- 1School of Life Sciences, Anhui Medical University, Hefei, China
- 2School of Life Sciences, Hefei Normal University, Hefei, China
Veillonella atypica is a bacterium that is present in the gut and the oral cavity of mammals and plays diverse roles in different niches. A recent study demonstrated that Veillonella is highly associated with marathon running and approved that V. atypica gavage improves treadmill run time in mice, revealing that V. atypica has a high biotechnological potential in improving athlete performance. However, a comprehensive analysis of the genetic diversity, function traits, and genome editing method of V. atypica remains elusive. In the present study, we conducted a systemically comparative analysis of the genetic datasets of nine V. atypica strains. The pan-genome of V. atypica consisted of 2,065 homologous clusters and exhibited an open pan-genome structure. A phylogenetic analysis of V. atypica with two different categories revealed that V. atypica OK5 was the most distant from the other eight V. atypica strains. A total of 43 orthologous genes were identified as CAZyme genes and grouped into 23 CAZyme families. The CAZyme components derived from accessory clusters contributed to the differences in the ability of the nine V. atypica strains to utilize carbohydrates. An integrated analysis of the metabolic pathways of V. atypica suggested that V. atypica strains harbored vancomycin resistance and were involved in several biosynthesis pathways of secondary metabolites. The V. atypica strains harbored four main Cas proteins, namely, CAS-Type IIIA, CAS-Type IIA, CAS-Type IIC, and CAS-Type IIID. This pilot study provides an in-depth understanding of and a fundamental knowledge about the biology of V. atypica that allow the possibility to increase the biotechnological potential of this bacterium.
Introduction
Veillonella atypica, which belongs to family Veillonellaceae and genus Veillonella, is a Gram-negative bacterium and anaerobic coccus. To date, species of genus Veillonella, including V. atypica, but with the exception of Veillonella seminalis (Aujoulat et al., 2014), are well known for their lactate fermenting abilities, allowing them to utilize lactate and transform it to propionate and acetate (Kolenbrander, 2006). The strains of V. atypica can be found in the intestines and the oral mucosa of mammals (Beighton et al., 2008; Vesth et al., 2013) and play diverse roles in different niches. For example, the coaggregation properties of Veillonella spp. of the human oral cavity affect the colonization site and the ecology of oral microbial communities (Hughes et al., 1988). Moreover, oral Veillonella spp., including V. atypica, together with Streptococcus spp., are known as early colonizers in oral biofilm formation and affect the development of dental plaques (Mashima and Nakazawa, 2015). A case report stated that V. atypica, along with Actinomyces odontolyticus, led to pulmonary infection in a 65-year-old, male immunocompetent patient with dental caries (Crisafulli et al., 2019). Besides that, metal reduction by microbes has attracted increased attention (Lloyd, 2003), and numerous microorganisms with the ability to reduce metals have been isolated, identified, and studied (Zhang et al., 2019a, b; Wang et al., 2020). As a metal-reducing bacterium, V. atypica has been identified as a selenium-respiring bacterium and possesses the ability to transform biogenic selenite (Se) (Nancharaiah and Lens, 2015). V. atypica reduces Se(IV) by using hydrogen as electron donors and involves a metal-reducing mechanism different from that of Geobacter sulfurreducens and Shewanella oneidensis (Pearce et al., 2009). Following the effective detoxification of Cr(VI) via a sulfur-based mixotrophic bio-reduction process (Zhang et al., 2020), bioengineering of V. atypica strains will increase their potential applications as selenium-respiring bacterium with an important role in the selenium cycle. However, the genes and/or proteins associated with this mechanism remain elusive. Understanding the functional traits of genes and/or proteins of V. atypica will be beneficial in increasing the ability of this species to transform biogenic selenite. Additionally, a study found that the relative abundance of gut Veillonella is significantly associated with marathon running and V. atypica gavage improves treadmill run time in mice (Scheiman et al., 2019). The mechanism involves crossing of serum lactate from the epithelial barrier into the gut lumen, and then the gut V. atypica transforms lactate to acetate and propionate, which is sufficient to improve treadmill run time (Scheiman et al., 2019). Therefore, understanding the genetic diversity and the functional characteristics of V. atypica will expand its biotechnological applications in environmental restoration and exercise performance.
To date, nine V. atypica strains, namely, V. atypica KON, V. atypica CMW7756B, V. atypica ACS-049-V-Sch6, V. atypica ACS-134-V-Col7a, V. atypica KON ATCC 17744, V. atypica NCTC11830, V. atypica AF36-15BH, V. atypica KHUD_V1, and V. atypica OK5, have been sequenced and their genome sequences can be available in public database. The genome of strain V. atypica OK5 was the first to be completely sequenced among the V. atypica strains. This strain was isolated from a human saliva sample to reveal the genetic transformability among the species of Veillonella genus. Based on the complete genome sequence of V. atypica OK5, a genetic study provided an in-depth understanding of the ecology of human oral biofilms (Zhou et al., 2015). The genome sequences of the other eight strains are incomplete. A study of the genetic diversity of genus Veillonella and 137 prokaryotic genomes, including eight Veillonella spp., suggested that the genus Veillonella is relatively homogeneous (Vesth et al., 2013). However, the genetic diversity and the functional traits of V. atypica, especially concerning the composition of carbohydrate-active enzymes (known as CAZy enzymes or CAZymes) and CRISPR–Cas system, which is widely used in editing the genetic elements of microbiota (Alkhnbashi et al., 2019; Horvath and Barrangou, 2010), remain unclear. Hence, a comprehensive analysis of the characteristics of V. atypica is warranted.
In this study, we collected the available genomic datasets of nine V. atypica strains and conducted a comparative analysis to investigate the strains’ genetic diversity, functional traits, including CAZyme, Cluster of Orthologous Group (COG), Gene Ontology (GO) function, and metabolic pathways, and CRISPR–Cas composition. The results revealed differences in the genetic features of these strains and demonstrated that V. atypica exhibited an open pan-genome structure. The evolutionary trees of nine V. atypica strains constructed with two different strategies showed that the nine V. atypica strains can be divided into two clusters, and V. atypica OK5 may not the suitable representative strain for V. atypica. The differences in the ability of the strains to utilize carbohydrates were derived from the CAZyme component of accessory clusters. Several proteins of V. atypica were annotated to the Kyoto Encyclopedia of Genes and Genomes (KEGG) pathways involved in antibiotic resistance and biosynthesis of secondary metabolites. The V. atypica strains harbored four main Cas proteins, namely, CAS-Type IIIA, CAS-Type IIA, CAS-Type IIC, and CAS-Type IIID.
Results and Discussion
Pan-Genome Construction and Analysis
In general, the use of complete genomes is recommended for estimating the genetic diversity in pan-genome analysis, and the mixture of complete and draft genomes is also allowed in pan-genome analysis (Veras et al., 2018). For example, the genetic diversity of Corallococcus was evaluated on the basis of two complete genomes and 21 draft genomes of Corallococcus strains via pan-genome analysis (Livingstone et al., 2018), and the 11 genomes (five complete and six draft genomes) were used to obtain a first approximation of the Piscirickettsia salmonis pan-genome (Bravo and Martinez, 2016). Therefore, in this present study, to conduct the comparative analysis of species V. atypica, nine available genomic datasets, including complete and draft genomes, and their corresponding protein datasets for V. atypica strains were collected and downloaded from National Center for Biotechnology Information (NCBI). Except for V. atypica OK5, the sequenced genomes of the V. atypica strains were mainly assembled at contig and scaffold levels, and the number of scaffolds ranged from 1 to 94. The assembled genome of V. atypica ranged from 1.99 to 2.19 Mb, whereas the protein number of V. atypica ranged from 1,781 to 1,973 (Table 1). The results showed that the nine V. atypica strains were mainly isolated from the oral cavity, vagina, and feces of Homo sapiens (Table 1). It is important to note that although this study is limited in that the pan-genome analysis was performed with a restricted quantity of fully sequenced genomes, the genetic diversity and the characteristics of V. atypica were explored with mainstream databases and tools of pan-genome analysis, which was used to evaluate the genetic diversity and the functional traits of Aeromonas (Zhong et al., 2019) and Shewanella (Zhong et al., 2018) via pan-genome analysis. This pilot study is an important step toward understanding the functional traits and the functional variants of V. atypica.
