Corrigendum: Low-Temperature Adaptation of the Snow Alga Chlamydomonas nivalis Is Associated With the Photosynthetic System Regulatory Process
- 1Key Laboratory of Algal Biology, Institute of Hydrobiology, Chinese Academy of Sciences, Wuhan, China
- 2University of Chinese Academy of Sciences, Beijing, China
- 3State Key Laboratory of Crop Stress Adaptation and Improvement, School of Life Sciences, Henan University, Kaifeng, China
- 4Innovation Academy for Seed Design, Chinese Academy of Sciences, Wuhan, China
The alga Chlamydomonas nivalis thrives in polar snow fields and on high-altitude mountain tops, and contributes significantly on primary production in the polar regions, however, the mechanisms underlying this adaptation to low temperatures are unknown. Here, we compared the growth, photosynthetic activity, membrane lipid peroxidation, and antioxidant activity of C. nivalis with those of the model alga C. reinhardtii, under grow temperature and low temperatures. C. nivalis maintained its photosynthetic activity in these conditions by reducing the light-harvesting ability of photosystem II and enhancing the cyclic electron transfer around photosystem I, both of which limited damage to the photosystem from excess light energy and resulted in ATP production, supporting cellular growth and other physiological processes. Furthermore, the increased cyclic electron transfer rate, carotenoid content, and antioxidant enzyme activities jointly regulated the reactive oxygen species levels in C. nivalis, enabling recovery from excess excitation energy and reduced photooxidative damage to the cell. Therefore, we propose a model in which adaptive mechanisms related to photosynthetic regulation promote the survival and even blooming of C. nivalis under polar environment, suggesting that C. nivalis can provide organic carbon sources as an important primary producer for other surrounding life in the polar regions for maintaining ecosystem.
Introduction
The eukaryotic and prokaryotic single-celled organisms known as microalgae are important inhabitants of ocean, freshwater, and even terrestrial ecosystems (Msanne et al., 2012; Li et al., 2016). The efficient photosynthesis of microalgae provided the basic conditions required for all complex life on Earth (Zhang et al., 2014; Chen et al., 2015b; Li et al., 2016). Microalgae are ideal model organisms due to their fast growth, strong adaptability to extreme environments, and high oil contents.
Snow algae grow in the polar snow fields and in high-altitude mountain tops. Most of the algae in polar snow algal community belong to Chlorophyceae, Chlamydomonadales or Volvocales, some of which have received more attention in recent years, such as Chlamydomonas nivalis (Cvetkovska et al., 2016), Chlamydomonas cf. nivalis strain CCALA 970 (Lukes et al., 2014), Chloromonas reticulate (Matsuzaki et al., 2012), Sanguina nivaloides, and Sanguina aurantia (Procházková et al., 2019). C. nivalis, belonging to the genera Chlamydomonas (Chlorophyta) and closely relating to the model algae Chlamydomonas reinhardtii, is a typical snow alga and a leading system for investigating cold adaptation (Cvetkovska et al., 2016). C. nivalis produces substantial biomass even under extreme conditions, such as low temperature, high light, low pH, nutrient deficiency, freeze-thaw cycles, and UV irradiation, and thus serves as a vital food source for other cold-adapted organisms, such as ice worms, collembola, and bacteria (Thomas and Duval, 1995; Ursula et al., 1996; Painter et al., 2001).
C. nivalis cells possess specialized mechanisms that allow them to withstand extreme environmental stresses, such as a high accumulation of lipids and carotenoids, a reduced number of light-harvesting pigment–protein complexes, and high levels of astaxanthin esterified with fatty acids, which reduces light damage and photoinhibition, maintaining maximum photosynthesis efficiency (Yong and Lee, 1991; Bidigare et al., 1993; Rezanka et al., 2014; Hulatt et al., 2017). Nonetheless, the adaptive mechanisms by which C. nivalis withstands low temperatures are unclear.
Photosynthesis, which converts carbon dioxide into chemical energy using energy from sunlight, is the major mechanism by which most photosynthetic organisms harvest energy (Liang et al., 2013). Photosynthesis takes place in the thylakoid membrane and involves a four-subunit protein complex comprising photosystem II (PSII), photosystem I (PSI), the cytochrome b6/f (Cyt b6f) complex, and ATP synthase (Hohmann-Marriott and Blankenship, 2011). PSII, PSI, and Cyt b6f are connected in a linear electron transfer (LET) chain and couple proton pumping with ATP synthesis via ATP synthase (Zhan et al., 2016). Around PSI, two types of electron transfer exist: LET, which generates ATP and NADPH, and cyclic electron transfer (CET), which generates ATP at times of NADPH shortage (Yamori et al., 2015). CET regulates the balance of ATP/NADPH in photosynthetic cells and protects the light system from high levels of light damage. Under low temperatures, NDH-dependent CET plays an important role in relieving oxidative damage in chloroplasts in photosynthetic organisms (Shikanai, 2007; Yamori et al., 2011; Zhang et al., 2013).
When photosynthetic organisms are exposed to stress, the rate of photosynthesis decreases and excess electrons are transferred to molecular oxygen (O2) to form reactive oxygen species (ROS) (Mittler, 2002; Liu et al., 2017). ROS include 1O2, H2O2, O2–, and HO., which cause oxidative damage to proteins, DNA, and lipids (Apel and Hirt, 2004; Song et al., 2014; Chen et al., 2015a). The scavenging system of ROS includes antioxidant enzymes [such as superoxide dismutase (SOD), catalase (CAT), and peroxidase (POD)] and non-enzymatic scavengers [such as carotenoids, Vitamin E (VE), and Vitamin C (VC)] (Edreva, 2005; Szivak et al., 2009; Zhao Q. et al., 2018).
To elucidate the adaptive mechanisms by which C. nivalis survives low temperatures, we investigated the cell growth, photosynthetic activity, and antioxidant mechanisms of this alga. In contrast to the model green alga C. reinhardtii, C. nivalis grows well in low temperatures by maintaining a normal level of photosynthetic activity. Moreover, the CET rate in C. nivalis rapidly rose in cold temperatures, which reduced the damage caused by excess light, while the activities of the antioxidant enzymes were also dramatically enhanced, mitigating the effects of excess ROS production. All above adaptive mechanisms promote the survival and even blooming of C. nivalis under polar environment.
Materials and Methods
Algal Cultures
Chlamydomonas reinhardtii and Chlamydomonas nivalis strains were purchased from Chlamydomonas Resource Center1 and UTEX Culture Collection of Algae2, respectively. C. nivalis (UTEX 2824) and C. reinhardtii were grown in TAP medium, at temperatures of 4, 12, and 22°C with a light intensity of 100 μmol m–2 s–1. The cell biomass was recorded using a cell counter (Z1 Dual Beckman Coulter, United States), and the cell size was observed using a fluorescence microscope (Olympus BX53, Japan).
Pigment Quantifications
Measurement of chlorophyll content followed Chen et al. (2018) and Guan et al. (2018) with some modifications. After 72 h culture of algal cells (106 cells ml–1) grown at 22°C and treated by turning from 22 to 4°C (similarly hereinafter), algal cells were precipitated, respectively, by centrifugation at 4,000 rpm for 5 min at 22 and 4°C. The supernatant was discarded and the pellet was resuspended in 80% acetone, overnight at 4°C, and then centrifuged at 12,000 rpm for 3 min at room temperature. A spectrophotometer was used to determine the concentrations of various photosynthetic pigments using the following formulae (Lichtenthaler, 1987):
Chlorophyll a (Chl a) (mg ml–1) = 12.25 A663.2 − 2.79 A646.8;
Chlorophyll b (Chl b) (mg ml–1) = 21.50 A646.8 − 5.10 A663.2;
Total chlorophylls (Chl a+b) (mg ml–1) = 7.15 A663.2 + 18.71 A646.8;
Total carotenoids (mg ml–1) = (1,000 A470–1.82 Chl a − 85.02 Chl b)/198.
Chlorophyll Fluorescence Analysis
Chlorophyll fluorescence was quantified using a Dual-PAM-100 Chl fluorescence photosynthesis analyzer (Walz, Germany). Intact cells from 22 and 4°C culture were adapted for 15 min in the dark at 22 and 4°C, respectively, prior to measuring their initial (Fo) and maximum (Fm) fluorescence levels. The maximum quantum yield of PSII photochemistry was calculated as Fv/Fm = (Fm − Fo)/Fm, as described by Geller et al. (2018). The parameter 1-qL, which estimates the proportion of closed PSII reaction centers or the excitation pressure of PSII, was calculated using the following formula: 1-qL = 1 − [(Fm′ − F′) / (Fm′ − Fo′) × (Fo′/F′)]. The effective photochemical quantum yield of PSII was calculated as Y (II) = (Fm′ − F)/Fm (Kramer et al., 2004). The non-regulated energy dissipation Y (NO) was calculated as Y(NO) = F/Fm. The regulated energy dissipation Y (NPQ) was calculated as Y (NPQ) = F/Fm′ − F/Fm (Kramer et al., 2004). Y (ND), the non-photochemical energy dissipation caused by donor-side limitations, was calculated as Y (ND) = (P − Po)/Pm. Transient increases in chlorophyll fluorescence after turning off actinic light (AL) were detected as described by Shikanai et al. (1998).
Electron Transfer Rates
The electron transfer rates of PS II, PS I, and CET were monitored using a Clark-type oxygen electrode (Oxylab 2, Hansatech, United Kingdom) at 22 and 4°C, as described by Wang et al. (2008) and Chen et al. (2015a). White light was provided at an intensity of 500 μmol m–2 s–1. Thylakoid membranes were used for all measurements, and the chlorophyll contents were adjusted to 15 mg ml–1. The PSI reaction mixture included 40 mM methylviologen (MV), 5 mM NH4Cl, 2 mM ascorbic acid, 0.1 mM 2,6-dichlorophenolindophenol (DCPIP), 2 mM NaN3, 40 μM 3-(3,4-dichlorophenyl)-1,1-dimethylurea (DCMU), 40 mM tricine (pH 7.5), and 100 mM sucrose. The electrons in the mixture were transferred from DCPIP/ascorbic acid via PSI to MV. One oxygen molecule was consumed for each electron transport event. The PSII reaction mixture contained 5 mM NH4Cl, 4 mM K3FeCN, 1 mM phenyl-p-benzoquinone (BQ), 40 mM tricine (pH 7.5), and 100 mM sucrose and was used to measure the electron transport from H2O via PSII to BQ. One oxygen molecule was produced for every four electrons transported. The CET reaction mixture contained 10 μM 3-(3,4-dichlorophenyl)-1,1-dimethylurea (DCMU), 0.5 mM NADPH, 10 mM NaCl, 5 mM MgCl2, 10 mM KCl, 0.25 mM KH2PO4, and 2 mM NaCl ethylene diamine tetraacetic acid (EDTA), 1 mM MnCl2, and 50 mM 4-(2-hydroxyethyl)-1-piperazineethanesulfonic acid (HEPES, pH 7.6) and was used to measure the electron transport from NADPH via PSI to O2. One oxygen molecule was consumed for each electron transport event. DCMU inhibited the transfer of electrons from PSII. The evolution/consumption of O2 was followed for 3 min, and the rate, V, was calculated as follows: electron transport rates (μmol O2 mg–1 Chl a h–1) = V × 60 × 1,000/30.
Immunoblot Assays
Immunoblot detection was performed as described (Hu et al., 2014; Zhang H. et al., 2018; Zhao X. et al., 2018) with some modifications. Proteins for the SDS-PAGE immunoblot analysis were extracted using 40 mM Tris-HCl (pH 8.0) and then quantified using a BCA Kit (Tiangen, China). Equivalent concentrations of protein for each sample were denatured in 5 × SDS-PAGE loading buffer. Rabbit primary antibodies and goat anti-rabbit secondary antibodies (Sigma-Aldrich, United States) were diluted with skim milk powder (1:3,000 and 1:6,000, respectively). The hybridized proteins were detected with chemiluminescence. Antibody against CAS was produced in rabbits as described by Chen et al. (2015a). Antibodies against D1 and CP43 were produced in rabbits, and the antibody preparation and verification of antibodies specificity were showed in Supplementary Figure S1. The quantification of CAS, D1, and CP43 protein was performed using ImageJ (ver1.41, NIH) (Tsihlis et al., 2010).
Real-Time PCR Analysis
Real-time PCR analysis was performed as described (Zhao et al., 2013, 2015; Chen et al., 2017a; Dong et al., 2018; Zhang J. et al., 2018) with some modifications. Cells were harvested from solutions containing 107 cells ml–1 by centrifugation at 3,000 rpm for 5 min. The precipitate was resuspended in a 1.5 ml Eppendorf tube containing 1 ml TRIZOL reagent (Thermo Fisher Scientific, United States), precipitated in 100% isopropanol, and washed in 75% ethanol. The resulting RNA pellet was suspended in 20 μl DEPC water, after which the RNA was quantified using a NanoDrop (Thermo Fisher Scientific). Aliquots were stored at –70°C.
