- 1Faculty of Health and Life Sciences, Northumbria University, Newcastle upon Tyne, United Kingdom
- 2Institut de Biologie Moléculaire des Plantes, Centre National de Recherche Scientifique (CNRS), Université de Strasbourg, Strasbourg, France
- 3School of Natural and Environmental Sciences, Newcastle University, Newcastle upon Tyne, United Kingdom
- 4Laboratoire de Spectrométrie de Masse Bio-organique, Institut Pluridisciplinaire Hubert Curien, UMR 7178 CNRS, Université de Strasbourg, Strasbourg, France
- 5Department of Biological Sciences, College of Science, Kuwait University, Safat, Kuwait
- 6Environmental Biotechnology Program, Life Sciences Department, College of Graduate Studies, Arabian Gulf University, Manama, Bahrain
Rhodococcus strain IGTS8 is the most extensively studied model bacterium for biodesulfurization of fossil fuels via the non–destructive sulfur–specific 4S pathway. This strain was initially assigned to Rhodococcus rhodochrous and later to Rhodococcus erythropolis thus making its taxonomic status debatable and reflecting the limited resolution of methods available at the time. In this study, phylogenomic analyses of the whole genome sequences of strain IGTS8 and closely related rhodococci showed that R. erythropolis and Rhodococcus qingshengii are very closely related species, that Rhodococcus strain IGTS8 is a R. qingshengii strain and that several strains identified as R. erythropolis should be re-classified as R. qingshengii. The genomes of strains assigned to these species contain potentially novel biosynthetic gene clusters showing that members of these taxa should be given greater importance in the search for new antimicrobials and other industrially important biomolecules. The plasmid-borne dsz operon encoding fossil fuel desulfurization enzymes was present in R. qingshengii IGTS8 and R. erythropolis XP suggesting that it might be transferable between members of these species.
Introduction
The implementation of green and economic technologies in the petroleum industry is of increasing interest. This is particularly important in view of the continuously increasing global demand for cleaner fuels, stricter environmental regulations and dwindling oil prices (Sahu et al., 2015; Head and Gray, 2016; Ismail et al., 2017). The biotechnological desulfurization of crude oil and transportation fuels, such as diesel and gasoline, holds the potential to overcome or mitigate the technical, economic and environmental hurdles of conventional thermochemical desulfurization technologies (Kilbane, 2006; Mohebali and Ball, 2016). Rhodococcus strain IGTS8 (ATCC 53968) was the first bacterium to be isolated based on its unique ability to break C-S bonds of fuel-born organosulfur compounds and utilize them as a sole sulfur source (Denome et al., 1994). This discovery raised the prospect of using biocatalysts for removal of sulfur from fossil fuels via biodesulfurization (Kilbane and Bielaga, 1990; Kilbane II and Jackowski, 1992).
The biodesulfurization reactions are catalyzed by two monooxygenases (DszC and DszA) and a desulfinase (DszB) which constitute the non-destructive 4S pathway. This pathway was first reported and characterized in the IGTS8 strain which for many years has served as the most commonly adopted model for biodesulfurization studies. The genes of the 4S pathway, dszA, dszB, and dszC, are carried as an operon on a linear megaplasmid in the IGTS8 strain (Kilbane and Stark, 2016). These genes have been found in R. erythropolis strains A66 and A69 which use various organosulfur and organonitrogen compounds as sole sources of sulfur and nitrogen, respectively (Santos et al., 2007). DszC shows sulfur acquisition oxidoreductase (SfnB family) and acyl-CoA dehydrogenase activities and catalyzes the conversion of dibenzothiophene to dibenzothiophene sulfone (Gray et al., 1996). DszA, a FMN-dependent oxidoreductase of the nitrilotriacetate monooxygenase family, converts dibenzothiophene sulfone to 2-(2′-hydroxyphenyl) benzenesulfinate which is catalytically converted to 2-hydroxybiphenyl and sulfite by the desulfinase DszB.
Rhodococcus strain IGTS8 was classified as Rhodococcus rhodochrous (Zopf, 1891; Tsukamura, 1974) by Kayser et al. (1993) prior to being reclassified as R. erythropolis (Gray and Thornton, 1928; Goodfellow and Alderson, 1977; Oldfield et al., 1998; Santos et al., 2007). These initial classifications likely reflect the limited resolution of the taxonomic methods available at the time (Goodfellow et al., 1998, 1999). Members of the genus Rhodococcus (Zopf, 1891) emend Goodfellow et al. (1998) are known for their metabolic versatility and potential biotechnological applications in areas such as bioremediation, biotransformation and biocatalysis. They also produce biosurfactants, carotenoids and bioflocculation agents of industrial interest (Gürtler and Seviour, 2010; Jones and Goodfellow, 2012).
The extent of the heterogeneity encompassed within the genus Rhodococcus became apparent as a result of the application of phylogenomic methods (Sangal et al., 2016, 2019). Such studies have benefited from the use of improved metrics for the definition of species and genera (Chun and Rainey, 2014; Qin et al., 2014; Chun et al., 2018; Sant’Anna et al., 2019). Sangal and his colleagues assigned representative rhodococci to multiple well circumscribed species-groups named after the species that held priority (Sangal et al., 2016, 2019). The R. erythropolis species-group included the type strains R. erythropolis, R. enclensis (Dastager et al., 2014), R. globerulus (Goodfellow et al., 1982) and R. qingshengii (Xu et al., 2007; Táncsics et al., 2014).
The importance of resolving the taxonomic status of rhodococci which show remarkable catabolic activity is often overlooked despite significant improvements in the classification of the genus Rhodococcus. In the present study, the whole genome sequence of Rhodococcus strain IGTS8 was compared with those of R. erythropolis and R. qingshengii strains to establish its taxonomic status and to compare the biosynthetic and biodesulfurization capabilities of strains assigned to these species.
Materials and Methods
Bacterial Strain
Rhodococcus strain IGTS8 (American Type Culture Collection, ATCC 53968) was isolated by Kilbane and colleagues at the Gas Technology Institute-Chicago based on its sulfur-specific fuel biodesulfurization capabilities (Kilbane and Bielaga, 1990; Kilbane II and Jackowski, 1992).
Genomic DNA Extraction and Sequencing
Rhodococcus strain IGTS8 was grown overnight in nutrient broth at 28°C and the cells pelleted by centrifugation. Genomic DNA was extracted with the Wizard Genomic DNA purification kit (Promega) using the manufacturer’s instructions for Gram positive bacteria and a library constructed with the Nextera XT DNA Library Prep kit (Ilumina) using 1 ng of genomic DNA. The library was evaluated with a 2100 Bioanalyzer (Agilent Technologies) before sequencing on an Illumina Miseq system (2 × 150 paired end reads).
Genome Assembly and Computational Analyses
The PCR duplicates in the libraries were filtered with FastUniq 1.1 (Xu et al., 2012) and low-quality (<30) and adapter sequences trimmed with cutadapt 1.14 (Martin, 2011). Contaminant screening was performed with FastQ Screen 0.11.1 (Wingett and Andrews, 2018) to filter reads mapping on the PhiX sequence, vector sequences in the NCBI UniVec database1 and other species including Homo sapiens (hg19), Arabidopsis thaliana (TAIR10), Escherichia coli (NC_000913.3), Stenotrophomonas maltophilia (NC_010943.1) and Pseudomonas aeruginosa (NC_002516.2). The remaining singleton reads in the data were excluded with the tool makepairs of the Pairfq algorithm2 and the quality of the data checked with FastQC3. The genome sequence was assembled with SPAdes 3.10.1 (Bankevich et al., 2012) using kmer values of 25, 35, 45, 55, 65, 85, 105 and 125 in the gage mode. The quality of the assembly was assessed with QUAST 4.5 (Gurevich et al., 2013). A 16S rRNA gene sequence extracted from strain IGTS8 using BARRNAP 0.8 (Seemann, 2013) was BLAST-searched into the GenBank (Camacho et al., 2009). An extended scaffold was created from the reference genome of Rhodococcus qingshengii strain djl6-2 using the software Ragout 2.0 (Kolmogorov et al., 2014) and the resulting assembly annotated using the RAST-SEED pipeline (Aziz et al., 2008).
