- 1Department of Microbiology, Immunobiology and Genetics, Max Perutz Labs, Vienna BioCenter (VBC), University of Vienna, Vienna, Austria
- 2Department of Theoretical Chemistry, Faculty of Chemistry, University of Vienna, Vienna, Austria
- 3Research Group Bioinformatics and Computational Biology, Faculty of Computer Science, University of Vienna, Vienna, Austria
Carbapenems are often the antibiotics of choice to combat life threatening infections caused by the opportunistic human pathogen Pseudomonas aeruginosa. The outer membrane porins OprD and OpdP serve as entry ports for carbapenems. Here, we report that the RNA chaperone Hfq governs post-transcriptional regulation of the oprD and opdP genes in a distinctive manner. Hfq together with the recently described small regulatory RNAs (sRNAs) ErsA and Sr0161 is shown to mediate translational repression of oprD, whereas opdP appears not to be regulated by sRNAs. At variance, our data indicate that opdP is translationally repressed by a regulatory complex consisting of Hfq and the catabolite repression protein Crc, an assembly known to be key to carbon catabolite repression in P. aeruginosa. The regulatory RNA CrcZ, which is up-regulated during growth of P. aeruginosa on less preferred carbon sources, is known to sequester Hfq, which relieves Hfq-mediated translational repression of genes. The differential carbapenem susceptibility during growth on different carbon sources can thus be understood in light of Hfq-dependent oprD/opdP regulation and of the antagonizing function of the CrcZ RNA on Hfq regulatory complexes.
Introduction
The opportunistic human pathogen Pseudomonas aeruginosa can cause severe infections. It is particularly devastating for immunocompromised individuals and patients with cystic fibrosis, leading to high morbidity and mortality. As they are less prone to degradation by extended spectrum β-lactamases, carbapenems are frequently used to treat severe infections of Gram-negative bacteria including P. aeruginosa (Papp-Wallace et al., 2011; Fritzenwanker et al., 2018). Carbapenem-resistant P. aeruginosa strains are increasingly occurring (McDougall et al., 2013; Castanheira et al., 2014; Buehrle et al., 2017), which prompted the World Health Organization to rank carbapenem-resistant P. aeruginosa among the priority pathogens to investigate new drug treatments (Tacconelli et al., 2018). P. aeruginosa exhibits several resistance mechanisms toward carbapenems including the production of metallo-β-lactamases and carbapenemase (Sacha et al., 2008; Bassetti et al., 2018) as well as dedicated efflux systems (Chalhoub et al., 2016). Another notable feature of P. aeruginosa’s high intrinsic antibiotic resistance is the low outer membrane permeability (Hancock and Woodruff, 1988; Breidenstein et al., 2011). P. aeruginosa utilizes a variety of specialized outer membrane porins (Hancock and Brinkman, 2002; Eren et al., 2012). Two of them, OprD/OccD1 and OpdP/OccD3, show high sequence similarity (51%) and serve as entry ports for basic amino acids and small peptides as well as for certain carbapenems, e.g., imipenem and meropenem (Quinn et al., 1986; Trias et al., 1989; Tamber and Hancock, 2006; Papp-Wallace et al., 2011; Isabella et al., 2015). In fact, the first documented case of clinical resistance to carbapenems was found to be due to a loss of the monocistronic oprD gene (Quinn et al., 1986). The deletion of oprD resulted in a decreased susceptibility to carbapenems, but deletion of the opdP gene alone, residing in an operon together with genes encoding a dipeptide ABC transport system (Chevalier et al., 2017), did not cause a significant change. However, the deletion of both genes led to a remarkable increase in resistance when compared to the deletion of oprD alone (Isabella et al., 2015).