The expression of CAT, SOD, POD, and a gene encoding the chloroplast-localized Ca2+ sensor (CAS) was analyzed using real-time PCR (Ursula et al., 1996). First-strand cDNA was generated using a PrimeScript RT Reagent Kit with a gDNA Eraser, following the manufacturer’s instructions (#RR047A, Takara Bio, Japan). Specific primers were designed to produce 100- to 200-bp PCR products (Supplementary Table S1) using 18S rRNA and CBLP as references. Quantitative real-time PCR was performed using the 2 × iTaq Universal SYBR Green Supermix (#172, Bio-Rad Laboratories, United States) by a Bio-Rad CFX96 Thermal Cycler. Differences in expression were calculated by melt-curve analysis using Bio-Rad CFX manager software v3.0 (Bio-Rad). The PCR conditions were as follows, 95°C for 1 min followed by 40 cycles of 95°C for 5 s, 55°C for 30 s. The melting curve was completed by 65–95°C and 5 s increased by 0.5°C.
Assessment of Lipid Peroxidation and ROS Scavenging Enzyme Activities
Malondialdehyde (MDA) levels and CAT, POD, and SOD activities were measured as described by Zhang et al. (2013) with some modifications. Cells (107 cells ml–1) were harvested by centrifugation at 4,000 g for 5 min, after which the cell pellet was washed with ultrapure water and resuspended in 0.2 M sodium phosphate buffer (pH 7.8). The resuspended cells were broken using an ultrasonic cell disruptor (Scientz, China) and then centrifuged at 1,800 g for 10 min at 4°C. The supernatants were used to analyze MDA levels and enzyme activities. Their protein contents were assayed using a BCA Protein Quantification Kit (Tiangen Biotech, China). The MDA levels and CAT, POD, and SOD activities were measured using an MDA Assay Kit (Beyotime, China), CAT Activity Assay Kit (Beyotime), POD Activity Assay Kit (Nanjing Bioengineering Institute, China), and SOD Activity Assay Kit (Beyotime), respectively, according to the manufacturer’s instructions. The MDA level (nM mg–1 protein) was calculated as nanomoles of MDA per milligram of protein. CAT activity (U mg–1 protein) was defined as the amount of enzyme that caused a micromole reduction in H2O2 each second per milligram of cellular protein at 37°C. POD activity (U mg–1 protein) was defined as the amount of enzyme that catalyzed a milligram of substrate each minute per milligram of cellular protein at 37°C. SOD activity (U mg–1 protein) was defined as the amount of enzyme that caused a 50% decrease of the SOD-inhabitable NBT per milligram of cellular protein at 37°C.
Thermoluminescence Measurements
High-temperature thermoluminescence (HTL) was measured by Thermoluminescence System TL 400/PMT (Photon Systems Instruments, Brno, Czech Republic). Fifty microliter suspensions of C. reinhardtii and C. nivalis cells (109 cells) were acquired by filtration (0.45 μm, GE Healthcare Life Sciences, United States) pressed against copper film, dark-adapted for 10 min at 20°C, and cooled at 10°C for 1 min. The HTL emissions of the samples were recorded while the suspensions were warmed from 10 to 160°C (heating rate: 0.1°C s–1). N2 gas was used to desiccate samples and prevent any oxidation induced by high temperatures. The instruments were driven by a computer, using a specially developed acquisition program. Detailed instructions can be obtained elsewhere (Ducruet, 2003; Guerrero et al., 2014).
77 K Fluorescence Spectroscopy
Fluorescence emission spectra at 77 K were measured using an F-4700 Fluorescence Spectrophotometer (Hitachi) fitted with a liquid nitrogen cold-finger dewar. Both the excitation and emission widths were 5 nm. The excitation wavelength was 430 nm. Samples were adjusted to a Chl concentration of 15 μg ml–1. Data were normalized at 687 nm.
Statistical Analysis
Four or five biological replicates were performed for each experiment. All data were analyzed using SPSS ver19, with the significance level set to a confidence interval of 95 or 99%. A t-test was applied to evaluate the means and SD of replicated studies. The differences between the control and test values were measured using a one-way ANOVA test, and statistical differences were determined as P < 0.05 or P < 0.01.
Results
C. nivalis Adapts to Low Temperatures
The growth of C. reinhardtii and C. nivalis cells was evaluated at three temperatures (4, 12, and 22°C) to determine the temperature tolerance of the two strains. C. nivalis showed a slightly reduced growth at 12°C compared to its growth at 22°C (Figure 1A), but C. reinhardtii severely retarded growth at 12°C (Figure 1B). Both strains failed to grow at 4°C when cultured statically (Figures 1A,B). However, C. nivalis cells with stationary culture always stuck to the reactor wall, which is not conducive to cell growth. Therefore, shaking culture was used in subsequent culture. C. nivalis showed a rapid increase in cell growth after the 10th day of shaking culture at 4°C. Although C. reinhardtii cultured at 4°C didn’t die for a long time, it consistently failed to grow (Figure 1C). This indicates that C. nivalis adapted to the low temperatures, but C. reinhardtii was more seriously affected by the cold. Therefore, in the following experiments, 22 and 4°C were chosen as the control and low-temperature conditions.
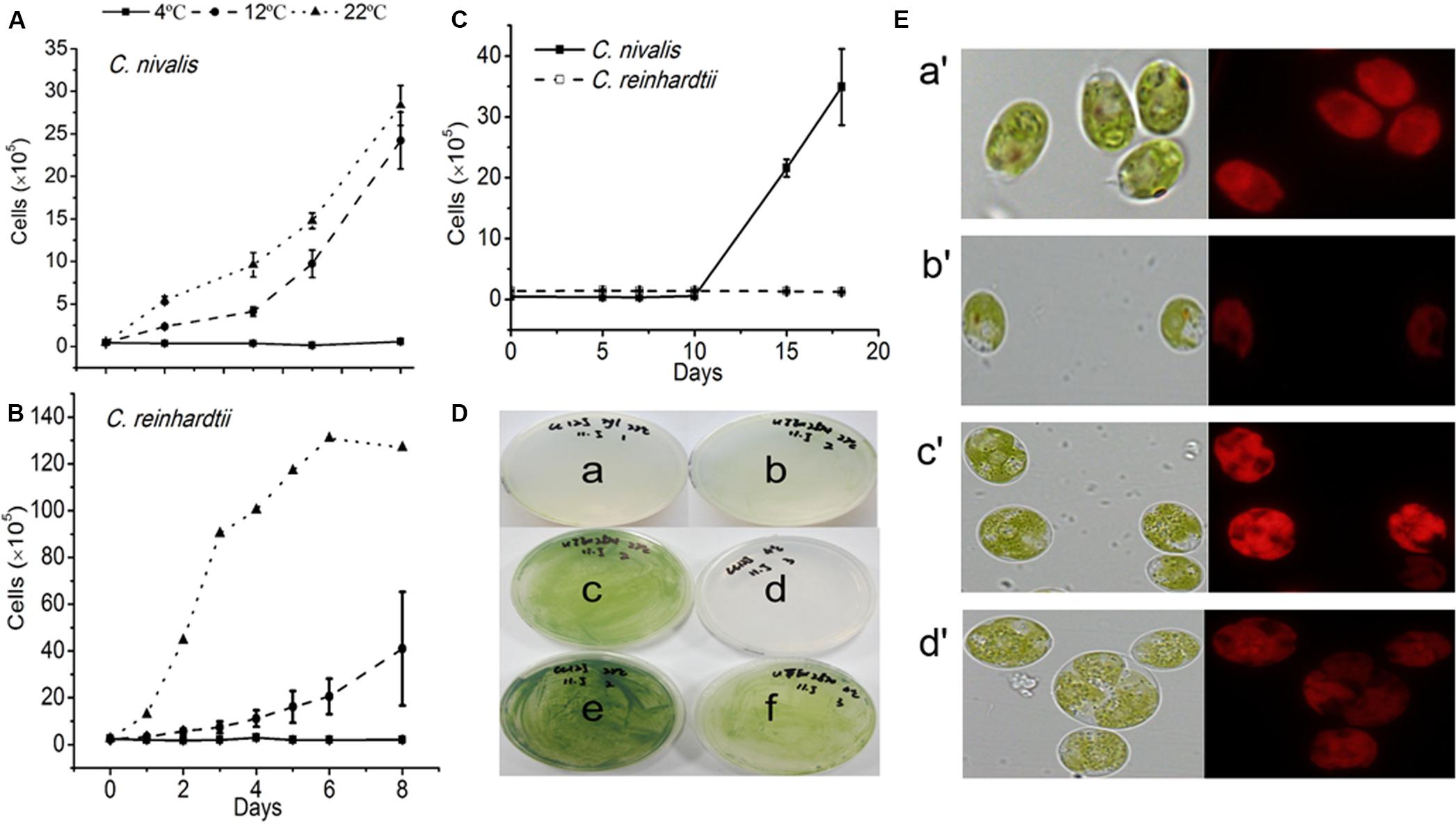
Figure 1. The growth rates and states of C. nivalis and C. reinhardtii at different temperatures. (A,B) Growth curves of C. nivalis and C. reinhardtii under static culture at 4, 12, and 22°C. (C) Growth curves of C. nivalis and C. reinhardtii under shaking culture at 4°C. (D) C. nivalis and C. reinhardtii cells cultured on a solid medium at 22 and 4°C. (a,b) Growth of C. reinhardtii and C. nivalis, respectively, after 0 days. (c,f) Growth of C. nivalis for 20 days at 22 and 4°C, respectively. (d,e) Growth of C. reinhardtii for 20 days at 4 and 22°C, respectively. (E) Changes in cell size and chlorophyll fluorescence based on assessment of 105 cells under 22°C and 4°C. (a′,b′), Changes in C. reinhardtii after 20 days at 22 and 4°C, respectively. (c′,d′) Changes in C. nivalis after 20 days at 22 and 4°C, respectively. All data points in the current and following figures represent the means and SD of four or five biological replicates (t-test, p< 0.05).
C. nivalis and C. reinhardtii growth on solid media was consistent with the results described above (Figure 1D). In addition, while C. reinhardtii displayed a diminished cell size based on assessment of 105 cells and much weaker chlorophyll autofluorescence at 4°C than at 22°C, C. nivalis showed a slightly reduced chlorophyll fluorescence but increased cell size at low temperatures (Figure 1E). The increase in cell size helps C. nivalis to defense against cold with increased cell content, and decreased surface/volume ratio (which means lowered contact with cold). These observations suggest that the larger cell size of C. nivalis at low temperatures may increase its ability to withstand such conditions.
C. nivalis Maintains Robust Photosynthetic Activity at Low Temperatures
Photosynthesis plays an important role in the cellular growth of microalgae and is often affected by adverse conditions. To evaluate the effects of low temperature on photosynthesis, we determined the intracellular chlorophyll contents of C. nivalis and C. reinhardtii at 4°C. In C. nivalis, the contents of both Chl a and Chl b were not significantly affected by the low temperatures, while the photoprotective pigments, carotenoid content, and the Chl a/Chl b ratio, the representative of the reaction center to antenna ratio increased significantly, which may contribute to the low temperature tolerance of this species (Table 1). By contrast, although the carotenoid content and Chl a/Chl b ratios of C. reinhardtii were dramatically increased at low temperatures, the contents of both Chl a and Chl b were significantly reduced, indicating serious damaging effects of low temperatures on photosynthetic pigments.
Fv/Fm represents the maximum quantum yield of PSII photochemistry, and a decrease in Fv/Fm can directly reflect the effect of stresses on photosynthesis (Demetriou et al., 2007; Zhang et al., 2013). A change in chlorophyll fluorescence was determined for C. reinhardtii under low temperature, however, the Fv/Fm of C. nivalis was not significantly affected (Figure 2A). The 1-qL value indicates the proportion of closed PSII centers, which is related to PSII activation. Y (II) represents actual quantum yield of PSII in any illumination levels. The 1-qL and Y (II) values of C. nivalis remained stable under low temperatures, unlike those of C. reinhardtii (Figures 2B,C). All these results indicate that C. nivalis could maintain good photosynthetic activity at low temperatures. By contrast, the rapid decreases of Fv/Fm and Y (II) and the significant increase of 1-qL revealed the inactivation of PSII in C. reinhardtii during the severe stress inflicted at low temperatures.