The genome sequences of other R. qingshengii strains and those of the closely related Rhodococcus erythropolis were obtained from the GenBank (Table 1). These genomes were also annotated using the RAST pipeline in order to have an equivalence of annotation for the comparative analyses. The genome sequences were compared using Roary (Page et al., 2015). A phylogenetic tree was calculated from concatenated nucleotide sequences of the core genome (1985 genes) after removing the sites with gaps using IQ-Tree (Nguyen et al., 2015). Pairwise digital DNA-DNA hybridization (dDDH) values were calculated using GGDC 2.1 (Auch et al., 2010) and average nucleotide identity (ANI) values determined using FastANI (Jain et al., 2018) between the strains within the dataset. Average amino acid identities (AAI) from reciprocal best hits were calculated between R. erythropolis NBRC 15567T, R. qingshengii JCM 15477T and the IGTS8 strain using the web server (Rodriguez-Rivera and Konstantinidis, 2014)4.
Biosynthetic and Biodesulfurization Potential
Whole genome sequences of all of the strains were analyzed using antiSMASH v5.1.1 to identify biosynthetic gene clusters (BGCs) using “Strict” detection criteria and additional features (KnownClusterBlast, ClusterBlast, SubClusterBlast, ActiveSiteFinder and Cluster Pfam analysis; Blin et al., 2019). The BGCs from the strains were sorted into groups based on their predicted activity. The protein sequences of the biodesulfurization enzymes DszA, DszB and DszC were obtained from the GenBank (accession number: L37363) and sought within the dataset using BLASTP (Camacho et al., 2009), as mentioned above.
Results and Discussion
Genomic Features
The sequencing reads for strain IGTS8 were assembled into 25 contigs (Table 2). The largest scaffold was 6.4 Mb in size (digital GC content 61.7 mol%) and was annotated with 6,132 coding DNA sequences, 2 rRNA and 54 tRNA genes. Other smaller contigs were potential plasmid sequences that varied from 1.2 to 88.4 kb in size and contained 0–94 genes (Table 2). The genome assembly of the IGTS8 strain was submitted to GenBank under the accession numbers CP029297–CP029321.
Phylogenomic Characterization
A BLAST search of an extracted 16S rRNA gene sequence from the genome of Rhodococcus strain IGTS8 showed 99.92% identity to R. erythropolis strain X5 and R. qingshengii strain RL1. Consequently, we downloaded the genome sequences of 29 R. erythropolis and R. qingshengii strains available from GenBank and calculated dDDH values against strain IGTS8 (Table 3). The dDDH value between the latter and R. erythropolis NBRC 15567T was 62.1% which is below the accepted species cut-off of 70% (Auch et al., 2010). In contrast, the dDDH value between strain IGTS8 and R. qingshengii JCM 15477T was 98.2%, hence well above this threshold. The corresponding ANI values between strain IGTS8 and the type strains of R. erythropolis and R. qingshengii were 95.48 and 99.68, respectively, that is slightly and well above the 95% recognized threshold for the delineation of species (Konstantinidis and Tiedje, 2005). Similarly, the AAI value between strain IGTS8 and R. qingshengii JCM 15477T (99.31%; SD: 5.07%) was much higher than that calculated against R. erythropolis NBRC 15567T (96.67%; SD: 7.09%). In addition, partial sequences of the catA and gyrB genes of R. qingshengii strain djl-6T (= DSM 45222T = JCM 15477T) were obtained from the GenBank (accession numbers KF500432.1 and KF374699.1, respectively). BLAST search (Altschul et al., 1997; Camacho et al., 2009) for these genes not only gave single hits in the genome of strain IGTS8 but also showed 100% identity in each case. These results indicate that strain IGTS8 is more closely related to the R. qingshengii strain than to the type strain of R. erythropolis.
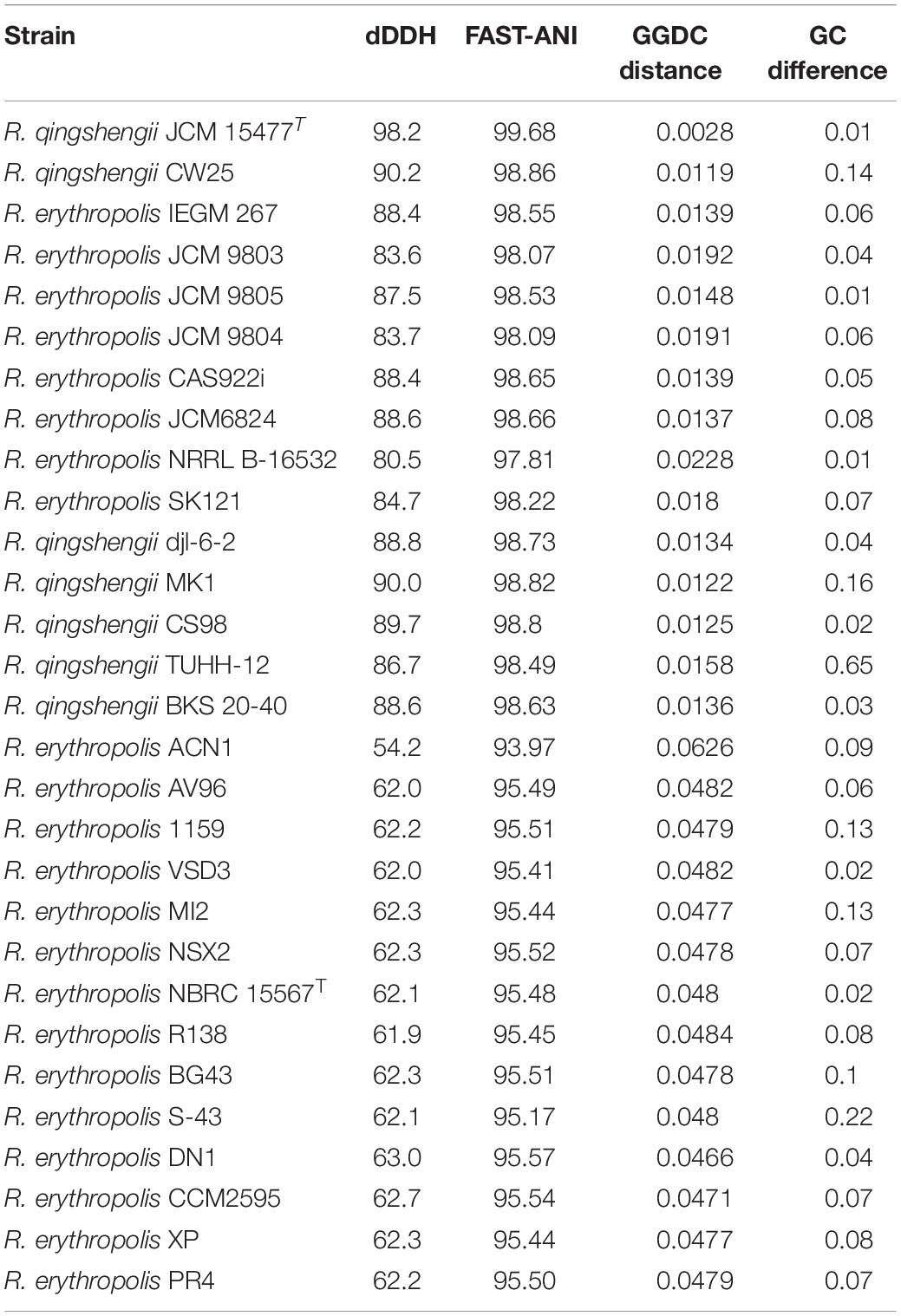
Table 3. dDDH values (recommended formula #2), ANI values, GGDC distances and differences in GC content between the genomes of Rhodococcus strain IGTS8 and R. erythropolis and R. qingshengii strains obtained from GenBank.