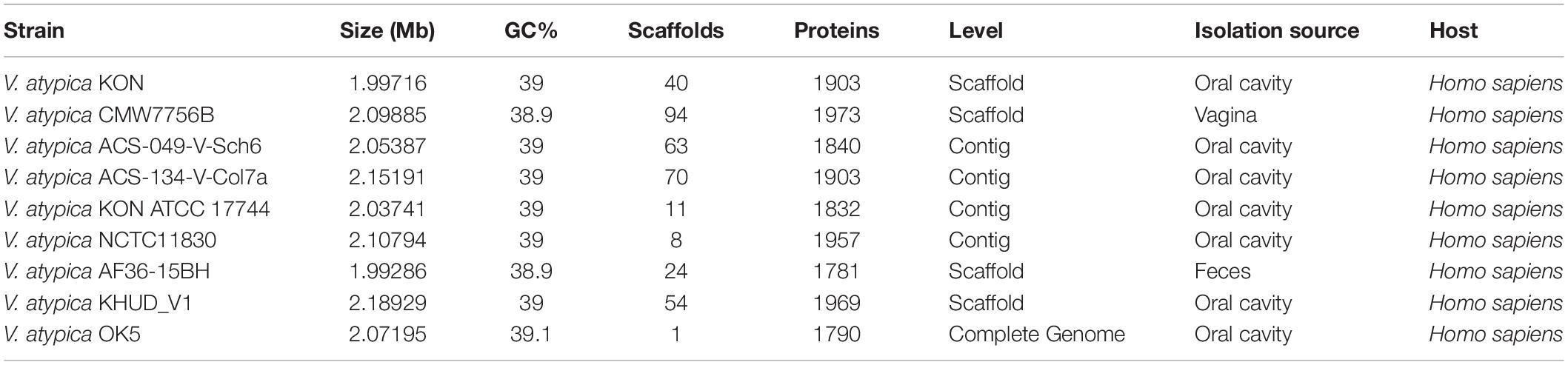
Table 1. Genomic datasets of the nine Veillonella atypica strains used in this study. The genomic features of the nine V. atypica strains were collected from National Center for Biotechnology Information.
To characterize the differences in genomic features among these nine V. atypica strains, the orthologs were identified from 16,948 high-quality proteins of V. atypica. A total of 2,065 homologous clusters were identified from V. atypica strains. Among these homologous clusters, 1,512 homologous clusters (73.22%) were found in all the nine V. atypica strains and accordingly identified as the core genome of V. atypica (Figure 1A). In addition, 1,468 homologous clusters from the core genome were identified as single-copy core families. The number of accessory families of V. atypica ranged from 199 to 376 (Figure 1A), whereas 13 homologous clusters were identified as specific families (unique genes) for the nine V. atypica strains, the number of specific families of which ranged from 0 to 4. Interestingly, no specific families were found in the strains of V. atypica KON and V. atypica KON ATCC 17744. Additionally, the number of genes in the specific families of the other V. atypica strains were all more than two, suggesting that these multi-copy specific genes may play an important role in the adaptation of the V. atypica strains to specific niches.
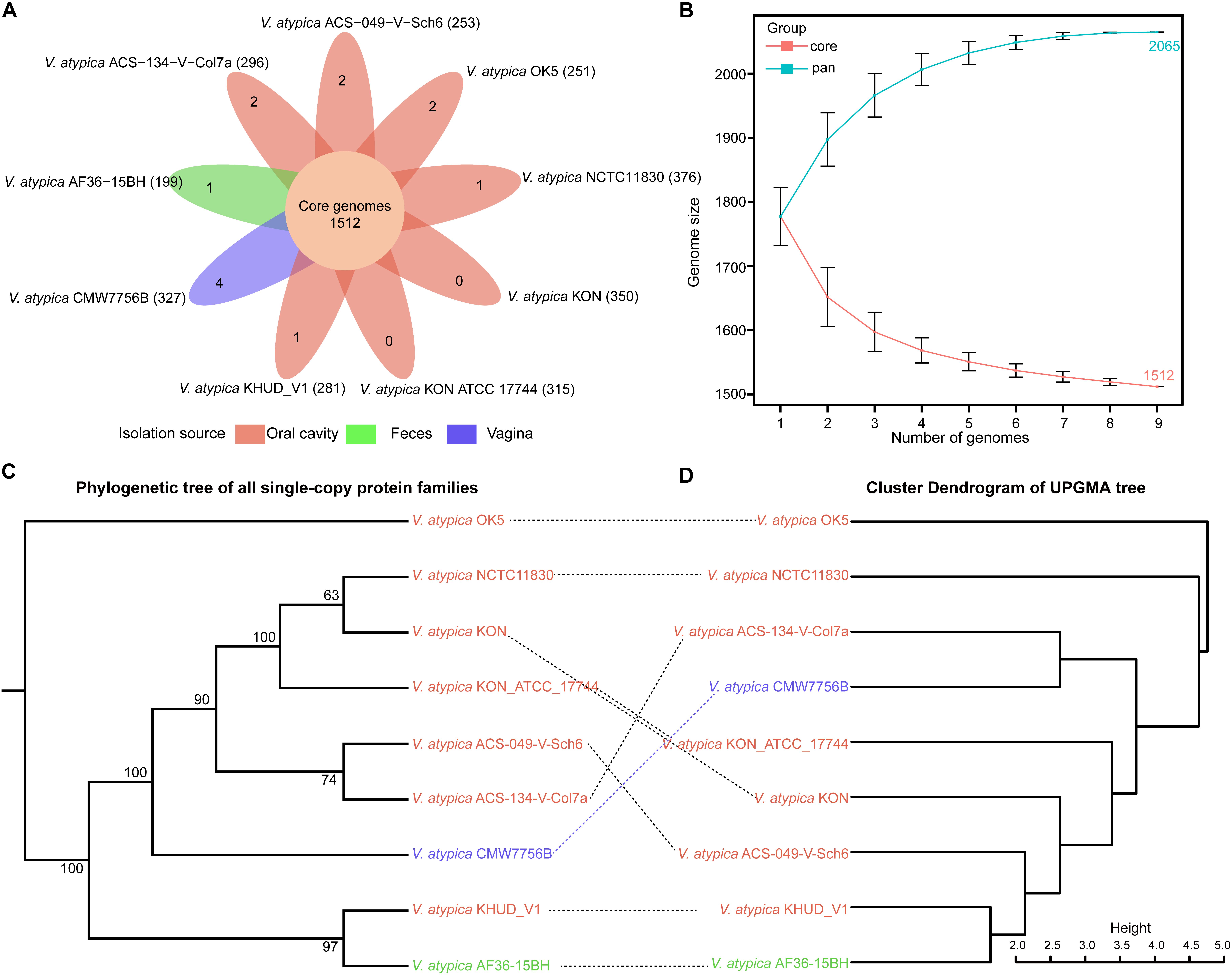
Figure 1. Genetic diversity in Veillonella atypica strains. (A) Core, accessory, and specific gene families of the nine V. atypica strains. The number of core genomes shared by all strains is in the center (1,512). The number of non-overlapping portions of each oval represents the size of specific families (unique genes). The number of accessory families of each strain is shown in parentheses after the strain name. (B) The size of pan-genome (top) and core genome (bottom) shared by different strains, respectively. Phylogenetic relationship of nine V. atypica strains. (C) Phylogenetic tree based on all single-copy protein sequences. (D) The UPGMA tree of Manhattan distance based on the pan-genome composition of the nine V. atypica strains.
Moreover, the accumulation curve of homologous clusters has just reached the plateau stage, which suggested that V. atypica exhibited an open pan-genome structure (Figure 1B). With the emergence of new V. atypica strains, the pan-genome size of V. atypica tended to increase gradually, whereas the core genome size of V. atypica tended to decrease progressively. Moreover, the pan-genome and the core-genome sizes of V. atypica were estimated to be 2,065 and 1,512 non-redundant genes within the nine V. atypica strains, respectively (Figure 1B). The distribution of the core, accessory, and specific genes revealed that the genomes of the V. atypica strains were remarkably diverse.