The RNA chaperone Hfq is a pleiotropic regulator and virulence factor in P. aeruginosa (Sonnleitner et al., 2003, 2006, 2018). Hfq is involved in the control of mRNA translation through distinct mechanisms. In riboregulation, Hfq can act indirectly by facilitating base-pairing interactions of small regulatory RNAs (sRNAs) with cognate mRNA targets (Vogel and Luisi, 2011; Kavita et al., 2018). On the other hand, Hfq can directly repress translation, by binding to A-rich sequences at or in the vicinity of translation initiation sites (Sonnleitner and Bläsi, 2014). Hfq has several distinct RNA binding sites. Crystallographic and biophysical data showed that RNA recognition is mediated by distinct interactions with distal, proximal, and rim faces of the hexameric ring (Schumacher et al., 2002; Link et al., 2009; Sauer et al., 2012; Panja et al., 2013). Many sRNAs bind to the proximal side of Hfq via U-rich stretches (Schumacher et al., 2002; Mikulecky et al., 2004; Link et al., 2009) or through the poly-uridine tails derived from rho-independent terminators (Otaka et al., 2011; Sauer and Weichenrieder, 2011; Ishikawa et al., 2012). Internal U/A-rich regions in sRNAs as well as in mRNAs were found to interact with arginine patches on the lateral rim of the Hfq-hexamer (Sauer et al., 2012; Peng et al., 2014; Schu et al., 2015). The distal side recognizes A-rich regions, previously defined as ARN repeats, where A is an adenine, R is any purine nucleotide and N is any nucleotide, which are often present in mRNAs around the ribosome binding site (Link et al., 2009; Murina et al., 2013; Robinson et al., 2014; Sonnleitner and Bläsi, 2014).
A GRIL-seq approach identified two base-pairing small regulatory sRNAs, Sr0161, and EsrA, as negative translational regulators of oprD (Zhang et al., 2017). ErsA is transcriptionally controlled by the envelope stress response regulator AlgU/T (Ferrara et al., 2015), and its expression is further up-regulated after a shift from high to low oxygen supply, and upon entry into stationary phase (Ferrara et al., 2015; Zhang et al., 2017). Sr0161 did not show any phase dependent expression in full broth (Zhang et al., 2017). The study by Zhang et al. (2017) also suggested an interaction between Sr0161 and opdP mRNA. RT-qPCR showed that the opdP levels were decreased after overexpression of the sRNA and modestly increased in a sr0161 deletion mutant (Zhang et al., 2017). However, in contrast to Hfq-Sr0161/EsrA-mediated negative translational regulation of oprD, it remains unknown whether Sr0161 directly regulates translation of opdP (Zhang et al., 2017).
In P. aeruginosa, carbon catabolite repression (CCR) operates at the post-transcriptional level. In the presence of preferred C-sources (e.g., succinate), the RNA chaperone Hfq binds to the translation initiation region (TIR) of mRNAs encoding proteins important for the uptake and utilization of less preferred C-sources, and represses their translation (Sonnleitner and Bläsi, 2014). Upon binding, the Hfq/RNA complex forms a “platform” to which the catabolite repression protein Crc can bind, which results in stabilization of the repressive complex (Sonnleitner et al., 2018; Pei et al., 2019). When the preferred C-source is exhausted, the levels of the Hfq-titrating RNA CrcZ increase, which in turn leads to relieve of Hfq/Crc-mediated CCR (Sonnleitner and Bläsi, 2014). Previous studies provided some hints that oprD is regulated by CCR (Ochs et al., 1999; Linares et al., 2010). However, recent omics studies are somewhat inconsistent. A ChIP-seq approach identified oprD mRNA among the mRNAs that were co-immunoprecipitated with antibodies directed against Hfq as well as against Crc (Kambara et al., 2018). Nevertheless, transcriptomic and proteomic analyses designed to reveal the Crc regulon revealed only opdP as a candidate for Crc-mediated post-transcriptional regulation but not oprD (Corona et al., 2018).
Taken these studies together with the observation that a PAO1hfq-deletion mutant was more susceptible to carbapenems when compared with the parental strain (Ducret et al., 2016; Pusic et al., 2018), it appeared safe to assume that Hfq is involved in regulation of both, oprD and opdP. In this study, we addressed the question whether post-transcriptional regulation of oprD and opdP occurs through riboregulation and/or through CCR, i.e., through Hfq/Crc repressive complexes. To dissect the regulatory mechanism(s) for oprD and opdP, we used a genetic approach employing translational reporter constructs in conjunction with different P. aeruginosa O1 mutant strains. These studies verify that oprD is regulated by Hfq-mediated riboregulation and strongly indicate that opdP is directly translationally repressed by Hfq/Crc.