The size and digital GC content of the genome of strain IGTS8 were similar to those of the strains received as R. erythropolis and R. qingshengii. These values ranged from 6.28 to 7.43 Mb and from 61.7 to 62.5 mol%, respectively (Table 1). A phylogenetic tree generated from concatenated core genome sequences of these strains showed that they fell into two distinct clades, one encompassing 13 R. erythropolis strains including R. erythropolis NBRC 15567T and the other all of the R. qingshengii strains together with 7 strains received as R. erythropolis (Figure 1). Pairwise dDDH calculations indicated the presence of three species in the dataset (Supplementary Table S1). These results are consistent with previous studies in which R. erythropolis and R. qingshengii strains fell into a single taxon, namely species-group D (Sangal et al., 2016, 2019). Eight isolates initially classified as R. erythropolis (strains CAS922i, IEGM 267, JCM 6824, JCM 9803, JCM 9804, JCM 9805, NRRL B-16532 and SK121) showed ≥ 80% dDDH values with R. qingshengii JCM 15477T and should be re-classified as R. qingshengii. Similarly, the dDDH values between R. erythropolis NBRC 15567T and strains 1159, AV96, BG43, CCM 2595, DN1, MI2, NSX2, PR4, R138, S-43, XP and VSD3 varied from 85.7 to 90.2% which is consistent with their designation as R. erythropolis. One strain, R. erythropolis ACN1, formed a distinct phyletic line and probably merits species status (Figure 1 and Supplementary Table S1).
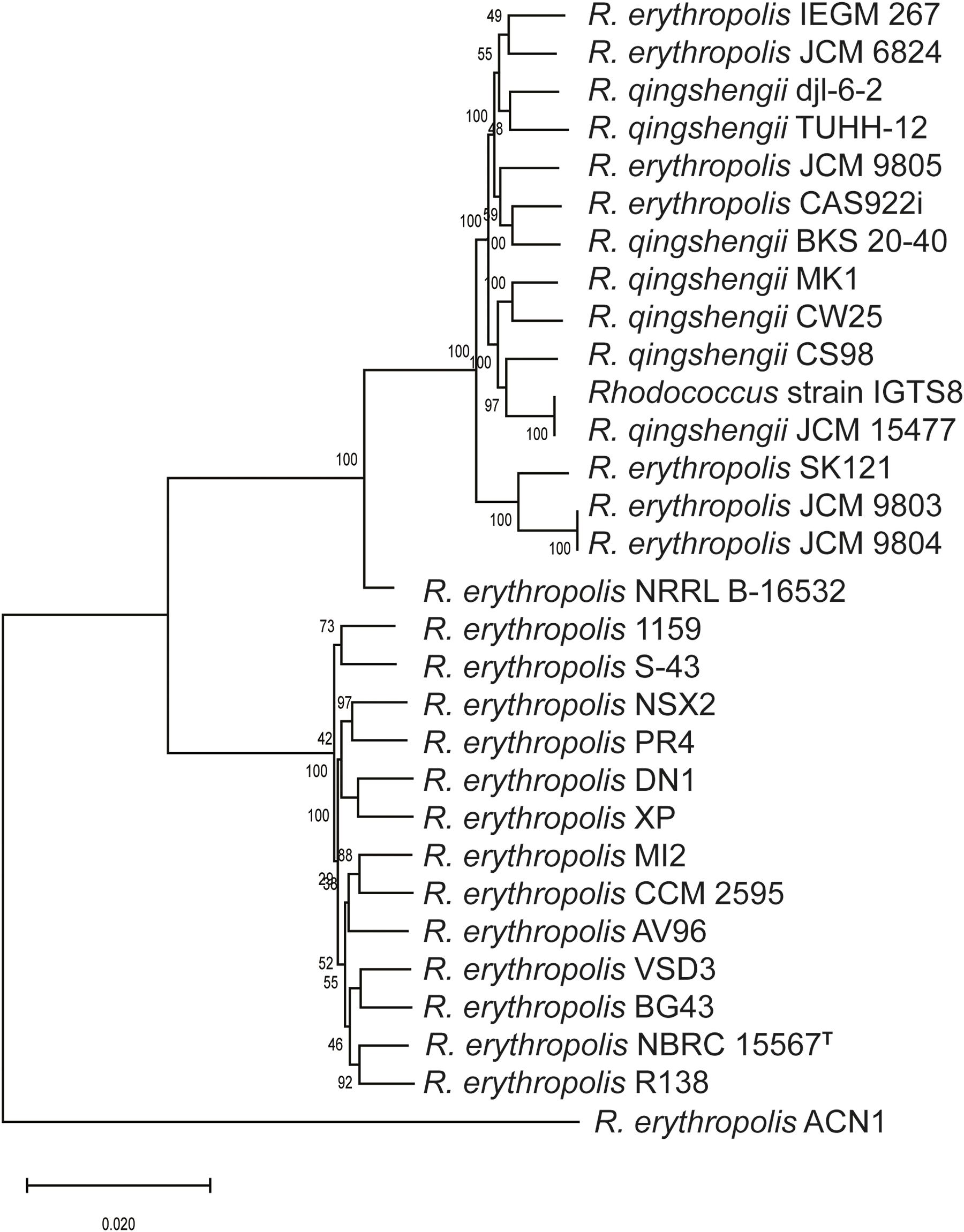
Figure 1. A maximum-likelihood phylogenomic tree generated from concatenated nucleotide sequences of the core genomes of the R. erythropolis and R. qingshengii strains. The scale bar represents the number of nucleotide substitutions per site.
ANI values between members within the R. qingshengii and R. erythropolis clades varied between 97.41 and 99.86% (average: 98.43 ± 0.38; Supplementary Table S2) and 98.16–98.79% (average: 98.56 ± 0.13), respectively which is well above the cut-off point for assigning them to these species. Pairwise ANI values between the strains from the two clades were marginally higher than the 95–96% threshold suggesting that they might belong to a single species (ANI values: 95.11–96.43%; average: 95.53 ± 0.21). The corresponding AAI value between R. erythropolis NBRC 15567T and R. qingshengii JCM 15477T (AAI: 96.64%; SD: 7.14%) underpins this point.
R. qingshengii was proposed as a novel species based on phenotypic differences and an experimental DDH value of 23.8% between strain djl-6T (JCM 15477T) and R. erythropolis DSM 43066T (NBRC 15567T; Xu et al., 2007). Computational DDH values between the individuals of the two clades defined in the present study were 61.7–68.9% (average: 62.9 ± 1.4), values that are much higher than the corresponding experimental value between the R. qingshengii and R. erythropolis type strains but still below the 70% cut-off value (Supplementary Table S1). An ANI value of 96% was found to be suitable for Aeromonas species delineations that correlated with the dDDH cut-off of 70% (Colston et al., 2014). Similarly, in the present study ANI and dDDH values were found to be strongly correlated (R2 = 0.9932; Figure 2). It has been pointed out that the use of a universal cut-off point is not appropriate as ANI values reflect the methods used to determine them and that the evolutionary history of the taxa under study should be considered (Palmer et al., 2020).