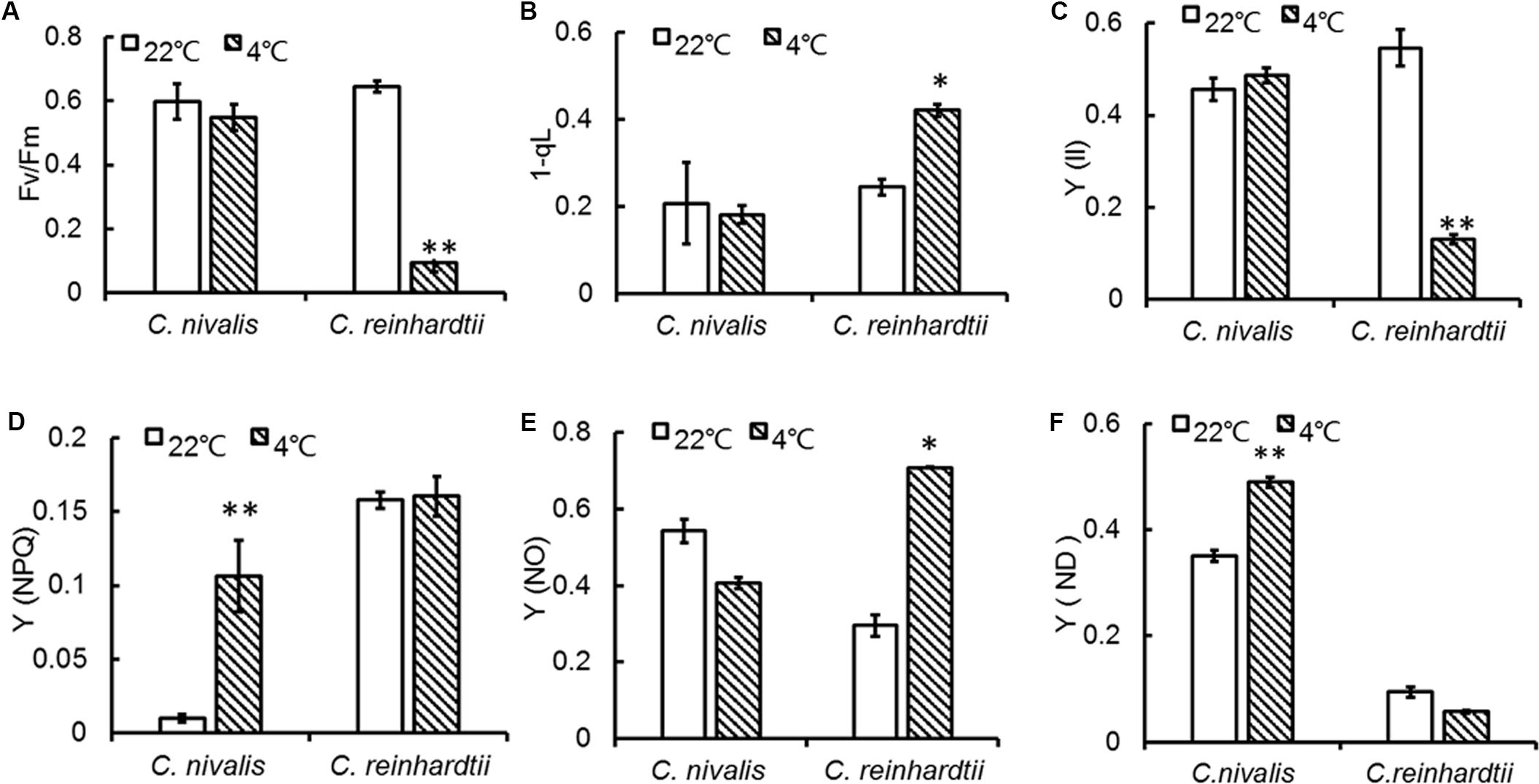
Figure 2. PSII changes in C. nivalis and C. reinhardtii at low temperatures. (A) Fv/Fm, the maximum quantum yield of PSII photochemistry. (B) 1-qL, the proportion of closed PSII centers. (C) Y (II), the effective photochemical quantum yield of PSII. (D) Y (NPQ), regulated energy dissipation. (E) Y (NO), non-regulated energy dissipation. (F) Y (ND), non-photochemical energy dissipation due to donor-side limitation. The significance of the differences between 22 and 4°C in the same strain in the current and following figures were tested using a one-way ANOVA. *p < 0.05, **p < 0.01.
During photosynthesis, some of the unused light energy is dissipated. Y (NPQ) reflects the dissipation of excess light energy in the form of heat, representing photoprotection (Nama et al., 2018). Y (NO) indicates the excess light energy not lost to heat dissipation, representing photodamage (Christof Klughammer, 2008; Erhard and Schreiber, 2008; Nama et al., 2018). The Y (NPQ) of C. nivalis was markedly increased at low temperatures compared to higher temperatures (Figure 2D), while Y (NO) was dramatically increased in C. reinhardtii but slightly decreased in C. nivalis (Figure 2E). These results indicate that PSII of C. nivalis was not damaged at low temperatures and that the excess light energy was dissipated as heat rather than photodamage, thereby maintaining PSII activity.
Y (ND) represents the quantum yield of donor side-limited heat dissipation in PSI, which is induced by the downregulation of Cyt b6f activity and/or PSII damage. The value of Y (ND) in C. nivalis dramatically increased at low temperatures, while for C. reinhardtii, it decreased (Figure 2F). The above results revealed that PSI activation was undamaged in C. nivalis. The electron transfer rates around PSI and PSII were further detected to assess photosynthetic activity (Figure 3). While the PSII electron transfer, PSI electron transfer, and CET were blocked in C. reinhardtii under low temperatures, the electron transfer rate around PSI and PSII, and particularly the CET, of C. nivalis increased at low temperatures, further highlighting the stable photosynthetic system function in this species. Also, the expression of the gene encoding CAS, a protein involved in CET, was upregulated in C. nivalis at 4°C (Figure 3D).
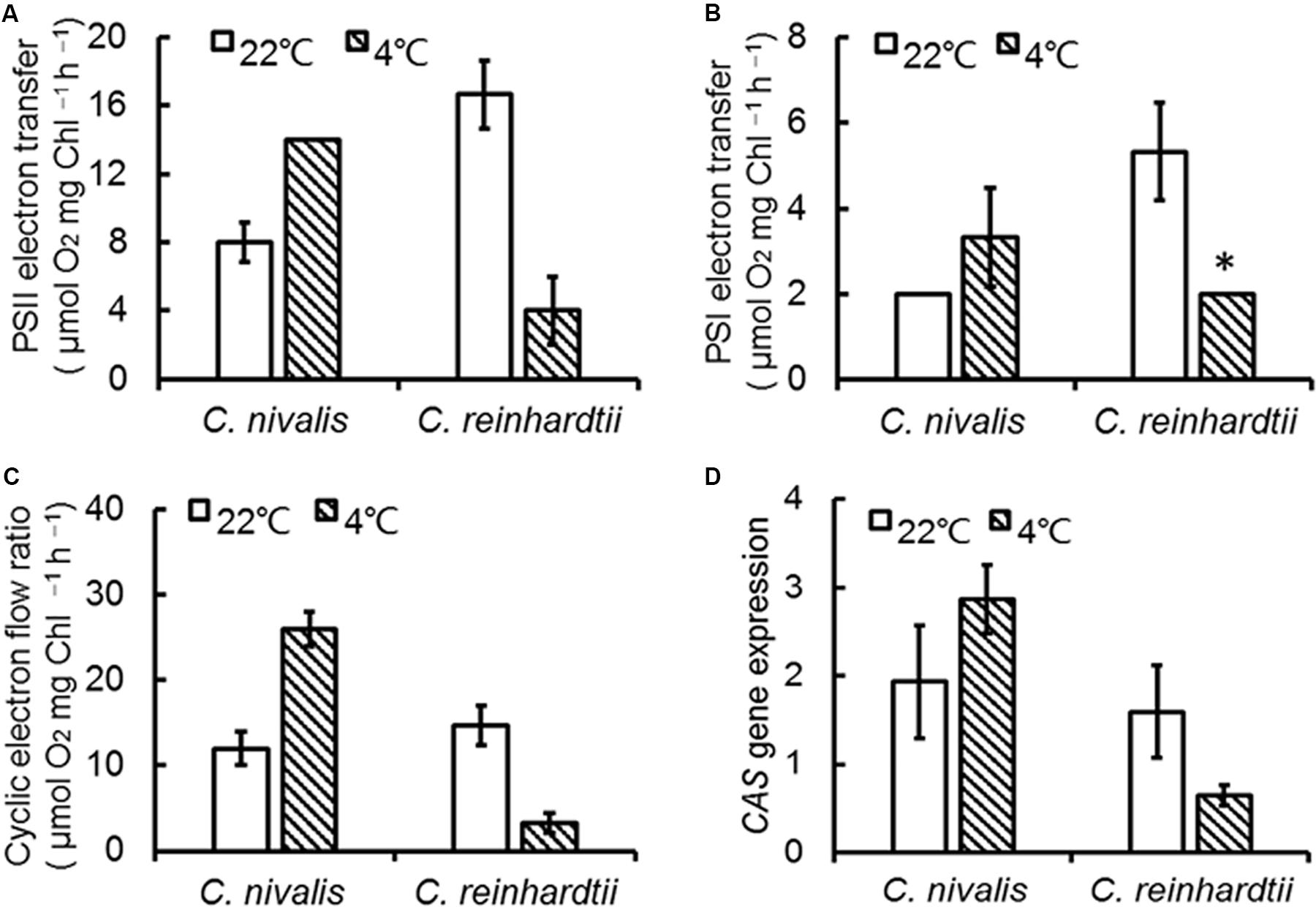
Figure 3. Electron transfer rate of C. nivalis and C. reinhardtii at 22 and 4°C. (A) PSII electron transfer. (B) PSI electron transfer. (C) Cyclic electron transfer. (D) Expression of CAS using real-time fluorescence quantitative PCR. The significance of the differences between 22 and 4°C in the same strain were tested using a one-way ANOVA. *p < 0.05, **p < 0.01.
Furthermore, we determined the protein levels of the PSII central proteins (CP43 and D1) to evaluate the effects of low temperature on PSII. The levels of CP43 and D1 in C. nivalis were similar at 4 and 22°C, and the levels of D1 were significantly higher in C. nivalis than C. reinhardtii at both temperatures (Figure 4B), indicating that low temperature does not affect the integral PSII proteins of C. nivalis, and CP43 in C. nivalis may have been post-translationally modified in C. nivalis, potentially for the adaptation of this species to low temperatures. The possible post-translational modification (PTM) was also detected in the CAS protein in C. nivalis, while the increased level of CAS in this species indicated its improved CET at low temperatures, both of which may have facilitated its adaptation to low temperatures (Figure 4B).
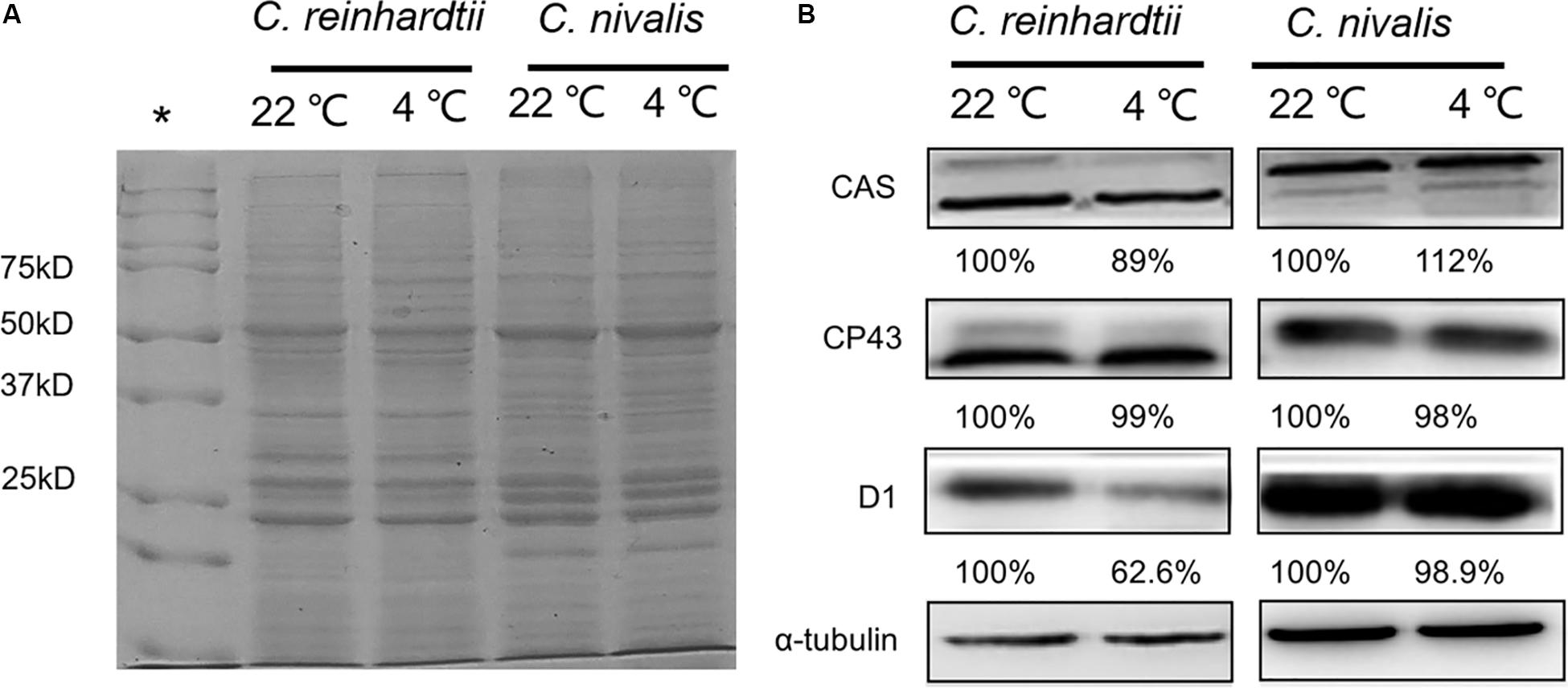
Figure 4. Protein level and relative protein abundance. (A) Representative image of SDS-PAGE analysis of total proteins extracted from C. nivalis and C. reinhardtii at 22 and 4°C as the loading control in the immunoblot analysis. A 20 μg aliquot of proteins from each sample was separated on a 12% SDS-PAGE gel and stained with Coomassie Brilliant Blue. (B) Relative protein abundances of the CAS, CP43, D1, and reference protein α-tubulin. *: Standards.