Materials and Methods
Bacterial Strains, Plasmids, and Growth Conditions
Strains and plasmids used in this study are listed in Supplementary Table S1. If not indicated otherwise, the cultures were grown at 37°C in either Lysogeny-broth (LB) (Sambrook et al., 1989) or basal-salt medium (BSM) supplemented with the indicated carbon sources (Sonnleitner et al., 2009). When required, the following concentrations of antibiotics were used: 15 μg/ml gentamicin, 25 μg/ml tetracycline, and 100 μg/ml ampicillin for Escherichia coli; 50 μg/ml gentamicin, 100 μg/ml of tetracycline, and 250 μg/ml carbenicillin for P. aeruginosa.
Construction of PAO1 Deletion Strains
The deletion of the first 82 nucleotides (nt) of ersA (coordinates 6183580-6183661 of the PAO1 genome; Winsor et al., 2016) and the deletion of the sr0161 3′ end including additional 24 nt downstream of sr0161 (coordinates 184302–184482 of the PAO1 genome; Winsor et al., 2016) was created by homologous recombination, respectively (Zhang et al., 2017). Briefly, plasmids pEXG2-sr0161 (for generation of PAO1Δsr0161 and PAO1ΔhfqΔsr0161) and pEXG2-ersA (for generation of PAO1ΔersAΔsr0161 and PAO1ΔhfqΔersAΔsr0161) were mobilized into the strains PAO1, PAO1Δhfq, PAO1Δsr0161, and PAO1ΔhfqΔsr0161 with the aid of E. coli strain S17-1, and then chromosomally integrated through selection for gentamicin. Excision of the vector by a second crossover event was achieved by selection of sucrose insensitive cells as the pEXG2 vector encodes the Bacillus subtilis sacB gene, whose gene product – levan sucrase – renders Gram-negative cells sensitive to sucrose (Rietsch et al., 2005; Hmelo et al., 2015).
Construction of Plasmid pME6015Ptac
For construction of a cloning vector suited for the generation of translational lacZ fusions transcribed under the control of the Ptac promoter, a 1,522-base pair (bp) fragment encoding the LacIq repressor gene and the Ptac promotor together with the operator sites located downstream of the transcriptional start site were amplified by PCR using the oligonucleotides L85 (5′-GAT ATC GAA TTC GAA CGC CAG CAA GAC-3′) and M85 (5′-TTT TTG GAT CC A ATT GTT ATC CGC TCA CAA TTC C-3′) and plasmid pME6032 as template. The PCR fragment was cleaved with EcoRI and BamHI, and then ligated into the corresponding sites of plasmid pME6015.
Construction of Plasmids pME6015PtacoprD::lacZ and pME6015PtacopdP::lacZ
To construct translational gene fusions between oprD and opdP, respectively, and lacZ under transcriptional control of the Ptac promoter, a 71-bp fragment of the TIR of oprD (coordinates 1045365–1045294 of the PAO1 genome; Winsor et al., 2016) and a 118-bp fragment of the TIR of opdP (coordinates 5038800–5038918 of the PAO1 genome; Winsor et al., 2016) were amplified by PCR using the oligonucleotide pairs A123 (5′-ACG TGG ATC C AC AAG AAG AAC TAG CCG TCA C-3′)/H112 (5′-ACG TCT GCA G GC TCC ACT TCA TCA CTT TCA TTG-3′) for oprD and Q144 (5′-ACG TGG ATC CTC GCC GCG CCG TCT TCG-3′)/O144 (5′-ACG TCT GCA G AG CGT TCC TGG CGG AAC-3′) for opdP, respectively, and chromosomal DNA of PAO1 as template. The PCR fragments were cleaved with BamHI and PstI, and then ligated into the corresponding sites of plasmid pME6015Ptac. Despite the presence of LacIq, the Ptac promoter was leaky in the pME6015Ptac derivatives (not shown). Thus, IPTG was not added in the experiments described below.
