal E. faecalis and Streptococcus spp. strains, and paired-end sequenced using a Genome Sequencer Illumina HiSeq 1000 System at Eurofins Genomics (Konstanz, Germany). Quality-filtered reads were assembled in contigs using Spades v.3.6.2 software (Bankevich et al., 2012). Genomes were annotated using the RAST annotation system (Aziz et al., 2008) and the NCBI Prokaryotic Genome Annotation Pipeline (Tatusova et al., 2016). DNA and deduced protein sequences of interest were examined for homology using the on-line BLAST tool as above. The homology of genes and proteins involved in antimicrobial resistance was further investigated by searching the CARD (McArthur et al., 2013), ResFinder (Zankari et al., 2012), and ARG-ANNOT (Gupta et al., 2014) databases.
Strains harboring tet(M) or both tet(M) and tet(L) were selected for filter mating conjugations, using as recipient strains Lactococcus lactis subsp. cremoris MG1614, L. lactis subsp. lactis biovar. diacetylactis Bu2-60, E. faecalis OG1RF and Lactobacillus plantarum NC8. All recipients were plasmid-free and susceptible to tetracycline. The two L. lactis strains were resistant to streptomycin (500 μg mL–1) and rifampicin (100 μg mL–1), E. faecalis OG1RF was resistant to rifampicin (100 μg mL–1) and fusidic acid (25 μg mL–1), and L. plantarum NC8 was resistant to streptomycin (500 μg mL–1).
Conjugation was performed by filter mating as previously described (Flórez et al., 2008) with minor modifications. Briefly, donor and recipient strains were grown separately overnight in M17 broth (Formedium, Hunstanton, United Kingdom) with 0.5% glucose (w/v) (GM17) at 32°C (L. lactis) or 37°C (E. faecalis, S. anginosus, and L. plantarum). After incubation, the donor and recipient strains were mixed (10:1) and filtered through a sterile membrane filter with a pore size of 0.45 μm (PALL, Life Sciences, Mexico). The filters were then incubated on GM17 agar with and without 50 ng per mL of tetracycline for 24 h at the optimal temperature for the recipient. After incubation the filters were washed with 2 mL of GM17 broth and suspended in the same medium. Finally, serial dilutions of the suspension were plated on GM17 agar with appropriate antibiotics for counting donor and recipient numbers, and selecting transconjugants. The plates were incubated at 32–37°C for 24–72 h and, to distinguish transconjugants from mutants, the colonies were typed with primer OPA18 using the RAPD-PCR technique as described above.
The general procedures used for DNA manipulation were essentially those described by Sambrook and Russell (2001). Restriction endonucleases (Fermentas, St. Leon-Rot, Germany), T4 DNA ligase (Roche, Mannheim, Germany), and Pfx DNA polymerase (Invitrogen, Carlsbad, CA, United States) were used according to the manufacturers’ instructions. The tet(L) gene from S. anginosus VA01-10AN was amplified by PCR with primers incorporating SalI and EcoRI restriction enzyme sites (Supplementary Table 1). Amplicons and pUC19 were digested with the two enzymes, ligated and electroporated into electrocompetent Escherichia coli DH10B cells using a GenePulser apparatus (Bio-Rad Laboratories, Richmond, CA, United States). Plasmid DNA from E. coli was purified as described by Sambrook and Russell (2001), analyzed by restriction enzyme analysis and sequenced.
The genome sequences of E. faecalis VA02-2 and VA37-4, S. anginosus VA01-10AN and VA01-14AN, and S. salivarius VA08-2AN were deposited in the GenBank database under the BioProject PRJNA604905 with biosample accession numbers SAMN14086434, SAMN14086702, SAMN14086703, SAMN14086908, and SAMN14086913, respectively.
Fifteen vaginal cocci colonies surrounded by clear halos on MRS agar with 0.5% CaCO3 (thus producing large quantities of lactic acid) were recovered. All isolates were Gram-positive, catalase negative, and γ-hemolytic. Sequencing and sequence comparison of the 16S rRNA genes identified the isolates as Enterococcus faecalis (nine isolates), Streptococcus anginosus (four isolates), and Streptococcus salivarius (two isolates) (Table 1).
Table 1. Minimum inhibitory concentration (MIC) values of 16 antibiotics to vaginal Enterococcus faecalis and Streptococcus spp. isolates.
Since the number of isolates was small, and since large phenotypic variation has been seen even among closely related isolates (Kürekci et al., 2016), all were subjected to susceptibility testing. According to the compiled clinical breakpoints from CLSI (2019) and EUCAST (2019) (Table 1), all isolates were susceptible or showed low MIC values to gentamicin, streptomycin, erythromycin, chloramphenicol, ampicillin, penicillin, vancomycin, linezolid, and trimethoprim. Among the E. faecalis isolates, four showed an MIC of tetracycline higher than the breakpoint (8 μg mL–1). Five E. faecalis isolates were considered resistant to both clindamycin and quinupristin-dalfopristin (MICs > 256 and ≥ 8 μg mL–1, respectively), and four to rifampicin (MIC 8 μg mL–1). The MIC values of E. faecalis isolates to neomycin (32–128 μg mL–1) were comparable to those seen for all other aminoglycosides. As for the Streptococcus spp., all four S. anginosus isolates showed resistance to tetracycline. Although a breakpoint for ciprofloxacin in S. anginosus has yet to be established, the MICs of this antibiotic to isolates of this species (32–64 μg mL–1) was higher than in all others. Finally, the MIC of kanamycin to S. salivarius VAN08-2AN was three dilutions higher than that to the other isolate of this species.