CET Around PSI and ROS-Scavenging Mechanisms Play Important Roles in the Adaptation to Low Temperatures
Our previous studies revealed that the rate of CET around PSI increased significantly, thereby supplementing ATP, in an adaptive response to environmental stress in Chlorella (Zhang et al., 2013; Chen et al., 2015a). Some studies pointed out the transient rise in chlorophyll fluorescence was due to the reduction of PQ under dark conditions, which was dependent on the activity of NDH, so fluorescence dark rise is indicating the activity of NDH (Kotera et al., 2005). NDH is a multi-subunit complex embedded in the thylakoid membrane that participated in the CET around the PSI (Shikanai, 2007) suggesting the indication of CET by NDH activity. In addition, NDH is also involved in chlororespiration (Desplats et al., 2009). Therefore, the transient fluorescence increase in the dark has been reported as a tool to investigate the CET around PSI or the level of chlororespiration (Houille-Vernes et al., 2011; Peng et al., 2011). C. reinhardtii displayed a typical chlorophyll fluorescence curve at 22°C (Figure 5A). C. nivalis showed an increase in chlorophyll fluorescence at 4°C after termination of AL (Figures 5D,E), however, no increase was detected in C. reinhardtii (Figures 5B,C), suggesting the increase of NDH activity in C. nivalis, which can be influenced by CET or chlororespiration. Furthermore, for determining the change of CET more intuitively, the electron transfer rate of CET was measured using oxygen electrode by providing electron donors and receptors, and the results clearly showed that the CET of C. nivalis increased at low temperatures (Figure 3C). 77 K fluorescence emission spectra of C. nivalis at 22°C showed a major peak at 722 nm (F722), which corresponded to PSI, and a smaller peak at 687 nm (F687), which originated mainly from PSII (Figure 6). However, the increased F722 but decreased F687 at 4°C indicated that PSII was more susceptible at low temperature, but PSI content were significantly enhanced for responding to low temperature. The increased F722 at low temperature correspondence with the increased CET around PSI measured (Figures 3C, 5E). All these results indicate that the CET ratio of C. nivalis was significantly enhanced at low temperatures, suggesting a mechanism that resists stress by enhancing CET at low temperatures.
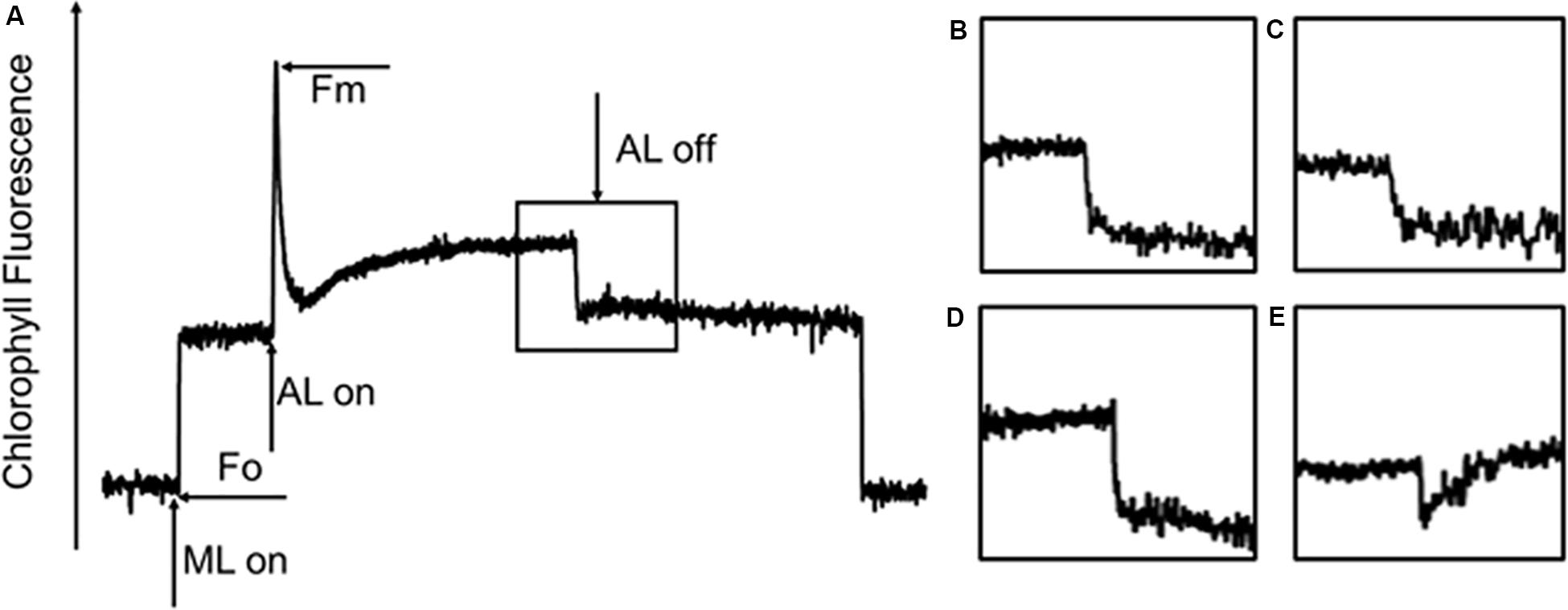
Figure 5. Chlorophyll fluorescence analysis of C. nivalis and C. reinhardtii at 22 and 4°C. (A) Transient post-illumination increase in Chl fluorescence. ML, measure light; AL, actinic light; Fo, initial fluorescence level; Fm, maximum fluorescence level. The curve exhibits a representative trace of Chl fluorescence in C. reinhardtii at 22°C. The black box represents cells exposed to AL (100 μE) for 5 min, after which the AL was turned off. Short-lived increases in chlorophyll fluorescence were detected under low ML. (B–E). Magnified traces from the boxed area. (B) C. reinhardtii at 22°C. (C) C. reinhardtii at 4°C. (D) C. nivalis at 22°C. (E) C. nivalis at 4°C.
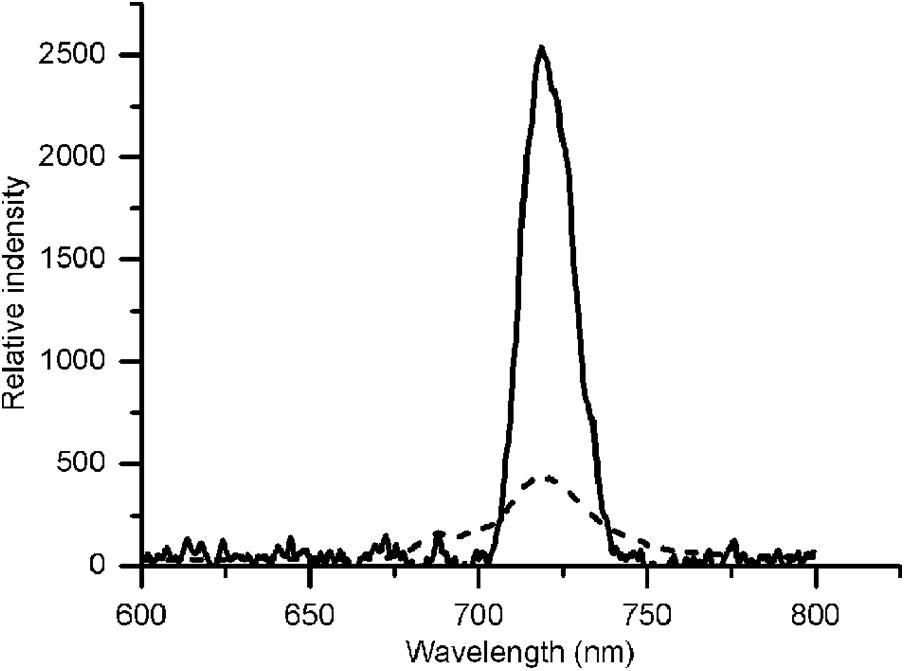
Figure 6. 77 K fluorescence emission spectra of C. nivalis at 22°C and 4°C. Broken line, 22°C- C. nivalis. Solid line, 4°C- C. nivalis.
Higher Chl a/Chl b ratios could decrease the collection of light in relation to the rate of PSII photochemistry, while the increased carotenoid content of C. nivalis had an antioxidative role in trapping excess light that would otherwise be absorbed by the chloroplasts (Table 1). Both of these factors could impact ROS accumulation; therefore, we next measured the lipid peroxidation levels and antioxidant enzyme activities to monitor the level of ROS in the cells. The lipid peroxidation level was evaluated by examining the MDA content and high-temperature thermoluminescence (HTL) of the algae.
The MDA contents of C. reinhardtii were significantly increased under low temperatures, but the MDA content of C. nivalis at 4°C was similar to that at 22°C (Figure 7A), indicating no accumulation of ROS. HTL measurements also reflect the level of lipid peroxidation in photosynthetic organisms (Havaux, 2003). Increasing the temperature from 120 to 140°C can be used to assess the specificity of the band, which represents the level of lipid peroxidation due to triplet carbonyls and singlet oxygens (Vavilin and Ducruet, 1998; Ducruet, 2003; Havaux, 2003). A broad HTL peak at around 135°C represents the level of lipid peroxidation, with taller peaks indicating more extensive lipid peroxidation. At 4°C, C. reinhardtii exhibited very high levels of lipid peroxidation, but the lipid peroxidation level in C. nivalis was not significantly elevated (Figures 8B,D), suggesting that C. nivalis possessed effective ROS scavenging mechanisms under low temperatures. This may also be an important element for the adaptation of C. nivalis to low temperatures.