Phylogenetic Analysis of V. atypica Strains
To compare the similarity and the distance of the V. atypica strains and gain insights into the evolutionary relationship of these nine V. atypica strains, two strategies were applied to construct the phylogenetic trees and explore the phylogenetic relationships among the nine V. atypica strains. One strategy was based on the protein sequences of concatenated alignments of 1,468 single-copy core genes shared by the nine V. atypica strains (Figure 1C). This strategy can eliminate the effects of genome size and sequencing quality. The other strategy was based on the Manhattan distance between the nine V. atypica strains. The distance was calculated on the basis of the absence or the presence of each protein homolog (Figure 1D). Although the information from NCBI BioSample and the List of Prokaryotic names with Standing in Nomenclature (LPSN)1 revealed that V. atypica KON, V. atypica KON ATCC 17744, and V. atypica NCTC11830 are the same strain and the result of the phylogenetic tree of 16S rRNA showed that these three strains are in the same cluster (Supplementary Figure S1), the result in Figure 1D showed that V. atypica KON, V. atypica NCTC11830, and V. atypica KON ATCC 17744 are not in a same cluster. These results suggested that V. atypica KON, V. atypica KON ATCC 17744, and V. atypica NCTC11830 are different substrains of V. atypica KON. We speculated that the genetic variations of these three substrains contributed to their remarkable differences. For example, the number of 16S rRNA gene copies of these substrains ranged from 1 to 4. One 16S rRNA gene copy was presented in V. atypica KON, four 16S rRNA gene copies were presented in V. atypica KON ATCC 17744, and three 16S rRNA gene copies were presented in V. atypica NCTC11830. The results of the similarity analysis among the genomes of V. atypica KON, V. atypica NCTC11830, and V. atypica KON ATCC 17744 showed that the symmetric identity between V. atypica KON ATCC 17744 and V. atypica KON is 98.91%, while the symmetric identity between V. atypica KON ATCC 17744 and V. atypica NCTC11830 is 97.51%. In these two trees, V. atypica KHUD_V1 showed a closer evolutionary relationship with V. atypica AF36-15BH than with the other strains, although these two strains were isolated from different sources (oral cavity and feces). A previous study reported that V. atypica OK5 was isolated from a human saliva sample and the first transformable strain in Veillonella genus (Zhou et al., 2017). Another study sequenced the complete genome and successfully established a counter-selectable markerless mutagenesis system for the strain V. atypica OK5; these advances made the strain V. atypica OK5 an important and preferred strain in genetic studies of Veillonella genus (Zhou et al., 2015). However, the two different phylogenetic trees in the present study revealed that the evolutionary relationship of V. atypica OK5 was farther than previously thought from that of the remaining eight V. atypica strains (Figures 1C,D). This result suggested that V. atypica OK5 may not be a suitable representative strain of V. atypica. Hence, additional strains should be re-selected for sequencing and model construction to facilitate future studies on Veillonella genus. Additionally, although V. atypica NCTC11830, V. atypica KON, V. atypica KON ATCC 17744, V. atypica ACS-049-V-Sch6, V. atypica ACS-134-V-Col7a, and V. atypica CMW7756B showed closer phylogenetic relationships with one another, their evolutionary relationships remained intricate (Figures 1C,D). This observation suggested that these strains were dissimilar. Moreover, these strains contained more accessory families than V. atypica KHUD_V1, V. atypica AF36-15BH, and V. atypica OK5, with the exception of V. atypica ACS-049-V-Sch6. Furthermore, the numbers of accessory families of these six strains were diverse. Hence, we speculated that the gain and the loss of different genes from different strains contributed to the large differences in the genetic compositions of V. atypica strains and led to evolutionary divergence among V. atypica strains.
Identification of CAZymes for V. atypica Strains
Previous studies have reported that the members of Veillonella are generally unable to ferment carbohydrates but rather grow well on media containing lactate, pyruvate, malate, or fumarate under anaerobic conditions (Kolenbrander, 2006). The members of Veillonella have been suggested to exhibit unusual metabolism of carbohydrates, but this type of metabolism has not been clarified in the V. atypica strains. Hence, in the present study, 2,065 homologous clusters of V. atypica were systemically identified against the CAZy database to obtain a comprehensive understanding of the catalytic ability of carbohydrate of V. atypica (Yin et al., 2012). A total of 43 orthologous genes were identified and grouped into 23 CAZyme families (Figure 2A). Among these 43 orthologous genes, 29 and 14 orthologous genes belonged to accessory clusters and core clusters and categorized into 11 and 18 CAZyme families (Figures 2B,C), respectively. The number in Figure 2 represents the number of genes classified into each CAZyme family for each V. atypica strain and shows the distribution of CAZyme families in the nine V. atypica strains. It was also noted that the 14 orthologous genes belonging to the core clusters all belonged to the single-copy gene clusters and the distribution of CAZyme derived from the core clusters are same in the V. atypica strains (Figure 2B). We observed that the CAZymes derived from the core clusters were mainly divided into glycosyltransferase (GT), glycoside hydrolase (GH), and carbohydrate esterase (CE). Previous studies have reported that the primary function of GTs is to catalyze glycoside synthesis, the substrates of which are various sugar-1-phosphate derivatives (Lairson et al., 2008). During the process of reaction, GT utilizes activated sugar donors and catalyzes glycosyl group transfer to appropriate acceptors (Martinez-Fleites et al., 2006). In the present study, eight kinds of GTs derived from the core clusters were found in the nine V. atypica strains, namely, GT19, GT2, GT30, GT4, GT45, GT51, GT83, and GT9 (Figure 2B). Among these GT families, GT2 and GT4 families are comprised of chitin synthase, cellulose synthase, α-glucosyltransferase, etc. (Bohra et al., 2019), and GT4 is one of the largest families and contains several enzymes that contribute to lipopolysaccharide synthesis and antibiotic avilamycin A synthesis (Martinez-Fleites et al., 2006). GT9 and GT19 are heptosyltransferase (lipopolysaccharide N-acetylglucosaminyltransferase) and lipid-A-disaccharide synthase, respectively, and they contribute to the synthesis of lipopolysaccharide (Qi et al., 2019). In the present study, the numbers of GT2, GT4, GT9, and GT19 were pre-dominant in the nine V. atypica strains. Owing to the presence of CAZyme families, V. atypica has an unusual preference for organic acid carbon sources and plays a crucial role in the removal of toxic metabolites from biofilm communities (Delwiche et al., 1985; Knapp et al., 2017).
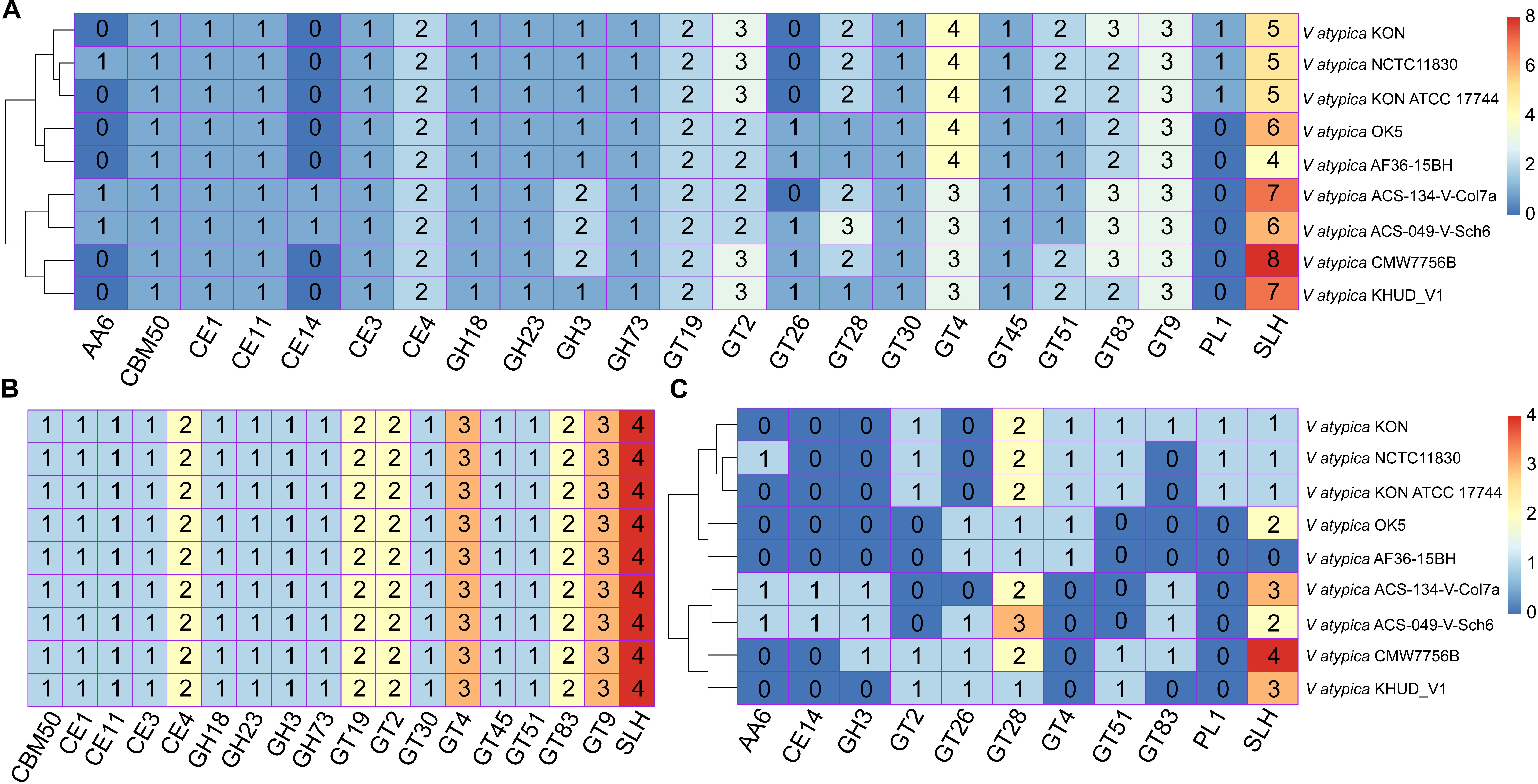
Figure 2. Distribution of CAZymes in the nine Veillonella atypica strains. (A) Distribution of CAZymes of pan-genome in each V. atypica strain. (B) Distribution of CAZymes of pan-genome in each V. atypica strain grouped by core clusters. (C) Distribution of CAZymes of pan-genome in each V. atypica strain grouped by accessory clusters. The numbers shown in subgraphs represent the orthologous genes assigned by CAZymes. GT, glycosyltransferase; GH, glycoside hydrolase; CE, carbohydrate esterase; CBM, carbohydrate-binding molecule; AA, auxiliary activities; PL, polysaccharide lyases; SLH, S-layer homology.