As for the phenotypic analysis, the small number allowed us to test by PCR the presence of a vast array of antibiotic resistance genes in all isolates; this will uncover the possible existence of the so-called silent genes (Flórez et al., 2006). Amplification of genes involved in tetracycline resistance was done with universal primer for genes encoding RPPs, as well as with gene-specific primers. Using universal primers, amplification was obtained for all eight tetracycline resistant isolates (Table 1). Further analysis using tet(M)-specific primers showed amplicons to be produced for all eight isolates. In addition, an amplicon was produced for all tetracycline-resistant S. anginosus with tet(L)-specific primers. The sequenced amplicons of tet(M) were nucleotide-identical among themselves. In contrast, those of tet(L) from S. anginosus showed identity to different gene variants.
No silent or inactive genes associated with resistance to chloramphenicol (cat), β-lactam antibiotics (bla), aminoglycosides [aac(6’)-aph(2″), and aad(E)], glycopeptides [van(A)], macrolides [erm(A), erm(B), erm(C), erm(F), and mef(A)], or encoding ribosomal protecting proteins [tet(O), tet(S), and tet(W)], or efflux protein [tet(K)] involved in tetracycline resistance, were detected in any of the isolates.
The putative housekeeping efflux-encoding gene lsaA of E. faecalis was amplified from both clindamycin and quinupristin-dalfopristin resistant and susceptible strains, and their sequences compared. The lsaA gene was complete in strains showing resistance, while mutations generating stop codons were noted in sequences from all strains displaying susceptibility to these antibiotics. As a high-fidelity polymerase with proofreading activity was not used, these results, however, cannot be considered conclusive.
The pooled results of the independent fingerprinting analyses of the isolates by RAPD-PCR and rep-PCR suggested the presence of six strains among the E. faecalis isolates, two among the S. anginosus, and one among the two S. salivarius isolates (Supplementary Figure 1). Based on the phenotypic and genotypic results, five strains were selected for genome sequencing: E. faecalis VA02-2 (resistant to tetracycline and rifampicin), E. faecalis VA37-4 (resistant to clindamycin and quinupristin-dalfopristin), and S. anginosus VA01-10AN and 01-14AN (resistant to tetracycline, and MIC of ciprofloxacin 64 and 32 μg mL–1, respectively), and S. salivarius VA08-2AN (MIC of kanamycin 128 μg mL–1).
As expected, the genome analysis confirmed the presence of tet(M) and tet(L) in the tetracycline-resistant strains in which they were previously detected by PCR. These genes were detected by searches in all three specific antimicrobial resistance databases (CARD, ResFinder, and ARG-ANNOT). CARD and ARG-ANNOT databases further identified wild type genes dfrE (encoding a dihydrofolate reductase) and efrA (encoding an ATP-binding cassette-ABC efflux pump) as involved in antibiotic resistance in E. faecalis (García-Solache and Rice, 2019). Mutations in these genes have been associated with resistance to trimethoprim, and macrolide and fluoroquinolone resistance, respectively. In addition, ResFinder and ARG-ANNOT also included lsaA gene from E. faecalis as an antibiotic resistance gene.
The tet(M) gene in E. faecalis VA02-2 was found to be encoded on a large contig of 179,144 bp (JAAJBI010000007.1; locus tag G5T25_09190). The genetic organization of around 40 kbp of this contig is depicted in Figure 1A. A detailed analysis of all open reading frames (ORFs) in this section of the contig is summarized in Supplementary Table 2. tet(M) was identified within a DNA region harboring a complete copy of the transposon Tn916 (18,031 bp). Compared to the Tn916 copy in E. faecalis DS16 (U09422.1), the copy in VA02-2 contains 14 nucleotide replacements, two deletions of one base pair, and an insertion of one base pair. None of these changes had any effect on translation, except for one of the insertions which induced a shorter version of the protein encoded by locus tag G5T25_09170 (ORF15; Supplementary Table 2). The sequence homology to that of ORF15 of Tn916 in E. faecalis DS16 is lacking from amino acid 653 onward. This gene encodes a functionally uncharacterized protein containing a YtxH domain. Nonetheless, versions of Tn916 with identical nucleotide and amino acid sequences to those of E. faecalis VA02-2 can be found for many strains in databases, including E. faecalis KUB3007 (AP018543.1), Enterococcus durans VREdu (CP042597.1), Staphylococcus aureus B6-55A (CP042110.1), and Streptococcus agalactiae PLGBS13 (CP029749.1). Tn916 in E. faecalis VA02-2 appears to be inserted into the intergenic region between the genes encoding an ATP-binding subunit of an ABC transporter for betaine/proline/choline (ORF6; locus tag G5T25_09120) and a glyoxalase (ORF25; locus tag G5T25_09225) (Figure 1A and Supplementary Table 2). In the downstream region, the nucleotide sequence of Tn916 is immediately followed by chromosomal sequences of E. faecalis, while in the upstream region a scar of six base pairs (ATTATA) between the transposon and the chromosome seems to be inserted (Figure 1A).