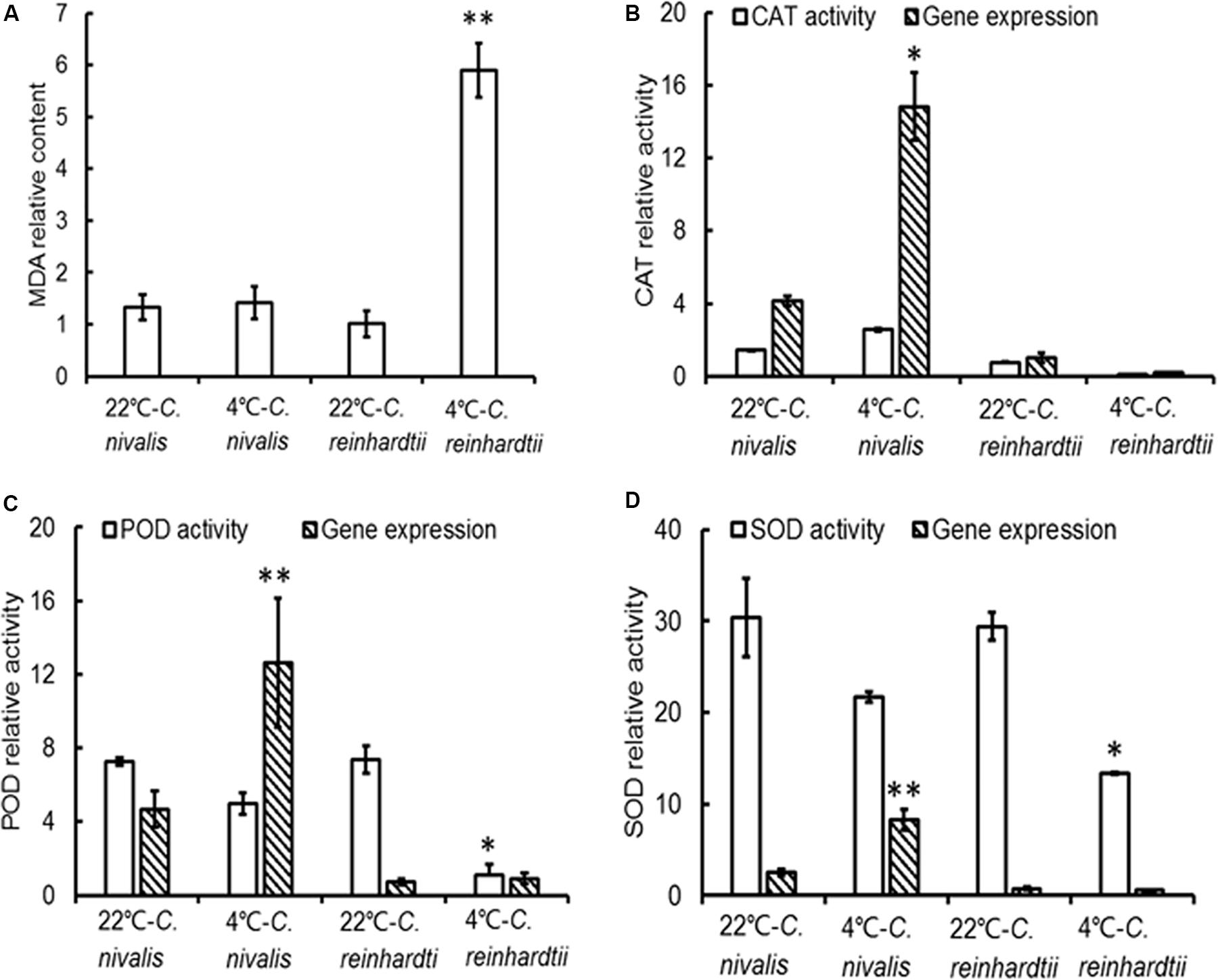
Figure 7. MDA levels, antioxidant enzyme activities, and gene expression in C. nivalis and C. reinhardtii at 22 and 4°C. (A) MDA content (mg prot ml–1). (B) CAT activity (U mg–1 protein s–1) and expression levels. (C) POD activity (U mg–1 prot) and expression levels. (D) SOD activities (U mg–1 prot) and expression levels. The significance of the differences between 22 and 4°C in the same strain were tested using a one-way ANOVA. *p < 0.05, **p < 0.01.
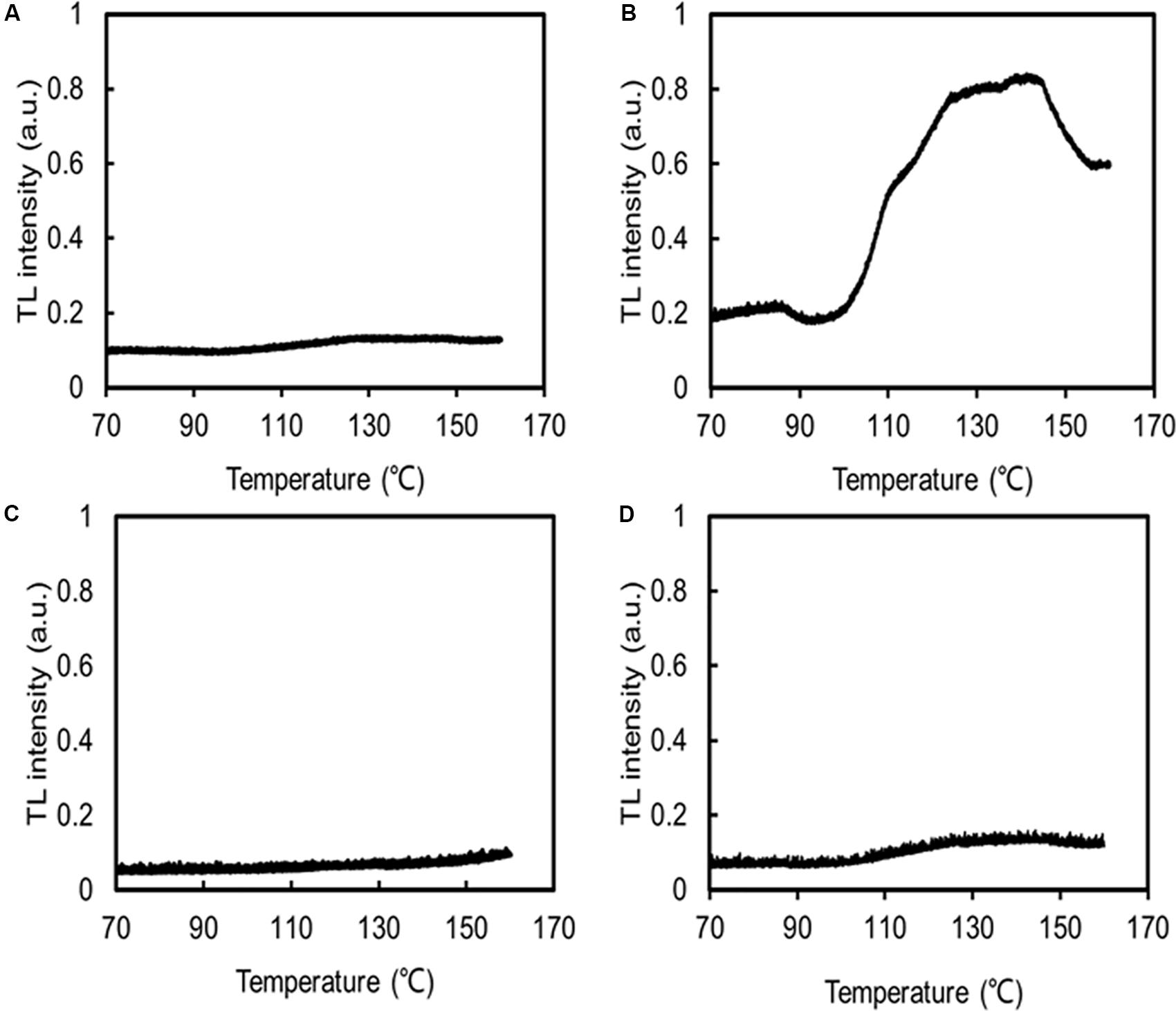
Figure 8. HTL glow curves indicating lipid peroxidation. The same concentrations of C. nivalis and C. reinhardtii were measured. Samples were heated from 10 to 160°C, at a rate of 0.1°C s–1, and their emissions were subsequently recorded. N2 gas was flushed through the samples to dry them and prevent any oxidation induced by high temperatures, a.u. arbitrary units. (A) C. reinhardtii at 22°C. (B) C. reinhardtii at 4°C. (C) C. nivalis at 22°C. (D) C. nivalis at 4°C.
The expression level of genes related to light-harvesting in C. nivalis at 22 and 4°C were detected to evaluate the light-harvesting ability of photosystem (Figure 9). It is found that gene expression levels of chlorophyll-binding protein in light-harvesting complex and PSI were decreased significantly at low temperature. C. nivalis cells were more susceptible to light stress at low temperature, and the reducing light energy absorption can effectively reduce light damage and enhance light protection to avoid ROS accumulation. Furthermore, we evaluated the antioxidant enzyme activities and gene expression levels at low temperatures. Compared with C. reinhardtii, the activity and corresponding gene expression levels of CAT, POD, and SOD were dramatically increased in C. nivalis at 4°C (Figure 7). In particular, the CAT activity increased markedly in C. nivalis from 22 to 4°C (Figure 7B). These antioxidant enzymes may have effectively cleared the intracellular ROS produced in C. nivalis under low temperatures and maintained cellular activity.
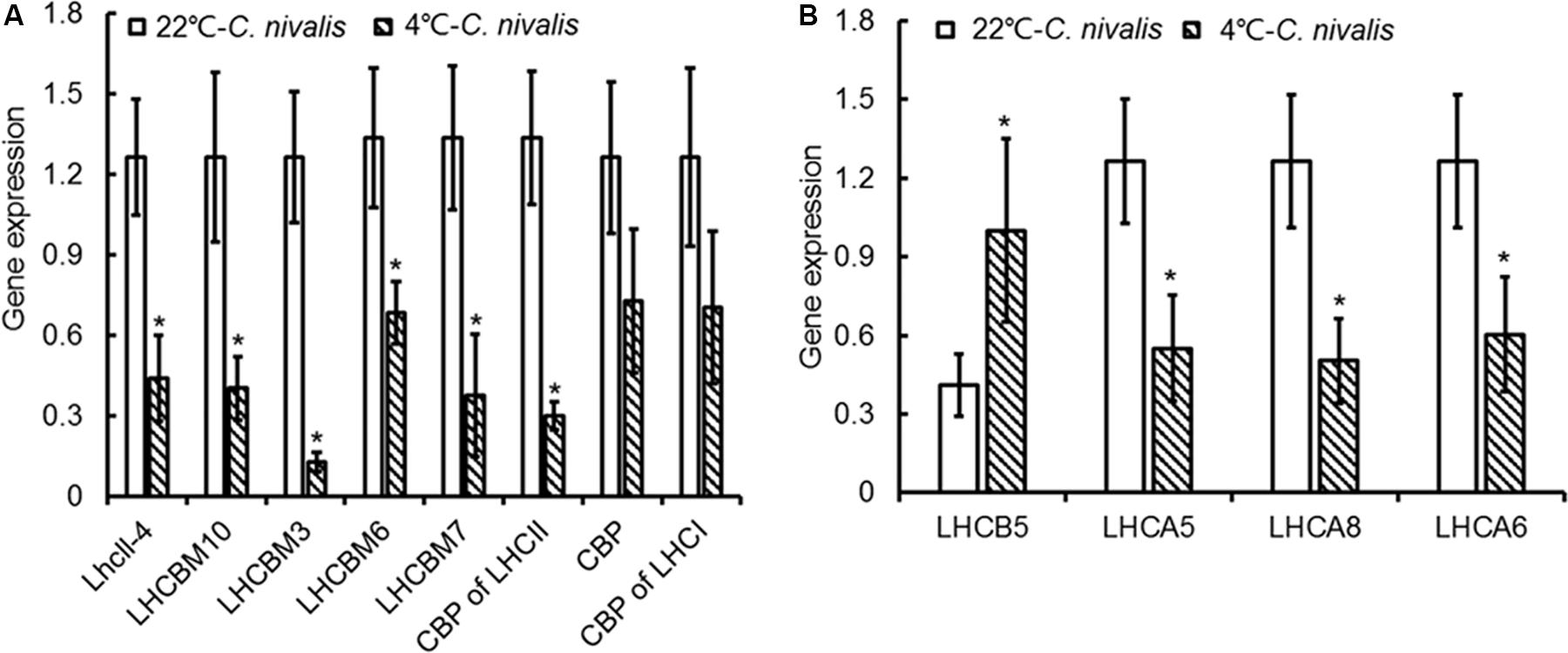
Figure 9. Expression of genes related to light-harvesting in C. nivalis at 22°C and 4°C. (A) Chlorophyll-binding protein in light-harvesting complex. (B) Chlorophyll-binding protein in photosystem. LhcII-4, light-harvesting chlorophyll-a/b binding protein; LHCBM10, light-harvesting complex II protein m10; LHCBM3, light-harvesting complex II chlorophyll-a/b binding protein M3; LHCBM6, chlorophyll-a/b binding protein of LHCII type I, chloroplast precursor; CPB of LHCII, chlorophyll-a/b binding protein of LHCII; CPB, chlorophyll-a/b binding protein; CPB of LHCI, chlorophyll-a/b binding protein of LHCI; LHCB5, light-harvesting chlorophyll-a/b binding protein of PSII; LHCA5, light-harvesting chlorophyll-a/b binding protein of PSI; LHCA8, light-harvesting chlorophyll-a/b binding protein of PSI; LHCA6, light-harvesting chlorophyll-a/b binding protein of PSI. The significance of the differences between 22 and 4°C in the C. nivalis were tested using a one-way ANOVA. *p < 0.05, **p < 0.01.
In addition, the increased gene expression of POD and SOD in C. nivalis under low temperatures contrasted with their reduced enzymatic activity (Figures 7C,D), suggesting that POD and SOD were regulated at the transcriptional level.
Discussion
All environmental conditions influence the metabolic pathways in cells. Specialized microalgae culture methods are more susceptible to external conditions, which affect the metabolism of the cultured cells. C. nivalis is an unusual Chlamydomonas in that it can survive and grow well when exposed to cold temperatures (Figure 1), indicating that this species possesses special mechanisms that allow it to adapt to cold environments. This is consistent with the results of Geller et al. (2018), who showed that snow algae possess higher growth rates than typical algae under highlight and low temperatures.