Additionally, the differences in CAZymes among the nine V. atypica strains were attributed to the CAZyme components derived from the accessory clusters (Figure 2C). For example, V. atypica NCTC11830, V. atypica ACS-134-V-Col7a, and V. atypica ACS-049-V-Sch6 were found to harbor one orthologous gene belonging to AA6, which is 1,4-benzoquinone reductase and involved in the biodegradation of aromatic compounds (Luo et al., 2018). V. atypica ACS-134-V-Col7a and V. atypica ACS-049-V-Sch6 harbored one orthologous gene belonging to CE14, whereas V. atypica KON, V. atypica ACS-134-V-Col7a, V. atypica ACS-049-V-Sch6, and V. atypica CMW7756B harbored one orthologous gene belonging to GT83 (Figure 2C). The distributions of CAZymes in the nine V. atypica strains were different, suggesting the remarkable differences in the ability of each V. atypica strain in carbohydrate metabolism.
Functional Traits of V. atypica Strains
The pan-genome of V. atypica was profiled against COG to gain insight into the functional composition of V. atypica and characterize its functional traits for the accessory, core, and specific clusters (Figure 3A). The orthologous clusters of the pan-genome were mainly dominant in “general function” (R, 218, 12.54%), “amino acid transport and metabolism” (E, 186, 10.7%), and “translation, ribosomal structure, and biogenesis” (J, 145, 8.34%). The functions of the accessory clusters were mainly enriched in “replication, recombination, and repair” (L, 50, 13.93%), “general function” (R, 49, 13.65%), “inorganic ion transport and metabolism” (P, 28, 7.8%), “amino acid transport and metabolism” (E, 23, 6.41%), “translation, ribosomal structure, and biogenesis” (J, 24, 6.69%), and “coenzyme transport and metabolism” (H, 22, 6.13%). The specific genes of the nine V. atypica strains are the primary contributors to the functions involved in “cell wall/membrane/envelope biogenesis,” “general function,” and “transcription.”
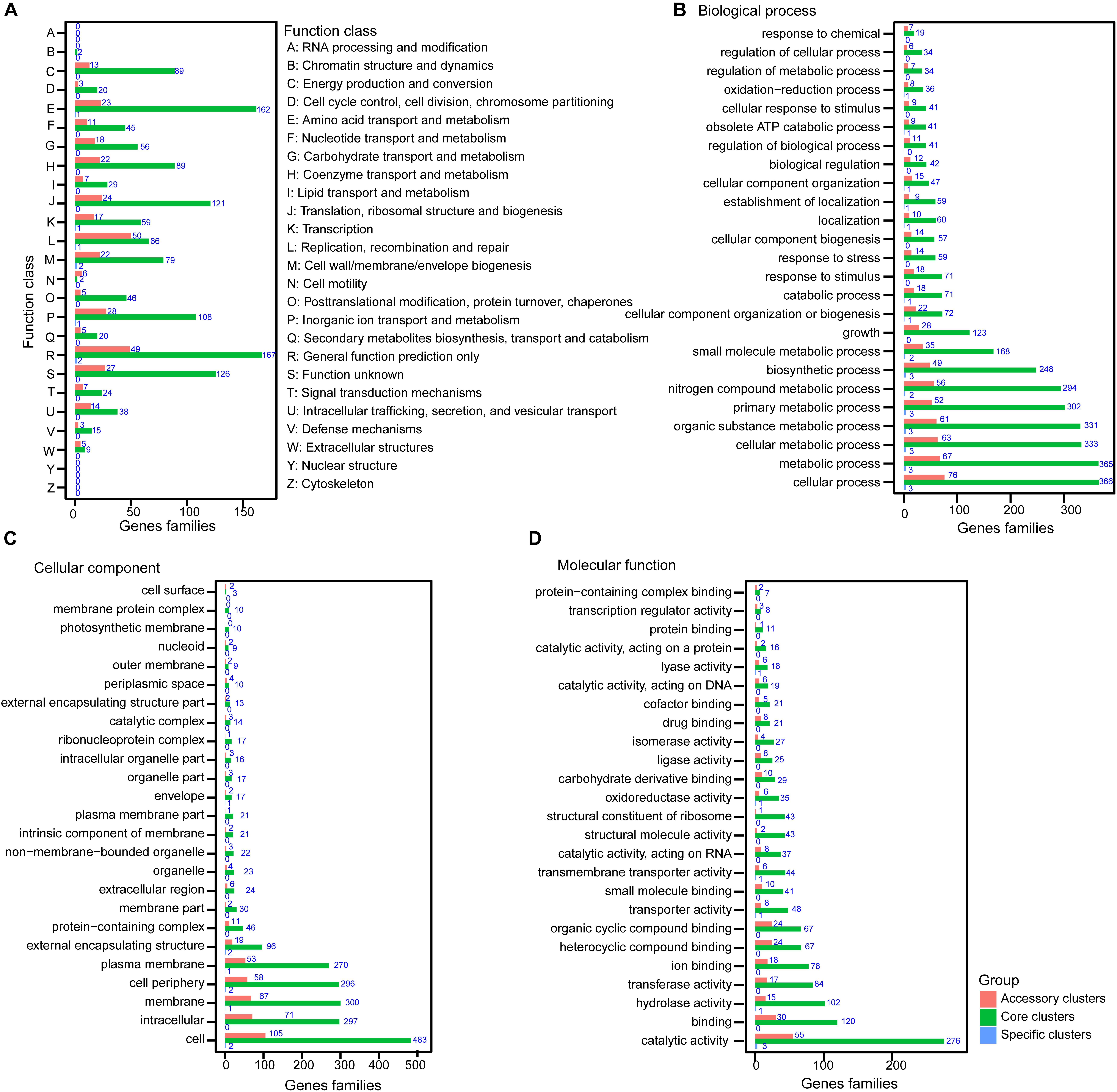
Figure 3. Cluster of Orthologous Group (COG) and Gene Ontology (GO) annotations of gene families for the pan-genome of Veillonella atypica. (A) Distribution of 25 functional categories according to COG annotation for V. atypica. Distribution of GO annotation of pan-genome in (B) biological process, (C) cellular component, and (D) molecular function for V. atypica. The pink, green, and wathet blue bars of each subgraph represent the accessory, core, and specific clusters, respectively.
In addition, GO analysis was performed to characterize the genetic functions of V. atypica and obtain a deep understanding of the function catalogs of this species. Based on the genetic functions of the orthologous clusters, these genes were categorized into biological process, cellular component, and molecular function (Figures 3B,D). An enrichment analysis of biological processes revealed that high proportions of the core and the accessory genes contributed to the functions involved in “cellular process” (442, 58.01%), “metabolic process” (432, 56.69%), “cellular metabolic process” (396, 51.97%), “organic substance metabolic process” (392, 51.44%), “primary metabolic process” (354, 46.46%), “nitrogen compound metabolic process” (350, 45.93%), “biosynthetic process” (297, 38.98%), and “small molecule metabolic process” (203, 26.64%) (Figure 3B). The majority of the genes assigned to cellular components was grouped into “cell” (600, 78.74%), “intracellular” (368, 48.29%), “membrane” (368, 48.29%), “cell periphery” (356, 46.72%), and “plasma membrane” (324, 42.52%) (Figure 3C). An enrichment analysis of molecular function showed that many genes were grouped into the functions involved in “catalytic activity” (334, 43.83%), “binding” (150, 19.69%), “hydrolase activity” (118, 15.49%), and “transferase activity” (101, 13.25%) (Figure 3D).