Figure 1. Diagram showing the genetic organization of ORFs in the contigs around the tetracycline resistance genes identified in the genome sequence of Enterococcus faecalis VA02-2 (A; JAAJBI000000007.1) and Streptococcus anginosus VA01-14AN (B; JAAJBF000000008.1). Color code of the different ORFs: purple, antibiotic resistance genes, tet(M) of E. feacalis (locus tag G5T25_09190) and tet(M) and tet(L) of S. anginosus (locus tags G5T15_06645 and G5T15_06650, respectively); red, genes involved in plasmid replication and control; yellow, transposase- associated genes; green, integrase-, mobilization-, and conjugation-associated genes; orange, genes encoding transcription regulators; white, genes involved in other processes. The broken line symbol indicates that the sequence of the contig extends beyond this point.
Similarly to tet(M) in E. faecalis, the tet(M) and tet(L) genes in S. anginosus VA01-10AN (JAAJBG010000010.1) and VA01-14AN (JAAJBF000000008.1) were identified as being adjacent in contigs larger of 65.0 kbp with an identical genetic organization. This result reinforces the genetic relatedness of the strains as determined by the typing analyses (Supplementary Figure 1). In contrast to E. faecalis VA02-2 that proved to be plasmid-free, a plasmid of 17.5 kbp was present in both S. anginosus VA01-10AN and VA01-14AN strains (data not shown). However, tet(M) and tet(L) were not found to be associated with this plasmid. The genetic organization around the tet(M) and tet(L) gene in S. anginosus VA01-14AN is depicted in Figure 1B, and the analysis of ORFs in this region is outlined in Supplementary Table 2. The two resistance genes were found to be arranged in tandem, separated by an intergenic region of only 102 bp. Canonical ribosome binding sites (GGAGG) were identified in front of the two genes, as well as upstream sequences that might function as promoters. The genes were flanked by ORFs encoding a truncated protein involved in the replication of plasmid pUB110 (from Staphylococcus aureus) on one side (locus tag G5T15_06655), and by Tn916-related ORFs involved in DNA mobilization on the other (locus tag G5T15_06635). The same organization of the region harboring the tet(M) and tet(L) is found in plasmids, e.g., in pC27-2 of Enterococcus faecium (MH784602.1), and in the chromosome of strains belonging to different species, such as Streptococcus pasteurianus ATCC 43144 (AP012054.1), Streptococcus equinus NCTC11436 (LR134203.1), Streptococcus pneumoniae IC161 (HG799499.1), E. faecium E4413 (LR135185.1), E. faecalis ATCC 29212 (CP008816.1), and many others. The existence of mobilization features from transposons and plasmids around the genes resembles somehow the genetic organization of the integrative and conjugative elements (ICEs) of staphylococci (Sansevere and Robinson, 2017).
Regarding the genetic basis of other resistances, the lsaA gene (JAAJBH010000005.1; locus tag G5T22_RS09575) of E. faecalis VA37-4 (clindamycin and quinupristin-dalfopristin resistant) was identified in a contig of 249,478 bp. This gene is believed to encode an ABC superfamily efflux protein, which provides resistance to lincosamides, pleuromutilins, and streptogramin A antibiotics (Singh et al., 2002). The gene and its derived protein sequence were analyzed and compared to those from susceptible and resistant strains of the same species. The lsaA gene of VA37-4 was found to be complete, encoding a protein of 498 amino acids. The LsaA of VA37-4 was of the same length and showed only two amino acid replacements (at positions 77 and 495) to the protein of E. faecalis OG1RF (NC_017316.1), another clindamycin and quinupristin-dalfopristin resistant strain (Supplementary Figure 2). Conversely, the lsaA gene sequence in the susceptible strain E. faecalis VA02-2 contained two mutations that destroyed the ORF and lead to the production of a truncated peptide of 152 amino acids long (Supplementary Figure 2). Thus, genome analysis confirmed the previous lsaA amplification and sequencing results in both E. faecalis VA02-2 and VA37-4. As concerns rifampicin resistance, genome analysis of VA02-2 strain failed to identify the genetic basis of its MIC to rifampicin (8 μg mL–1). Genes and well-described mutations (such as those in the rpoB gene) known to be involved in rifampicin resistance were not detected in this strain. Genome analysis of S. salivarius VA08-2AN did not identify either any gene known to be involved in kanamycin and/or aminoglycoside resistance (MIC 128 μg mL–1). Indeed, mutations in key genes known to cause resistance to aminoglycosides, such as those coding for the 16S rRNA molecule and the ribosomal protein S12, were not recognized. In the same sense, transferable trimethoprim-insensitive dihydrofolate reductase-encoding genes (dfr) (Bergmann et al., 2014) explaining the high MIC value of VA08-2AN to trimethoprim (8 μg mL–1) were not found. Further, the dihydropteroate synthase (DHPS) enzyme of S. salivarius was rather different to those of Streptococcus pneumoniae and Streptococcus pyogenes to search for substitutions causing resistance.
Tetracycline-resistant strains containing one or two antibiotic resistance genes [tet(M) and/or tet(L)] were selected as donors for conjugation experiments. Under the assay conditions, neither of the two donors used, E. faecalis VA02-2 and S. anginosus VA01-10AN, was able to transfer resistance to tetracycline to any of the four recipient strains, with a detection limit < 10–8 transconjugants per donor cell.
As a proof of concept of heterologous expression of the tetracycline resistance genes, the tet(L) gene from S. anginosus VA01-10AN was amplified with specific primers incorporating restriction enzyme sites for SalI and EcoRI, ligated in double-digested pUC19, and electrotransformed in E. coli. Restriction enzyme analysis and sequencing of recombinant plasmids proved the inserted DNA to consist in the amplified tet(L) gene. E- test analysis of several E. coli transformants demonstrated the gene provided a tetracycline resistance of 48–64 μg mL–1 to this heterologous host.
