- 1Institute of Food, Nutrition and Health, Laboratory of Food Biotechnology, Department of Health Sciences and Technology, ETH Zurich, Zurich, Switzerland
- 2Genetic Diversity Centre, Department of Environmental Systems Sciences, ETH Zurich, Zurich, Switzerland
- 3Institute for Food Safety and Hygiene, University of Zurich, Zurich, Switzerland
Lactobacillus reuteri is a natural inhabitant of selected animal and human gastrointestinal tract (GIT). Certain strains have the capacity to transform glycerol to 3-hydroxypropionaldehyde (3-HPA), further excreted to form reuterin, a potent antimicrobial system. Reuterin-producing strains may be applied as a natural antimicrobial in feed to prevent pathogen colonization of animals, such as in chicken, and replace added antimicrobials. To date, only seven L. reuteri strains isolated from chicken have been characterized which limits phylogenetic studies and host-microbes interactions characterization. This study aimed to isolate L. reuteri strains from chicken GIT and to characterize their reuterin production and antimicrobial resistance (AMR) profiles using phenotypic and genetic methods. Seventy strains were isolated from faces, crops and ceca of six chicken from poultry farms and samples from slaughterhouse. Twenty-five strains were selected for further characterization. Draft genomes were generated for the new 25 isolates and integrated into a phylogenetic tree of 40 strains from different hosts. Phylogenetic analysis based on gene content as well as on core genomes showed grouping of the selected 25 L. reuteri chicken isolates within the poultry/human lineage VI. Strains harboring pdu-cob-cbi-hem genes (23/25) produced between 156 mM ± 11 and 330 mM ± 14 3-HPA, from 600 mM of glycerol, in the conditions of the test. All 25 chicken strains were sensitive to cefotaxime (MIC between 0.016 and 1 μg/mL) and penicillin (MIC between 0.02 and 4 μg/mL). Akin to the reference strains DSM20016 and SD2112, the novel isolates were resistant to penicillin, possibly associated with identified point mutations in ponA, pbpX, pbpF and pbpB. All strains resistant to erythromycin (4/27) carried the ermB gene, and it was only present in chicken strains. All strains resistant to tetracycline (5/27) harbored tetW gene. This study confirms the evolutionary history of poultry/human lineage VI and identifies pdu-cob-cbi-hem as a frequent trait but not always present in this lineage. L. reuteri chicken strains producing high 3-HPA yield may have potential to prevent enteropathogen colonization of chicken.
Introduction
Lactobacillus reuteri inhabits the gastrointestinal tract (GIT) of selected animals where it forms biofilms on the non-glandular, squamous epithelium lining the upper GIT. In poultry, L. reuteri is the most abundant Lactobacillus species in the GIT, mainly found in the crop and the cecum (Wang et al., 2014). Distinct phylogenetic lineages of L. reuteri are coherent with host origin, reflecting co-evolution of this species with the vertebrate hosts (Oh et al., 2010). The evolutionary adaptation differentiates the species in host-adapted phylogenetic lineages comprised of isolates from rodents (lineages I and III), humans (lineage II), pigs (lineages IV and V) and poultry/human (lineage VI) (Oh et al., 2010; Spinler et al., 2014). Newly identified herbivore strains isolated from goat, sheep, cow, and horse have not yet been assigned to phylogenetic lineages (Yu et al., 2018). Host adaption has been linked to the occurrence of specific functional traits, e.g., rodent L. reuteri isolates possess the genes responsible for the synthesis of urease, as the strains are exposed continuously to urea in the forestomach of mice (Walter et al., 2011). In case of herbivore isolates, specific genes involved in porphyrin and chlorophyll metabolism and biosynthesis of amino acids have been identified, indicating possible host-adaptation mechanisms involving amino acids biosynthesis in herbivores (Yu et al., 2018).
Genomes of poultry and human L. reuteri isolates (lineages II and VI) have been shown to harbor the pdu-cbi-cob-hem operon, as a lineage-specific trait (Frese et al., 2011). This operon contains genes for glycerol and propanediol utilization (pdu) and cobalamin biosynthesis (cbi-cob), hem genes and some accessory genes. Cobalamin is a co-factor for glycerol/diol dehydratase PduCDE (EC 4.2.1.30). PduCDE catalyses the conversion of 1,2-propanediol to propanal, which can be further metabolized by other enzymes of the pdu operon to propanol or propionate (Engels et al., 2016a). Glycerol, a second substrate of PduCDE, is transformed into the intermediate 3-hydroxypropionaldehyde (3-HPA) which can be further metabolized to 1,3-propanediol or 3-hydroxypropionate (Gänzle, 2015). 3-HPA produced from glycerol is released from the cell forming the dynamic multi-compound reuterin system, with broad antimicrobial spectrum and consisting of 3-HPA, its hydrate and dimer and acrolein (Stevens et al., 2010; Engels et al., 2016b). Acrolein, a highly reactive toxicant, was recently shown to be the main component for the antimicrobial activity of reuterin (Engels et al., 2016b; Asare et al., 2018).
Due to the high persistence of L. reuteri in the chicken GIT and the established antimicrobial activity of reuterin, L. reuteri has high potential to be applied as a natural antimicrobial in feed to prevent pathogen infection of chicken (Hou et al., 2015). L. reuteri strains isolated from chicken intestine were shown effective against Salmonella spp. and Escherichia coli resistant to various antibiotics (Nitisinprasert et al., 2000). Moreover, L. reuteri in the early post-hatching period appear to modify the composition of ileum microbiota of broiler chicken, resulting in enrichment of potentially beneficial lactobacilli and suppression of Proteobacteria (Nakphaichit et al., 2011). To select functional L. reuteri strains, a key trait is the determination of their antimicrobial resistance (AMR) profiles to identify intrinsic and extrinsic resistances that may be potentially transferred. Lactobacilli are known to be intrinsically resistant against vancomycin. However, the occurrence of tetracycline and erythromycin genes on mobile elements has been reported for different Lactobacillus spp. (Egervärn et al., 2009).
As of April 2018, among the complete genomes of L. reuteri deposited in the National Center for Biotechnology Information (NCBI), only seven strains were isolated from chicken, representing only 4% of all available NCBI L. reuteri genomes (n = 187) and thus limiting any phylogenetic analysis and host-microbes adaptation studies. The majority of the NCBI L. reuteri deposited genomes comes from strains which had been isolated from mouse (47), human (19) and pig (28), while few originated from sourdough (7), chicken (7), goat (5), cow (5), rat (4) sheep (4), dairy and fermented products (4), horse (3), probiotic capsule (1), and wine (1).
It was, therefore, the aim of this study to isolate and characterize L. reuteri strains from chicken and characterize their reuterin production and AMR profiles using phenotypic and genotypic methods. Draft genomes of the isolates were analyzed combined with 40 L. reuteri genomes of strains previously isolated from different hosts to assess genetic diversity and gain insight into distinguishing features related to chicken and enrich previously phylogenetic characterization of L. reuteri.
Materials and Methods
Bacterial Strains and Growth Conditions
L. reuteri DSM20016 (Leibniz Institute DSMZ-German Collection of Microorganisms and Cell Cultures, Braunschweig, Germany) and L. reuteri SD2112 (BioGaia AB, Stockholm, Sweden) were used as reference strains. Reference strains, as well as all L. reuteri isolated in this study, were propagated anaerobically (Oxoid, AnaeroGenTM, Basingstoke, United Kingdom) at 37°C in de Man, Rogosa and Sharpe (MRS) broth medium (Biolife, Milan, Italy).
Bacterial Isolation
Six Lohmann brown layer chicken (13 weeks old) were obtained from six chicken farms in Switzerland. Chicken samples were taken under the supervision of a veterinarian, as per Swiss animal handling regulation. Ceca and feces were aseptically collected. In parallel, 10 whole gut of Cobb 500 broiler chicken were obtained from Schönholzer Werner abattoir in Wädenswil (Zurich) and transported to the lab within 1 h.
L. reuteri strains were isolated from the chicken GIT samples using the protocol previously described (Walter et al., 2011), with some modifications. Briefly, 1 g of crop, cecal or fecal content was added to 10 mL of sterile phosphate buffer saline (PBS) (137 mM NaCl, 2.7 mM KCl, 10 mM Na2HPO4, 2 mM KH2PO4, pH 7.4) and homogenized in a stomacher (BagMixer® 400 P, Interscience, Saint Nom, France) at high speed for 1 min. To ensure the initial cultivation of L. reuteri from chicken crop and feces, the modified MRS medium (mMRS) was used, containing maltose, the preferred substrate of heterofermentative lactobacilli, and fructose as electron acceptor (Zhao and Gänzle, 2018). The mMRS contained (g/l): tryptone, 10; yeast extract, 5; meat extract, 5; maltose, 10; fructose, 5; K2HPO4.3H20, 2.6; KH2PO4, 4; cysteine-HC1, 0.5; NH4CI, 3; Tween 80, 1 (Stolz et al., 1995; Zhao and Gänzle, 2018). Suspensions from samples were serially diluted and spread on mMRS. The agar plates were incubated overnight at 42°C under anaerobic condition using AnaeroGen 2.5 L (Thermo Fisher Diagnostics AG, Pratteln, Switzerland). Replica plates were prepared using Scienceware replica plater and velveteen squares (Sigma-Aldrich, Buchs, Switzerland), and incubated overnight as presented above. After incubation, one plate was overlaid with 500 mM glycerol agar (1% agar) and incubated at 37°C for 30 min for testing reuterin production of colonies. A colourimetric method was used with the addition of 5 mL 2,4-dinitrophenylhydrazine (0.1% in 2 M HCl), 3 min incubation, removal of the solution, and addition of 5 mL 5M KOH. L. reuteri colonies showing purple zones indicating reuterin synthesis were streaked on MRS agar plates, and single colony were subcultured three times in MRS broth (1% inoculum, 18 h at 42°C). Few colonies which show a colony morphology of L. reuteri but no purple zone were picked as negative controls. Species confirmation and reuterin production quantification was performed for both positive and selected negative selected colonies. The strains were named PTA X, with X indicating the chicken ID, with no number being assigned to the abattoir sample; F and C indicate isolating from feces and cecum, respectively, followed by the number of the isolated strains.
Bacterial Identification
Genomic DNA was isolated using a lysozyme-based cell wall digestion followed by the Wizard genomic DNA purification kit (Promega, Dübendorf, Switzerland). Total DNA was quantified by absorbance at 260 nm using NanoDrop® ND-1000 Spectrophotometer (Witec AG, Littau, Switzerland). The DNA quality was analyzed by electrophoresis in 1.2% (w/v) agarose gel and Gel Red staining (VWR International AG, Dietikon, Switzerland). The DNA samples were stored at −20°C until further analysis.
To confirm the identity of the isolates, the 1.6 kbp full region of 16S rRNA gene was amplified by PCR using universal primers bak4 (5′-AGGAGGTGATCCARCCGCA-3′) and bak11w (5′-AGTTTGATCMTGGCTCAG -3′) (Greisen et al., 1994; Goldenberger et al., 1995). The 16S rRNA PCR assay consisted of 5 min at 95°C, followed by 35 cycles of 15 s at 95°C, 30 s at 60°C and 2 min at 72°C and final extension for 7 min at 72°C. Sanger sequencing of the PCR amplicon was performed at GATC (Konstanz, Germany). To identify the closest homologs, DNA sequences obtained were aligned using the Basic Local Alignment Search Tool (BLAST) (Altschul et al., 1990). Sequence with 100% homology was used to identify L. reuteri.
Strain Typing
The enterobacterial repetitive intergenic consensus (ERIC) sequence was used to differentiate between isolates, as ERIC has the ability to discriminate Lactobacillus reuteri isolates to species and strain levels (Stephenson et al., 2009). The ERIC-PCR assay was performed using ERIC1R (5′-ATGTAAGCTCCTGGGGATTCAC-3′) and ERIC2 (5′-AAGTAAGTGACTGGGGTGAGCG-3′) primers (Versalovic et al., 1991; Ventura and Zink, 2002; Stephenson et al., 2010). The ERIC-PCR assay was performed using 100 ng of template DNA of each isolate. The protocol consisted of 7 min at 95°C, followed by 30 cycles of 30 s at 90°C, 1 min at 52°C, and 8 min at 65°C, and a final extension for 16 min at 65°C. The ERIC-PCR amplicons were analyzed on 2% (wt/vol) agarose gels for 6 h at 60 Volts. GeneRuler DNA ladder mix (Fermentas, Le Mont-sur-Lausanne, Switzerland) was used as a molecular size marker according to the manufacturer’s directions, and gels were visualized by Gel Red staining. Gels were analyzed using Gel Compar II version 6.5 software package (Applied Maths, Sint-Martens-Latem, Belgium). L. reuteri strains with unique ERIC profiles were visually selected, and amplicons re-run on a single agarose gel. A dendrogram of similarity was generated from the gel with selected isolates using the Pearson correlation similarity coefficient and the unweighted-pair group method (UPGMA) with arithmetic averages, and 1% optimization. A cutoff of 84% similarity was used to define the “ERIC type.” Based on the clustering obtained, confirmed isolates with unique ERIC type were selected for whole-genome sequencing and characterization.