A comparison of all 30 genomes revealed a pangenome encompassing 32,515 genes, including 1,985 core genes present amongst all of the strains (Supplementary Table S3). Based on the default protein BLAST identity of 95%, only 34 and 35 genes were found to be unique to strains in the R. erythropolis and R. qingshengii clades, respectively. However, 53–60% of these genes encode hypothetical proteins (Supplementary Table S3). For the remaining clade-specific genes, homologs (other genes encoding proteins with the same functions) were observed among the other clades (Supplementary Table S3). The default 95% identity cut-off is highly stringent for strains of different species which has potentially separated some of the orthologous genes as clade-specific genes.
Many genes (218) were found to be specific to strain IGTS8 with 116 encoding hypothetical proteins or proteins of unknown function and 10 belonging to mobile genetic elements such as transposases (Supplementary Table S3). Again, homologs of the remaining 92 genes with annotated functions were present among other strains. However, five genes encoding 3-hydroxy-3-isohexenylglutaryl-CoA:acetate lyase (re30.peg.6706), acetophenone carboxylase subunit Apc3 (re30.peg.6438), cyanate ABC transporter substrate binding protein (re30.peg.6484), a possible dicarboxylate carrier protein (re30.peg.6646) and a small heat shock protein (re30.peg.4427) appear to be specific to IGTS8 and may have introduced minor functional variations in strain IGTS8 in comparison to other R. qingshengii strains. Molecular investigations are required to understand the functional impact of these genes and those encoding hypothetical proteins on the strain IGTS8.
The genomic data from this study not only underline the close relationship between R. erythropolis and R. qingshengii strains (Xu et al., 2007; Dastager et al., 2014; Táncsics et al., 2014; Sangal et al., 2016, 2019) but also show that the case for assigning them to a single species is problematic. In such instances, recommended thresholds of genomic indices for species delineation not only need to be interpreted with care but should also take into account the broader taxonomic properties of the organisms (Colston et al., 2014; Riesco et al., 2018). Given the assignment of the IGTS8 strain to a distinct phylogenomic clade corresponding to R. qingshengii (Figure 1), supporting in silico dDDH similarity values (Table 3) and genomic features (Supplementary Tables S1, S2) together with available phenotypic data (Gray et al., 1996; Xu et al., 2007; Jones and Goodfellow, 2012), it is prudent to continue to recognize the taxonomic status of R. erythropolis and R. qingshengii.
Biosynthetic Potential
The number of predicted biosynthetic gene clusters (BGCs) was similar amongst the R. erythropolis and R. qingshengii strains ranging between 13 and 24 (Supplementary Table S4). The draft assemblies with higher numbers of contigs are predicted to have more gene clusters than the finished genomes, e.g., R. erythropolis strain S-43 with 533 contigs has the highest number of gene clusters. The six complete genomes of strains assigned to the two species have 15–17 BGCs (Figure 1; Supplementary Table S4). These observations are consistent with those of Sanchez-Hidalgo et al. (2018) who identified more gene clusters in genomes with smaller contigs amongst Amycolatopsis strains. The BGCs were assigned to 12 types all of which were detected in the genomes of both the R. erythropolis and R. qingshengii strains. In contrast, Ceniceros et al. (2017) found that predicted BGCs in rhodococci, including R. erythropolis strains, were clade-specific and lacked any homology with gene clusters encoding known natural products.
AntiSMASH predicts BGCs and potential products based on the percentage of genes from the closest known BGC showing significant BLAST hits to the query genome (Blin et al., 2019). In this study, twelve gene clusters including four BGCs potentially encoding bacteriocin, ectoine, heterobactin A/heterobactin S2 (non-ribosomal peptide synthetase, NRPS) and erythrochelin (NRPS) were highly conserved among the R. erythropolis or R. qingshengii strains and were predicted among 21–30 genomes (Supplementary Table S4). The cluster potentially encoding bacteriocin was present in all 30 strains with 75–100% of genes similar to known bacteriocin-producing clusters (Supplementary Table S4). Bacteriocins are commonly produced by bacteria to inhibit other strains competing for resources, some are extensively used in the food industry (Riley and Wertz, 2002; Settanni and Corsetti, 2008; Yang et al., 2014). The BGC predicted to encode ectoine biosynthesis was also identified in all 30 strains. Ectoine, a protective molecule, enables bacteria to survive in extreme conditions (Graf et al., 2008), indicating that R. erythropolis and R. qingshengii strains may be able to withstand harsh environmental conditions such as osmotic stress. In addition, it is used commercially as an osmotic stabilizing agent in healthcare and cosmetics (Kunte et al., 2014; Czech et al., 2018).
The number of NRPS clusters ranged from 5 out of 15 in R. erythropolis strain PR4 to 16 out of 24 in R. erythropolis strain VSD3 (Supplementary Table S4). Five NRPS gene clusters were highly conserved in the dataset, including a cluster with 90–100% of the genes coding for heterobactin A/heterobactin S2 biosynthesis (Supplementary Table S4). Only R. erythropolis strains NBRC 15567T and S-43 showed lower proportions of genes (36 and 63%, respectively) similar to those coding for heterobactin A/heterobactin S2 biosynthetic enzymes. Heterobactins are siderophores involved in iron uptake which is essential for bacterial growth. Heterobactin biosynthesis was previously detected in R. erythropolis PR4 and the IGTS8 strains (Carran et al., 2001; Bosello et al., 2013). A predicted erythrochelin BGC was found to be conserved among 27 genomes (Supplementary Table S4). However, the corresponding cluster in R. qingshengii strain JCM 15477T was shown to encompass a relatively lower proportion (42%) of genes when compared to known erythrochelin BGCs. Erythrochelin is another class of siderophores involved in iron uptake (Robbel et al., 2010). A third NRPS cluster showed partial gene similarities (18–27% of the genes) to that encoding coelichelin biosynthesis, a siderophore reported in Streptomyces coelicolor (Challis and Ravel, 2000). Siderophores have a wide range of roles as biosensors and as bioremediation and chelation agents involved in weathering of soil minerals and in enhancing plant growth (Ahmed and Holmstrom, 2014).
One of the conserved NRPS biosynthetic clusters present in the genomes of 22 of the strains showed limited gene similarities (3–4% of the genes) to those encoding rifamorpholines A-E (Supplementary Table S4). Rifamorpholines A-E are macrolactams, a new subclass of rifamycin antibiotics produced by Amycolatopsis strain HCa4 which show antimicrobial activity against methicillin-resistant Staphylococcus aureus (Xiao et al., 2017). A NRPS-terpene hybrid cluster was detected in the genomes of 27 strains with 6% of genes similar to a BGC responsible for biosynthesis of SF2575 (an anticancer tetracycline; Pickens et al., 2009).