This study examines the antibiotic resistance profiles of 15 isolates belonging to a phylogenetically related group of lactic acid-producing cocci from the human vagina. The isolates belonged to the species E. faecalis (9), S. anginosus (4), and S. salivarius (2). These numbers can be compared with the retrieval from the same samples of 25 lactic acid-producing rods (mostly lactobacilli) (Sirichoat et al., 2020). Strains of these cocci species are found in a variety of environments, including food, and human and animal mucosa. E. faecalis strains have been used as starters in food fermentations, and as human and animal probiotics (Nami et al., 2014; Ben Braïek and Smaoui, 2019). However, such uses are not without controversy due to the opportunistic pathogenicity of some strains, mostly in hospital settings, and the large antibiotic resistance carriage (Ben Braïek and Smaoui, 2019). The ability of the strains in this study to produce large amounts of lactic acid might assure the inhibition of most acid-susceptible vaginal pathogens (Matu et al., 2010).
In agreement with many other works in the literature (Huys et al., 2004; Hummel et al., 2007; Fernández-Fuentes et al., 2014; Kürekci et al., 2016; Chajêcka-Wierzchowska et al., 2019), all strains were susceptible to chloramphenicol, β-lactams (ampicillin and penicillin), vancomycin, linezolid, and trimethoprim. Enterococci are thought to be susceptible to vancomycin, and are considered intrinsically resistant to clindamycin, quinupristin-dalfopristin, cephalosporins and aminoglycosides (García-Solache and Rice, 2019). In agreement with this, the present enterococci strains proved to be quite resistant to all aminoglycosides (MIC ≥ 30 μg mL–1), with the exception of gentamicin. The MIC value of kanamycin in S. salivarius VA08-2AN was higher (128 μg mL–1) than in all other Streptococcus spp. isolates. However, genome analysis of this strain did not identify any gene or mutation known to be involved in kanamycin resistance. Unspecific mechanisms, including reduced uptake of the antibiotic by the lack of cytochrome-mediated transport or decreased cell permeability can lead to a concomitant resistance to kanamycin and other aminoglycosides (Pagès, 2017). Further, the situation of kanamycin resistance in S. salivarius can somehow be similar to that reported in anaerobic and facultative bacteria, where the presence of transferase-encoding genes, such as aac(6′)-aph(2′′), ant(6), aph(3′)-IIIa, aph(E) and sat(3), has not been unequivocally associated with resistance to this antibiotic (Ammor et al., 2008). Genome analysis of E. faecalis VA02-2 failed to identify a genetic cause for its low rifampicin resistance. Unspecific mechanisms, such as reduced permeability or reduced activity of efflux/influx mechanisms, have been suggested in other bacteria to be involved in low resistance to this antibiotic (Goldstein, 2014). Further, exceeding the MIC by one dilution falls within the normal variation for the microdilution assay (Huys et al., 2010).
Surprisingly, only five out of nine E. faecalis were resistant to clindamycin; these were also resistant to quinupristin-dalfopristin. The resistance of E. faecalis to clindamycin and quinupristin-dalfopristin is considered intrinsic and related to the activity of an ABC-F type efflux protein, LsaA, encoded by the lsaA gene (Singh et al., 2002; García-Solache and Rice, 2019). Sequence analysis of the amplicons from susceptible and resistant strains of this species showed the former to have mutations in the gene disrupting its ORF, leading to the production of non-functional proteins. This was corroborated by genome analysis of resistant and susceptible strains, including that of the reference strain E. faecalis OG1RF.
Enterococci and streptococci are mostly susceptible to tetracycline (García-Solache and Rice, 2019), although acquired resistance to this antibiotic has been reported widespread (Hummel et al., 2007; Cox and Wright, 2013; Fernández-Fuentes et al., 2014; Miller et al., 2014; Kürekci et al., 2016). PCR analyses identified tet(M) in all E. faecalis resistant strains, and both tet(M) and tet(L) in those of S. anginosus resistant to this antibiotic. The excretion of tetracycline as an active drug in urine could reasonably be a driver for the maintaining and spread of resistance genes in the vaginal ecosystem (Agwuh and MacGowan, 2006).
The location in Enterococcus and Streptococcus of AMR genes in transposons, integrons and ICEs is well known (Rizzotti et al., 2009; Roberts and Mullany, 2011; Mingoia et al., 2014; Zahid et al., 2017; León-Sampedro et al., 2019). The conjugative transference of tet(M) and tet(L) from different enterococci, such as E. faecalis, E. faecium, Enterococcus mundtii and E. durans, to enterococci and Listeria species has also been reported (Huys et al., 2004; Rizzotti et al., 2009). However, under the conditions of the present study, no transfer of tet(M) and/or tet(L) from E. faecalis or S. anginosus was seen. The Tn916 transposon was found to be complete, but the sequence contained nucleotide changes and mutations that might affect its transfer to other bacteria. A substantial amount of data suggests that sub-inhibitory concentrations of antibiotics can increase the conjugation frequency of some genes (Lopatkin et al., 2016). However, in our study, a subinhibitory concentration of tetracycline during mating did not induce the conjugation process. ICEs, some of which carry tetracycline resistance genes, are composed of modules formed by genes or group of genes involved in maintenance and dissemination (Carraro and Burrus, 2014). Like Tn916 in E. faecalis VA02-2, the ICE-like element found in S. anginosus VA01-10AN might be incomplete or non-functional. Nonetheless, spread of AMR genes can be accomplished by other processes such as transduction or natural transformation (Lerminiaux and Cameron, 2019). Moreover, tet(L) has been naturally found in more than 15 genera of Gram-positive and Gram-negative bacteria (Roberts and Schwarz, 2016). Expression of the tet(L) gene from S. anginosus in a heterologous host was, therefore, not surprising. This strongly suggests that if the gene is transferred to a bacterium by any means, it will likely be functional.