Co-immunoprecipitation of mRNAs Bound to Hfq
A total of 40 ml of PAO1 culture was grown in BSM complex medium (Sonnleitner et al., 2018) and harvested at an OD600 of 1.5. The cells were first washed in lysis buffer (20 mM Tris pH 8.0, 150 mM KCl, 1 mM MgCl2, 1 mM DTT, and 0.05% Triton X-100) and then snap frozen in liquid nitrogen. The cells were lysed by sonication (six times for 10 sec on ice) in 800 μl lysis buffer in the presence of 200 U RiboLock® RNase inhibitor (Fermentas). Cell debris were removed by centrifugation and anti-Hfq antibodies (Pineda) were added to 60 μl supernatant and incubated for 2 h at 4°C on a rotating wheel. Then, 5 μl Dynabeads® Protein G beads (Novex) were added, and the incubation was continued for 1 h. The beads were washed three times with lysis buffer and finally collected in 200 μl of lysis buffer without Triton X-100. Then, 100 μl phenol was added and the beads were shaken at 900 rpm for 30 min at room temperature. The RNA was purified by phenol-chloroform extraction, which was followed by ethanol precipitation. Libraries were constructed using NEBNext® UltraTM Directional RNA Library Prep Kit from Illumina. A total of 100 bp single end sequence reads were generated using the Illumina HiSeq 2000 platform at the Vienna BioCenter Core Facility1. Sequencing adapter removal was performed with cutadapt (Martin, 2011). Mapping of oprD and opdP RNA against the PAO1 reference genome (NCBI accession number NC_002516.2) was performed with Segemehl (Hoffmann et al., 2009) with default parameters. The mapped sequencing data were prepared for visualization using the ViennaNGS tool box, and visualized with the UCSC Genome Browser (Wolfinger et al., 2015). The raw sequencing data were deposited in the European nucleotide archive (ENA) as a study under the accession number PRJEB37368.
Microscale Thermophoresis
For in vitro transcription of the 100 nt long oprD RNA fragment [nt −61 to nt +39 with regard to the A (+1) of the AUG start codon] and the 150 nt long opdP RNA fragment [nt −111 to nt +39, with regard to the G (+1) of the GUG start codon], the AmpliScribe T7-Flash Transcription Kit (Epicentre Biotechnologies) was used according to the manufacturer’s instructions. First, PCR fragments were generated with the primer pairs Y133 (5′-TCT AGA CGT AAT ACG ACT CAC TAT AGG CTA GCC GTC ACT GCG GCA C-3′)/Z133 (5′-AAC CGC CAG TGC AAT GGC-3′) (oprD) and B148 (5′-TCT AGA CGT AAT ACG ACT CAC TAT AGG CCA GGA GCG CTC GCC TC-3′)/C148 (5′-TCC CAG CGT CGC GCC GGT C-3′) (opdP), and P. aeruginosa chromosomal DNA. The forward primers contained a T7 promoter sequence (underlined).
For microscale thermophoresis (MST) (Wienken et al., 2010), 20 μM of Hfq protein was labeled with Monolith NTTM Protein Labelling Kit RED-NHS according to the manufacturer’s instructions (Nanotemper Technologies). For determination of protein-RNA interactions, 56 nM labeled Hfq was used in the presence of increasing concentrations of non-labeled in vitro transcribed RNA (either oprD–61 –+ 39 or opdP–111 –+ 39). The ligands were dissolved in MST-buffer (50 mM Tris pH 7.4, 150 mM NaCl, and 10 mM MgCl2) in the presence of 0.1% Tween-20 and 0.1% BSA. After 30 min incubation at 37°C, the samples were loaded onto NT.115 hydrophilic capillaries (Nanotemper Technologies) and measured in an MST Monolith NT.115 Green/Red instrument at the Vienna BioCenter Core Facility2. The MST measurements were performed in duplicate. The data for MST analysis were recorded at 25°C using the red LED (excitation: 625 nm and emission: 680 nm); MST Power 40%, LED Power 50%. Data analysis was performed with NTAffinityAnalysis v2.0.2 for thermophoresis and T-jump analysis 0 and 5 s after the pulse. For determination of the Kd-values the Hill-fit model within the NTAffinityAnalysis software was used.