Generation and Annotation of Draft Genomes
Genomic DNA from the isolated strains was obtained as described above and standardized to 100 ng/μL. The whole genomes of 25 L. reuteri isolates were sequenced with the standard set of 96 Illumina paired-end barcodes on a HiSeq 2500 Illumina Technology (Illumina Inc., San Diego, CA, United States) with 2× 125 high output mode. The genomic library was generated using reagents from NEBNext Illumina preparation kit. Raw paired-end reads were quality trimmed using the default settings of Trimmomatic (Bolger et al., 2014). Read pairs were merged by FLASH (Mag and Salzberg, 2011). De novo assembly was performed with SPAdes assembler (version 3.12) with –careful option (Bankevich et al., 2012). The quality of the assembly based on evolutionarily informed expectations of gene content from near-universal single-copy orthologs selected from OrthoDB v9 was assessed by BUSCO (Simão et al., 2015) and QUAST (Gurevich et al., 2013). Reference guided ordering of scaffolds based on iterative alignment steps was performed by QUAST using L. reuteri DSM20016 genome as a reference. SeqKit (Shen et al., 2016) and QUAST were used to retrieve genome features. The complete genome of L. reuteri DSM20116 type strain was compared individually with each of the 25 draft genomes of L. reuteri chicken isolates. Average nucleotide identity (ANI) values between two genomic datasets were calculated using JSpecies (Richter et al., 2016). Genomes with ANI values above 95% were considered as belonging to the same species (Goris et al., 2007).
Twenty-five L. reuteri draft genomes (this study) and 40 NCBI deposited L. reuteri genomes of strains isolated from different hosts (chicken, human, mouse, rat, pig, sourdough, goat, sheep, cow, horse; Supplementary Table S1) were structurally annotated using the PROKKA 3.10.1 suite (Seemann, 2014). The 40 NCBI complete genomes were chosen to cover host diversity of L. reuteri, and included genomes previously used in comparative studies (Oh et al., 2010; Duar et al., 2017) and all available genomes from chicken isolates at the time of data analysis (April 2018). Only contigs higher than 500 bp were included in the analysis, and Lactobacillus was selected as the reference database for the annotation (options used: –genus Lactobacillus –species reuteri –usegenus Lactobacillus –mincontiglen 500).
Comparative Genomics
Comparative genome analysis was based on gene content tree, core genome phylogenetic tree and a nucleotide-content similarity matrix (ANI matrix). For the generation of the gene content tree, a matrix based on gene content (binary data for presence or absence of each annotated gene) was generated comprising all annotated genes of 25 L. reuteri draft genomes plus the 40 L. reuteri NCBI genomes. The gene content tree was then constructed using the hierarchical cluster analysis (hcust) on R 3.4.4 while a core genome phylogenetic tree was calculated using EDGAR 2.3 (Blom et al., 2016). Concatenated sequences were used to calculate a distance matrix which provided the input for the neighbor-joining method with PHYLIP implementation. EDGAR 2.3 calculated the core genome of each identified clusters as a set of orthologous genes present in all strains belonging to each cluster. For the generation of the similarity matrix, ANI was calculated on EDGAR 2.3 with all-against-all comparisons at the nucleotide level for all 65 L. reuteri strains.
Identified lineages were named based on host origin of the strains, and lineages specific features were determined using “Define metacontigs” function in EDGAR 2.3. Core groups were created for the sets of strains derived from the phylogenetic analysis. Venn diagrams were designed in EDGAR to identify and count the number of shared genes of the core genomes of identified clusters of strains (cluster A, B and C) as well as between human VI and poultry VI strains, human VI and human II strains and poultry lineage VI, human lineage VI and human lineage II of L. reuteri strains. Unique genes of poultry/human lineage VI were manually categorized based on UniProt protein description into the following groups: transport proteins, DNA-binding proteins, transferases, lyases, oxidoreductases, membrane proteins, hydrolases, virulence-related proteins, RNA-binding and prophage-related proteins. The presence of genes of the pdu-cob-hem-cbi cluster was manually checked, and a heatmap of presence/absence of genes of interest was designed with GraphPad Prism 8.0 (GraphPad Software Inc. La Jolla California, United States). For each of the reuterin-positive L. reuteri strains, the nucleotide sequence of pduA, pduB, pduC, pduD and pduE was extracted and concatenated. Concatenated sequences were used to construct a phylogenetic tree with the Neighbor-Joining Method and Tamura-Nei genetic distance model using MEGA version X (Kumar et al., 2018). The resulting trees were rooted using pduABCDE genes of Salmonella Typhimurium SL1344 as outgroup. By using the same method, individual phylogenetic trees per each of the pdu genes were also built.
The presence of AMR genes in the assembled genomes was also manually checked and integrated with the results from ResFinder 3.0 tool (Zankari et al., 2012). A database was created with all (n = 17) NCBI deposited L. reuteri plasmid sequences. The database was used to determine location of extracted ermB and tetW gene sequences associated with resistance phenotypes, by NCBI Blastn alignment. AMR genes that aligned completely with more than 99% identity with one of the plasmids of the database, were considered plasmid located.
For penicillin-resistant L. reuteri strains, point mutations (SNPs) in penicillin-binding proteins genes ponA, pbpX, pbpF and pbpB were checked by aligning deduced amino acid sequences of resistant and sensitive strains with NCBI-deposited sequences for Lactobacillus in Geneious 9.1.8.
PCR for tetW and ermB Detection
PCR for tetW and ermB genes was performed to confirm the genomic data. TetW was amplified with tetw-rev and tetw-fw primers (Kastner et al., 2006; Egervärn et al., 2009). The PCR protocol comprised 35 cycles of 95°C for 30 s, 56°C 45 s and 72°C 30 s. The ermB gene was amplified with primers ermA1 (5′-TCTAAAAAGCATGTAAAAGAA-3′) and ermA2 (5′-CTTCGATAGTTTATTAATATTAGT-3′) using 30 PCR cycles composed of 95°C for 30 s, 52°C 45 s, 72°C 2 min (Egervärn et al., 2009).
Reuterin Production
Reuterin production was determined using a two-step process (Stevens et al., 2013). Cell pellets obtained from 16 h L. reuteri cultures (OD600 ≈ 8.0) were collected by centrifugation, resuspended in 600 mM glycerol solution and incubated at 25°C for 2 h. For reuterin biosynthesis, the concentrations of glycerol, 3-HPA and acrolein were measured by High Performance Liquid Chromatography using Refractive Index (HPLC-IR, Hitachi LaChrome, Merck, Dietikon, Switzerland) and Ion-Exclusion Chromatography with Pulsed-Amperometric Detection (IC-PAD) analysis, respectively (Engels et al., 2016b; Asare et al., 2018). For HPLC-RI, an Aminex HPx-87H column and sulfuric acid (10 mM) was used as eluent. The IC-PAD Thermo Scientific (Reinach, Switzerland) ICS-5000+ system was equipped with a quaternary gradient pump, a thermostated autosampler and an electrochemical detector with a cell containing an Ag/AgCl reference electrode and a disposable thin-film platinum working electrode tempered at 25°C. Analytes were separated with a Thermo Scientific IonPac ICE-AS1 4× 250 mm ion-exclusion column with a guard column, operated at 30°C. The solvent system was isocratic 0.1 M methanesulfonic acid at 0.2 mL/min for 36 min.
Antimicrobial Susceptibility Profiling
L. reuteri chicken isolates and reference strains L. reuteri SD2112 and DSM20016 were tested for susceptibility to cefotaxime (CFX), erythromycin (ERM), penicillin (PEN), tetracycline (TET), vancomycin (VAN), and ciprofloxacin (CIP), using gradient diffusion MTSTM strips (Liofilchem, Roseto degli Abruzzi, Italy). These antibiotic were selected as they belong to World Organisation for Animal Health (OIE) list of antimicrobials of veterinary importance, and to the World Health Organisation (WHO) list of antimicrobials of critical importance for human health (World Organisation for Animal Health [OIE], 2015; World Health Organisation [WHO], 2019). In particular, one antibiotic per critically important antibiotic family was tested: (i) quinolone family (CIP), (ii) tetracycline family (TET), (iii) glycopeptides family (VAN); (iv) B-lactam family (PEN); (v) macrolide family (ERM); (vi) cephalosporin family (CFX). The concentration range tested was 0.016 to 256 μg/mg, except for CIP (0.002 to 32 μg/mL). Briefly, 16 h overnight L. reuteri cultures were added to a sterile MRS broth (1% v/v) and incubated at 37°C for 6 h. The bacterial cultures were standardized to OD600 1.0 (corresponding to approximately 108 CFU/mL) using a PowerWave XS microplate spectrophotometer (BioTek, Sursee, Switzerland). The diluted culture (106 CFU/mL) was evenly swabbed on MRS agar plates in duplicate using a sterile cotton bud. MTSTM strips were placed on the surface of the agar and incubated at 37°C for 24 h in anaerobic condition supplied by the gas package (AnaeroGen, Thermo Fisher Diagnostics AG, Pratteln, Switzerland). The minimum inhibitory concentration (MIC) was recorded as the point where inhibition curves intersect the scale on the MTSTM strip.
Data Accession Number
The draft genomes of 25 L. reuteri strains have been deposited in NCBI under the accession numbers indicated in Table 1. This Whole Genome Shotgun project has been deposited at DDBJ/ENA/GenBank under the accession PRJNA473635.
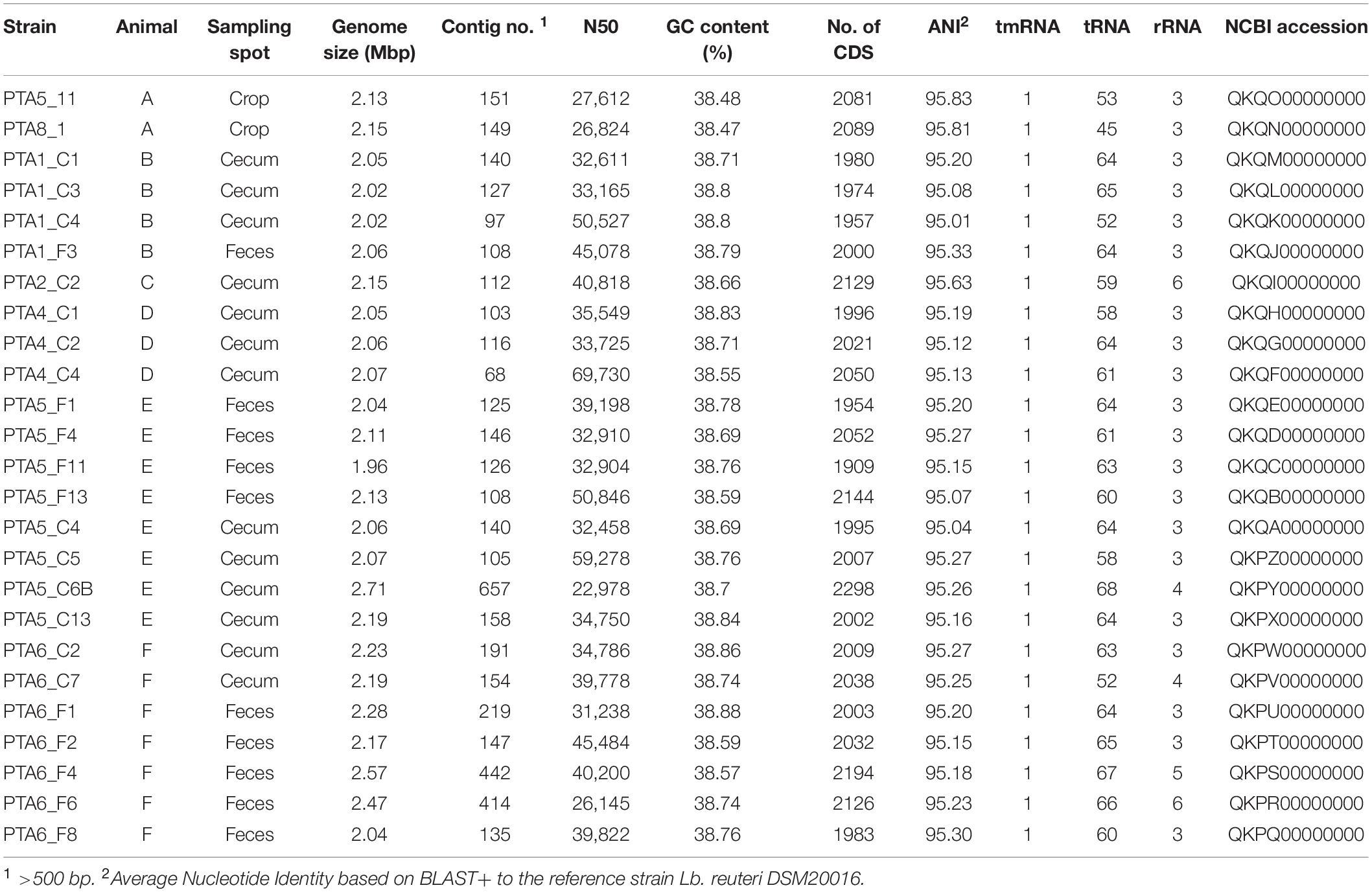
Table 1. Draft genome features of 25 L. reuteri strains isolated from chicken GIT and sequenced in this study.