In the vaginal ecosystem of the women involved in this study, only tetracycline resistance seems to have spread among E. faecalis and S. anginosus, which correlates with the presence of tet(M), or tet(L) plus tet(M), in the genomes of the resistant strains. In contrast, susceptibility to clindamycin and quinupristin-dalfopristin in some E. faecalis strains seemed to be due to disruptive mutations in lsaA. Neither tet(L) nor tet(M) from either the E. faecalis or S. anginosus strains was seen to be transferable under the assay conditions of this work. Nevertheless, tet(L) from S. anginosus proved to be functional in a heterologous host, indicating that its carriage represents a hazard. This study provides new data on the antibiotic resistance/susceptibility profiles of vaginal E. faecalis and Streptococcus spp. strains and on the genetic basis of their intrinsic and acquired resistances.
The datasets presented in this study can be found in online repositories. The names of the repository/repositories and accession number(s) can be found at: https://www.ncbi.nlm.nih.gov/nuccore/?term=BioProject+PRJNA604905, BioPro- ject PRJNA604905.
The study was approved by the Khon Kaen Ethics Committee in Human Research (Reference HE581191), and the sampling was performed following the standardized clinical guidelines of the Srinagarind Hospital (Khon Kaen). Written informed consent was obtained from each participant.
AS isolated the strains, performed the experimental work, and drafted the manuscript. AF and LV contributed to the antimicrobial testing and genome analyses. PB and MP contributed to planning the research and reviewed the drafts. VL and BM provided the financial and material resources, planned the experimental works, and reviewed the manuscript. All authors read and approved the final version.
This study was supported by a grant of the Royal Golden Jubilee (RGJ) Ph.D. Program from the Thailand Research Fund (TRF), Thailand, Ref. PHD/0133/2558, and a project from Asturias Principality, Spain, Ref. IDI/2018/000114.
The authors declare that the research was conducted in the absence of any commercial or financial relationships that could be construed as a potential conflict of interest.
We acknowledge the support of the publication fee by the CSIC Open Access Publication Support Initiative through its Unit of Information Resources for Research (URICI).
The Supplementary Material for this article can be found online at: https://www.frontiersin.org/articles/10.3389/fmicb.2020.01438/full#supplementary-material
Agwuh, K. N., and MacGowan, A. (2006). Pharmacokinetics and pharmacodynamics of the tetracyclines including glycylcyclines. Antimicrob. Chemother. 58, 256–265. doi: 10.1093/jac/dkl224
Altschul, S. F., Madden, T. L., Schäffer, A. A., Zhang, J., Zhang, Z., Miller, W., et al. (1997). Gapped BLAST and PSI-BLAST: a new generation of protein database search programs. Nucleic Acids Res. 25, 3389–3402. doi: 10.1093/nar/25.17.3389
Ammor, M. S., Flórez, A. B., van Hoek, A. H. A., de los Reyes-Gavilán, C. G., Aarts, H. J. M., Margolles, A., et al. (2008). Molecular characterization of intrinsic and acquired antibiotic resistance in lactic acid bacteria and bifidobacteria. J. Mol. Microbiol. Biotechnol. 14, 6–15. doi: 10.1159/000106077
Aziz, R. K., Bartels, D., Best, A. A., DeJongh, M., Disz, T., Edwards, R. A., et al. (2008). The RAST Server: rapid annotations using subsystems technology. BMC Genomics 9:75. doi: 10.1186/1471-2164-9-75
Bankevich, A., Nurk, S., Antipov, D., Gurevich, A. A., Dvorkin, M., Kulikov, A. S., et al. (2012). SPAdes: a new genome assembly algorithm and its applications to single-cell sequencing. J. Comput. Biol. 19, 455–477. doi: 10.1089/cmb.2012.0021
Ben Braïek, O., and Smaoui, S. (2019). Enterococci: between emerging pathogens and potential probiotics. Biomed. Res. Int. 2019:5938210. doi: 10.1155/2019/5938210
Bengtsson-Palme, J., Kristiansson, E., and Larsson, D. G. J. (2018). Environmental factors influencing the development and spread of antibiotic resistance. FEMS Microbiol. Rev. 42:fux053. doi: 10.1093/femsre/fux053
Bergmann, R., van der Linden, M., Chhatwal, G. S., and Nitsche-Schmitz, D. P. (2014). Factors that cause trimethoprim resistance in Streptococcus pyogenes. Antimicrob. Agents Chemother. 58, 2281–2288. doi: 10.1128/AAC.02282-13
Bolukaoto, J. Y., Monyama, C. M., Chukwu, M. O., Lekala, S. M., Nchabeleng, M., Maloba, M. R., et al. (2015). Antibiotic resistance of Streptococcus agalactiae isolated from pregnant women in Garankuwa, South Africa. BMC Res. Notes 8:364. doi: 10.1186/s13104-015-1328-0
Carraro, N., and Burrus, V. (2014). Biology of three ICE families: SXT/R391, ICEBs1, and ICESt1/ICESt3. Microbiol. Spectr. 2:MDNA3-0008-2014. doi: 10.1128/microbiolspec.MDNA3-0008-2014
Chajêcka-Wierzchowska, W., Zadernowska, A., Zarzecka, U., Zakrzewski, A., and Gajewska, J. (2019). Enterococci from ready-to-eat food - horizontal gene transfer of antibiotic resistance genes and genotypic characterization by PCR melting profile. J. Sci. Food Agric. 99, 1172–1179. doi: 10.1002/jsfa.9285
CLSI (2019). Performance Standards for Antimicrobial Susceptibility Testing. Supplement M100, 29th Edn. Wayne, PA: Clinical and Laboratory Standards Institute.