Unlike the previous studies that focused on the pan-genome of Aeromonas and Shewanella, the present study found that the distribution of the functional categories of V. atypica was different from that of Aeromonas and Shewanella. The pan-genome analysis of the Aeromonas strains showed that several COG categories had a higher percentage in the accessory clusters than in the other clusters but were less represented in the core clusters, such as “energy production and conversion,” “amino acid transport and metabolism,” “carbohydrate transport and metabolism,” and “transcription” (Zhong et al., 2019). By comparison, the pan-genome analysis of 24 Shewanella strains showed that the functional traits affiliated with “plasma membrane” had a higher percentage in the specific clusters than the other functional traits but were less represented in the core and the accessory clusters (Zhong et al., 2018). In the present study, the functional traits of the V. atypica strains had a higher percentage in the core clusters than in the accessory and the specific clusters (Figures 3B,D), suggesting that the primary genetic functions of the V. atypica strains depended on its core genome of V. atypica, while the differences between the accessory and the specific clusters contributed to the other metabolism functions of V. atypica.
Metabolic Pathways Annotated by KEGG Server for V. atypica Strains
To obtain a deeper understanding of the metabolic pathways of the V. atypica strains, we annotated the proteins of the nine V. atypica strains against KEGG database. The outputs of the KEGG Automatic Annotation Server (KAAS) server for the nine V. atypica strains are summarized in Supplementary Table S1. The metabolic pathways detected in the nine V. atypica strains were mainly involved in primary metabolic synthesis and secondary metabolic synthesis.
Firstly, a large proportion of the proteins was involved in the metabolic pathways of ribosome (4.57–4.8%), ABC transporters (3.78–4.37%), porphyrin and chlorophyll metabolism (3–3.3%), purine metabolism (2.87–2.97%), pyrimidine metabolism (2.42–2.56%), pyruvate metabolism (2.37–2.53%), cysteine and methionine metabolism (2.28–2.41%), two-component system (2.12–2.56%), aminoacyl-tRNA biosynthesis (2.24–2.32%), quorum sensing (2.17–2.4%), carbon fixation pathways in prokaryotes (1.85–2.08%), phenylalanine, tyrosine, and tryptophan biosynthesis (1.7–1.8%), and lipopolysaccharide biosynthesis (1.71–1.79%) (Supplementary Table S1). Notably, the number of genes detected in these pathways varied in the nine V. atypica strains, and several proteins were annotated to the KEGG pathways of antibiotic resistance, including vancomycin resistance and platinum drug resistance (Supplementary Table S1). Vancomycin resistance in genus Veillonella has already been reported (Al-Otaibi and Al-Mohizea, 2014; Carlier, 2015), but our new finding indicated that the functional information of the nine V. atypica strains was involved in the metabolic pathway of vancomycin, which is summarized in Supplementary Tables S2, S3. Our results reinforced the results of previous studies associated with vancomycin in V. atypica and provided valuable information from the perspective of bioinformatics and pan-genome, which is helpful for researchers who are interested in the V. atypica strains to conduct more in-depth research.
Secondly, based on the annotations of the proteins for the nine V. atypica strains, we constructed the pathway maps and the modules for the nine V. atypica strains. These pathway maps and modules are summarized in Supplementary Tables S2, S3. Among the integrated metabolic pathways, a large proportion of the proteins was involved in metabolic pathways, ranging from 19.08 to 19.41%, for the nine V. atypica strains (Supplementary Table S2). The top pathways annotated with maximum KEGG orthology hits were biosynthesis of secondary metabolites (8.17–8.35%), biosynthesis of antibiotics (5.62–5.85%), microbial metabolism in diverse environments (4.36–4.5%), and biosynthesis of amino acids (4.12–4.27%) (Supplementary Table S2) in the nine V. atypica strains. Combined with the information provided in Supplementary Table S1, we found that the KEGG pathways were involved in the biosynthesis of antibiotics, including novobiocin biosynthesis, streptomycin biosynthesis, carbapenem biosynthesis, prodigiosin biosynthesis, acarbose and validamycin biosynthesis, penicillin and cephalosporin biosynthesis, biosynthesis of ansamycins, and biosynthesis of vancomycin group antibiotics. Additionally, 138 KEGG modules were identified in the nine V. atypica strains (Supplementary Table S3). The distributions of the number of genes identified in these pathways and modules were almost the same in the nine V. atypica strains.
Overall, an investigation of the metabolic pathways of the nine V. atypica strains will expand our knowledge of these processes and also aid in the transformation of the metabolic pathways of several substrates. Besides that, it should be noted that the prediction of functional traits and metabolic pathways of the V. atypica strains in this present study depended on their genomes, whereas in the actual natural situation, microorganisms exist in the form of a microbial community. Following previous studies (Zhang et al., 2018; Zhong et al., 2019), to further profile and confirm the functional composition of the V. atypica strain, it is necessary to conduct a deeper analysis based on the metagenomic data and/or (meta-)transcriptomic data of the microbial communities containing the V. atypica strains. Moreover, the functions, especially the metal reducing mechanism, of the V. atypica strains need to be verified via wet experiments in further studies.
CRISPR–Cas Systems for V. atypica Strains
To efficiently edit the genetic elements of the metabolic pathways of V. atypica, we identified the CRISPR array and the Cas proteins of CRISPR–Cas systems for the nine V. atypica strains. Firstly, the distribution patterns of the number of CRISPR arrays were different among the nine V. atypica strains (Table 2). V. atypica ACS-049-V-Sch6 harbored the lowest number of CRISRP arrays (two), whereas V. atypica ACS-134-V-Col7a harbored the highest number of CRISPR arrays (12) (Table 2). The CRISRP arrays are DNA segments captured by prokaryotic bacteria from invading viruses and suited as virus recognition keys. The CRISPR arrays consist of a number of spacers which, in a CRISPR array of a prokaryotic bacteria, are maximized for protection against viral attacks (Martynov et al., 2017). Owing to the repetitive nature of the repeating sequences, the CRISPR arrays have been proven difficult to generate. Variations in the number of CRISPR arrays among the nine V. atypica strains suggested that the ability of these nine V. atypica strains to accept foreign DNA was different. Secondly, the Cas proteins were detected in V. atypica ACS-049-V-Sch6 (two Cas proteins), V. atypica ACS-134-V-Col7a (four Cas proteins), V. atypica KON (three Cas proteins), V. atypica OK5 (one Cas protein), V. atypica KON ATCC 17744 (three Cas proteins), V. atypica KHUD V1 (three Cas proteins), and V. atypica NCTC11830 (one Cas protein) (Table 2). By contrast, the Cas proteins were not identified in V. atypica CMW7756B and V. atypica AF36-15BH, probably because the genomes of these two strains were not complete. The Cas proteins are known to use the genetic information stored in the CRISPR arrays to initiate a host immune response against mobile genetic elements that carry a homologous sequence to achieve gene editing (Westra et al., 2016). The variation in the number of cas genes among these nine V. atypica strains likely resulted from rapid evolution and extensive horizontal gene transfer (Westra et al., 2016), which revealed the variation of the CRISPR–Cas system among the V. atypica strains and suggested that it is important to obtain a deeper understanding of the genetic background of the V. atypica strains to achieve gene editing effectively. Thirdly, we observed that these seven V. atypica strains almost harbored CAS-Type IIIA, and we detected CAS-Type IIA, CAS-Type IIC, and CAS-Type IIID in the V. atypica strains (Table 2). Taken together, these results suggested that various and diverse Cas proteins can be selected to edit the genetic elements of the V. atypica strains.