Results and Discussion
L. reuteri Isolation and Typing
In the chicken GIT, L. reuteri forms biofilm in the crop and is among the most abundant Lactobacillus species commonly found in the cecum and colon. However, to date, only seven chicken L. reuteri strains (P43, An71, An166, 1366, JCM 1081, CSF8, and SKK-OGDONS-01) have been sequenced, and some of them included in previous phylogenetic analysis (Oh et al., 2010; Frese et al., 2011; Wegmann et al., 2015; Duar et al., 2017; Lee et al., 2017).
By applying a reuterin selective colourimetric method on the plate, a total of 70 L. reuteri strains were isolated from feces and cecum of 6 chicken and from the crops of chicken obtained from the abattoir. Among them, 50 were reuterin-positive while 20 were reuterin-negative. The identity of all strains was confirmed by sequencing of the 16S rRNA gene with 100% similar to the reference strain L. reuteri DSM20016. The colourimetric method applied allowed an easy phenotypic isolation of reuterin-positive colonies, that accounted for approximately 50% of the bacterial colonies obtained on MRS isolation plates. This result indicated the high frequency of reuterin-producing L. reuteri strains in the chicken GIT, compared to the absence of reuterin production phenotype for isolated rodent strains (Walter et al., 2011).
Based on the similarity obtained from ERIC PCR profiles, 31 isolates with unique ERIC profiles were visually selected and further clustered with Bionumerics to confirm the uniqueness (Supplementary Figure S1). Based on the dendrogram obtained for the 31 isolates, 23 reuterin-positive strains with unique ERIC profiles and 2 reuterin-negative strains were selected for whole genome sequencing and further functional characterization (Supplementary Figure S1). Among them, 13 were isolated from cecum and 10 from feces while the 2 reuterin-negative strains originated from the crop.
Genomes Analysis of New L. reuteri Chicken Isolates
The whole genomes of 25 L. reuteri strains were sequenced, and draft genomes were characterized. Seven genomes from chicken L. reuteri isolates were available prior to this study. The 25 new L. reuteri chicken isolates obtained in this study significantly increase the proportion of chicken strain in the L. reuteri genome database (n = 184).
Strains had an average genome size of 2.159 ± 0.17 Mbp (Table 1). BUSCO assembly assessment showed good quality of assembly with 431 to 433 single-copy orthologous on a total of 433 (Supplementary Figure S2). Three genomes appeared to have lower quality (PTA5_C6B, PTA6_F4, and PTA6_F6), this was reflected by higher number of contigs, bigger genome size, and higher number of CDS compared to the other genomes (Table 1 and Supplementary Figure S3b). Shotgun reads were assembled into contigs higher than 500 bp, ranging from 68 (PTA4_C4) to 657 (PTA5_C6B). The average N50 value, defined as the minimum contig length needed to cover 50% of the genome, was 38337 ± 10.6 while the average guanine-cytosine (GC) content was 38.1 ± 0.11%. The total number of coding sequences (CDs) ranged from 1909 to 2298, depending on the isolates. In every draft genome, 1 tmRNA gene, 45 to 68 tRNA genes and 3 to 6 rRNA genes were identified (Table 1). A total number of 2078 genes was annotated, with a core set of 817 shared genes among the 25 new chicken isolates. The number of annotated genes that were unique to each strain, as compared to the other newly annotated strains, ranged from 0 to 31 (data not shown), showing a similar genetic content of the new chicken isolates of L. reuteri. The genomic features of L. reuteri isolates of this study in terms of genome size, GC content and number of CDS were comparable with that of the 40 L. reuteri NCBI analyzed genomes from different hosts, suggesting not genetic diversity driven by the host (Supplementary Figure S3).
Genomes of Strains Isolated From Crop and Cecum/Feces Differ in Composition
ERIC profiles did not distinguish isolates from feces and cecum this could be due to the transition of strains in the chicken GIT from the cecum to feces. On the other hand, non-reuterin producing L. reuteri isolates from the crop (PTA8_1, PTA14_19, PTA5_11) appeared to have a unique ERIC profile (Supplementary Figure S1). To further investigate the ecology of isolates originating from different regions of the chicken GIT, we compared the number of unique and shared genes of isolates from cecum, feces or crop (Figure 1 and Supplementary Table S2). When comparing cecum and feces isolates, few unique genes were identified for cecum isolates, as compared to feces isolates (Figure 1B). Despite a high number of shared genes between crop and cecum (Figure 1A), and crop and feces isolates (Figure 1C), 630 and 616 genes appeared to be unique in crop isolates as compared to cecum and feces, respectively (Figures 1A,C and Supplementary Table S2). When isolates originating from the three GIT regions were compared, 592 genes appeared unique to crop strains (Figure 1D). Indeed, some of the genes unique to strains obtained from the chicken crop include enzymes related arginine, glutaminase, and glutathione metabolism (Supplementary Table S2). These genes were previously shown to support acid resistance in L. reuteri (Teixeira et al., 2014), and were upregulated in murine forestomach biofilms compared to gene expression in the lumen of colon and cecum (Schwab et al., 2014). Interestingly, citrate lyase required for citrate metabolism was present only in crop isolates of L. reuteri. The optimum pH for citrate metabolism lay between 4.0 to 5.0 (Palles et al., 1998) which is the pH usually measured in the crop of chicken (Kierończyk et al., 2016). Our observations might suggest that there are two populations of L. reuteri in the chicken GIT, one inhabiting the crop and the other in the cecum recovered in the feces. A more robust selection and genomes sequencing of strains from chicken crop and cecum is required to test the adaptation of L. reuteri to specific location in the chicken GIT.”
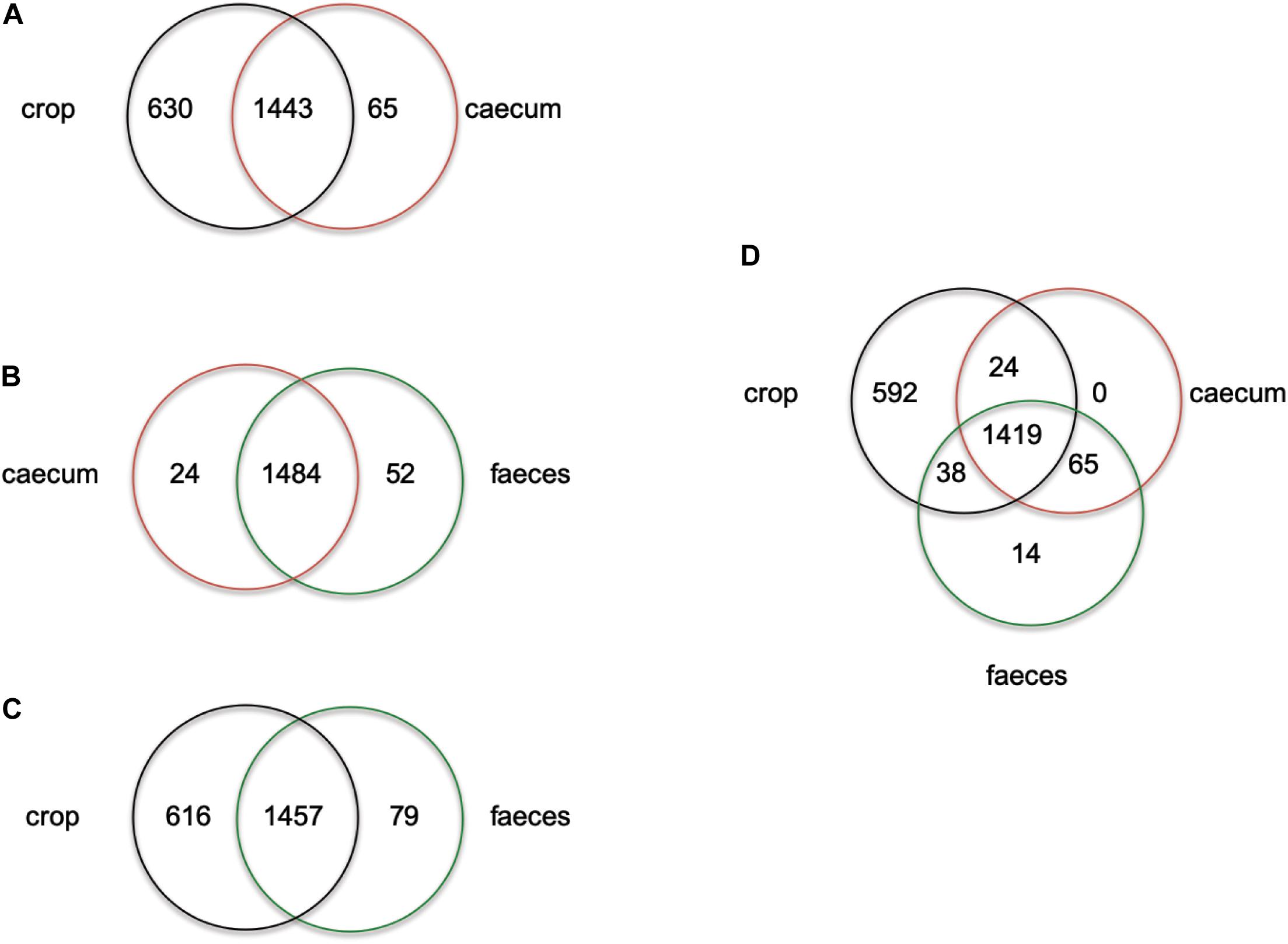
Figure 1. Venn diagram showing the number of unique or shared genes between L. reuteri chicken isolates from crop vs. cecum (A), cecum vs. feces (B), crop vs. feces (C) and cecum vs. feces vs. crop (D). Individual unique genes of crop isolates, in each comparison, are listed in Supplementary Table S2 in alphabetic order.
Comparative Phylogenetic Analysis of L. reuteri Strains From Different Hosts
The apparent relatedness between microbial community composition in the gut and host phylogeny has been interpreted as evidence of coevolution (Ley et al., 2008). Symbiotic gut microbes associated with the host are predicted to evolve host-specific traits and, as a result, display enhanced ecological performances in their host (Garcia and Gerardo, 2014; Duar et al., 2017). To assess evolution and adaptation of L. reuteri strains to different hosts, the gene content of chicken isolates was analyzed together with that of 40 L. reuteri strains available by NCBI, obtained from different hosts: human (6), rat (1), mouse (3), pig (4), sourdough (4), goat (5), sheep (4), cow (4), horse (3) and chicken (6).
The gene content tree, in which strains sharing more genes clustered together, identified three main clusters namely cluster A, cluster B, and cluster C, that contained previously observed L. reuteri lineages (Oh et al., 2010) (Figure 2 and Supplementary Table S1). Those host-adapted lineages were first described after the characterization of the genetic structure of L. reuteri strains isolated from human, mouse, rat, pig, chicken, and turkey, and the same lineage names were also applied in our study for coherency (Oh et al., 2010). Regarding herbivore strains, no lineages have been defined before our study. Cluster A, corresponding to the previously defined poultry/human lineage VI, comprised all 25 L. reuteri chicken isolates of this study and all, except one (P43), chicken NCBI isolates (Figure 2). The same cluster also included two human strains (SD2112 and CF48-3A). Those two isolates were previously described as clustering in an unexpected way (Oh et al., 2010; Duar et al., 2017; Lee et al., 2017). Identified cluster B included the majority of herbivorous isolates (new defined herbivorous lineage VII of our study) in addition to four human (DSM20016, MM2_3, IRT and JCM1112), one sourdough (CRL1098), one rodent (mlc3) and one porcine (20-02) strains, belonging to lineages II, III and IV previously defined (Oh et al., 2010). Cluster C was composed of pig, herbivorous, sourdough, and rodent isolates (Figure 2) corresponding to lineages I, III, V and newly herbivorous VIII lineage. ANI analysis of the 65 L. reuteri isolates (Supplementary Figure S4) identified the same three clusters, except strains 20_02 (pig) and mlc3 (mouse) that were assigned to cluster C instead of cluster B.