As an important driver of cell growth and other physiological processes in photosynthetic organisms, photosynthesis is often adversely affected under stress conditions. For example, we previously showed that nitrogen starvation, high levels of nitrite, and high levels of ammonia could substantially inhibit the photosynthesis and respiration of Chlorella (Zhang et al., 2013; Zhao et al., 2014; Chen et al., 2015a, 2016; Mo et al., 2015; Li et al., 2016; Wang et al., 2018). Maintaining good levels of photosynthetic activity under stress conditions is a key factor enabling microalgae to adapt to stress conditions. In addition, Johnson and Alric (2012) showed that the acetate in TAP medium had the impact on the dark redox state of the chloroplast in C. reinhardti. Both inorganic and organic carbon are contained in TAP medium, indicating that two Chlamydomonas strains in this study grew mixotrophically. Heifetz et al. (2000) also pointed out that mixotrophy with acetate did not affect algal growth, PSII efficiency, chlorophyll content and respiration of Chlamydomonas. In this study, we focused on the detection and compare of photosynthetic system activity including algal growth, PSII efficiency and chlorophyll content (Figures 1–4 and Table 1), so the addition of acetate would not interference on the results.
Degrenne et al. (2010) reported that when Chlamydomonas cells were culture mixotrophically with organic carbon and inorganic carbon together, acetate was initially utilized as an organic carbon source and was almost completely exhausted after about 4 day, and then inorganic carbon was utilized subsequently. In this study, two Chlamydomonas strains were cultured at normal temperature for 5 day, and the acetate might completely consumed before low temperature culture of 72 h, which should be few additional effect on the changes of photosynthetic activity. In addition, Terauchi et al. (2010) pointed out that organic carbon in the medium could ensure steady respiration when photosynthesis was affected. Form Figure 1C, it was observed that when C. nivalis with low cell density was cultured directly at low temperature, photosynthesis and cell activity were largely suppressed and respiration might be maintained by acetate for subsequent recovery. Therefore, it is suggested that C. nivalis needs the presence of organic matter to help it adapt to low temperature, and snow algae may form a mutually beneficial symbiotic relationship with other microorganisms in their original habitat, by promoting the recycling of limited nutrients via various metabolic ways.
In this study, we showed that, unlike the severe damage caused to the photosynthetic system in C. reinhardtii by low temperatures, C. nivalis maintained a robust level of photosynthetic activity under low temperatures, as shown by its sustained levels of cell growth, photosynthetic pigment concentrations, chlorophyll fluorescence, electron transfer rate, and photosystem-related proteins and gene expression (Figures 1–4 and Table 1). In addition, this species displayed an increased ratio of Chl a/Chl b, caused by the changes in the ratio between the central protein of PSII and the peripheral light-harvesting proteins, and the decrease of genes expression related to light-harvesting; that is, the light-harvesting ability of PSII and PSI decreased, indicating that C. nivalis reduced light damage by reducing light-harvesting to adapt to low temperatures (Table 1 and Figure 9). Y (II) and Y (NPQ) were significantly increased in the C. nivalis cells at low temperatures, while 1-qL and Y (NO) were decreased, indicating that C. nivalis could increase its light-protective ability by increasing the dissipation of excess light energy as heat and by reducing the light-harvesting capacity of PSII to maintain typical levels of photosynthesis and protect the cell (Figure 2).
Protein PTMs are a vital cellular control mechanism for modulating the diverse properties of proteins and regulating their function (Hart and Ball, 2013). Among the hundreds of different PTMs, lysine acetylation is a highly dynamic and tightly regulated PTM. CP43 is the main subunit involved in the light-harvesting activity of PSII, as well as in the electron transfer reactions. In a previous study, we found that CP43 was acetylated in the cyanobacterium Synechocystis sp. PCC 6803 and that this lysine acetylation played a regulatory role in photosynthesis under high-light conditions (Mo et al., 2015). In addition, phosphorylation is an important protein PTM. One study showed that the level and function of CAS under high light were both regulated by phosphorylation and that the higher the light level, the more significant the phosphorylation of CAS (Vainonen et al., 2008). This phosphorylated protein therefore indicates the light changes in the chloroplasts and transmits signals through direct protein–protein interactions, playing a role in the signal cascade reaction coordinating plant growth and the response to environmental stress (Vainonen et al., 2008). In this study, possible protein PTMs might be also observed in CP43 and CAS in C. nivalis (Figure 4), suggesting that phosphorylation or lysine acetylation took effect in the transmission of stress signals and the corresponding regulation of the photosynthetic process, contributing to the adaptation of this species to low temperatures. D1 is the primary target of photooxidative damage and is rapidly degraded and replaced by the de novo biosynthesis of subunits.
In a study by Warner et al. (1999), PSII activity was significantly decreased, with a significant decline in the D1 protein content due to its greater rate of degradation than de novo biosynthesis. They also pointed out that increasing the conversion rate of the D1 protein could significantly reduce photoinhibition. High D1 protein contents could effectively delay PSII degradation under stress, maintaining photosynthesis and prolonging carbohydrate biosynthesis (Scoma et al., 2012). In this study, the decrease in D1 protein levels observed in C. reinhardtii were consistent with the significant decrease in PSII activity observed in these cells (Figures 2, 4). By comparison, the expression levels of the D1 protein in C. nivalis were relatively stable both at 4 and 22°C; therefore, C. nivalis effectively maintained robust photosynthetic activity under low temperatures (Figures 2, 4).
CET plays an important role in enabling photosynthetic organisms to tolerate or adapt to adverse conditions (Zhao et al., 2014), with the basic function of balancing the ratio between NADPH and ATP. Even under normal conditions, this CET function is indispensable for C3 plants (Yamori and Shikanai, 2016). Some studies have shown that, under nitrogen deficiency, CET was gradually enhanced to supplement ATP and regulate the ATP/NADPH balance during the reduction of photophosphorylation and respiratory phosphorylation in Chlorella (Zhang et al., 2013; Chen et al., 2014, 2015a; Li et al., 2016). In this study, the transient rise of chlorophyll fluorescence in the dark, which can be influenced by CET or chlororespiration, was used to evaluate the response process of C. nivalis at low temperature (Shikanai, 2007; Desplats et al., 2009). Segura and Quiles (2015) demonstrated that chlororespiration and other routes of electron input to the electron transfer chain was probably essential when plants are subjected to intense freeze damage, suggesting the important role of chlororespiration in coping with environmental stress. The fluorescence dark rise in C. nivalis suggests that CET, chlororespiration or both play roles in responding to low temperatures (Figures 5D,E). Oxygen electrode was also applied in measurement of the electron transfer rate of CET by providing electron donors and receptors, which clearly showed the increase in CET rate of C. nivalis at low temperatures (Figure 3C). Thus, the increased CET rate in C. nivalis under low temperatures may contribute to ATP supplement and the consumption of light energy. The excess excitation energy in this species could therefore be recovered and the ATP/NADPH balance could be regulated to protect PSI and avoid ROS accumulation and membrane lipid peroxidation. In addition, the possible role of chlororespiration in the response of C. nivalis to low temperature should be further confirmed.
Besides the increased CET rate, the increased carotenoid content and the activity of the antioxidant enzymes in C. nivalis under low temperatures may also have played a role in ROS scavenging, as demonstrated by the reduced ROS levels revealed by the results of the MDA and HTL analyses (Figures 7, 8 and Table 1). In oxygenic photosynthetic organisms, most stress conditions can ultimately be described as oxidative stresses, which generate adverse effects on cell physiological function (Elstner, 1991). The ROS scavenging mechanisms above contributed to the maintenance of robust photosynthesis levels in C. nivalis and its adaptation to low temperatures by protecting these cells from ROS damage induced by the adverse conditions.
Microalgae can be virtually found everywhere close the surface of the planet (Chen et al., 2019). From the hot equator to the perennially frozen polar regions, algae can be found in seas, rivers, lakes, wet surfaces, rocks, even deserts, and snow. Photosynthetic microalgae are the main contributors to primary production, and algal photosynthesis accounts for almost more than half of the total global primary productivity (Iriarte and Purdie, 1994; Chen et al., 2017b). Especially in a particular ecological environment, microalgae are important primary producers that provide nutrients to other native organisms through photosynthesis (Pulz et al., 2000; Mock and Thomas, 2005; Gattuso et al., 2006). In polar regions, severe environment and climatic conditions limit the spread of terrestrial higher plants, and cold-loving or cold-tolerant algae become the most important photosynthetic primary producers in polar habitats, and form the basis of polar carbon cycle. Williams et al. (2003) detected the photosynthesis of the snow alga community and found that the alga community could fix 2300 μmol CO2 m–2 day–1, and about 3.1 g CO2 per month. With the adaptive mechanisms related to photosynthetic regulation, snow algae such as C. nivalis can fix CO2 through photosynthesis, release oxygen, and produce organic matter (e.g., organic acid, polysaccharide) for providing a comfortable breeding environment for other native organisms. C. nivalis and other organisms use different metabolic modes to form a harmonious symbiosis, which promotes the recycling of limited nutrient elements, playing an important role in snow carbon cycle and energy cycle.
In summary, as shown in Figure 10A photosynthesis of C. reinhardtii was seriously damaged by low temperature, indicated by the significantly decreased photosynthetic pigments (Chl a, Chl b, and Car) content and photosynthetic activity, and led to photo-oxidative damage to the membrane system, proteins, lipids and DNA inside the cell. As a result, C. reinhardtii cells failed to survive under low temperatures. As a representative snow alga, C. nivalis maintains a robust level of photosynthetic activity under low temperatures, promoting its survival and cellular growth. The possible adaptive mechanisms responsible for this are displayed in Figure 10B. Under low temperatures, two main processes promote photosystem fitness and maintained cellular growth in C. nivalis. The light-harvesting ability of PSI and PSII are reduced, which decreases the damage to the photosystem caused by the accumulation of excess light energy. Furthermore, the excess light energy is consumed by CET around PSI, which produces ATP for cellular growth and other physiological processes. Simultaneously, the excess ROS originating from the transport of electrons (from the photosynthesis pathway or other metabolic pathways) to O2 were eliminated by the CET-mediated regulation of the ATP/NADPH balance, carotenoids, and antioxidant enzymes, all of which prevent the oxidative damage of intracellular membrane systems, proteins, lipids, and DNA. These adaptive mechanisms related to photosynthetic regulation not only promote the survival and even blooming of C. nivalis under polar environment, but also provide organic carbon sources for the surrounding polar organisms and contribute to the maintenance of polar ecosystems.
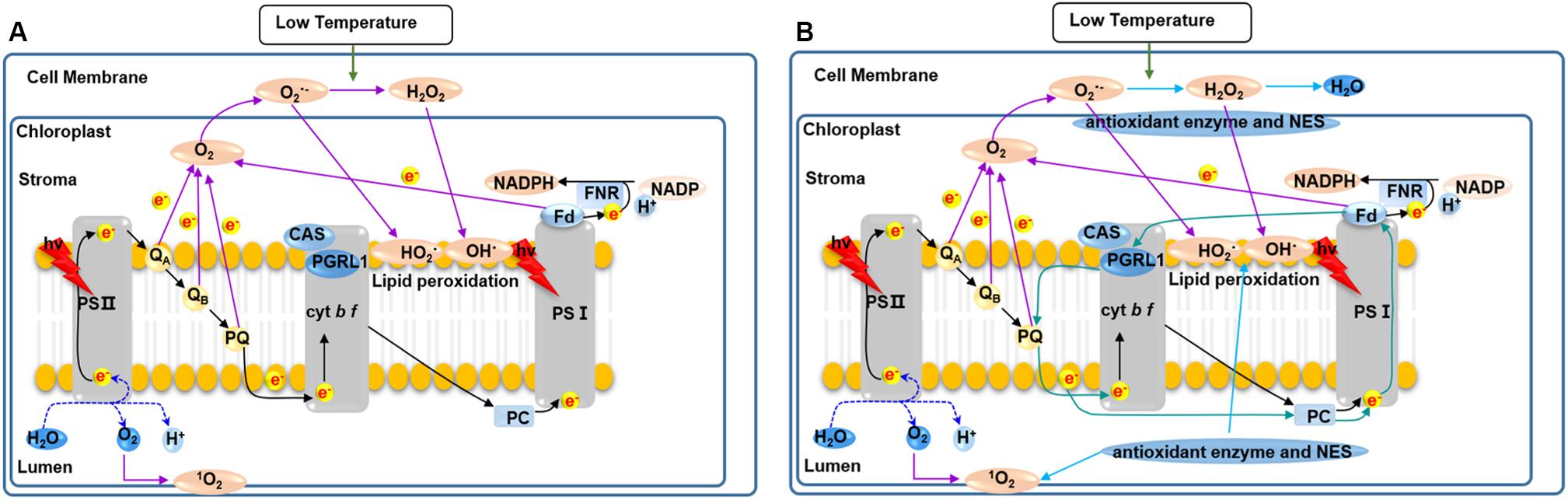
Figure 10. An outline of the putative response mechanisms of C. nivalis and C. reinhardtii at low temperatures. (A) C. reinhardtii. (B) C. nivalis. Black arrows, linear electron transfer. Atrovirens arrows, cyclic electron transport. Purple arrows, ROS generation. Blue arrows, ROS scavenging. NES, non-enzymatic ROS scavengers. When C. nivalis is exposed to low temperatures, the cyclic electron flow helps to eliminate excess light energy, while the activities of the antioxidant enzymes reduce oxidative damage.