Conserved BGCs include a butyrolactone and a lanthipeptide cluster (no known similarities to any existing BGC), a cluster for biosynthesis of a linear azol(in)e-containing peptide (LAP) type (11% of the genes similar to those encoding diisonitrile antibiotic SF2768), a terpene biosynthesis cluster (18–42% of the genes similar to ones encoding an isorenieratene pigment) and a type 1 polyketide synthase (T1PKS; 6–8% similar to a kirromycin-encoding cluster). Butyrolactones are involved in intracellular signaling and are intermediates in the biosynthesis of other molecules that have an important role in metabolism and stress response (Du et al., 2011). Lanthipeptides, also known as RiPPs (ribosomally synthesized and post-translationally modified peptides) are involved in multiple biological activities, including inhibition of pathogenic bacteria (Repka et al., 2017; Ongey et al., 2018). Diisonitrile SF2768 is responsible for chelation and transport of copper and has antifungal properties (Wang et al., 2017; Xu and Tan, 2019) while polyketides are known as antimicrobial, immune-suppressing and antifungal agents (Gomes et al., 2013).
In addition to the conserved BGCs, other clusters were present in some of the R. erythropolis and R. qingshengii strains (Supplementary Table S4). For instance, the genomes of R. qingshengii strains JCM 6824, JCM 15477 and IGTS8 contained a cluster showing 81% similarity to genes encoding arylpolyene (aurachin RE) and a cluster in the genome of R. erythropolis strain JCM 9804 had 60% of the genes similar to those encoding albachelin biosynthesis. Arylpolyenes are bacterial pigments structurally and functionally similar to carotenoids (Schoner et al., 2016) and are active as antimicrobial and antioxidant agents (Narsing Rao et al., 2017). Albachelin is a siderophore that was initially isolated from Amycolatopsis alba (Kodani et al., 2015). Other BGCs showed either no or limited similarities to known clusters and are likely to produce novel metabolites. Similarly, other NRPS BGCs showed partial similarities to different antibiotic-producing clusters (Supplementary Table S4). These clusters appear quite diverse compared with previously characterized clusters and may encode novel antibiotics and hence need molecular characterization.
In total, 17 BGCs were detected in strain IGTS8 (Supplementary Table S4). According to ClusterBLAST results, 15 BGCs potentially encoding biosynthesis of 9−methylstreptimidone (NRPS), bacillomycin D (NRPS), bacteriocin, butyrolactone, coelichelin (NRPS), ectoine, erythrochelin (NRPS), heterobactin A/heterobactin S2 (NRPS), isorenieratene (terpene), kirromycin (T1PKS), monensin (NRPS) and rifamorpholine A-E were also present among other R. erythropolis, R. qingshengii and some undefined Rhodococcus strains (Supplementary Figure S1: BGCs 2–11 and 13–17). The BGC predicted to encode aurachin RE biosynthesis was distantly related (3–5% gene similarity) to multiple Streptomyces strains including S. rosechromogenus (5%), and to strains of Amycolatopsis nigriscens (5%), Micromonospora tulbaghiae (3%) and Saccharothrix espanaenesis (4%; Supplementary Figure S1: BGC 12). This BGC was also present in two additional strains in the R. qingshengii clade (Supplementary Table S4). A BGC encoding a linear azol(in)e-containing peptide (similar to diisonitrile antibiotic SF2768) showed high relatedness to Frankia strain BMG 5.23 (75% of genes in the BGC) and moderate similarity (17–47% of genes in the BGC) with other Frankia strains, and with strains of Williamsia muralis, Rhodococcus fascians, and Kibdelospoangium phytohabitans (Supplementary Figure S1: BGC 1). Overall, these results demonstrate the presence of multiple BGCs in the dataset that are potentially capable of producing novel compounds and are largely shared by R. erythropolis and R. qingshengii strains.
Biodesulfurization Genes-the dsz Operon
R. qingshengii strain IGTS8 is the prototype of fuel-biodesulfurizing bacteria harboring the 4S biodesulfurization pathway which allows the use of diesel-born organosulfur compounds, such as dibenzothiophene as a sole sulfur source, converting it to 2-hydroxybiphenyl and releasing the sulfur atom as sulfite (Oldfield et al., 1998). The 4S pathway enzymes are encoded by the plasmid-borne dsz operon (Denome et al., 1994; Santos et al., 2007; Khan et al., 2017). A search for this dsz operon (Piddington et al., 1995) amongst the R. qingshengii and R. erythropolis strains showed that it was only present in the genome of R. erythropolis XP. This soil isolate, which possesses a plasmid carrying the dsz operon, is able to desulfurize benzonaphthothiophene (Yu et al., 2006; Tao et al., 2011). Interestingly, R. qingshengii IGTS8 and R. erythropolis XP strains belong to different rhodococcal clades (Figure 1) suggesting that the plasmid carrying the dsz operon may be transferable between R. qingshengii and R. erythropolis strains via horizontal gene transfer. This proposition is in line with the fact that the dsz genes are usually carried on conjugative plasmids close to transposable elements, such as insertion sequences (Kayser et al., 2002; Kilbane and Le Borgne, 2004; Mohebali and Ball, 2016).
Conclusion
The phylogenomic analyses show that R. erythropolis and R. qingshengii strains are members of very closely related species that belong to the R. erythropolis species-group. They also show that the catabolically active organism Rhodococcus strain IGTS8 is a bona fide member of R. qingshengii. Strains assigned to each of these species were shown to have genomes that contained 12 highly conserved BGCs, most of which showed limited similarity with clusters coding for the biosynthesis of known specialized metabolites thereby providing further evidence that rhodococci should feature prominently in drug discovery programmes. The finding that the genome of R. erythropolis strain XP, like that of R. qingshengii strain IGTS8, contains the biodesulfurization dsz operon suggests that plasmids carrying this operon may be readily transferable between R. erythropolis and R. qingshengii strains.
Data Availability Statement
The datasets presented in this study can be found in online repositories. The names of the repository/repositories and accession number(s) can be found in the article/Supplementary Material.
Author Contributions
WI and HM conceived the study. VS designed the strategy for the data analyses. VC and SK extracted genomic DNA, performed sequencing and assembled the reads into scaffolds. DT and VS analyzed the data. MG helped with the taxogenomic analyses and classification of strain IGTS8. HM, DH, CC, and AV provided intellectual input on the biosynthetic and catabolic potential of the bacterial strains. VS, WI, and MG drafted the manuscript. All authors contributed to the article and approved the submitted version.
Funding
This study was a part of a research project funded by the Arabian Gulf University (Grant No. LS_WE_2018).
Conflict of Interest
The authors declare that the research was conducted in the absence of any commercial or financial relationships that could be construed as a potential conflict of interest.
Acknowledgments
We would like to thank Jimmy Gibson for IT assistance.
Supplementary Material
The Supplementary Material for this article can be found online at: https://www.frontiersin.org/articles/10.3389/fmicb.2020.01417/full#supplementary-material
Footnotes
- ^ https://www.ncbi.nlm.nih.gov/tools/vecscreen/about/
- ^ https://github.com/djhshih/pairfq
- ^ https://www.bioinformatics.babraham.ac.uk/projects/fastqc/
- ^ http://enve-omics.ce.gatech.edu/aai/
References
Ahmed, E., and Holmstrom, S. J. (2014). Siderophores in environmental research: roles and applications. Microb. Biotechnol. 7, 196–208. doi: 10.1111/1751-7915.12117
Altschul, S. F., Madden, T. L., Schaffer, A. A., Zhang, J., Zhang, Z., Miller, W., et al. (1997). Gapped BLAST and PSI-BLAST: a new generation of protein database search programs. Nucleic Acids Res. 25, 3389–3402. doi: 10.1093/nar/25.17.3389
Auch, A. F., Von Jan, M., Klenk, H. P., and Göker, M. (2010). Digital DNA-DNA hybridization for microbial species delineation by means of genome-to-genome sequence comparison. Stand. Genomic Sci. 2, 117–134. doi: 10.4056/sigs.531120
Aziz, R. K., Bartels, D., Best, A. A., Dejongh, M., Disz, T., Edwards, R. A., et al. (2008). The RAST Server: rapid annotations using subsystems technology. BMC Genomics 9:75. doi: 10.1186/1471-2164-9-75
Bankevich, A., Nurk, S., Antipov, D., Gurevich, A. A., Dvorkin, M., Kulikov, A. S., et al. (2012). SPAdes: a new genome assembly algorithm and its applications to single-cell sequencing. J. Comput. Biol. 19, 455–477. doi: 10.1089/cmb.2012.0021
Blin, K., Shaw, S., Steinke, K., Villebro, R., Ziemert, N., Lee, S. Y., et al. (2019). antiSMASH 5.0: updates to the secondary metabolite genome mining pipeline. Nucleic Acids Res. 47, W81–W87.