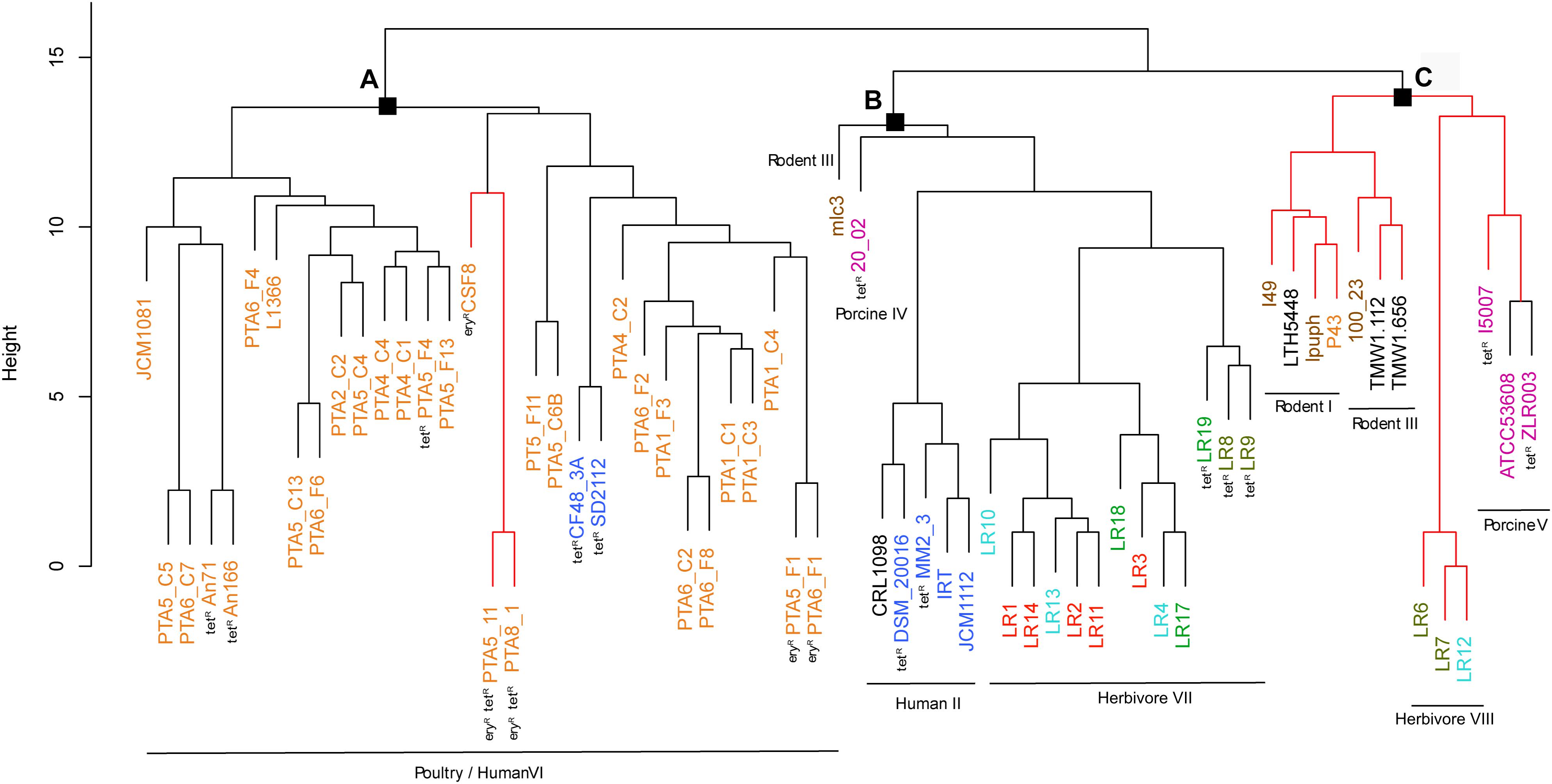
Figure 2. Phylogenetic tree based on gene content matrix (presence or absence of annotated gene) of 65 L. reuteri strains from different hosts (25 genomes from this study and 40 from NCBI, Supplementary Table S1). Different colors represent different hosts, blue: human; yellow: chicken; pink: pig; brown: mouse/rat; light blue: cow; red: goat; green: horse; brown/green: sheep; black: sourdough. The red branches indicate the reuterin-negative strains. (A–C) indicate identified clusters. Strains which harbor AMR genes for tetracycline (tet) and erythromycin (ery) are indicated as tetR and eryR, respectively.
The phylogenetic tree based on core genomes of the 65 genomes covered a core of 1152 genes per genome, for a total of 74880 genes. In agreement with the gene content tree, all isolated L. reuteri chicken strains clustered together with NCBI chicken isolates (strains JCM1081, CSF8, An71 and An166), except P43, forming poultry/human lineage VI (Oh et al., 2010). As indicated above, this cluster also included the two human isolates CF48-3A and SD2112 (cluster A, Figure 3). Cluster B was composed of strains from human lineage II and herbivorous lineage VII, as previously above in gene content tree, and the same applied for cluster C which was composed of porcine lineage V and herbivorous lineage VIII. L. reuteri strains belonging to rodent lineage III, rodent lineage I and porcine lineage IV clustered differently from the gene content tree, which includes accessory genes. This might indicate gene loss or acquisition of genes by horizontal gene transfer or be just the results from genome sequence quality.
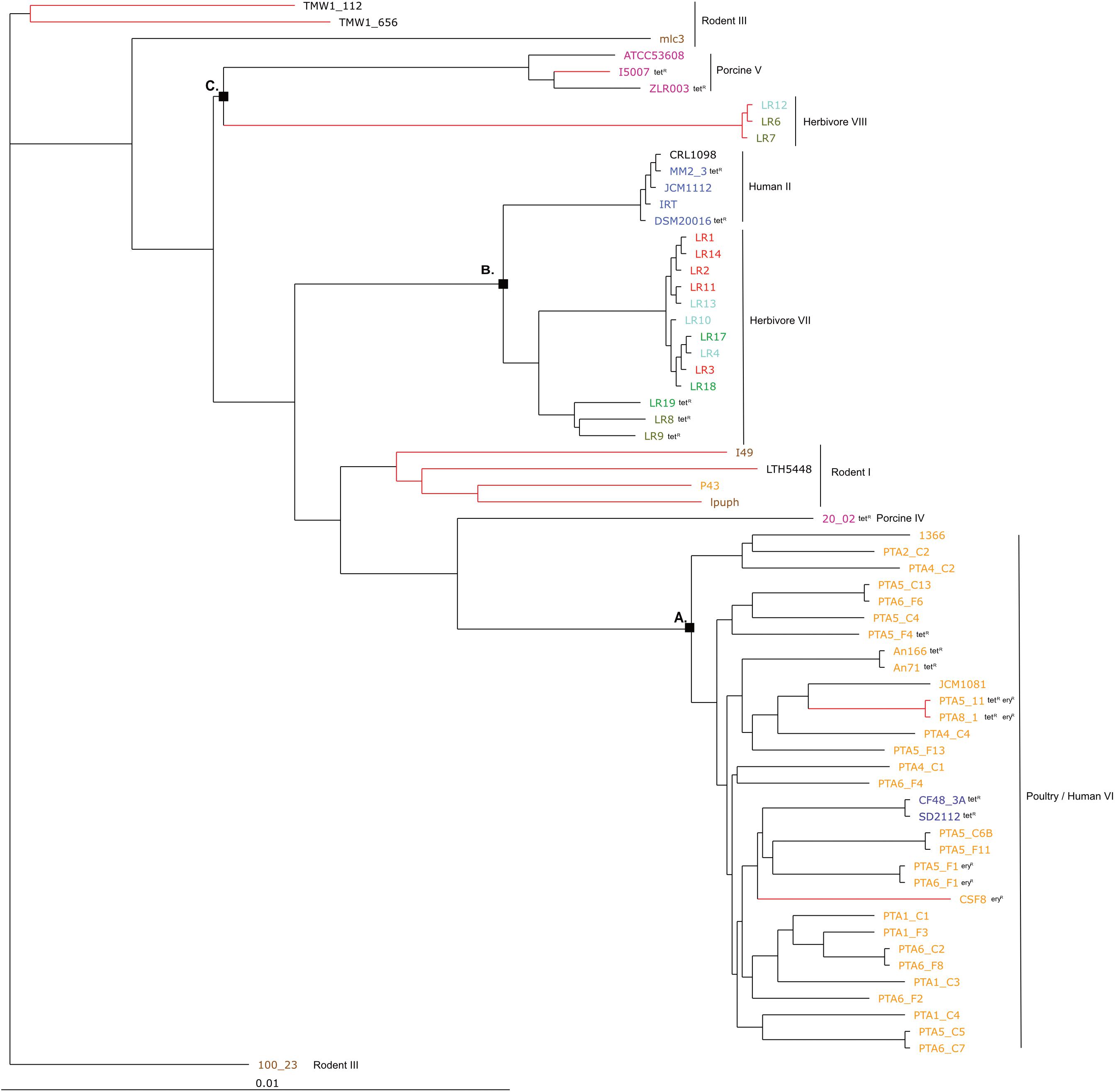
Figure 3. Phylogenetic tree based on core genes of 65 L. reuteri strains from different hosts (25 genomes from this study and 40 from NCBI, Supplementary Table S1) based on the neighbor-joining method (PHYLIP implementation). Different colors represent different hosts, blue: human; yellow: chicken; pink: pig; brown: mouse/rat; light blue: cow; red: goat; green: horse; brown/green: sheep; black: sourdough. The red branches indicate the reuterin-negative strains. A, B and C indicate identified clusters in the gene content tree (Figure 1). Strains which harbor AMR genes for tetracycline (tet) and erythromycin (ery) are indicated as tetR and eryR, respectively.
Overall, the three different analyses (gene content tree, ANI analysis and core genome tree) performed in this study grouped together human and poultry isolates of lineage VI similar to Frese et al. (2011) (cluster A), human strains of lineage II with herbivore strains of lineage VII strains (cluster B) and porcine strains of lineage V with herbivore strains of lineage VIII strains (cluster C). A previous study demonstrated that isolates of human lineage VI only colonized the chicken and not the human GIT (Duar et al., 2017). When the number of shared or unique genes among poultry lineage VI isolates or human lineage VI isolates were compared, 554 genes appeared to be unique in human VI isolates, compared to only 28 unique genes for chicken isolates (Figure 4B). Furthermore, when comparing human VI with human II isolates (Figure 4C), a higher number of shared genes was shown, and this was also the case when comparing poultry lineage VI with human lineage VI and human lineage II strains (Figure 4D). All analyses performed confirmed the existence and composition of the poultry/human lineage VI, which was substantially enriched by the 25 chicken isolates from our study. Those strains appear to share both core and accessory genes and to be highly similar also at the nucleotide level.