Data Availability Statement
All datasets generated for this study are included in the article/Supplementary Material.
Author Contributions
YZ and QW designed the study. YZ, CX, HC, CH, and QW collected, analyzed, and interpreted the data. YZ, HC, and QW wrote the manuscript.
Funding
This work was supported jointly by the National Natural Science Foundation of China (Grant Nos. 91851201, 91851103, 31870041, and 31770128), and Hubei Provincial Natural Science Foundation of China (Grant No. 2017CFA021).
Conflict of Interest
The authors declare that the research was conducted in the absence of any commercial or financial relationships that could be construed as a potential conflict of interest.
Supplementary Material
The Supplementary Material for this article can be found online at: https://www.frontiersin.org/articles/10.3389/fmicb.2020.01233/full#supplementary-material
Footnotes
References
Apel, K., and Hirt, H. (2004). Reactive oxygen species: metabolism, oxidative stress, and signal transduction. Annu. Rev. Plant Biol. 55, 373–399. doi: 10.1146/annurev.arplant.55.031903.141701
Bidigare, R. R., Ondrusek, M. E., Kennicutt, M. C., Iturriaga, R., Harvey, H. R., Hoham, R. W., et al. (1993). Evidence for a photoprotective function for secondary carotenoids of snow algae. J. Phycol. 29, 427–434. doi: 10.1111/j.1529-8817.1993.tb00143.x
Chen, H., Hu, J., Qiao, Y., Chen, W., Rong, J., Zhang, Y., et al. (2015a). Ca(2+)-regulated cyclic electron flow supplies ATP for nitrogen starvation-induced lipid biosynthesis in green alga. Sci. Rep. 5:15117. doi: 10.1038/srep15117
Chen, H., Li, T., and Wang, Q. (2019). Ten years of algal biofuel and bioproducts: gains and pains. Planta 249, 195–219. doi: 10.1007/s00425-018-3066-8
Chen, H., Qiu, T., Rong, J., He, C., and Wang, Q. (2015b). Microalgal biofuel revisited: an informatics-based analysis of developments to date and future prospects. Appl. Energy 155, 585–598. doi: 10.1016/j.apenergy.2015.06.055
Chen, H., Wang, J., Zheng, Y., Zhan, J., He, C., and Wang, Q. (2018). Algal biofuel production coupled bioremediation of biomass power plant wastes based on Chlorella sp. C2 cultivation. Appl. Energy 211, 296–305. doi: 10.1016/j.apenergy.2017.11.058
Chen, H., Zhang, Y., He, C., and Wang, Q. (2014). Ca2+ signal transduction related to neutral lipid synthesis in an oil-producing green alga Chlorella sp. C2. Plant Cell Physiol. 55, 634–644. doi: 10.1093/pcp/pcu015
Chen, H., Zheng, Y., Zhan, J., He, C., and Wang, Q. (2017a). Comparative metabolic profiling of the lipid-producing green microalga Chlorella reveals that nitrogen and carbon metabolic pathways contribute to lipid metabolism. Biotechnol. Biofuels 10:153. doi: 10.1186/s13068-017-0839-4
Chen, H., Zhou, W., Chen, W., Xie, W., Jiang, L., Liang, Q., et al. (2017b). Simplified, rapid, and inexpensive estimation of water primary productivity based on chlorophyll fluorescence parameter Fo. J. Plant Physiol. 211, 128–135. doi: 10.1016/j.jplph.2016.12.015
Chen, W., Zhang, S., Rong, J., Li, X., Chen, H., He, C., et al. (2016). Effective biological DeNOx of industrial flue gas by the mixotrophic cultivation of an oil-producing green alga Chlorella sp. C2. Environ. Sci. Technol. 50, 1620–1627. doi: 10.1021/acs.est.5b04696
Christof Klughammer, U. S. (2008). Complementary PS II quantum yields calculated from simple fluorescence parameters measured by PAM fluorometry and the saturation pulse method. PAM Appl. Notes 1, 27–35.
Cvetkovska, M., Hüner, N. P. A., and Smith, D. R. (2016). Chilling out: the evolution and diversification of psychrophilic algae with a focus on Chlamydomonadales. Polar Biol. 40, 1169–1184. doi: 10.1007/s00300-016-2045-4
Degrenne, B., Pruvost, J., Christophe, G., Cornet, J. F. O., Cogne, G., and Legrand, J. (2010). Investigation of the combined effects of acetate and photobioreactor illuminated fraction in the induction of anoxia for hydrogen production by Chlamydomonas reinhardtii. Int. J. Hydrogen Energy 35, 10741–10749. doi: 10.1016/j.ijhydene.2010.02.067
Demetriou, G., Neonaki, C., Navakoudis, E., and Kotzabasis, K. (2007). Salt stress impact on the molecular structure and function of the photosynthetic apparatus–the protective role of polyamines. Biochim. Biophys. Acta 1767, 272–280. doi: 10.1016/j.bbabio.2007.02.020
Desplats, C., Mus, F., Cuine, S., Billon, E., Cournac, L., and Peltier, G. (2009). Characterization of Nda2, a plastoquinone-reducing type II NAD(P)H dehydrogenase in Chlamydomonas chloroplasts. J. Biol. Chem. 284, 4148–4157. doi: 10.1074/jbc.M804546200
Dong, H., Bai, L., Zhang, Y., Zhang, G. Z., Mao, Y. Q., Min, L. L., et al. (2018). Modulation of guard cell turgor and drought tolerance by a peroxisomal acetate-malate shunt. Mol. Plant 11, 1278–1291. doi: 10.1016/j.molp.2018.07.008
Ducruet, J. M. (2003). Chlorophyll thermoluminescence of leaf discs: simple instruments and progress in signal interpretation open the way to new ecophysiological indicators. J. Exp. Bot. 54, 2419–2430. doi: 10.1093/jxb/erg268
Edreva, A. (2005). Generation and scavenging of reactive oxygen species in chloroplasts: a submolecular approach. Agric. Ecosyst. Environ. 106, 119–133. doi: 10.1016/j.agee.2004.10.022
Elstner, E. F. (1991). Mechanisms of oxygen activation in different compartments of plant cells. Curr. Top. Plant Physiol. 15, 342–343.
Erhard, P. C. K., and Schreiber, U. (2008). Monitoring the effects of reduced PS II antenna size on quantum yields of photosystems I and II using the Dual-PAM-100 measuring system. PAM Appl. Notes 1, 21–24.
Gattuso, J. P., Gentili, B., Duarte, C. M., Kleypas, J. A., Middelburg, J. J., and Antoine, D. (2006). Light availability in the coastal ocean: impact on the distribution of benthic photosynthetic organisms and their contribution to primary production. Biogeosciences 3, 489–513. doi: 10.5194/bg-3-489-2006
Geller, D. P., Das, K. C., Bagby-Moon, T., Singh, M., Hawkins, G., and Kiepper, B. H. (2018). Biomass productivity of snow algae and model production algae under low temperature and low light conditions. Algal Res. 33, 133–141. doi: 10.1016/j.algal.2018.05.005
Guan, Y. L., Liu, L., Wang, Q., Zhao, J. J., Li, P., Hu, J. Y., et al. (2018). Gene refashioning through innovative shifting of reading frames in mosses. Nat. Commun. 9:1555. doi: 10.1038/s41467-018-04025-x
Guerrero, F., Zurita, J. L., Roncel, M., Kirilovsky, D., and Ortega, J. M. (2014). The role of the high potential form of the cytochrome b559: study of Thermosynechococcus elongatus mutants. Biochim. Biophys. Acta 1837, 908–919. doi: 10.1016/j.bbabio.2014.02.024
Hart, G. W., and Ball, L. E. (2013). Post-translational modifications: a major focus for the future of proteomics. Mol. Cell. Proteomics 12:3443. doi: 10.1074/mcp.E113.036491
Havaux, M. (2003). Spontaneous and thermoinduced photon emission: new methods to detect and quantify oxidative stress in plants. Trends Plant Sci. 8, 409–413. doi: 10.1016/s1360-1385(03)00185-7
Heifetz, P. B., Förster, B., Osmond, C. B., Giles, L. J., and Boynton, J. E. (2000). Effects of acetate on facultative autotrophy in Chlamydomonas reinhardtii assessed by photosynthetic measurements and stable isotope analyses. Plant Physiol. 122, 1439–1445. doi: 10.1104/pp.122.4.1439
Hohmann-Marriott, M. F., and Blankenship, R. E. (2011). Evolution of photosynthesis. Annu. Rev. Plant Biol. 62, 515–548. doi: 10.1146/annurev-arplant-042110-103811
Houille-Vernes, L., Rappaport, F., Wollman, F.-A., Alric, J., and Johnson, X. (2011). Plastid terminal oxidase 2 (PTOX2) is the major oxidase involved in chlororespiration in Chlamydomonas. Proc. Natl. Acad. Sci. U.S.A. 108, 20820–20825. doi: 10.1073/pnas.1110518109
Hu, J., Deng, X., Shao, N., Wang, G., and Huang, K. (2014). Rapid construction and screening of artificial microRNA systems in Chlamydomonas reinhardtii. Plant J. 79, 1052–1064. doi: 10.1111/tpj.12606
Hulatt, C. J., Berecz, O., Egeland, E. S., Wijffels, R. H., and Kiron, V. (2017). Polar snow algae as a valuable source of lipids? Bioresour. Technol. 235, 338–347. doi: 10.1016/j.biortech.2017.03.130
Iriarte, A., and Purdie, D. (1994). Size distribution of chlorophyll-a biomass and primary production in a temperate estuary (Southampton Water)—the contribution of photosynthetic picoplankton. Mar. Ecol. Prog. Ser. 15, 283–297. doi: 10.3354/meps115283
Johnson, X., and Alric, J. (2012). Interaction between starch breakdown, acetate assimilation, and photosynthetic cyclic electron flow in Chlamydomonas reinhardtii. J. Biol. Chem. 287, 26445–26452. doi: 10.1074/jbc.M112.370205
Kotera, E., Tasaka, M., and Shikanai, T. (2005). A pentatricopeptide repeat protein is essential for RNA editing in chloroplasts. Nature 433, 326–330. doi: 10.1038/nature03229
Kramer, D. M., Johnson, G., Kiirats, O., and Edwards, G. E. (2004). New fluorescence parameters for the determination of Q(A) redox state and excitation energy fluxes. Photosynth. Res. 79, 209–218. doi: 10.1023/B:PRES.0000015391.99477.0d
Li, T., Xu, G., Rong, J., Chen, H., He, C., Giordano, M., et al. (2016). The acclimation of Chlorella to high-level nitrite for potential application in biological NOx removal from industrial flue gases. J. Plant Physiol. 195, 73–79. doi: 10.1016/j.jplph.2016.03.006
Liang, J. Y., Xia, J. Y., Liu, L. L., and Wan, S. Q. (2013). Global patterns of the responses of leaf-level photosynthesis and respiration in terrestrial plants to experimental warming. J. Plant Ecol. 6, 437–447. doi: 10.1093/jpe/rtt003
Lichtenthaler, H. K. (1987). Chlorophylls and carotenoids – pigments of photosynthetic biomembranes. Methods Enzymol. 148, 350–382. doi: 10.1016/0076-6879(87)48036-1
Liu, B., Sun, L. R., Ma, L. Y., and Hao, F. S. (2017). Both AtrbohD and AtrbohF are essential for mediating responses to oxygen deficiency in Arabidopsis. Plant Cell Rep. 36, 947–957. doi: 10.1007/s00299-017-2128-x
Lukes, M., Prochazkova, L., Shmidt, V., Nedbalova, L., and Kaftan, D. (2014). Temperature dependence of photosynthesis and thylakoid lipid composition in the red snow alga Chlamydomonas cf. nivalis (Chlorophyceae). FEMS Microbiol. Ecol. 89, 303–315. doi: 10.1111/1574-6941.12299
Matsuzaki, R., Hara, Y., and Nozaki, H. (2012). A taxonomic revision of Chloromonas reticulata (Volvocales, Chlorophyceae), the type species of the genus Chloromonas, based on multigene phylogeny and comparative light and electron microscopy. Phycologia 51, 74–85. doi: 10.2216/11-18.1
Mittler, R. (2002). Oxidative stress, antioxidants and stress tolerance. Trends Plant Sci. 7, 405–410. doi: 10.1016/s1360-1385(02)02312-9
Mo, R., Yang, M., Chen, Z., Cheng, Z., Yi, X., Li, C., et al. (2015). Acetylome analysis reveals the involvement of lysine acetylation in photosynthesis and carbon metabolism in the model cyanobacterium Synechocystis sp. PCC 6803. J. Proteome Res. 14, 1275–1286. doi: 10.1021/pr501275a
Mock, T., and Thomas, D. N. (2005). Recent advances in sea-ice microbiology. Environ. Microbiol. 7, 605–619. doi: 10.1111/j.1462-2920.2005.00781.x
Msanne, J., Xu, D., Konda, A. R., Casas-Mollano, J. A., Awada, T., Cahoon, E. B., et al. (2012). Metabolic and gene expression changes triggered by nitrogen deprivation in the photoautotrophically grown microalgae Chlamydomonas reinhardtii and Coccomyxa sp. C-169. Phytochemistry 75, 50–59. doi: 10.1016/j.phytochem.2011.12.007
Nama, S., Madireddi, S. K., Yadav, R. M., and Subramanyam, R. (2018). Non-photochemical quenching-dependent acclimation and thylakoid organization of Chlamydomonas reinhardtii to high light stress. Photosynth. Res. 139, 387–400. doi: 10.1007/s11120-018-0551-7
Painter, T. H., Duval, B., Thomas, W. H., Mendez, M., Heintzelman, S., and Dozier, J. (2001). Detection and quantification of snow algae with an airborne imaging spectrometer. Appl. Environ. Microbiol. 67, 5267–5272. doi: 10.1128/AEM.67.11.5267-5272.2001
Peng, L., Fukao, Y., Myouga, F., Motohashi, R., Shinozaki, K., and Shikanai, T. (2011). A chaperonin subunit with unique structures is essential for folding of a specific substrate. PLoS Biol. 9:e1001040. doi: 10.1371/journal.pbio.1001040
Procházková, L., Leya, T., Křížková, H., and Nedbalová, L. (2019). Sanguina nivaloides and Sanguina aurantia gen. et spp. nov. (Chlorophyta): the taxonomy, phylogeny, biogeography and ecology of two newly recognised algae causing red and orange snow. FEMS Microbiol. Ecol. 95:fiz064. doi: 10.1093/femsec/fiz064
Pulz, O., Scheibenbogen, K., Sandau, E., Hahlweg, R., and Storandt, R. (2000). Microalgae as a photoautotrophic component in systems of closed material cycles. Russ. J. Plant Physiol. 47, 688–697.