Bosello, M., Zeyadi, M., Kraas, F. I., Linne, U., Xie, X., and Marahiel, M. A. (2013). Structural characterization of the heterobactin siderophores from Rhodococcus erythropolis PR4 and elucidation of their biosynthetic machinery. J. Nat. Prod. 76, 2282–2290. doi: 10.1021/np4006579
Camacho, C., Coulouris, G., Avagyan, V., Ma, N., Papadopoulos, J., Bealer, K., et al. (2009). BLAST+: architecture and applications. BMC Bioinform. 10:421. doi: 10.1186/1471-2164-9-421
Carran, C. J., Jordan, M., Drechsel, H., Schmid, D. G., and Winkelmann, G. (2001). Heterobactins: a new class of siderophores from Rhodococcus erythropolis IGTS8 containing both hydroxamate and catecholate donor groups. Biometals 14, 119–125.
Ceniceros, A., Dijkhuizen, L., Petrusma, M., and Medema, M. H. (2017). Genome-based exploration of the specialized metabolic capacities of the genus Rhodococcus. BMC Genomics 18:593. doi: 10.1186/1471-2164-9-593
Challis, G. L., and Ravel, J. (2000). Coelichelin, a new peptide siderophore encoded by the Streptomyces coelicolor genome: structure prediction from the sequence of its non-ribosomal peptide synthetase. FEMS Microbiol. Lett. 187, 111–114. doi: 10.1111/j.1574-6968.2000.tb09145.x
Chun, J., Oren, A., Ventosa, A., Christensen, H., Arahal, D. R., Da Costa, M. S., et al. (2018). Proposed minimal standards for the use of genome data for the taxonomy of prokaryotes. Int. J. Syst. Evol. Microbiol. 68, 461–466. doi: 10.1099/ijsem.0.002516
Chun, J., and Rainey, F. A. (2014). Integrating genomics into the taxonomy and systematics of the Bacteria and Archaea. Int. J. Syst. Evol. Microbiol. 64, 316–324. doi: 10.1099/ijs.0.054171-0
Colston, S. M., Fullmer, M. S., Beka, L., Lamy, B., Gogarten, J. P., and Graf, J. (2014). Bioinformatic genome comparisons for taxonomic and phylogenetic assignments using Aeromonas as a test case. mBio 5:e02136.
Czech, L., Hermann, L., Stoveken, N., Richter, A. A., Hoppner, A., Smits, S. H. J., et al. (2018). Role of the extremolytes ectoine and hydroxyectoine as stress protectants and nutrients: Genetics, phylogenomics, biochemistry, and structural analysis. Genes 9:177. doi: 10.3390/genes9040177
Dastager, S. G., Mawlankar, R., Tang, S. K., Krishnamurthi, S., Ramana, V. V., Joseph, N., et al. (2014). Rhodococcus enclensis sp. nov., a novel member of the genus Rhodococcus. Int. J. Syst. Evol. Microbiol. 64, 2693–2699.
Denome, S. A., Oldfield, C., Nash, L. J., and Young, K. D. (1994). Characterization of the desulfurization genes from Rhodococcus sp. strain IGTS8. J. Bacteriol. 176, 6707–6716. doi: 10.1128/jb.176.21.6707-6716.1994
Du, Y. L., Shen, X. L., Yu, P., Bai, L. Q., and Li, Y. Q. (2011). Gamma-butyrolactone regulatory system of Streptomyces chattanoogensis links nutrient utilization, metabolism, and development. Appl. Environ. Microbiol. 77, 8415–8426. doi: 10.1128/aem.05898-11
Gomes, E. S., Schuch, V., and De Macedo Lemos, E. G. (2013). Biotechnology of polyketides: new breath of life for the novel antibiotic genetic pathways discovery through metagenomics. Braz. J. Microbiol. 44, 1007–1034. doi: 10.1590/s1517-83822013000400002
Goodfellow, M., and Alderson, G. (1977). The actinomycete-genus Rhodococcus: a home for the “rhodochrous” complex. J. Gen. Microbiol. 100, 99–122. doi: 10.1099/00221287-100-1-99
Goodfellow, M., Alderson, G., and Chun, J. (1998). Rhodococcal systematics: problems and developments. Antonie Van Leeuwenhoek 74, 3–20.
Goodfellow, M., Isik, K., and Yates, E. (1999). Actinomycete systematics: an unfinished synthesis. Nova Acta Leopold NF 80, 47–82.
Goodfellow, M., Weaver, C. R., and Minnikin, D. E. (1982). Numerical classification of some rhodococci, corynebacteria and related organisms. J. Gen. Microbiol. 128, 731–745. doi: 10.1099/00221287-128-4-731
Graf, R., Anzali, S., Buenger, J., Pfluecker, F., and Driller, H. (2008). The multifunctional role of ectoine as a natural cell protectant. Clin. Dermatol. 26, 326–333. doi: 10.1016/j.clindermatol.2008.01.002
Gray, K. A., Pogrebinsky, O. S., Mrachko, G. T., Xi, L., Monticello, D. J., and Squires, C. H. (1996). Molecular mechanisms of biocatalytic desulfurization of fossil fuels. Nat. Biotechnol. 14, 1705–1709. doi: 10.1038/nbt1296-1705
Gray, P. H. H., and Thornton, H. G. (1928). Soil bacteria that decompose certain aromatic compounds. Zentralbl. Bakteriol. Parasitenk. Infektionskr. Hyg. Abt. II 73, 74–96.
Gurevich, A., Saveliev, V., Vyahhi, N., and Tesler, G. (2013). QUAST: quality assessment tool for genome assemblies. Bioinformatics 29, 1072–1075. doi: 10.1093/bioinformatics/btt086
Gürtler, V., and Seviour, R. (2010). “Systematics of members of the genus Rhodococcus (Zopf, 1891) emend Goodfellow et al., 1998,” in Biology of Rhodococcus, ed. H. M. Alvarez (Heidelberg: Springer), 1–28. doi: 10.1007/978-3-642-12937-7_1
Head, I. M., and Gray, N. D. (2016). Microbial biotechnology 2020; microbiology of fossil fuel resources. Microb. Biotechnol. 9, 626–634. doi: 10.1111/1751-7915.12396
Ismail, W. A., Van Hamme, J. D., Kilbane, J. J., and Gu, J. D. (2017). Editorial: petroleum microbial biotechnology: challenges and prospects. Front. Microbiol. 8:833. doi: 10.3389/fmicb.2017.00833
Jain, C., Rodriguez, R. L., Phillippy, A. M., Konstantinidis, K. T., and Aluru, S. (2018). High throughput ANI analysis of 90K prokaryotic genomes reveals clear species boundaries. Nat. Commun. 9:5114.