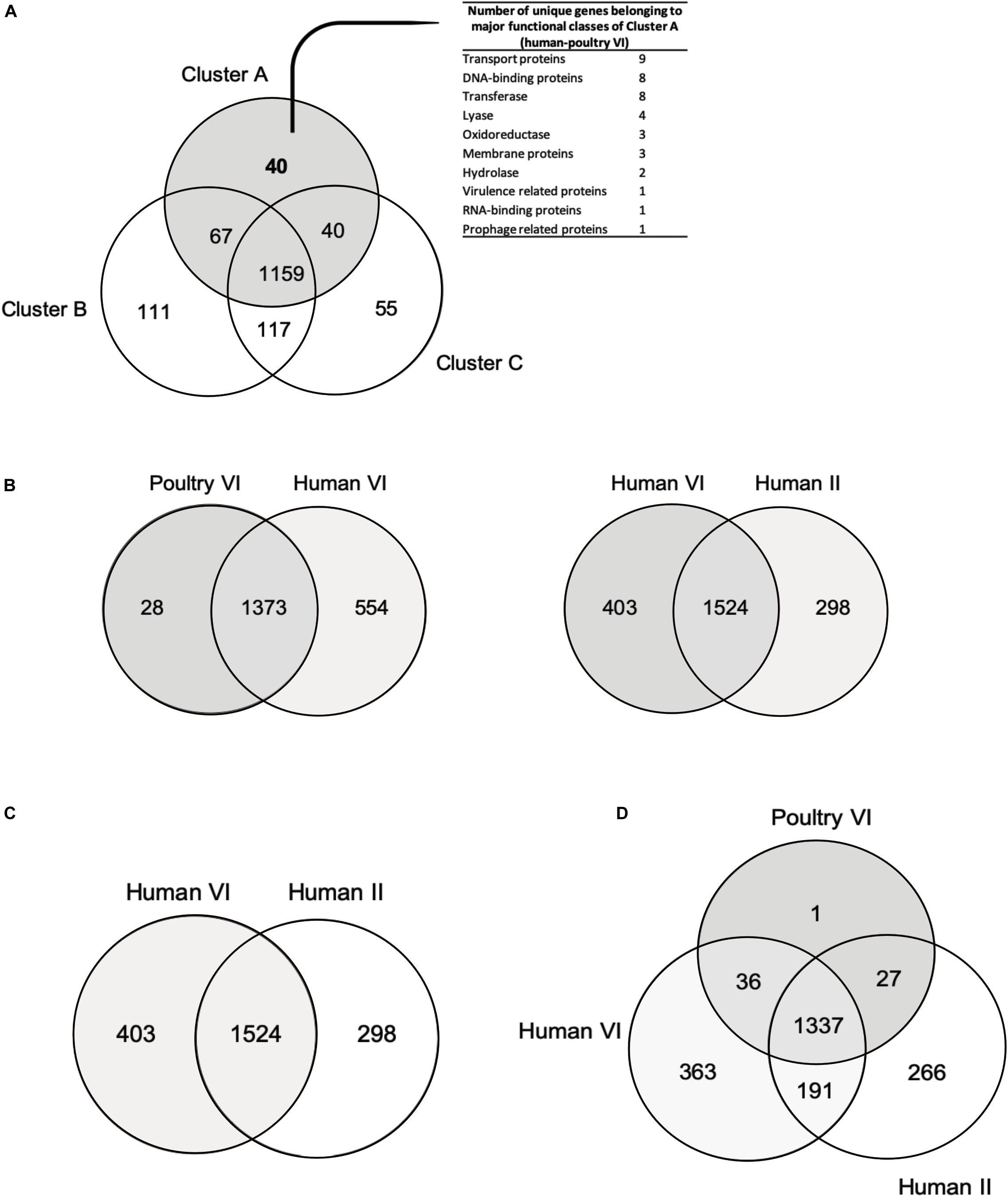
Figure 4. Venn diagrams showing number of reciprocal best hits among different L. reuteri subset of core genomes. (A) Number of shared and unique genes of core genomes of cluster A (poultry/human lineage VI), cluster B (lineages human II, herbivore VII, porcine IV and rodent III) and cluster C (lineages rodent I, rodent III, herbivore VIII and porcine V). (B) Number of shared and unique genes of poultry VI genomes and human VI genomes. (C) Number of shared and unique genes between human VI and human II strains. (D) Number of shared and unique between poultry VI, human VI and human II lineages. Unique genes of human/poultry VI clades (A) and human VI isolates (B) are listed in Supplementary Table S3.
Genes Unique for the Poultry/Human Lineage VI
Presence of host-specific lineages in itself does not necessarily provide evidence for natural selection, as a cluster can arise by neutral processes, such as genetic dispersion (Oh et al., 2010). It has been demonstrated how strains from rodent display elevated fitness in mice, and biofilm formation in the forestomach is restricted to strains from rodent lineages. Moreover, L. reuteri rodents strains were able to effectively colonize rodent host in vivo (Oh et al., 2010; Frese et al., 2011; Duar et al., 2017). However, this was not the case for pig isolates (Wegmann et al., 2015; Duar et al., 2017). Here, forty unique genes of the poultry/human lineage VI were identified (Figure 4A and Supplementary Table S3) and were mainly categorized as transport proteins DNA-binding proteins and transferase proteins. Such genes could not be directly linked with adaptation to chicken physiology or feeding. Further studies are needed to elucidate the specific role(s) of those unique genes that may be linked to chicken adaptation.
Reuterin Synthesis
The presence and composition of reuterin operon genes (pdu-cbi-cob-hem) was investigated in all 65 genomes (Figure 5), and reuterin production was determined as a marker for PduCDE activity. All L. reuteri strains isolated in this study that possessed the complete pdu-cbi-cob-hem operon produced 3-HPA when incubated in 600 mM glycerol. In contrast, strains, PTA5_11 and PTA8_1 only possessed hemH, hemA, cobC and cobB but lacked all the other operon genes (Figure 5) and therefore did not produce 3-HPA. Under the conditions of the test, 3-HPA yield ranged from 156.9 mM ± 11.0 (PT6_F1) to 330.2 mM ± 14.9 (PTA4_C4) starting from 600 mM glycerol (Figure 5). Reference strains DSM 20016 (human lineage II) produced 132.8 ± 4.3 mM while SD2112 (human lineage VI) produced 432.9 ± 9 0 mM 3-HPA.
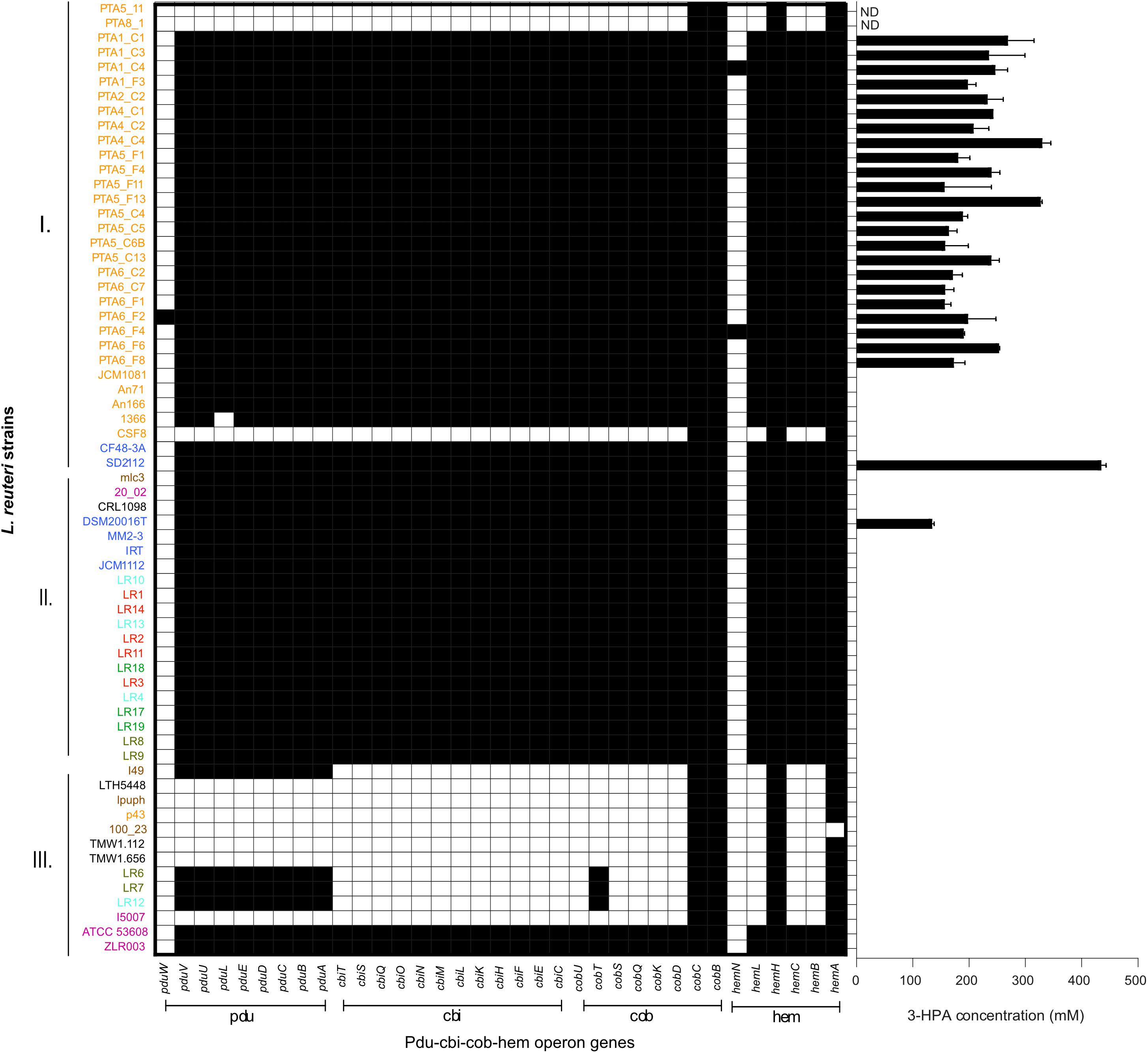
Figure 5. Reuterin operon genes (pdu-cbi-cob-hem) detected in the genomes of 65 L. reuteri listed in Supplementary Table S1 and 3-HPA production of 25 strains isolated from chicken in this study. Black indicates the presence of a gene. Different colors represent different hosts, blue: human; yellow: chicken; pink: pig; brown: mouse/rat; light blue: cow; red: goat; green: horse; brown/green: sheep; black: sourdough. ND not determined.
All cluster A strains (corresponding to poultry/human lineage VI) harbored pdu-cob-cbi-hem genes, except for a small sub-cluster of three by reuterin-negative chicken isolates CSF8, PTA5_11 and PTA8_1. Isolates assigned to Cluster B possessed pdu-cob-cbi-hem and have been shown to mostly form reuterin on MRS agar plates overlaid with 500 mM glycerol agar (Walter et al., 2011). Isolates of this cluster lacked pduW and hemN genes, which therefore seem not essential for reuterin production. These genes were also not detected in the vast majority of reuterin-positive strains isolated in our study (Figure 5). In contrast, the prevalence of pdu-cob-cbi-hem scattered in Cluster C comprising isolates of rodent lineages I and III, herbivorous and porcine lineages VIII and V, respectively. Only strains ATCC53608 and ZLR003 possessed a complete functional pdu-cob-cbi-hem operon while cluster C rodent isolates lpuph and 100_23 lack the majority of the operon genes.
Interestingly, herbivore isolates LR6, LR7, LR12 and the rodent isolate I49 possessed the pdu but not the cbi and cob genes, although cobalamin (cbi and cob gene) is a cofactor for 3-HPA production. Therefore these strains are likely not able to form 3-HPA from glycerol (or propanal from 1,2-propanediol) unless they acquire the vitamin from other sources or microbes. When looking at the pduABCDE phylogenetic tree, LR6, LR12 and I49 are clustered all together, indicating a highly conserved pdu gene cluster (Figure 6).
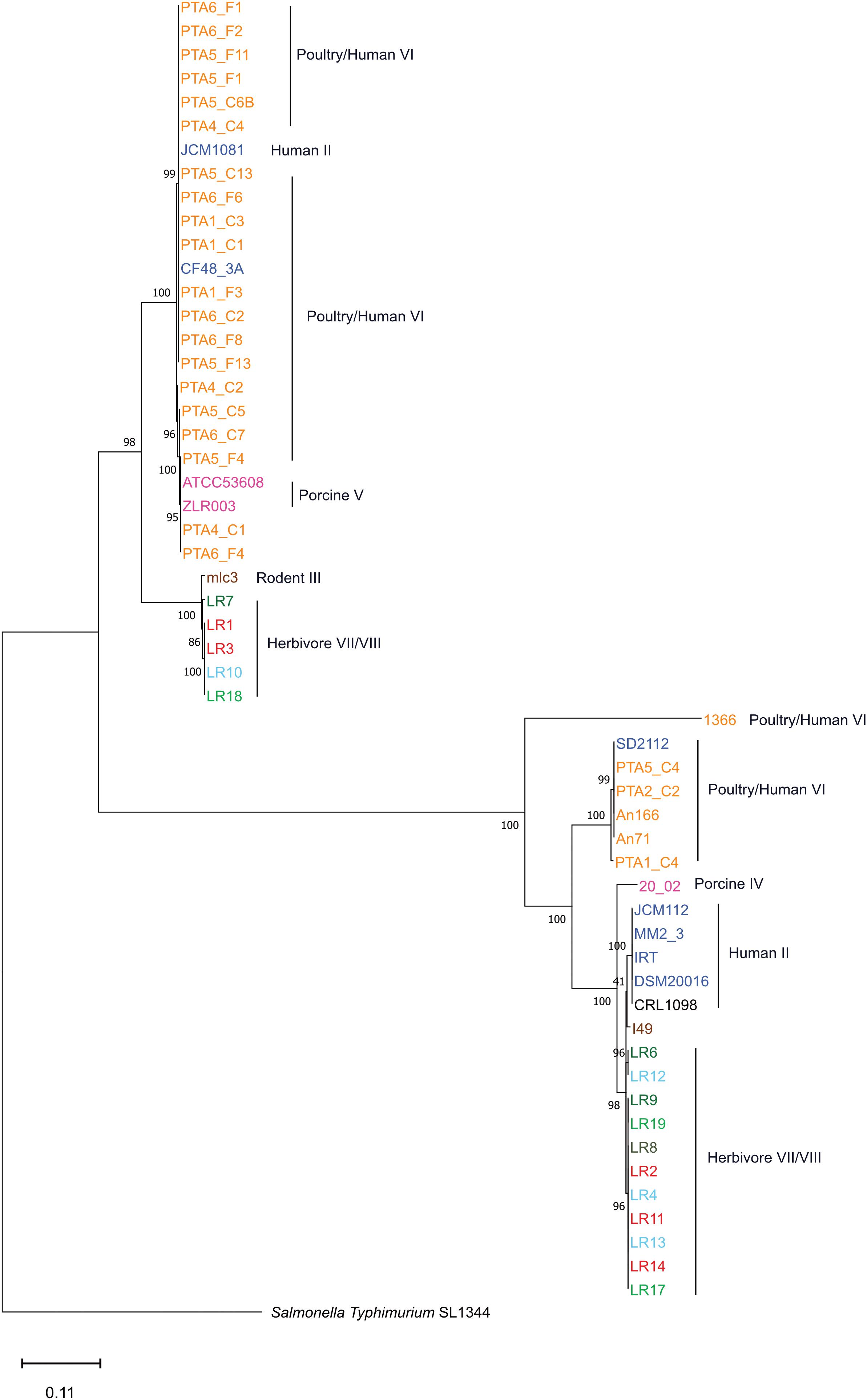
Figure 6. Gene tree based on the nucleotide sequence of concatenated pduA, pduB, pduC, pduD, pduE genes of L. reuteri reuterin-positive genomes of this study (Neighbor Joining Method). Different color represents different hosts, blue: human; yellow: chicken; pink: pig; brown: mouse/rat; light blue: cow; red: goat; green: horse; brown/green: sheep; black: sourdough.