Rezanka, T., Nedbalova, L., Prochazkova, L., and Sigler, K. (2014). Lipidomic profiling of snow algae by ESI-MS and silver-LC/APCI-MS. Phytochemistry 100, 34–42. doi: 10.1016/j.phytochem.2014.01.017
Scoma, A., Krawietz, D., Faraloni, C., Giannelli, L., Happe, T., and Torzillo, G. (2012). Sustained H(2) production in a Chlamydomonas reinhardtii D1 protein mutant. J. Biotechnol. 157, 613–619. doi: 10.1016/j.jbiotec.2011.06.019
Segura, M. V., and Quiles, M. J. (2015). Involvement of chlororespiration in chilling stress in the tropical species Spathiphyllum wallisii. Plant Cell Environ. 38, 525–533. doi: 10.1111/pce.12406
Shikanai, T. (2007). Cyclic electron transport around photosystem I: genetic approaches. Annu. Rev. Plant Biol. 58, 199–217. doi: 10.1146/annurev.arplant.58.091406.110525
Shikanai, T., Endo, T., Hashimoto, T., Yamada, Y., Asada, K., and Yokota, A. (1998). Directed disruption of the tobacco ndhB gene impairs cyclic electron flow around photosystem I. Proc. Natl. Acad. Sci. U.S.A. 95, 9705–9709. doi: 10.1073/pnas.95.16.9705
Song, Y. W., Miao, Y. C., and Song, C. P. (2014). Behind the scenes: the roles of reactive oxygen species in guard cells. New Phytol. 201, 1121–1140. doi: 10.1111/nph.12565
Szivak, I., Behra, R., and Sigg, L. (2009). Metal-induced reactive oxygen species production in Chlamydomonas reinhardtii (Chlorophyceae)(1). J. Phycol. 45, 427–435. doi: 10.1111/j.1529-8817.2009.00663.x
Terauchi, A. M., Peers, G., Kobayashi, M. C., Niyogi, K. K., and Merchant, S. S. (2010). Trophic status of Chlamydomonas reinhardtii influences the impact of iron deficiency on photosynthesis. Photosynth. Res. 105, 39–49. doi: 10.1007/s11120-010-9562-8
Thomas, W. H., and Duval, B. (1995). Sierra-Nevada, California, USA, snow algae - snow albedo changes, algal bacterial interrelationships, and ultraviolet-radiation effects. Arct. Alp. Res. 27, 389–399. doi: 10.2307/1552032
Tsihlis, N. D., Murar, J., Kapadia, M. R., Ahanchi, S. S., Oustwani, C. S., Saavedra, J. E., et al. (2010). Isopropylamine NONOate (IPA/NO) moderates neointimal hyperplasia following vascular injury. J. Vasc. Surg. 51, 1248–1259. doi: 10.1016/j.jvs.2009.12.028
Ursula, E. M., Gibson, C. A. H., and Williams, P. M. (1996). A novel method for real time quantitative RT PCR. Genome Res. 6, 995–1001.
Vainonen, J. P., Sakuragi, Y., Stael, S., Tikkanen, M., Allahverdiyeva, Y., Paakkarinen, V., et al. (2008). Light regulation of CaS, a novel phosphoprotein in the thylakoid membrane of Arabidopsis thaliana. FEBS J. 275, 1767–1777. doi: 10.1111/j.1742-4658.2008.06335.x
Vavilin, D. V., and Ducruet, J. M. (1998). The origin of 115-130 degrees C thermoluminescence bands in chlorophyll-containing material. Photochem. Photobiol. 68, 191–198. doi: 10.1111/j.1751-1097.1998.tb02488.x
Wang, J., Zhou, W., Chen, H., Zhan, J., He, C., and Wang, Q. (2018). Ammonium nitrogen tolerant Chlorella strain screening and its damaging effects on photosynthesis. Front. Microbiol. 9:3250. doi: 10.3389/fmicb.2018.03250
Wang, Q., Jantaro, S., Lu, B., Majeed, W., Bailey, M., and He, Q. (2008). The high light-inducible polypeptides stabilize trimeric photosystem I complex under high light conditions in Synechocystis PCC 6803. Plant Physiol. 147, 1239–1250. doi: 10.1104/pp.108.121087
Warner, M. E., Fitt, W. K., and Schmidt, G. W. (1999). Damage to photosystem II in symbiotic dinoflagellates: a determinant of coral bleaching. Proc. Natl. Acad. Sci. U.S.A. 96, 8007–8012. doi: 10.1073/pnas.96.14.8007
Williams, W. E., Gorton, H. L., and Vogelmann, T. C. (2003). Surface gas-exchange processes of snow algae. Proc. Natl. Acad. Sci. U.S.A. 100, 562–566. doi: 10.1073/pnas.0235560100
Yamori, W., and Shikanai, T. (2016). Physiological functions of cyclic electron transport around photosystem I in sustaining photosynthesis and plant growth. Annu. Rev. Plant Biol. 67, 81–106. doi: 10.1146/annurev-arplant-043015-112002
Yamori, W., Sakata, N., Suzuki, Y., Shikanai, T., and Makino, A. (2011). Cyclic electron flow around photosystem I via chloroplast NAD(P)H dehydrogenase (NDH) complex performs a significant physiological role during photosynthesis and plant growth at low temperature in rice. Plant J. 68, 966–976. doi: 10.1111/j.1365-313X.2011.04747.x
Yamori, W., Shikanai, T., and Makino, A. (2015). Photosystem I cyclic electron flow via chloroplast NADH dehydrogenase-like complex performs a physiological role for photosynthesis at low light. Sci. Rep. 5:13908. doi: 10.1038/srep13908
Yong, Y. Y. R., and Lee, Y. K. (1991). Do carotenoids play a photoprotective role in the cytoplasm of Haematococcus lacustris (Chlorophyta). Phycologia 30, 257–261. doi: 10.2216/i0031-8884-30-3-257.1
Zhan, J., Zhu, X., Zhou, W., Chen, H., He, C., and Wang, Q. (2016). Thf1 interacts with PS I and stabilizes the PS I complex in Synechococcus sp. PCC7942. Mol. Microbiol. 102, 738–751. doi: 10.1111/mmi.13488
Zhang, H., Yue, M. X., Zheng, X. K., Gautam, M., He, S. B., and Li, L. J. (2018). The role of promoter-associated histone acetylation of Haem Oxygenase-1 (HO-1) and Giberellic Acid-Stimulated Like-1 (GSL-1) genes in heat-induced lateral root primordium inhibition in maize. Front. Plant Sci. 9:1520. doi: 10.3389/fpls.2018.01520
Zhang, J., Wang, F. R., Zhang, C. Y., Zhang, J. H., Chen, Y., Liu, G. D., et al. (2018). A novel VIGS method by agroinoculation of cotton seeds and application for elucidating functions of GhBI-1 in salt-stress response. Plant Cell Rep. 37, 1091–1100. doi: 10.1007/s00299-018-2294-5
Zhang, X., Rong, J., Chen, H., He, C., and Wang, Q. (2014). Current status and outlook in the application of microalgae in biodiesel production and environmental protection. Front. Energy Res. 2:32. doi: 10.3389/fenrg.2014.00032
Zhang, Y. M., Chen, H., He, C. L., and Wang, Q. (2013). Nitrogen starvation induced oxidative stress in an oil-producing green alga Chlorella sorokiniana C3. PLoS One 8:e69225. doi: 10.1371/journal.pone.0069225
Zhao, J., Gao, F., Zhang, J., Ogawa, T., and Ma, W. (2014). NdhO, a subunit of NADPH dehydrogenase, destabilizes medium size complex of the enzyme in Synechocystis sp. strain PCC 6803. J. Biol. Chem. 289, 26669–26676. doi: 10.1074/jbc.M114.553925
Zhao, Q., Chen, W. X., Bian, J. Y., Xie, H., Li, Y., Xu, C. X., et al. (2018). Proteomics and phosphoproteomics of heat stress-responsive mechanisms in spinach. Front. Plant Sci. 9:800. doi: 10.3389/fpls.2018.00800
Zhao, X., Wang, J., Yuan, J., Wang, X. L., Zhao, Q. P., Kong, P. T., et al. (2015). NITRIC OXIDE-ASSOCIATED PROTEIN1 (AtNOA1) is essential for salicylic acid-induced root waving in Arabidopsis thaliana. New Phytol. 207, 211–224. doi: 10.1111/nph.13327
Zhao, X., Wang, Y. L., Qiao, X. R., Wang, J., Wang, L. D., Xu, C. S., et al. (2013). Phototropins function in high-intensity blue light-induced hypocotyl phototropism in Arabidopsis by altering cytosolic calcium. Plant Physiol. 162, 1539–1551. doi: 10.1104/pp.113.216556
Keywords: antioxidant enzymes, Chlamydomonas nivalis, cyclic electron transfer, low temperature, photosynthesis
Citation: Zheng Y, Xue C, Chen H, He C and Wang Q (2020) Low-Temperature Adaptation of the Snow Alga Chlamydomonas nivalis Is Associated With the Photosynthetic System Regulatory Process. Front. Microbiol. 11:1233. doi: 10.3389/fmicb.2020.01233
Received: 29 September 2019; Accepted: 14 May 2020;
Published: 10 June 2020.
Edited by:
Weiwen Zhang, Tianjin University, ChinaReviewed by:
Harvey J. M. Hou, Alabama State University, United StatesMatteo Ballottari, University of Verona, Italy
Copyright © 2020 Zheng, Xue, Chen, He and Wang. This is an open-access article distributed under the terms of the Creative Commons Attribution License (CC BY). The use, distribution or reproduction in other forums is permitted, provided the original author(s) and the copyright owner(s) are credited and that the original publication in this journal is cited, in accordance with accepted academic practice. No use, distribution or reproduction is permitted which does not comply with these terms.
*Correspondence: Qiang Wang, d2FuZ3FpYW5nQGhlbnUuZWR1LmNu; d2FuZ3FpYW5nQGloYi5hYy5jbg==