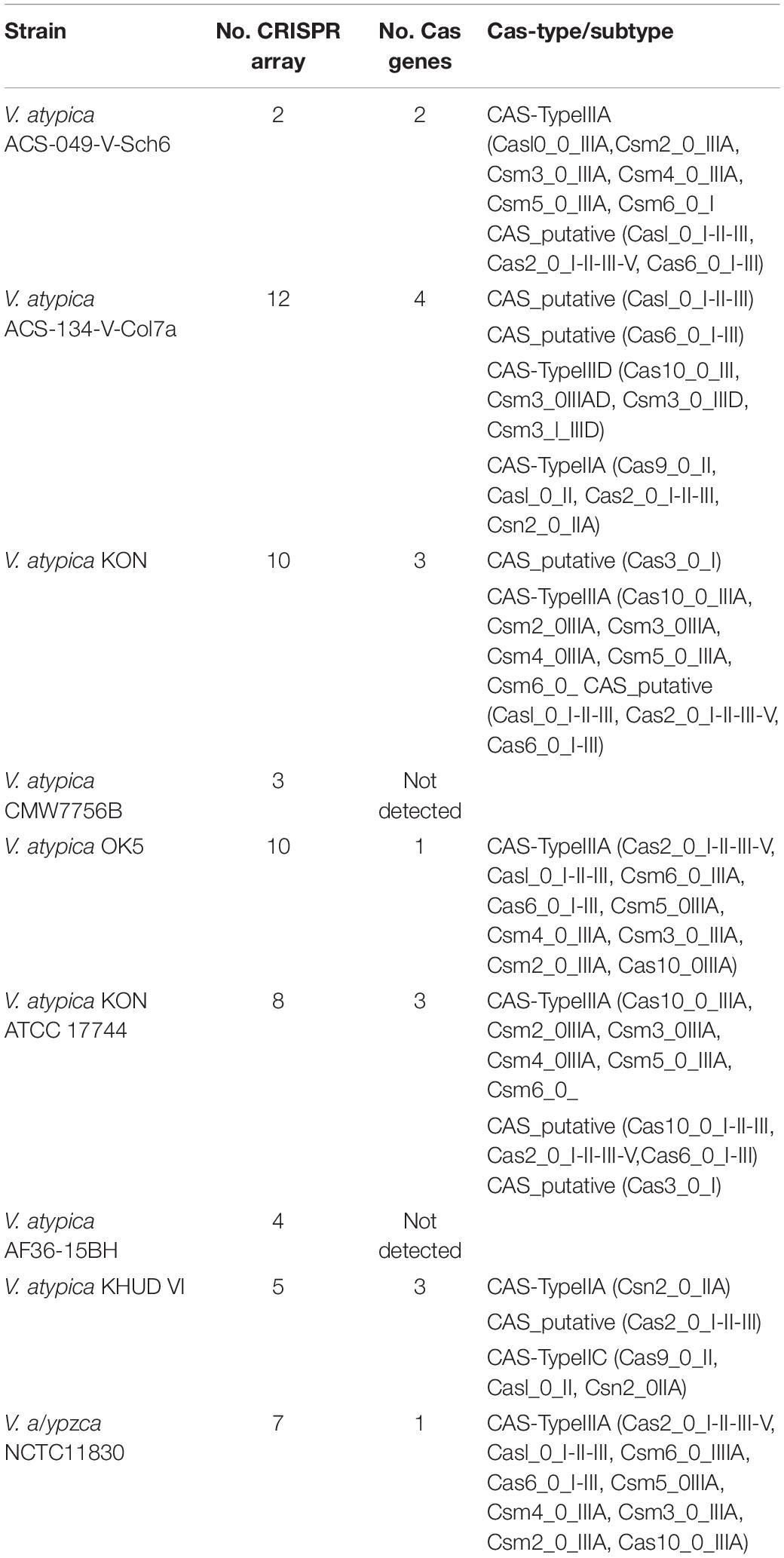
Table 2. CRISPR arrays and Cas proteins detected in the CRISPR–Cas systems of the nine Veillonella atypica strains.
Conclusion
In conclusion, we performed a comparative analysis on the pan-genome of species V. atypica on the basis of complete and draft genomes of the V. atypica strains in this study. The results demonstrated that V. atypica exhibited an open pan-genome structure. A phylogenetic analysis revealed that V. atypica OK5 may not be the suitable representative strain for V. atypica. Moreover, the results suggested that another strain or additional strains are needed to be selected for sequencing and model construction for Veillonella genus in future studies. The functional annotations of the nine V. atypica strains were profiled against the CAZyme, COG, and GO databases. Our results demonstrated that the differences in CAZymes among the nine V. atypica strains were attributed to the CAZyme component derived from the accessory clusters. Additionally, the core clusters were more highly represented in the COG and the GO annotations than the accessory clusters and the specific clusters, suggesting that the primary genetic functions of the V. atypica strains depend on the core genome of V. atypica. By contrast, the differences in the accessory clusters and the specific clusters contributed to the other metabolism functions of V. atypica. Moreover, several proteins were annotated to the KEGG pathways involved in antibiotic resistance and biosynthesis of secondary metabolites, demonstrating that the V. atypica strains harbored vancomycin resistance. Besides that, four main Cas proteins of the CRISPR–Cas systems for the V. atypica strains, namely, CAS-Type IIIA, CAS-Type IIA, CAS-Type IIC, and CAS-Type IIID, were harbored in the V. atypica strains. These various Cas proteins can be used to edit the genetic elements of the V. atypica strains. The genetic diversity and the functional traits of V. atypica strains were together uncovered using their genomic information in this present study. The present study found considerable differences among the V. atypica strains. Hence, the results suggested that we should have a deep understanding of the genetic background of the V. atypica strains before we realize their biological potential, including their probiotic potential for humans, clarification of the metabolic pathways associated with secondary metabolic synthesis, and potential application as selenium-respiring bacterium with an important role in the selenium cycle. Nevertheless, our pilot comparative study was on the basis of one complete genome (V. atypica OK5) and eight draft genomes (the others) of the V. atypica strains, and the limitations of the genetic datasets might have possibly affected the revelation of the real comparative analysis results of V. atypica, which suggests and requires researchers to isolate more V. atypica strains from more ecological niches, especially the V. atypica strains isolated from the gut, in future studies. Additional isolated V. atypica strains and their corresponding complete genomes should be used in the comparative analysis of V. atypica, and more attention should be paid to investigate the pan-genome analysis of V. atypica to optimize the potential applications of this bacterium in future studies. It is important to note that our study is a pilot study, and we focused on the genetic diversity and the functional characteristics of the V. atypica strains. This study provides an in-depth understanding of the genetic diversity, functional composition, and metabolic pathways of the V. atypica strains and profiles their CRISPR–Cas systems on the basis of the genomic information of the V. atypica strains. The results provide insights into the biosynthetic engineering of V. atypica.
Materials and Methods
Collection of Genomic Datasets of V. atypica
To date, nine strains of V. atypica have been sequenced, and their genomic datasets have been uploaded to the genome database of NCBI. The nine strains of V. atypica are V. atypica KON, V. atypica CMW7756B, V. atypica ACS-049-V-Sch6, V. atypica ACS-134-V-Col7a, V. atypica KON ATCC 17744, V. atypica NCTC11830, V. atypica AF36-15BH, V. atypica KHUD_V1, and V. atypica OK5. The genome and the protein sequences of these nine V. atypica strains were downloaded from the GenBank database of NCBI for comparative genomics analysis (Table 1). Among these nine V. atypica strains, according to the information from NCBI BioSample and LPSN, V. atypica KON, V. atypica KON ATCC 17744, and V. atypica NCTC11830 are defined as the same type of strain of V. atypica.
Identification of the Protein Orthologs of V. atypica
To obtain a better understanding of genetics knowledge on V. atypica and the evolutionary relationship of the V. atypica strains, a systematic comparative genomic analysis of the V. atypica strains was conducted. The protein orthologs of V. atypica were identified using OrthMCL (version: 2.0.9) (Li et al., 2003), with an e-value < 1e-5 and an inflation parameter of 1.5 (Zhong et al., 2018). The protein homologous clusters were clustered into core, accessory, and specific groups. The core group represented the genes or the proteins shared by all nine V. atypica strains. The accessory group comprised the genes or the proteins shared by at least two strains but not by all nine V. atypica strains. The remaining genes or proteins occurring only in one V. atypica strain were cataloged into the specific group (strain-specific genes or proteins). In this present study, the clustering result of the proteins yielded 2,065 homologous clusters, 1,468 of which were identified as single-copy protein families.
Construction of the Phylogenetic Tree of V. atypica
To obtain the evolutionary relationship of the nine V. atypica strains, three phylogenetic trees for these nine strains were constructed. The 16S rRNA sequences were extracted from the genomic sequences by using Barrnap,2 and then a phylogenetic tree on the basis of the 16S rRNA sequences was built with MEGA software (version: 7.0.26) (Tamura et al., 2011). The other phylogenetic trees were built with different strategies. Specifically, the protein sequences of these single-copy protein families were concatenated and aligned using MUSCLE (version 3.8.31) (Edgar, 2004), with default settings. The results of multiple protein sequence alignment were eliminated using Gblocks (version 0.91b) (Talavera and Castresana, 2007) to remove the regions that were divergent, misaligned, or with a larger number of gaps. Then, the phylogenetic tree was constructed in Phylip (version 3.696) (Jd, 1999) by using the maximum likelihood algorithm with 100 bootstrap iterations. Additionally, a pan-genome tree was constructed based on the absence or the presence of each homologous protein using the Manhattan distance to evaluate the evolutionary relationships among the nine V. atypica strains (Zhong et al., 2018, 2019). The phylogenetic trees were generated and visualized using MEGA software (version 7.0.26).