Rodent strains, which are mostly reuterin-negative based on the analysis of operon genes in this study, are considered the root of the evolutionary history of L. reuteri-host associates (Duar et al., 2017), which as a consequence, suggests that the pdu-cbi-cob-hem operon and thus reuterin production was acquired later during L. reuteri. To further study development of the pdu cluster, concatenated sequences of pduABCDE were aligned, and a gene tree was constructed (Figure 6). The pduABCDE gene tree separated into two different branches each mainly composed of herbivores or poultry/human isolates, not showing clear host-dependent separation (Figure 6). The same clustering was obtained when gene trees were built based on individual pduA-pduE genes (Supplementary Figure S5).
Antimicrobial Susceptibility Profiles of L. reuteri Strains
The horizontal transfer of AMR genes is a rising risk concern, and the absence of transferable AMR genes must be demonstrated for application of new strains in food and feed (Rychen et al., 2018). Antimicrobials used in farmed animals for diseases prevention have been associated with an increase frequency of resistant bacteria in chickens, swine, and other food-producing animals GIT (Chang et al., 2015). The high use of antimicrobials in animal production is likely to accelerate the development of AMR in pathogens, as well as in commensal organisms, resulting in treatment failures, economic losses and source of the gene pool for transmission to humans (Chang et al., 2015). Chicken is one of the most widespread food industries worldwide, and various antimicrobials are used to treat infections mainly in chicks (Sahoo et al., 2010; Agyare et al., 2019).
The antimicrobial susceptibility profile of L. reuteri chicken isolates and reference strains DSM20016 and SD2112 showed that all strains were sensitive to cefotaxime (MIC values from 0.016 to 1 μg/mL) (Table 2). All chicken isolates were also sensitive to penicillin with MIC values from 0.02 and 3 μg/mL, in contrast to DSM20116 and SD2112 that showed resistance to this antibiotic (MIC > 256 ug/mL). Penicillin resistance was shown to result from point mutations of the chromosomally located genes encoding penicillin-binding proteins Pbp (Rosander et al., 2008). Penicillin-binding genes pbpX, pbpF, pbpB and ponA were identified in all 65 strains with both resistant and sensitive phenotype (Table 2 and Supplementary Table S4). Several SNPs were observed for DSM20016 (Table 3), especially in ponA and pbpX_2, which led to point mutations of the corresponding proteins. Only one amino acid substitution at position 134 of PbpX_2 was shared among the two resistant strains: DSM20016 possessed a Q instead of H (H134Q) while in the same position strain SD2112 had a Y (H134Y). The substitution at this position may be the one responsible for the penicillin-resistant phenotype observed for DSM20016 and SD2112.
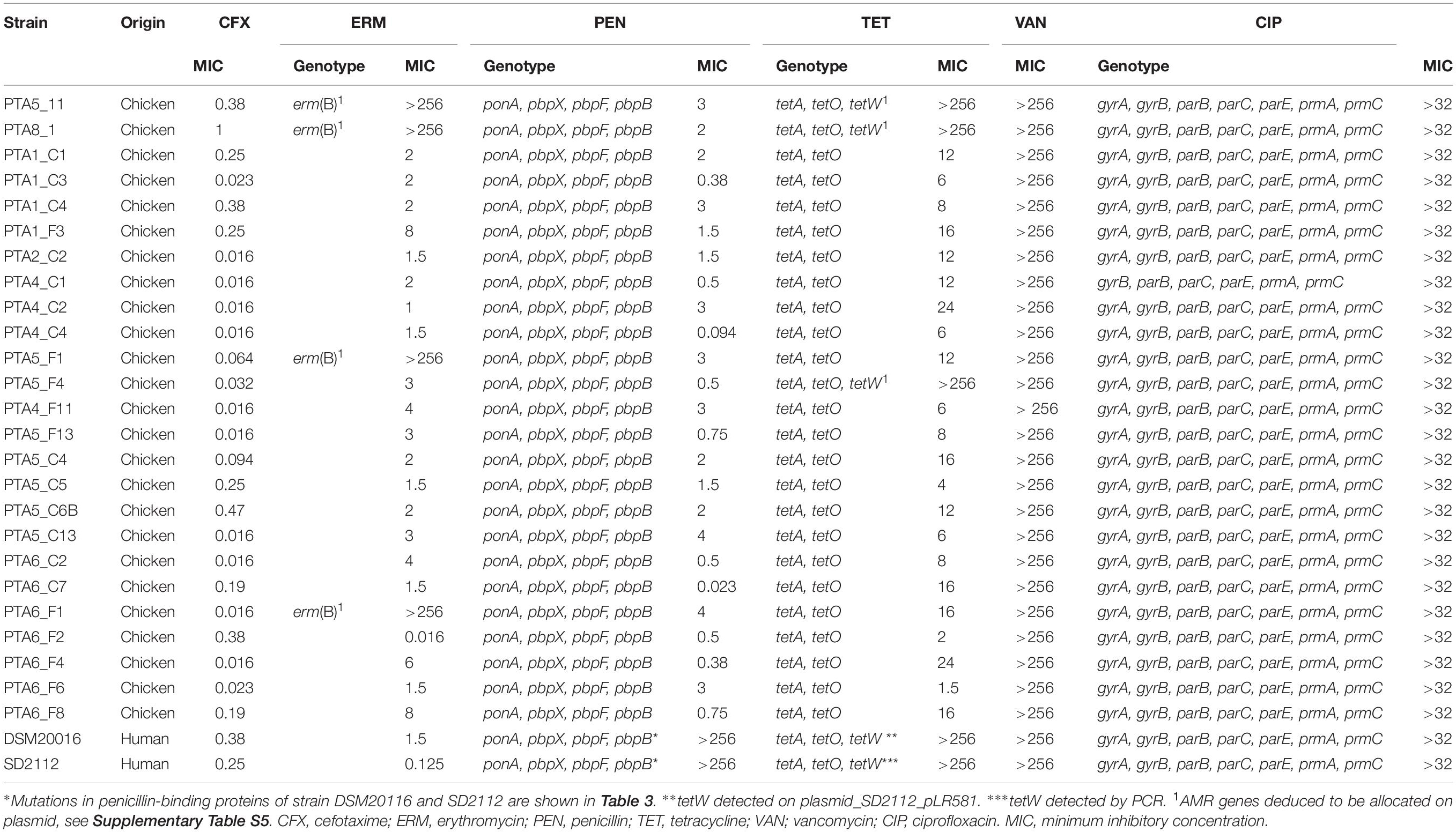
Table 2. Antimicrobial resistance (AMR) profiles of 25 L. reuteri strains isolated from chicken in this study and of reference strains measured using MTSTM strips (MIC, μg/mL), and associated AMR genes detected in their draft genomes.
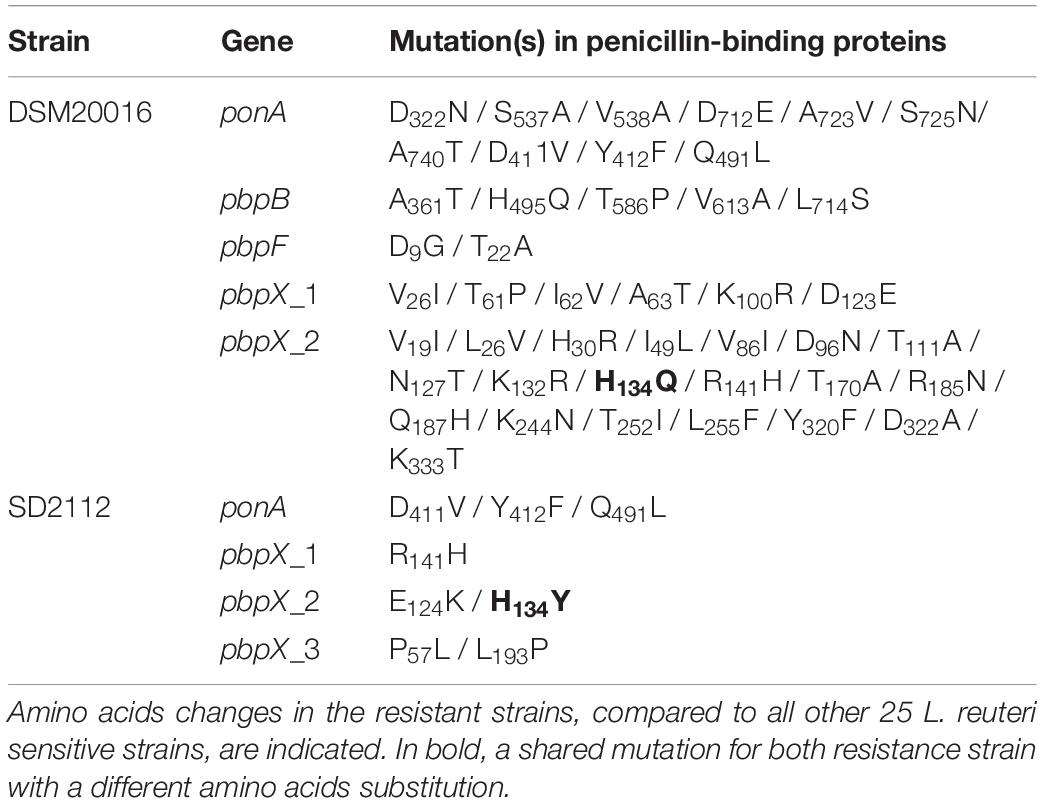
Table 3. Point mutations identified in penicillin-binding protein genes of resistant strains L. reuteri DSM20016 and L. reuteri SD2112.
Four chicken isolates of this study (PTA5_11, PTA8_1, PTA5_F1 and PTA6_F1) showed resistance phenotype (MIC > 256 μg/mL) to erythromycin, confirmed by the presence of ermB, which is usually found on a plasmid (Egervärn et al., 2009; Abriouel et al., 2015). The ermB gene encodes enzymes that modify the 23S rRNA by adding one or two methyl groups, reducing the binding to the ribosome of different classes of antibiotics (Gupta et al., 2003). The presence or absence of ermB gene in the genome of erythromycin resistant strains was confirmed by using PCR (data not shown). For all resistant strains (PTA5_11, PTA8_1, PTA5_F1, and PTA6_F1), ermB appeared to be mostly likely located on plasmid (Supplementary Table S5). Among the 40 NCBI strains analyzed in this study, ermB was also detected in the genome of chicken isolate CSF8 (Figures 2, 3).
Tetracycline resistance genes tetA and tetO were detected in all 65 L. reuteri genomes analyzed but did not appear to be directly correlated with this resistance phenotype (Table 2 and Supplementary Table S4). The only 3 chicken isolates resistant to tetracycline (MIC > 256 μg/mL) possessed additionally the tetW in their genome (PTA5_11, PTA8_1, and PTA5_F4), similar to DSM20016 and SD2112 (MIC > 256 μg/mL). Tetracycline resistance has been described to be located on a plasmid, for example, in SD2112 (pLR581) (Kastner et al., 2006). For DSM20016, tetW was not detected on the chromosome but was amplified by PCR. For all resistant chicken strains of this study (PTA5_11, PTA8_1, and PTA5_F4), tetW gene was deduced to be plasmid located (Supplementary Table S5). Besides tetW, tetM, tetL and tetC were also associated with tetracycline resistance were identified in the genomes of different isolates (MM2_3, I5007, 20_02, ZLR003, LR8, LR9, and LR19). Interestingly, tetracycline-resistant chicken isolates (An71, An166) harbored tetW while herbivorous resistant strains (LR8, LR9, and LR19) possessed tetM. In contrast, pig isolates harbored tetM and tetW (I5007 and 20_02) or tetW and tetL (ZLR003) (Supplementary Table S4). Tetracyclines are widespread antimicrobials extensively used in livestock partly due to their broad-spectrum activity and low cost compared to other antibiotics (Economou and Gousia, 2015; Granados-Chinchilla and Rodríguez, 2017). This might explain the widespread of tetracycline resistance gene pool in farm animals.