Cox, G., and Wright, G. D. (2013). Intrinsic antibiotic resistance: mechanisms, origins, challenges and solutions. Int. J. Med. Microbiol. 303, 287–292. doi: 10.1016/j.ijmm.2013.02.009
EFSA BIOHAZ Panel (2017). Scientific opinion on the update of the list of QPS-recommended biological agents intentionally added to food or feed as notified to EFSA. EFSA J. 15:e04664. doi: 10.2903/j.efsa.2017.4664
EFSA FEEDAP Panel (2012). Guidance on the assessment of bacterial susceptibility to antimicrobials of human and veterinary importance. EFSA J. 10:2740. doi: 10.2903/j.efsa.2012.2740
EUCAST (2019). The European Committee on Antimicrobial Susceptibility Testing. Breakpoint tables for interpretation of MICs and zone diameters. Version 9.0. Available online at: http://www.eucast.org (accessed February 03, 2020).
FDA (2010). Generally Recognized as Safe (GRAS). Notifications. Available online at: https://www.fda.gov/food/generally-recognized-safe-gras/microorganisms-microbial-derived-ingredients-used-food-partial-list (accessed April 1, 2018).
Fernández-Fuentes, M. A., Abriouel, H., Ortega Morente, E., Pérez Pulido, R., and Gálvez, A. (2014). Genetic determinants of antimicrobial resistance in Gram positive bacteria from organic foods. Int. J. Food Microbiol. 172, 49–56. doi: 10.1016/j.ijfoodmicro.2013.11.032
Flórez, A. B., Ammor, M. S., Alvarez-Martín, P., Margolles, A., and Mayo, B. (2006). Molecular analysis of tet(W) gene-mediated tetracycline resistance in dominant intestinal Bifidobacterium species from healthy humans. Appl. Environ. Microbiol. 72, 7377–7379. doi: 10.1128/AEM.00486-06
Flórez, A. B., Ammor, M. S., and Mayo, B. (2008). Identification of tet(M) in two Lactococcus lactis strains isolated from a Spanish traditional starter-free cheese made of raw milk and conjugative transfer of tetracycline resistance to lactococci and enterococci. Int. J. Food Microbiol. 121, 189–194. doi: 10.1016/j.ijfoodmicro.2007.11.029
Frank, J. A., Reich, C. I., Sharma, S., Weisbaum, J. S., Wilson, B. A., and Olsen, G. J. (2008). Critical evaluation of two primers commonly used for amplification of bacterial 16S rRNA genes. Appl. Environ. Microbiol. 74, 2461–2470. doi: 10.1128/AEM.02272-07
Fuochi, V., Cardile, V., Petronio Petronio, G., and Furneri, P. M. (2019). Biological properties and production of bacteriocins-like-inhibitory substances by Lactobacillus sp. strains from human vagina. J. Appl. Microbiol. 126, 1541–1550. doi: 10.1111/jam.14164
García-Solache, M., and Rice, L. B. (2019). The Enterococcus: a model of adaptability to its environment. Clin. Microbiol. Rev. 32:e00058-18. doi: 10.1128/CMR.00058-18
Goldstein, B. P. (2014). Resistance to rifampicin: a review. J. Antibiot. (Tokyo) 67, 625–630. doi: 10.1038/ja.2014.107
Gupta, S. K., Padmanabhan, B. R., Diene, S. M., Lopez-Rojas, R., Kempf, M., Landraud, L., et al. (2014). ARG-ANNOT, a new bioinformatic tool to discover antibiotic resistance genes in bacterial genomes. Antimicrob. Agents Chemother. 58, 212–220. doi: 10.1128/AAC.01310-13
Huang, B., Fettweis, J. M., Brooks, J. P., Jefferson, K. K., and Buck, G. A. (2014). The changing landscape of the vaginal microbiome. Clin. Lab. Med. 34, 747–761. doi: 10.1016/j.cll.2014.08.006
Hummel, A., Holzapfel, W. H., and Franz, C. M. (2007). Characterisation and transfer of antibiotic resistance genes from enterococci isolated from food. Syst. Appl. Microbiol. 30, 1–7. doi: 10.1016/j.syapm.2006.02.004
Huys, G., D’Haene, K., Cnockaert, M., Tosi, L., Danielsen, M., Flórez, A. B., et al. (2010). Intra- and inter-laboratory performances of two commercial antimicrobial susceptibility testing methods for bifidobacteria and nonenterococcal lactic acid bacteria. Antimicrob. Agents Chemother. 54, 2567–2574. doi: 10.1128/AAC.00407-10
Huys, G., D’Haene, K., Collard, J. M., and Swings, J. (2004). Prevalence and molecular characterization of tetracycline resistance in Enterococcus isolates from food. Appl. Environ. Microbiol. 70, 1555–1562. doi: 10.1128/aem.70.3.1555-1562.2004
IDF (2010). Milk and Milk Products : Determination of the Minimal Inhibitory Concentration (MIC) of Antibiotics Applicable to Bifidobacteria and non-Enterococcal Lactic acid Bacteria. Brussels: International Dairy Federation.