Functional Annotations
To gain more insights into the functional characteristics of V. atypica, a total of 2,065 homologous proteins were assigned against the CAZyme, COG function, and eggNOG annotation databases. Specifically, we annotated the protein sequences of 2,065 homologous clusters against CAZy database, which was downloaded from dbCAN (Lombard et al., 2014),3 to explore the functional composition of V. atypica related to carbohydrate metabolism. According to the manual of dbCAN CAZyme annotation, the protein sequences were annotated by running hmmscan of HMMER (version: 3.1b1) (Cantarel et al., 2008; Mäkelä et al., 2018), with default settings, and the annotated results were summarized into GT, GH, carbohydrate-binding module, auxiliary activity, polysaccharide lyase, S-layer homology, and CE. The protein sequences of 2,065 homologous clusters were annotated to COG proteins (Tatusov et al., 2003), which were downloaded from NCBI,4 by using local BLASTP with a cutoff E-value of 1e-4. The top one of the annotation results was selected as the best annotation for each homologous cluster, and then it was assigned to 25 functional categories. The protein sequences of 2,065 homologous clusters were aligned to the eggNOG database by using the eggnog-mapper (Huerta-Cepas et al., 2015). GO annotations were summarized for the core, accessory, and specific groups based on the GO annotations of 2,065 homologous clusters, respectively. The results of the GO annotations of the core, accessory, and specific groups were divided into three groups, including biological process, molecular function, and cellular component, and then visualized using Web Gene Ontology Annotation Plotting (version 2.0) (Ye et al., 2018).
KEGG Pathway Analyses
To obtain a comprehension of functional and metabolic interactions in the nine V. atypica strains, their proteins were submitted to the KAAS5 to identify the metabolic pathways (Moriya et al., 2007). When using the server, BLAST was chosen as the search program, and bi-directional best hit was selected as the preferred assignment method. Additionally, the references of default organisms for prokaryotes were chosen to obtain KEGG annotations.
Prediction of CRISPR–Cas Systems for V. atypica Strains
In general, the CRISPR–Cas systems are used for editing the genomes of diverse organisms. To obtain a better understanding of the CRISPR–Cas systems of the V. atypica strains, we conducted a comprehensive characterization of the CRISPR–Cas systems in the nine V. atypica strains. In this present study, the online tool CrisprCasFinder6 (Martinez-Fleites et al., 2006) was applied to detect the components of the CRISPR–Cas system, including the CRISPR arrays and the cas genes of the nine V. atypica strains. In using CrisprCasFinder, 100 bp was selected as the size threshold of the flanking regions for CRISPR array identification, and the CRISPR array contained truncated repeats.
Data Availability Statement
The raw data supporting the conclusions of this article will be made available by the authors, without undue reservation, to any qualified researcher.
Author Contributions
MH and YZ designed the study. MH and DW collected and downloaded the data. MH, GL, and YZ analyzed the data. MH, GL, YC, and YZ wrote the initial draft of the manuscript. All the authors revised the manuscript.
Funding
This work was partially supported by Grants for Scientific Research of BSKY (No: XJ201916) from Anhui Medical University, Natural Science Foundation of the Anhui Higher Education Institutions of China (Grant No. KJ2018A0494), Youth Elite Support Plan in Universities of Anhui Province (Grant No. gxyq201805), and National Science Foundation of China (Grant No. 31702030).
Conflict of Interest
The authors declare that the research was conducted in the absence of any commercial or financial relationships that could be construed as a potential conflict of interest.
Supplementary Material
The Supplementary Material for this article can be found online at: https://www.frontiersin.org/articles/10.3389/fmicb.2020.01219/full#supplementary-material
Footnotes
- ^ https://www.bacterio.net/
- ^ https://github.com/tseemann/barrnap
- ^ http://csbl.bmb.uga.edu/dbCAN/
- ^ https://www.ncbi.nlm.nih.gov/COG/
- ^ http://www.genome.jp/tools/kaas/
- ^ https://crisprcas.i2bc.paris-saclay.fr/CrisprCasFinder/Index
References
Alkhnbashi, O. S., Meier, T., Mitrofanov, A., Backofen, R., and Voss, B. (2019). CRISPR-Cas bioinformatics. Methods 172, 3–11. doi: 10.1016/j.ymeth.2019.07.013
Al-Otaibi, F. E., and Al-Mohizea, M. M. (2014). Non-vertebral Veillonella species septicemia and osteomyelitis in a patient with diabetes: a case report and review of the literature. J. Case Rep. Med. 8:365.
Aujoulat, F., Bouvet, P., Jumas-Bilak, E., Jean-Pierre, H., and Marchandin, H. (2014). Veillonella seminalis sp. nov., a novel anaerobic Gram-stain-negative coccus from human clinical samples, and emended description of the genus Veillonella. Intern. J. Syst. Evol. Microbiol. 64, 3526–3531. doi: 10.1099/ijs.0.064451-0
Beighton, D., Clark, D., Hanakuka, B., Gilbert, S., and Do, T. (2008). The predominant cultivable Veillonella spp. of the tongue of healthy adults identified using rpoB sequencing. Oral Microbiol. Immunol. 23, 344–347. doi: 10.1111/j.1399-302x.2007.00424.x
Bohra, V., Dafale, N. A., and Purohit, H. J. (2019). Understanding the alteration in rumen microbiome and CAZymes profile with diet and host through comparative metagenomic approach. Archiv. Microbiol. 201, 1385–1397. doi: 10.1007/s00203-019-01706-z
Bravo, C., and Martinez, V. (2016). Whole-genome comparative analysis of the pathogen Piscirickettsia salmonis. Vet. Microbiol. 196, 36–43. doi: 10.1016/j.vetmic.2016.10.015
Cantarel, B. L., Coutinho, P. M., Rancurel, C., Bernard, T., Lombard, V., and Henrissat, B. (2008). The carbohydrate-active EnZymes database (CAZy): an expert resource for glycogenomics. Nucleic Acids Res. 37(Suppl._1), D233–D238.
Carlier, J. P. (2015). Veillonella. Bergey’s Manual Of Systematics Of Archaea And Bacteria. Hoboken, NJ: Wiley Online Library.
Crisafulli, E., Bernardinello, N., Alfieri, V., Pellegrino, F., Lazzari, C., Gnetti, L., et al. (2019). A pulmonary infection by Actinomyces odontolyticus and Veillonella atypica in an immunocompetent patient with dental caries. Respirol. Case Rep. 7:e00493.
Delwiche, E., Pestka, J., and Tortorello, M. (1985). The Veillonellae: gram-negative cocci with a unique physiology. Ann. Rev. Microbiol. 39, 175–193.
Edgar, R. C. (2004). MUSCLE: multiple sequence alignment with high accuracy and high throughput. Nucleic Acids Res. 32, 1792–1797. doi: 10.1093/nar/gkh340
Horvath, P., and Barrangou, R. (2010). CRISPR/Cas, the immune system of bacteria and archaea. Science 327, 167–170. doi: 10.1126/science.1179555
Huerta-Cepas, J., Szklarczyk, D., Forslund, K., Cook, H., Heller, D., Walter, M. C., et al. (2015). eggNOG 4.5: a hierarchical orthology framework with improved functional annotations for eukaryotic, prokaryotic and viral sequences. Nucleic Acids Res. 44, D286–D293.
Hughes, C. V., Kolenbrander, P. E., Andersen, R. N., and Moore, L. V. (1988). Coaggregation properties of human oral Veillonella spp.: relationship to colonization site and oral ecology. Appl. Environ. Microbiol. 54, 1957–1963. doi: 10.1128/aem.54.8.1957-1963.1988
Jd, R. (1999). Phylogenetic analysis using PHYLIP. Methods Mol. Biol. 132, 243–258. doi: 10.1385/1-59259-192-2:243
Knapp, S., Brodal, C., Peterson, J., Qi, F., Kreth, J., and Merritt, J. (2017). Natural competence is common among clinical isolates of Veillonella parvula and is useful for genetic manipulation of this key member of the oral microbiome. Front. Cell. Infect. Microbiol. 7:139. doi: 10.3389/fcimb.2017.00139
Kolenbrander, P. (2006). “The genus Veillonella,” in The Prokaryotes: Volume 4: Bacteria: Firmicutes, Cyanobacteria, eds M. Dworkin, S. Falkow, E. Rosenberg, K.-H. Schleifer, and E. Stackebrandt (New York, NY: Springer), 1022–1040. doi: 10.1007/0-387-30744-3_36
Lairson, L. L., Henrissat, B., Davies, G. J., and Withers, S. G. (2008). Glycosyltransferases: structures, functions, and mechanisms. Ann. Rev. Biochem. 77, 521–555. doi: 10.1146/annurev.biochem.76.061005.092322
Li, L., Stoeckert, C. J. Jr., and Roos, D. S. (2003). OrthoMCL: identification of ortholog groups for eukaryotic genomes. Genome Res. 13, 2178–2189. doi: 10.1101/gr.1224503
Livingstone, P. G., Morphew, R. M., and Whitworth, D. E. (2018). Genome sequencing and pan-genome analysis of 23 Corallococcus spp. strains reveal unexpected diversity, with particular plasticity of predatory gene sets. Front. Microbiol. 9:3187. doi: 10.3389/fcimb.2017.03187
Lloyd, J. R. (2003). Microbial reduction of metals and radionuclides. FEMS Microbiol. Rev. 27, 411–425. doi: 10.1016/s0168-6445(03)00044-5
Lombard, V., Golaconda Ramulu, H., Drula, E., Coutinho, P. M., and Henrissat, B. (2014). The carbohydrate-active enzymes database (CAZy). Nucleic Acids Res. 42, D490–D495.