Lactobacilli are suggested to be intrinsically resistant to vancomycin and ciprofloxacin (Hummel et al., 2007). In agreement with previous studies (Egervärn et al., 2009), all chicken L. reuteri strains of this study were resistant to vancomycin (MIC > 256 μg/mL). Vancomycin resistance in lactobacilli is linked to the vanX gene encoding a d-Ala-d-Ala dipeptidase (Klein et al., 2000). Other vancomycin resistance genes were described in the literature with vanA, vanB, vanC and vanE (Klein et al., 2000; Kastner et al., 2006). None of those genes was detected in the genomes of L. reuteri in agreement with previous studies (Klein et al., 2000; Kastner et al., 2006). However, changes in membrane composition have also been associated with intrinsic vancomycin resistance (Delcour et al., 1999). VanH, a D-lactate dehydrogenase gene was detected in one pig strain (ATCC 53608) (Faron et al., 2016). The same gene had been previously associated with vancomycin resistance of Enterococcus faecium (Bugg et al., 1991). Ciprofloxacin resistance seems to be widely spread among lactobacilli (Hummel et al., 2007; Li et al., 2015; Guo et al., 2017). All 25 chicken isolates were resistant to ciprofloxacin with MIC > 32 μg/mL, and all 65 genomes analyzed possessed the six genes for which mutations were correlated with ciprofloxacin resistance, namely gyrB, parB, parC, parE, prmA and prmC, while gyrA was present in all but one (PTA4_C1) isolate.
L. reuteri has been affiliated to different hosts, which might be exposed to different levels and types of antimicrobials and is commercially used as probiotic in food and feed (Hou et al., 2015; Ávila et al., 2017; Asare et al., 2018; Chen et al., 2018). The results of this study indicated that L. reuteri chicken isolates harbor some AMR genes. In view of application L. reuteri in feed to prevent pathogen infection, strains without transferable AMR genes must be carefully selected. The first applied L. reuteri probiotic strains (SD2112) harbors the tetracycline-resistant gene tetw on a plasmid (Kastner et al., 2006). This strain was, however, cured for the plasmid-free daughter strain DSM17938 (Rosander et al., 2008), which is commercially used.
Conclusion
This study substantially enriched the pool of chicken L. reuteri strains for comparative genomics and evolutionary studies. Isolates from the crop appeared to have a unique genetic profile compared to isolates from more distal region of the GIT, which might suggest that chicken are colonized by two populations of L. reuteri. The phylogenetic analysis confirmed the co-evolution of human isolates of lineage VI with chicken (poultry/human lineage VI). Despite the high number of chicken isolates of lineage VI, the two human isolates still shared a higher number of genes with isolates belonging to human lineage II, as compared to poultry lineage VI. The pool of L. reuteri chicken isolates of this study may be useful to select and characterize strains exhibiting reuterin production activity, and possibly develop application in chicken, as a natural antimicrobial system to prevent pathogen infections and colonization of the chicken GIT.
Data Availability Statement
The datasets generated for this study can be found in the BioProject ID: PRJNA473635.
Ethics Statement
Swiss regulation did not require the study to be reviewed or approved by an ethics committee because the samples were taken under direct and strict supervision of a veterinarian.
Author Contributions
AG, PA, CS, RS, and CL designed the study. AG and PA conducted the experiments and analyzed the data. AG, PA, CS, NZ, RG, and CL drafted the manuscript. All authors read and approved the final manuscript.
Funding
This project was funded by the COOP Research Program on “Sustainability in Food Value Chains” of the ETH-Zürich World Food System Centre and the ETH Zürich Foundation for supporting this project. The COOP Research Program is supported by the COOP Sustainability Fund. PA was supported by the Swiss Government Excellence Ph.D. Scholarships for foreign students. The EDGAR platform used in this study is financially supported by the BMBF grant FKZ 031A533 within the de.NBI network.
Conflict of Interest
The authors declare that the research was conducted in the absence of any commercial or financial relationships that could be construed as a potential conflict of interest.
Acknowledgments
This manuscript has been released as a Pre-Print at BioRxiv (Greppi et al., 2019). The authors are grateful to Alfonso Die for the HPLC measurements. The data analyzed in this paper were generated in collaboration with the Genetic Diversity Centre (GDC), ETH Zurich.
Supplementary Material
The Supplementary Material for this article can be found online at: https://www.frontiersin.org/articles/10.3389/fmicb.2020.01166/full#supplementary-material
References
Abriouel, H., Casado Muñoz, M. D. C., Lavilla Lerma, L., Pérez Montoro, B., Bockelmann, W., Pichner, R., et al. (2015). New insights in antibiotic resistance of Lactobacillus species from fermented foods. Food Res. Int. 78, 465–481. doi: 10.1016/j.foodres.2015.09.016
Agyare, C., Etsiapa Boamah, V., Ngofi Zumbi, C., and Boateng Osei, F. (2019). “Antibiotic use in poultry production and its effects on bacterial resistance,” in Antimicrobial Resistance – A Global Threat, ed. Y. Kumar, (London: InTech Open), doi: 10.5772/intechopen.79371
Altschul, S. F., Gish, W., Miller, W., Myers, E. W., and Lipman, D. J. (1990). Basic local alignment search tool. J. Mol. Biol. 215, 403–410. doi: 10.1016/S0022-2836(05)80360-2
Asare, P. T., Greppi, A., Stettler, M., Schwab, C., Stevens, M. J. A., and Lacroix, C. (2018). Decontamination of minimally-processed fresh lettuce using reuterin produced by Lactobacillus reuteri. Front. Microbiol. 9:1421. doi: 10.3389/fmicb.2018.01421
Ávila, M., Gómez-Torres, N., Delgado, D., Gaya, P., and Garde, S. (2017). Industrial-scale application of Lactobacillus reuteri coupled with glycerol as a biopreservation system for inhibiting Clostridium tyrobutyricum in semi-hard ewe milk cheese. Food Microbiol. 66, 104–109. doi: 10.1016/j.fm.2017.04.010
Bankevich, A., Nurk, S., Antipov, D., Gurevich, A. A., Dvorkin, M., Kulikov, A. S., et al. (2012). SPAdes: a new genome assembly algorithm and its applications to single-cell sequencing. J. Comput. Biol. 19, 455–477. doi: 10.1089/cmb.2012.0021
Blom, J., Kreis, J., Spänig, S., Juhre, T., Bertelli, C., Ernst, C., et al. (2016). EDGAR 2.0: an enhanced software platform for comparative gene content analyses. Nucleic Acids Res. 44, W22–W28. doi: 10.1093/nar/gkw255
Bolger, A. M., Lohse, M., and Usadel, B. (2014). Trimmomatic: a flexible trimmer for Illumina sequence data. Bioinformatics 30, 2114–2120. doi: 10.1093/bioinformatics/btu170
Bugg, T. D. H., Wright, G. D., Dutka-Malen, S., Arthur, M., Courvalin, P., and Walsh, C. T. (1991). Molecular basis for vancomycin resistance in Enterococcus faecium BM4147: biosynthesis of a depsipeptide peptidoglycan precursor by vancomycin resistance proteins VanH and VanA. Biochemistry 30, 10408–10415. doi: 10.1021/bi00107a007
Chang, Q., Wang, W., Regev-Yochay, G., Lipsitch, M., and Hanage, W. P. (2015). Antibiotics in agriculture and the risk to human health: how worried should we be? Evol. Appl. 8, 240–247. doi: 10.1111/eva.12185
Chen, S., Chen, L., Chen, L., Ren, X., Ge, H., Li, B., et al. (2018). Potential probiotic characterization of Lactobacillus reuteri from traditional Chinese highland barley wine and application for room-temperature-storage drinkable yogurt. J. Dairy Sci. 101, 5780–5788. doi: 10.3168/jds.2017-14139
Delcour, J., Ferain, T., Deghorain, M., Palumbo, E., and Hols, P. (1999). The biosynthesis and functionality of the cell-wall of lactic acid bacteria. Antonie Van Leeuwenhoek 76, 159–184.
Duar, R. M., Frese, S. A., Lin, X. B., Fernando, S. C., Burkey, T. E., Tasseva, G., et al. (2017). Experimental evaluation of host adaptation of Lactobacillus reuteri to different vertebrate species. Appl. Environ. Microbiol. 83:132. doi: 10.1128/AEM.00132-17
Economou, V., and Gousia, P. (2015). Agriculture and food animals as a source of antimicrobial-resistant bacteria. Infect. Drug Resist. 8, 49–61. doi: 10.2147/IDR.S55778
Egervärn, M., Roos, S., and Lindmark, H. (2009). Identification and characterization of antibiotic resistance genes in Lactobacillus reuteri and Lactobacillus plantarum. J. Appl. Microbiol. 107, 1658–1668. doi: 10.1111/j.1365-2672.2009.04352.x
Engels, C., Ruscheweyh, H. J., Beerenwinkel, N., Lacroix, C., and Schwab, C. (2016a). The common gut microbe Eubacterium hallii also contributes to intestinal propionate formation. Front. Microbiol. 7:713. doi: 10.3389/fmicb.2016.00713
Engels, C., Schwab, C., Zhang, J., Stevens, M. J. A., Bieri, C., Ebert, M.-O., et al. (2016b). Acrolein contributes strongly to antimicrobial and heterocyclic amine transformation activities of reuterin. in revision. Nat. Publ. Gr. 6, 1–13. doi: 10.1038/srep36246
Faron, M. L., Ledeboer, N. A., and Buchan, B. W. (2016). Resistance mechanisms, epidemiology, and approaches to screening for vancomycin-resistant Enterococcus in the health care setting. J. Clin. Microbiol. 54, 2436–2447. doi: 10.1128/JCM.00211-16
Frese, S. A., Benson, A. K., Tannock, G. W., Loach, D. M., Kim, J., Zhang, M., et al. (2011). The evolution of host specialization in the vertebrate gut symbiont Lactobacillus reuteri. PLoS Genet. 7:e01314. doi: 10.1371/journal.pgen.1001314
Gänzle, M. G. (2015). Lactic metabolism revisited: metabolism of lactic acid bacteria in food fermentations and food spoilage. Curr. Opin. Food Sci. 2, 106–117. doi: 10.1016/j.cofs.2015.03.001
Garcia, J. R., and Gerardo, N. M. (2014). The symbiont side of symbiosis: do microbes really benefit? Front. Microbiol. 5:510. doi: 10.3389/fmicb.2014.00510
Goldenberger, D., Perschil, I., Ritzler, M., Goldenberger, D., and Ritzler, M. (1995). A simple “ universal “ DNA extraction procedure using SDS and proteinase K is compatible with direct PCR amplification. Genome Res. 4, 368–370. doi: 10.1101/gr.4.6.368
Goris, J., Konstantinidis, K. T., Klappenbach, J. A., Coenye, T., Vandamme, P., and Tiedje, J. M. (2007). DNA-DNA hybridization values and their relationship to whole-genome sequence similarities. Int. J. Syst. Evol. Microbiol. 57, 81–91. doi: 10.1099/ijs.0.64483-0
Granados-Chinchilla, F., and Rodríguez, C. (2017). Tetracyclines in food and feedingstuffs: from regulation to analytical methods, bacterial resistance, and environmental and health implications. J. Anal. Methods Chem. 2017:497. doi: 10.1155/2017/1315497
Greisen, K., Loeffelholz, M., Purohit, A., and Leong, D. (1994). PCR primers and probes for the 16S rRNA gene of most species of pathogenic bacteria, including bacteria found in cerebrospinal fluid. J. Clin. Microbiol. 32, 335–351.