Koeuth, T., Versalovic, J., and Lupski, J. R. (1995). Differential subsequence conservation of interspersed repetitive Streptococcus pneumoniae BOX elements in diverse bacteria. Genome Res. 5, 408–418. doi: 10.1101/gr.5.4.408
Krzyściak, W., Pluskwa, K. K., Jurczak, A., and Kościelniak, D. (2013). The pathogenicity of the Streptococcus genus. Eur. J. Clin. Microbiol. Infect. Dis. 32, 1361–1376. doi: 10.1007/s10096-013-1914-9
Kürekci, C., Önen, S. P., Yipel, M., Aslanta”, Ö, and Gündoðdu, A. (2016). Characterisation of phenotypic and genotypic antibiotic resistance profile of enterococci from cheeses in Turkey. Korean J. Food Sci. Anim. Resour. 36, 352–358. doi: 10.5851/kosfa.2016.36.3.352
León-Sampedro, R., Fernández-de-Bobadilla, M. D., San Millan, A., Baquero, F., and Coque, T. M. (2019). Transfer dynamics of Tn6648, a composite integrative conjugative element generated by tandem accretion of Tn5801 and Tn6647 in Enterococcus faecalis. J. Antimicrob. Chemother. 74, 2517–2523. doi: 10.1093/jac/dkz239
Lerminiaux, N. A., and Cameron, A. D. S. (2019). Horizontal transfer of antibiotic resistance genes in clinical environments. Can. J. Microbiol. 65, 34–44. doi: 10.1139/cjm-2018-0275
Lopatkin, A. J., Huang, S., Smith, R. P., Srimani, J. K., Sysoeva, T. A., Bewick, S., et al. (2016). Antibiotics as a selective driver for conjugation dynamics. Nat. Microbiol. 1:16044. doi: 10.1038/nmicrobiol.2016.44
Martin, D. H. (2012). The microbiota of the vagina and its influence on women’s health and disease. Am. J. Med. Sci. 343, 2–9. doi: 10.1097/MAJ.0b013e31823ea228
Martín, R., Soberón, N., Vaneechoutte, M., Flórez, A. B., Vázquez, F., and Suárez, J. E. (2008). Characterization of indigenous vaginal lactobacilli from healthy women as probiotic candidates. Int. Microbiol. 11, 261–266. doi: 10.2436/20.1501.01.70
Mättö, J., Malinen, E., Suihko, M. L., Alander, M., Palva, A., and Saarela, M. (2004). Genetic heterogeneity and functional properties of intestinal bifidobacteria. J. Appl. Microbiol. 97, 459–470. doi: 10.1111/j.1365-2672.2004.02340.x
Matu, M. N., Orinda, G. O., Njagi, E. N., Cohen, C. R., and Bukusi, E. A. (2010). In vitro inhibitory activity of human vaginal lactobacilli against pathogenic bacteria associated with bacterial vaginosis in Kenyan women. Anaerobe 16, 210–215. doi: 10.1016/j.anaerobe.2009.11.002
McArthur, A. G., Waglechner, N., Nizam, F., Yan, A., Azad, M. A., Baylay, A. J., et al. (2013). The comprehensive antibiotic resistance database. Antimicrob. Agents Chemother. 57, 3348–3357. doi: 10.1128/AAC.00419-13
Miller, W. R., Munita, J. M., and Arias, C. A. (2014). Mechanisms of antibiotic resistance in enterococci. Expert. Rev. Anti. Infect. Ther. 12, 1221–1236. doi: 10.1586/14787210.2014.956092
Mingoia, M., Morici, E., Morroni, G., Giovanetti, E., Del Grosso, M., Pantosti, A., et al. (2014). Tn5253 family integrative and conjugative elements carrying mef(I) and catQ determinants in Streptococcus pneumoniae and Streptococcus pyogenes. Antimicrob. Agents Chemother. 58, 5886–5893. doi: 10.1128/AAC.03638-14
Nagaraja, P. (2008). Antibiotic resistance of Gardnerella vaginalis in recurrent bacterial vaginosis. Indian J. Med. Microbiol. 26, 155–157. doi: 10.4103/0255-0857.40531
Nami, Y., Abdullah, N., Haghshenas, B., Radiah, D., Rosli, R., and Yari Khosroushahi, A. (2014). A newly isolated probiotic Enterococcus faecalis strain from vagina microbiota enhances apoptosis of human cancer cells. J. Appl. Microbiol. 117, 498–508. doi: 10.1111/jam.12531
Nogacka, A., Salazar, N., Suárez, M., Milani, C., Arboleya, S., Solís, G., et al. (2017). Impact of intrapartum antimicrobial prophylaxis upon the intestinal microbiota and the prevalence of antibiotic resistance genes in vaginally delivered full-term neonates. Microbiome 5:93. doi: 10.1186/s40168-017-0313-3
Pagès, J. M. (2017). Antibiotic transport and membrane permeability: new insights to fight bacterial resistance. Biol. Aujourdhui 211, 149–154. doi: 10.1051/jbio/2017020
Peterson, E., and Kaur, P. (2018). Antibiotic resistance mechanisms in bacteria: relationships between resistance determinants of antibiotic producers, environmental bacteria, and clinical pathogens. Front. Microbiol. 9:2928. doi: 10.3389/fmicb.2018.02928
Rizzotti, L., La Gioia, F., Dellaglio, F., and Torriani, S. (2009). Molecular diversity and transferability of the tetracycline resistance gene tet(M), carried on Tn916-1545 family transposons, in enterococci from a total food chain. Antonie Van Leeuwenhoek 96, 43–52. doi: 10.1007/s10482-009-9334-7
Roberts, A. P., and Mullany, P. (2011). Tn916-like genetic elements: a diverse group of modular mobile elements conferring antibiotic resistance. FEMS Microbiol. Rev. 35, 856–871. doi: 10.1111/j.1574-6976.2011.00283.x
Roberts, M. C., and Schwarz, S. (2016). Tetracycline and phenicol resistance genes and mechanisms: importance for agriculture, the environment, and humans. J. Environ. Qual. 45, 576–592. doi: 10.2134/jeq2015.04.0207
Rossetti, L., and Giraffa, G. (2005). Rapid identification of dairy lactic acid bacteria by M13-generated, RAPD-PCR fingerprint databases. J. Microbiol. Methods 63, 135–144. doi: 10.1016/j.mimet.2005.03.001
Sambrook, J., and Russell, D. W. (2001). Molecular Cloning: a Laboratory Manual. New York, NY: Cold Spring Harbor Laboratory Press.