Jones, A. L., and Goodfellow, M. (2012). “Genus IV Rhodococcus (Zopf, 1891) emended. Goodfellow, Alderson and Chun 1998a,” in Bergey’s Manual of Systematic Bacteriology, The Actinobacteria, 2 Edn, Vol. 5, eds M. Goodfellow, P. Kämpfer, H.-J. Busse, M. E. Trujillo, K.-I. Suzuki, W. Ludwig, et al. (New York, NY: Springer), 437–464.
Kayser, K. J., Bielaga-Jones, B. A., Jackowski, K., Odusan, O., and Kilbane, J. J. (1993). Utilization of organosulphur compounds by axenic and mixed cultures of Rhodococcus rhodochrous IGTS8. Microbiology 139, 3123–3129. doi: 10.1099/00221287-139-12-3123
Kayser, K. J., Cleveland, L., Park, H. S., Kwak, J. H., and Kolhatkar, A. (2002). Isolation and characterization of a moderate thermophile, Mycobacterium phlei GTIS10, capable of dibenzothiophene desulfurization. Appl. Microbiol. Biotechnol. 59, 737–745.
Khan, S., Adhikari, D. K., Gupta, S., and Gupta, N. (2017). Degradation of carbazole, dibenzothiophene and polyaromatic hydrocarbons by recombinant Rhodococcus sp. Biotechnol. Lett. 39, 277–281. doi: 10.1007/s10529-016-2242-9
Kilbane, J. (2006). Microbial biocatalyst developments to upgrade fossil fuels. Curr. Opin. Biotechnol. 17, 305–314. doi: 10.1016/j.copbio.2006.04.005
Kilbane, J. J. II, and Jackowski, K. (1992). Biodesulfurization of water-soluble coal-derived material by Rhodococcus rhodochrous IGTS8. Biotechnol. Bioeng. 40, 1107–1114. doi: 10.1002/bit.260400915
Kilbane, J. J. II, and Stark, B. (2016). Biodesulfurization: a model system for microbial physiology research. World. J. Microbiol. Biotechnol. 32:137.
Kilbane, J. J., and Le Borgne, S. (2004). “Petroleum biorefining: the selective removal of sulfur, nitrogen, and metals,” in Studies in Surface Science and Catalysis, eds R. Vazquez-Duhalt and R. Quintero-Ramirez (Amsterdam: Elsevier), 29–65. doi: 10.1016/s0167-2991(04)80143-5
Kodani, S., Komaki, H., Suzuki, M., Hemmi, H., and Ohnishi-Kameyama, M. (2015). Isolation and structure determination of new Siderophore albachelin from Amycolatopsis alba. Biometals 28, 381–389. doi: 10.1007/s10534-015-9842-z
Kolmogorov, M., Raney, B., Paten, B., and Pham, S. (2014). Ragout-a reference-assisted assembly tool for bacterial genomes. Bioinformatics 30, i302–i309. doi: 10.1093/bioinformatics/btu280
Konstantinidis, K. T., and Tiedje, J. M. (2005). Genomic insights that advance the species definition for prokaryotes. Proc. Natl. Acad. Sci. U.S.A. 102, 2567–2572. doi: 10.1073/pnas.0409727102
Kunte, H. J., Lentzen, G., and Galinski, E. A. (2014). Industrial production of the cell protectant ectoine: protection mechanisms, processes, and products. Curr. Biotechnol. 3, 10–25. doi: 10.2174/22115501113026660037
Martin, M. (2011). Cutadapt removes adapter sequences from high-throughput sequencing reads. EMBnet J. 17, 10–12.
Mohebali, G., and Ball, A. S. (2016). Biodesulfurization of diesel fuels – past, present and future perspectives. Int. Biodeterior. Biodegrad. 110, 163–180. doi: 10.1016/j.ibiod.2016.03.011
Narsing Rao, M. P., Xiao, M., and Li, W. J. (2017). Fungal and bacterial pigments: secondary metabolites with wide applications. Front. Microbiol. 8:1113. doi: 10.3389/fmicb.2017.001113
Nguyen, L. T., Schmidt, H. A., Von Haeseler, A., and Minh, B. Q. (2015). IQ-TREE: a fast and effective stochastic algorithm for estimating maximum-likelihood phylogenies. Mol. Biol. Evol. 32, 268–274. doi: 10.1093/molbev/msu300
Oldfield, C., Wood, N. T., Gilbert, S. C., Murray, F. D., and Faure, F. R. (1998). Desulphurisation of benzothiophene and dibenzothiophene by actinomycete organisms belonging to the genus Rhodococcus, and related taxa. Antonie Van Leeuwenhoek 74, 119–132.
Ongey, E. L., Giessmann, R. T., Fons, M., Rappsilber, J., Adrian, L., and Neubauer, P. (2018). Heterologous biosynthesis, modifications and structural characterization of ruminococcin-A, a lanthipeptide from the gut bacterium Ruminococcus gnavus E1, in Escherichia coli. Front. Microbiol. 9:1688. doi: 10.3389/fmicb.2017.01688
Page, A. J., Cummins, C. A., Hunt, M., Wong, V. K., Reuter, S., Holden, M. T., et al. (2015). Roary: rapid large-scale prokaryote pan genome analysis. Bioinformatics 31, 3691–3693. doi: 10.1093/bioinformatics/btv421
Palmer, M., Steenkamp, E. T., Blom, J., Hedlund, B. P., and Venter, S. N. (2020). All ANIs are not created equal: implications for prokaryotic species boundaries and integration of ANIs into polyphasic taxonomy. Int. J. Syst. Evol. Microbiol. 70, 2937–2948. doi: 10.1099/ijsem.0.004124
Pickens, L. B., Kim, W., Wang, P., Zhou, H., Watanabe, K., Gomi, S., et al. (2009). Biochemical analysis of the biosynthetic pathway of an anticancer tetracycline SF2575. J. Am. Chem. Soc. 131, 17677–17689. doi: 10.1021/ja907852c
Piddington, C. S., Kovacevich, B. R., and Rambosek, J. (1995). Sequence and molecular characterization of a DNA region encoding the dibenzothiophene desulfurization operon of Rhodococcus sp. strain IGTS8. Appl. Environ. Microbiol. 61, 468–475. doi: 10.1128/aem.61.2.468-475.1995
Qin, Q. L., Xie, B. B., Zhang, X. Y., Chen, X. L., Zhou, B. C., Zhou, J., et al. (2014). A proposed genus boundary for the prokaryotes based on genomic insights. J. Bacteriol. 196, 2210–2215. doi: 10.1128/jb.01688-14
Repka, L. M., Chekan, J. R., Nair, S. K., and Van Der Donk, W. A. (2017). Mechanistic understanding of lanthipeptide biosynthetic enzymes. Chem. Rev. 117, 5457–5520. doi: 10.1021/acs.chemrev.6b00591
Riesco, R., Carro, L., Roman-Ponce, B., Prieto, C., Blom, J., Klenk, H. P., et al. (2018). Defining the species Micromonospora saelicesensis and Micromonospora noduli under the framework of genomics. Front. Microbiol. 9:1360. doi: 10.3389/fmicb.2017.01360
Riley, M. A., and Wertz, J. E. (2002). Bacteriocins: evolution, ecology, and application. Annu. Rev. Microbiol. 56, 117–137. doi: 10.1146/annurev.micro.56.012302.161024
Robbel, L., Knappe, T. A., Linne, U., Xie, X., and Marahiel, M. A. (2010). Erythrochelin–a hydroxamate-type siderophore predicted from the genome of Saccharopolyspora erythraea. FEBS J. 277, 663–676. doi: 10.1111/j.1742-4658.2009.07512.x
Rodriguez-Rivera, L. D., and Konstantinidis, K. T. (2014). Bypassing cultivation to identify bacterial species. ASM Microb. Mag. 9, 111–118. doi: 10.1128/microbe.9.111.1
Sahu, R., Song, B. J., Im, J. S., Jeon, Y. P., and Lee, C. W. (2015). A review of recent advances in catalytic hydrocracking of heavy residues. J. Ind. Eng. Chem. 27, 12–24. doi: 10.1016/j.jiec.2015.01.011
Sanchez-Hidalgo, M., Gonzalez, I., Diaz-Munoz, C., Martinez, G., and Genilloud, O. (2018). Comparative genomics and biosynthetic potential analysis of two lichen-isolated Amycolatopsis strains. Front. Microbiol. 9:369. doi: 10.3389/fmicb.2017.00369
Sangal, V., Goodfellow, M., Jones, A. L., Schwalbe, E. C., Blom, J., Hoskisson, P. A., et al. (2016). Next-generation systematics: an innovative approach to resolve the structure of complex prokaryotic taxa. Sci. Rep. 6:38392.