Greppi, A., Asare, P. T., Schwab, C., Zemp, N., Stephan, R., and Lacroix, C. (2019). Isolation and comparative genomic analysis of reuterin-producing Lactobacillus reuteri from poultry gastrointestinal tract. bioRxiv [Preprint], doi: 10.1101/793299
Guo, H., Pan, L., Li, L., Lu, J., Kwok, L., Menghe, B., et al. (2017). Characterization of antibiotic resistance genes from Lactobacillus isolated from traditional dairy products. J. Food Sci. 82, 724–730. doi: 10.1111/1750-3841.13645
Gupta, A., Vlamakis, H., Shoemaker, N., and Salyers, A. A. (2003). A new bacteroides conjugative transposon that carries an ermB gene. Appl. Environ. Microbiol. 69, 6455–6463. doi: 10.1128/AEM.69.11.6455-6463.2003
Gurevich, A., Saveliev, V., Vyahhi, N., and Tesler, G. (2013). QUAST: quality assessment tool for genome assemblies. Bioinformatics 29, 1072–1075. doi: 10.1093/bioinformatics/btt086
Hou, C., Zeng, X., Yang, F., Liu, H., and Qiao, S. (2015). Study and use of the probiotic Lactobacillus reuteri in pigs: a review. J. Anim. Sci. Biotechnol. 6, 1–8. doi: 10.1186/s40104-015-0014-3
Hummel, A. S., Hertel, C., Holzapfel, W. H., and Franz, C. M. A. P. (2007). Antibiotic resistances of starter and probiotic strains of lactic acid bacteria. Appl. Environ. Microbiol. 73, 730–739. doi: 10.1128/AEM.02105-06
Kastner, S., Perreten, V., Bleuler, H., Hugenschmidt, G., Lacroix, C., and Meile, L. (2006). Antibiotic susceptibility patterns and resistance genes of starter cultures and probiotic bacteria used in food. Syst. Appl. Microbiol. 29, 145–155. doi: 10.1016/j.syapm.2005.07.009
Kierończyk, B., Rawski, M., Długosz, J., Świa̧tkiewicz, S., and Józefiak, D. (2016). Avian crop function - A review. Ann. Anim. Sci. 16, 653–678. doi: 10.1515/aoas-2016-0032
Klein, G., Hallmann, C., Casas, I. A., Abad, J., Louwers, J., and Reuter, G. (2000). Exclusion of vanA, vanB and vanC type glycopeptide resistance in strains of Lactobacillus reuteri and Lactobacillus rhamnosus used as probiotics by polymerase chain reaction and hybridization methods. J. Appl. Microbiol. 89, 815–824. doi: 10.1046/j.1365-2672.2000.01187.x
Kumar, S., Stecher, G., Li, M., Knyaz, C., and Tamura, K. (2018). MEGA X: molecular evolutionary genetics analysis across computing platforms. Mol. Biol. Evol. 35, 1547–1549. doi: 10.1093/molbev/msy096
Lee, J.-Y., Han, G. G., Choi, J., Jin, G.-D., Kang, S.-K., Chae, B. J., et al. (2017). Pan-genomic approaches in Lactobacillus reuteri as a porcine probiotic: investigation of host adaptation and antipathogenic activity. Microb. Ecol. 74, 709–721. doi: 10.1007/s00248-017-0977-z
Ley, R. E., Lozupone, C. A., Hamady, M., Knight, R., and Gordon, J. I. (2008). Worlds within worlds: evolution of the vertebrate gut microbiota. Nat. Rev. Microbiol. 6, 776–788. doi: 10.1038/nrmicro1978
Li, S., Li, Z., Wei, W., Ma, C., Song, X., Li, S., et al. (2015). Association of mutation patterns in GyrA and ParC genes with quinolone resistance levels in lactic acid bacteria. J. Antibiot. 68, 81–87. doi: 10.1038/ja.2014.113
Mag, T., and Salzberg, S. L. (2011). FLASH: fast length adjustment of short reads to improve genome assemblies. Bioinformatics 1, 2957–2963. doi: 10.1093/bioinformatics/btr507
Nakphaichit, M., Thanomwongwattana, S., Phraephaisarn, C., Sakamoto, N., Keawsompong, S., Nakayama, J., et al. (2011). The effect of including Lactobacillus reuteri KUB-AC5 during post-hatch feeding on the growth and ileum microbiota of broiler chickens. Poult. Sci. 90, 2753–2765. doi: 10.3382/ps.2011-01637
Nitisinprasert, S., Nilphai, V., Bunyun, P., and Sukyai, P. (2000). Screening and identification of effective thermotolerant lactic acid bacteria producing antimicrobial activity against Escherichia coli and Salmonella sp. resistant to antibiotics. Kasetsart J. Nat. Sci. 34, 387–400.
Oh, P. L., Benson, A. K., Peterson, D. A., Patil, P. B., Moriyama, E. N., Roos, S., et al. (2010). Diversification of the gut symbiont Lactobacillus reuteri as a result of host-driven evolution. ISME J. 4, 377–387. doi: 10.1038/ismej.2009.123
Palles, T., Beresford, T., Condon, S., and Cogan, T. M. (1998). Citrate metabolism in Lactobacillus casei and Lactobacillus plantarum. J. Appl. Microbiol. 85, 147–154. doi: 10.1046/j.1365-2672.1998.00486.x
Richter, M., Rosselló-Móra, R., Oliver Glöckner, F., and Peplies, J. (2016). JSpeciesWS: a web server for prokaryotic species circumscription based on pairwise genome comparison. Bioinformatics 32, 929–931. doi: 10.1093/bioinformatics/btv681
Rosander, A., Connolly, E., and Roos, S. (2008). Removal of antibiotic resistance gene-carrying plasmids from Lactobacillus reuteri ATCC 55730 and characterization of the resulting daughter strain, L. reuteri DSM 17938. Appl. Environ. Microbiol. 74, 6032–6040. doi: 10.1128/AEM.00991-08
Rychen, G., Aquilina, G., Azimonti, G., Bampidis, V., de Bastos, M. L., Bories, G., et al. (2018). Guidance on the characterisation of microorganisms used as feed additives or as production organisms. EFSA J. 16, 1–24. doi: 10.2903/j.efsa.2018.5206
Sahoo, K. C., Tamhankar, A. J., Johansson, E., and Lundborg, C. S. (2010). Antibiotic use, resistance development and environmental factors: a qualitative study among healthcare professionals in Orissa, India. BMC Public Health 10:629. doi: 10.1186/1471-2458-10-629
Schwab, C., Tveit, A. T., Schleper, C., and Urich, T. (2014). Gene expression of lactobacilli in murine forestomach biofilms. Microb. Biotechnol. 7, 347–359. doi: 10.1111/1751-7915.12126
Seemann, T. (2014). Prokka: rapid prokaryotic genome annotation. Bioinformatics 30, 2068–2069. doi: 10.1093/bioinformatics/btu153
Shen, W., Le, S., Li, Y., and Hu, F. (2016). SeqKit: a cross-platform and ultrafast toolkit for FASTA/Q file manipulation. PLoS One 11:e0163962. doi: 10.1371/journal.pone.0163962
Simão, F. A., Waterhouse, R. M., Ioannidis, P., Kriventseva, E. V., and Zdobnov, E. M. (2015). BUSCO: assessing genome assembly and annotation completeness with single-copy orthologs. Bioinformatics 31, 3210–3212. doi: 10.1093/bioinformatics/btv351
Spinler, J. K., Sontakke, A., Hollister, E. B., Venable, S. F., Oh, P. L., Balderas, M. A., et al. (2014). From prediction to function using evolutionary genomics: human-specific ecotypes of Lactobacillus reuteri have diverse probiotic functions. Genome Biol. Evol. 6, 1772–1789. doi: 10.1093/gbe/evu137
Stephenson, D. P., Moore, R. J., and Allison, G. E. (2009). Comparison and utilization of repetitive-element PCR techniques for typing Lactobacillus isolates from the chicken gastrointestinal tract. Appl. Environ. Microbiol. 75, 6764–6776. doi: 10.1128/AEM.01150-09
Stephenson, D. P., Moore, R. J., and Allison, G. E. (2010). Lactobacillus strain ecology and persistence within broiler chickens fed different diets: identification of persistent strains. Appl. Environ. Microbiol. 76, 6494–6503. doi: 10.1128/AEM.01137-10
Stevens, M., Vollenweider, S., and Lacroix, C. (2010). The potential of reuterin produced by Lactobacillus reuteri as a broad spectrum preservative in food. Prot. Cult. Antimicrob. Metab. 2011, 129–160. doi: 10.1016/B978-1-84569-669-6.50005-4
Stevens, M. J. A., Vollenweider, S., Mertes, P., and Lacroix, C. (2013). Bisulfite as scavenger for enhanced biotechnological production of 3-hydroxypropionaldehyde by Lactobacillus reuteri. Biochem. Eng. J. 79, 239–245. doi: 10.1016/j.bej.2013.08.002
Stolz, P., Vogel, R. F., and Hammes, W. P. (1995). Utilization of electron acceptors by lactobacilli isolated from sourdough - II. Lactobacillus pontis, L. reuteri, L. amylovorus, and L. fermentum. Z. Lebensm. Unters. Forsch. 201, 402–410. doi: 10.1007/BF01192742
Teixeira, J. S., Seeras, A., Sanchez-Maldonado, A. F., Zhang, C., Su, M. S. W., and Gänzle, M. G. (2014). Glutamine, glutamate, and arginine-based acid resistance in Lactobacillus reuteri. Food Microbiol. 42, 172–180. doi: 10.1016/j.fm.2014.03.015
Ventura, M., and Zink, R. (2002). Speciesc identification and molecular typing analysis of Lactobacillus johnsonii by using PCR-based methods and pulsed-field gel electrophoresis. FEMS Microbiol. Lett. 217, 141–154. doi: 10.1111/j.1574-6968.2002.tb11468.x
Versalovic, J., Koeuth, T., and Lupski, J. R. (1991). Distribution of repetitive DNA sequences in eubacteria and application to fingerprinting of bacterial genomes. Nucleic Acids Res. 19, 6823–6831. doi: 10.1093/nar/19.24.6823
Walter, J., Britton, R. A., and Roos, S. (2011). Host-microbial symbiosis in the vertebrate gastrointestinal tract and the Lactobacillus reuteri paradigm. Proc. Natl. Acad. Sci. U.S.A. 108(Suppl.), 4645–4652. doi: 10.1073/pnas.1000099107
Wang, L., Fang, M., Hu, Y., Yang, Y., Yang, M., and Chen, Y. (2014). Characterization of the most abundant Lactobacillus species in chicken gastrointestinal tract and potential use as probiotics for genetic engineering. Acta Biochim. Biophys. Sin. 46, 612–619. doi: 10.1093/abbs/gmu037
Wegmann, U., MacKenzie, D. A., Zheng, J., Goesmann, A., Roos, S., Swarbreck, D., et al. (2015). The pan-genome of Lactobacillus reuteri strains originating from the pig gastrointestinal tract. BMC Genomics 16:1023. doi: 10.1186/s12864-015-2216-7
World Health Organisation [WHO] (2019). Critically Important Antimicrobials For Human Medicine. Geneva: WHO.
World Organisation for Animal Health [OIE] (2015). List of Antimicrobial Agents Of Veterinary Importance. Paris: World Organisation for Animal Health.
Yu, J., Zhao, J., Song, Y., Zhang, J., Yu, Z., and Zhang, H. (2018). Comparative genomics of the herbivore gut symbiont Lactobacillus reuteri reveals genetic diversity and lifestyle adaptation. Science 9, 1–12. doi: 10.3389/fmicb.2018.01151
Zankari, E., Hasman, H., Cosentino, S., Vestergaard, M., Rasmussen, S., Lund, O., et al. (2012). Identification of acquired antimicrobial resistance genes. J. Antimicrob. Chemother. 67, 2640–2644. doi: 10.1093/jac/dks261
Keywords: reuterin, antimicrobial, chicken, Lactobacillus reuteri, comparative genomics
Citation: Greppi A, Asare PT, Schwab C, Zemp N, Stephan R and Lacroix C (2020) Isolation and Comparative Genomic Analysis of Reuterin-Producing Lactobacillus reuteri From the Chicken Gastrointestinal Tract. Front. Microbiol. 11:1166. doi: 10.3389/fmicb.2020.01166
Received: 24 October 2019; Accepted: 07 May 2020;
Published: 04 June 2020.
Edited by:
John R. Battista, Louisiana State University, United StatesReviewed by:
Monique Zagorec, INRA Centre Angers-Nantes Pays de la Loire, FranceXiaodong Xia, Northwest A&F University, China
Jens Walter, University of Nebraska-Lincoln, United States
Copyright © 2020 Greppi, Asare, Schwab, Zemp, Stephan and Lacroix. This is an open-access article distributed under the terms of the Creative Commons Attribution License (CC BY). The use, distribution or reproduction in other forums is permitted, provided the original author(s) and the copyright owner(s) are credited and that the original publication in this journal is cited, in accordance with accepted academic practice. No use, distribution or reproduction is permitted which does not comply with these terms.
*Correspondence: Christophe Lacroix, Y2hyaXN0b3BoZS5sYWNyb2l4QGhlc3QuZXRoei5jaA==
†These authors have contributed equally to this work