Sansevere, E. A., and Robinson, D. A. (2017). Staphylococci on ICE: overlooked agents of horizontal gene transfer. Mob. Genet. Elements 7, 1–10. doi: 10.1080/2159256X.2017.1368433
Singh, K. V., Weinstock, G. M., and Murray, B. E. (2002). An Enterococcus faecalis ABC homologue (Lsa) is required for the resistance of this species to clindamycin and quinupristin-dalfopristin. Antimicrob. Agents Chemother. 46, 1845–1850. doi: 10.1128/aac.46.6.1845-1850.2002
Sirichoat, A., Buppasiri, P., Engchanil, C., Namwat, W., Faksri, K., Sankuntaw, N., et al. (2018). Characterization of vaginal microbiota in Thai women. PeerJ 6:e5977. doi: 10.7717/peerj.5977
Sirichoat, A., Flórez, A. B., Vázquez, L., Buppasiri, P., Panya, M., Lulitanond, V., et al. (2020). Antibiotic susceptibility profiles of lactic acid bacteria from the human vagina and genetic basis of acquired resistances. Int. J. Mol. Sci. 21:2594. doi: 10.3390/ijms21072594
Smith, S. B., and Ravel, J. (2017). The vaginal microbiota, host defence and reproductive physiology. J. Physiol. 595, 451–463. doi: 10.1113/JP271694
Tatusova, T., DiCuccio, M., Badretdin, A., Chetvernin, V., Nawrocki, E. P., Zaslavsky, L., et al. (2016). NCBI prokaryotic genome annotation pipeline. Nucleic Acids Res. 44, 6614–6624. doi: 10.1093/nar/gkw569
van Hoek, A. H., Mevius, D., Guerra, B., Mullany, P., Roberts, A. P., and Aarts, H. J. (2011). Acquired antibiotic resistance genes: an overview. Front. Microbiol. 2:203. doi: 10.3389/fmicb.2011.00203
Zahid, S., Bin-Asif, H., Hasan, K. A., Rehman, M., and Ali, S. A. (2017). Prevalence and genetic profiling of tetracycline resistance (Tet-R) genes and transposable element (Tn916) in environmental Enterococcus species. Microb. Pathog. 111, 252–261. doi: 10.1016/j.micpath.2017.09.009
Keywords: antibiotic resistance, tetracycline resistance, genome analysis, vaginal strains, Enterococcus faecalis, Streptococcus anginosus, Streptococcus salivarius
Citation: Sirichoat A, Flórez AB, Vázquez L, Buppasiri P, Panya M, Lulitanond V and Mayo B (2020) Antibiotic Resistance-Susceptibility Profiles of Enterococcus faecalis and Streptococcus spp. From the Human Vagina, and Genome Analysis of the Genetic Basis of Intrinsic and Acquired Resistances. Front. Microbiol. 11:1438. doi: 10.3389/fmicb.2020.01438
Received: 18 March 2020; Accepted: 03 June 2020;
Published: 26 June 2020.
Edited by:
Alessandra Polissi, University of Milan, ItalyReviewed by:
Prakash M. Halami, Central Food Technological Research Institute (CSIR), IndiaCopyright © 2020 Sirichoat, Flórez, Vázquez, Buppasiri, Panya, Lulitanond and Mayo. This is an open-access article distributed under the terms of the Creative Commons Attribution License (CC BY). The use, distribution or reproduction in other forums is permitted, provided the original author(s) and the copyright owner(s) are credited and that the original publication in this journal is cited, in accordance with accepted academic practice. No use, distribution or reproduction is permitted which does not comply with these terms.
*Correspondence: Baltasar Mayo, YmFsdGFzYXIubWF5b0BpcGxhLmNzaWMuZXM=
Disclaimer: All claims expressed in this article are solely those of the authors and do not necessarily represent those of their affiliated organizations, or those of the publisher, the editors and the reviewers. Any product that may be evaluated in this article or claim that may be made by its manufacturer is not guaranteed or endorsed by the publisher.
Research integrity at Frontiers
Learn more about the work of our research integrity team to safeguard the quality of each article we publish.