Luo, C., Li, Y., Liao, H., and Yang, Y. (2018). De novo transcriptome assembly of the bamboo snout beetle Cyrtotrachelus buqueti reveals ability to degrade lignocellulose of bamboo feedstock. Biotechnol. Biofuels 11:292.
Mäkelä, M., DiFalco, M., McDonnell, E., Nguyen, T. T. M., Wiebenga, A., Hildén, K., et al. (2018). Genomic and exoproteomic diversity in plant biomass degradation approaches among Aspergilli. Stud. Mycol. 91, 79–99. doi: 10.1016/j.simyco.2018.09.001
Martinez-Fleites, C., Proctor, M., Roberts, S., Bolam, D. N., Gilbert, H. J., and Davies, G. J. (2006). Insights into the synthesis of lipopolysaccharide and antibiotics through the structures of two retaining glycosyltransferases from family GT4. Chem. Biol. 13, 1143–1152. doi: 10.1016/j.chembiol.2006.09.005
Martynov, A., Severinov, K., and Ispolatov, I. (2017). Optimal number of spacers in CRISPR arrays. PLoS Comput. Biol. 13:e1005891. doi: 10.1371/journal.pcbi.1005891
Mashima, I., and Nakazawa, F. (2015). The interaction between Streptococcus spp. and Veillonella tobetsuensis in the early stages of oral biofilm formation. J. Bacteriol. 197, 2104–2111. doi: 10.1128/jb.02512-14
Moriya, Y., Itoh, M., Okuda, S., Yoshizawa, A. C., and Kanehisa, M. (2007). KAAS: an automatic genome annotation and pathway reconstruction server. Nucleic Acids Res. 35, W182–W185.
Nancharaiah, Y. V., and Lens, P. N. L. (2015). Ecology and biotechnology of selenium-respiring bacteria. Microbiol. Mol. Biol. Rev. 79, 61–80. doi: 10.1128/mmbr.00037-14
Pearce, C. I., Pattrick, R. A. D., Law, N., Charnock, J. M., Coker, V. S., Fellowes, J. W., et al. (2009). Investigating different mechanisms for biogenic selenite transformations: Geobacter sulfurreducens, Shewanella oneidensis and Veillonella atypica. Environ. Technol. 30, 1313–1326.
Qi, Z., Zhu, Y., Guo, H., Chen, Y., Zhao, Y., Zhou, Y., et al. (2019). Production of glycoprotein bioflocculant from untreated rice straw by a CAZyme-rich bacterium, Pseudomonas sp. HP2. J. Biotechnol. 306, 185–192. doi: 10.1016/j.jbiotec.2019.10.011
Scheiman, J., Luber, J. M., Chavkin, T. A., MacDonald, T., Tung, A., Pham, L.-D., et al. (2019). Meta-omics analysis of elite athletes identifies a performance-enhancing microbe that functions via lactate metabolism. Nat. Med. 25, 1104–1109. doi: 10.1038/s41591-019-0485-4
Talavera, G., and Castresana, J. (2007). Improvement of phylogenies after removing divergent and ambiguously aligned blocks from protein sequence alignments. Syst. Biol. 56, 564–577. doi: 10.1080/10635150701472164
Tamura, K., Peterson, D., Peterson, N., Stecher, G., Nei, M., and Kumar, S. (2011). MEGA5: molecular evolutionary genetics analysis using maximum likelihood, evolutionary distance, and maximum parsimony methods. Mol. Biol. Evol. 28, 2731–2739. doi: 10.1093/molbev/msr121
Tatusov, R. L., Fedorova, N. D., Jackson, J. D., Jacobs, A. R., Kiryutin, B., Koonin, E. V., et al. (2003). The COG database: an updated version includes eukaryotes. BMC Bioinform. 4:41. doi: 10.1186/1471-2105-4-41
Veras, A., Araujo, F., Pinheiro, K., Guimarães, L., Azevedo, V., Soares, S., et al. (2018). Pan4Draft: a computational tool to improve the accuracy of pan-genomic analysis using draft genomes. Sci. Rep. 8:9670.
Vesth, T., Ozen, A., Andersen, S. C., Kaas, R. S., Lukjancenko, O., Bohlin, J., et al. (2013). Veillonella, firmicutes: microbes disguised as Gram negatives. Stand. Genom. Sci. 9, 431–448. doi: 10.4056/sigs.2981345
Wang, S., Zhang, B., Li, T., Li, Z., and Fu, J. (2020). Soil vanadium(V)-reducing related bacteria drive community response to vanadium pollution from a smelting plant over multiple gradients. Environ. Intern. 138:105630. doi: 10.1016/j.envint.2020.105630
Westra, E. R., Dowling, A. J., Broniewski, J. M., and van Houte, S. (2016). Evolution and ecology of CRISPR. Ann. Rev. Ecol. Evol. Syst. 47, 307–331. doi: 10.1146/annurev-ecolsys-121415-032428
Ye, J., Zhang, Y., Cui, H., Liu, J., Wu, Y., Cheng, Y., et al. (2018). WEGO 2.0: a web tool for analyzing and plotting GO annotations, 2018 update. Nucleic Acids Res. 46, W71–W75.
Yin, Y., Mao, X., Yang, J., Chen, X., Mao, F., and Xu, Y. (2012). dbCAN: a web resource for automated carbohydrate-active enzyme annotation. Nucleic Acids Res. 40, W445–W451.
Zhang, B., Cheng, Y., Shi, J., Xing, X., Zhu, Y., Xu, N., et al. (2019a). Insights into interactions between vanadium (V) bio-reduction and pentachlorophenol dechlorination in synthetic groundwater. Chem. Eng. J. 375:121965. doi: 10.1016/j.cej.2019.121965
Zhang, B., Wang, S., Diao, M., Fu, J., Xie, M., Shi, J., et al. (2019b). Microbial community responses to vanadium distributions in mining geological environments and bioremediation assessment. J. Geophys. Res. Biogeosci. 124, 601–615. doi: 10.1029/2018jg004670
Zhang, B., Qiu, R., Lu, L., Chen, X., He, C., Lu, J., et al. (2018). Autotrophic vanadium(V) bioreduction in groundwater by elemental sulfur and zerovalent iron. Environ. Sci. Technol. 52, 7434–7442. doi: 10.1021/acs.est.8b01317
Zhang, B., Wang, Z., Shi, J., and Dong, H. (2020). Sulfur-based mixotrophic bio-reduction for efficient removal of chromium (VI) in groundwater. Geochim. Cosmochim. Acta 268, 296–309. doi: 10.1016/j.gca.2019.10.011
Zhong, C., Han, M., Yang, P., Chen, C., Yu, H., Wang, L., et al. (2019). Comprehensive analysis reveals the evolution and pathogenicity of aeromonas, viewed from both single isolated species and microbial communities. mSystems 4:e00252-19.
Zhong, C., Han, M., Yu, S., Yang, P., Li, H., and Ning, K. (2018). Pan-genome analyses of 24 Shewanella strains re-emphasize the diversification of their functions yet evolutionary dynamics of metal-reducing pathway. Biotechnol. Biofuels 11:193.
Zhou, P., Li, X., and Qi, F. (2015). Establishment of a counter-selectable markerless mutagenesis system in Veillonella atypica. J. Microbiol. Methods 112, 70–72. doi: 10.1016/j.mimet.2015.03.010
Keywords: Veillonella atypica, pan-genome, CAZymes, metabolic pathways, CAS-Type IIIA
Citation: Han M, Liu G, Chen Y, Wang D and Zhang Y (2020) Comparative Genomics Uncovers the Genetic Diversity and Characters of Veillonella atypica and Provides Insights Into Its Potential Applications. Front. Microbiol. 11:1219. doi: 10.3389/fmicb.2020.01219
Received: 18 March 2020; Accepted: 13 May 2020;
Published: 23 June 2020.
Edited by:
David W. Ussery, University of Arkansas for Medical Sciences, United StatesReviewed by:
Izumi Mashima, Ohu University, JapanBaogang Zhang, China University of Geosciences, China
Copyright © 2020 Han, Liu, Chen, Wang and Zhang. This is an open-access article distributed under the terms of the Creative Commons Attribution License (CC BY). The use, distribution or reproduction in other forums is permitted, provided the original author(s) and the copyright owner(s) are credited and that the original publication in this journal is cited, in accordance with accepted academic practice. No use, distribution or reproduction is permitted which does not comply with these terms.
*Correspondence: Yan Zhang, emhhbmd5YW5oZm51QGdtYWlsLmNvbQ==