Sangal, V., Goodfellow, M., Jones, A. L., Seviour, R. J., and Sutcliffe, I. C. (2019). “Refined systematics of the genus Rhodococcus based on whole genome analyses,” in Biology of Rhodococcus, ed. H. Alvarez (Cham: Springer).
Sant’Anna, F., Bach, E., Porto, R. Z., Guella, F., and Hayashi, H. (2019). Genomic metrics made easy: what to do and where to go in the new era of bacterial taxonomy. Crit. Rev. Microbiol. 45, 182–200. doi: 10.1080/1040841x.2019.1569587
Santos, S. C., Alviano, D. S., Alviano, C. S., Goulart, F. R., De Padula, M., Leitao, A. C., et al. (2007). Comparative studies of phenotypic and genetic characteristics between two desulfurizing isolates of Rhodococcus erythropolis and the well-characterized R. erythropolis strain IGTS8. J. Ind. Microbiol. Biotechnol. 34, 423–431. doi: 10.1007/s10295-007-0214-8
Schoner, T. A., Gassel, S., Osawa, A., Tobias, N. J., Okuno, Y., Sakakibara, Y., et al. (2016). Aryl polyenes, a highly abundant class of bacterial natural products, are functionally related to antioxidative carotenoids. Chembiochem 17, 247–253. doi: 10.1002/cbic.201500474
Seemann, T. (2013). BARRNAP 0.8: Rapid Ribosomal RNA Prediction. Available online at: https://github.com/tseemann/barrnap (accessed September 30, 2017).
Settanni, L., and Corsetti, A. (2008). Application of bacteriocins in vegetable food biopreservation. Int. J. Food Microbiol. 121, 123–138. doi: 10.1016/j.ijfoodmicro.2007.09.001
Táncsics, A., Benedek, T., Farkas, M., Mathe, I., Marialigeti, K., Szoboszlay, S., et al. (2014). Sequence analysis of 16S rRNA, gyrB and catA genes and DNA-DNA hybridization reveal that Rhodococcus jialingiae is a later synonym of Rhodococcus qingshengii. Int. J. Syst. Evol. Microbiol. 64, 298–301. doi: 10.1099/ijs.0.059097-0
Tao, F., Zhao, P., Li, Q., Su, F., Yu, B., Ma, C., et al. (2011). Genome sequence of Rhodococcus erythropolis XP, a biodesulfurizing bacterium with industrial potential. J. Bacteriol. 193, 6422–6423. doi: 10.1128/jb.06154-11
Tsukamura, M. (1974). A further numerical taxonomic study of the rhodochrous group. Jpn. J. Microbiol. 18, 37–44. doi: 10.1111/j.1348-0421.1974.tb00741.x
Wang, L., Zhu, M., Zhang, Q., Zhang, X., Yang, P., Liu, Z., et al. (2017). Diisonitrile natural product SF2768 functions as a chalkophore that mediates copper acquisition in Streptomyces thioluteus. ACS Chem. Biol. 12, 3067–3075. doi: 10.1021/acschembio.7b00897
Wingett, S. W., and Andrews, S. (2018). FastQ screen: a tool for multi-genome mapping and quality control. F1000Research 7:1338. doi: 10.12688/f1000research.15931.1
Xiao, Y. S., Zhang, B., Zhang, M., Guo, Z. K., Deng, X. Z., Shi, J., et al. (2017). Rifamorpholines A-E, potential antibiotics from locust-associated actinobacteria Amycolatopsis sp. Hca4. Org. Biomol. Chem. 15, 3909–3916.
Xu, H., Luo, X., Qian, J., Pang, X., Song, J., Qian, G., et al. (2012). FastUniq: a fast de novo duplicates removal tool for paired short reads. PLoS One 7:e52249. doi: 10.1371/journal.pone.0052249
Xu, J. L., He, J., Wang, Z. C., Wang, K., Li, W. J., Tang, S. K., et al. (2007). Rhodococcus qingshengii sp. nov., a carbendazim-degrading bacterium. Int. J. Syst. Evol. Microbiol. 57, 2754–2757.
Xu, Y., and Tan, D. S. (2019). Total synthesis of the bacterial diisonitrile chalkophore SF2768. Org. Lett. 21, 8731–8735.
Yang, S. C., Lin, C. H., Sung, C. T., and Fang, J. Y. (2014). Antibacterial activities of bacteriocins: application in foods and pharmaceuticals. Front. Microbiol. 5:241. doi: 10.3389/fmicb.2017.00241
Yu, B., Xu, P., Shi, Q., and Ma, C. (2006). Deep desulfurization of diesel oil and crude oils by a newly isolated Rhodococcus erythropolis strain. Appl. Environ. Microbiol. 72, 54–58.
Keywords: biodesulfurization, Rhodococcus, 4S pathway, phylogenomics, dibenzothiophene, average nucleotide identity, digital DNA-DNA hybridization
Citation: Thompson D, Cognat V, Goodfellow M, Koechler S, Heintz D, Carapito C, Van Dorsselaer A, Mahmoud H, Sangal V and Ismail W (2020) Phylogenomic Classification and Biosynthetic Potential of the Fossil Fuel-Biodesulfurizing Rhodococcus Strain IGTS8. Front. Microbiol. 11:1417. doi: 10.3389/fmicb.2020.01417
Received: 19 April 2020; Accepted: 02 June 2020;
Published: 07 July 2020.
Edited by:
Kian Mau Goh, University of Technology Malaysia, MalaysiaReviewed by:
Benjamin C. Stark, Illinois Institute of Technology, United StatesJuana Maria Navarro Llorens, Complutense University of Madrid, Spain
John Joseph Kilbane, Illinois Institute of Technology, United States
Copyright © 2020 Thompson, Cognat, Goodfellow, Koechler, Heintz, Carapito, Van Dorsselaer, Mahmoud, Sangal and Ismail. This is an open-access article distributed under the terms of the Creative Commons Attribution License (CC BY). The use, distribution or reproduction in other forums is permitted, provided the original author(s) and the copyright owner(s) are credited and that the original publication in this journal is cited, in accordance with accepted academic practice. No use, distribution or reproduction is permitted which does not comply with these terms.
*Correspondence: Wael Ismail, d2FlbGFtZUBhZ3UuZWR1LmJo
†These authors have contributed equally to this work