Agar Disk Diffusion Assay
PAO1 and mutants thereof were grown in BSM supplemented with either 40 mM succinate or mannitol to an OD600 of 1.8–2.0. Then, 200 μl of cultures were plated on agar plates containing the respective media and filter disks loaded with 10 μg imipenem were applied on top (Oxoid). The plates were incubated at 37°C. The diameter of the growth inhibition zones was measured in mm.
β-Galactosidase Assays
The β-galactosidase activities were determined as described (Miller, 1972). The cells were permeabilized with 5% toluene. Unless indicated otherwise, the β-galactosidase units in the different experiments were derived from at least two independent experiments, and are shown as mean. The error bars in the different figures represent standard deviations. Except for the data in Figure 3, statistical analyses were performed in Excel with a two tailed distributed Student’s T-test of two sample arrays with unequal variance, ns (non-significant); P > 0.05, ∗P ≤ 0.05, ∗∗P ≤ 0.01, and ∗∗∗P ≤ 0.001. Due to the multiple comparison in Figure 3, the results were statistically analyzed by an ANOVA test with post hoc multiple comparison. In short, Levene’s test was used to test for equality (homogeneity) of variances between the tested groups. Statistical significance was determined by one-way ANOVA with the Tukey’s post hoc test when more than two groups with normal distribution were compared (Figure 3A). When more than two groups with unequal variance were compared, the Brown-Forsythe and Welch’s ANOVA test with the Dunnett’s T3 post hoc test was used (Figures 3B–D), ns (non-significant); ∗P < 0.05, ∗∗P < 0.01, and ∗∗∗P < 0.001 were considered statistically significant results.
Western-Blot Analyses
Equal amounts of total proteins were separated on a 12% SDS-polyacrylamide gel, and then electro-blotted onto a nitrocellulose membrane. The blots were blocked with 5% dry milk in TBS buffer, and probed with rabbit anti-Hfq, rabbit anti-Crc or rabbit anti-E. coli-S1 antibodies (Sonnleitner and Bläsi, 2014). Immunodetection of ribosomal protein S1 served as a loading control. The antibody–antigen complexes were visualized with horseradish peroxidase (HRP) conjugated anti-rabbit antibodies (Cell Signaling Technology) using the SuperSignalTM West Pico PLUS chemiluminescent substrate kit (Thermo Scientific). The signals were detected with ChemiDocTM Touch Imaging System (BioRad) and analyzed with ImageLab 5.2.1 (BioRad).
Results
Hfq Is Involved in Translational Regulation of oprD and opdP
Recent studies revealed that P. aeruginosa hfq deletion mutants showed an increased susceptibility to imipenem (Ducret et al., 2016; Pusic et al., 2018), which coincided with higher oprD and opdP transcript levels in the hfq mutant strain when compared with the parental strain during growth in different media (Supplementary Figure S1 and Supplementary Table S2) (Ducret et al., 2016; Pusic et al., 2018). To verify the impact of Hfq on oprD and opdP translation, oprD::lacZ and opdP::lacZ translational gene fusions were constructed and the β-galactosidase activities conferred by the respective fusion proteins were determined in strains PAO1 and PAO1Δhfq harboring plasmids pME6015PtacoprD::lacZ and pME6015PtacopdP::lacZ, respectively. Transcription of either chimeric gene was driven by the Ptac promoter to control for potential transcriptional effects of Hfq (Ducret et al., 2016). The strains were grown in LB medium to an OD600 of 2.0, as the sRNAs ErsA and Sr0161 are transcribed under these conditions (Zhang et al., 2017). As reported by Zhang et al. (2017), our RNAseq analysis confirmed growth independent quantities of Sr0161 RNA in LB medium and increased levels of ErsA RNA in stationary phase when compared to logarithmically growing cells (Supplementary Figure S2). Translation of both reporter fusions was repressed in the presence of Hfq. However, the effect of Hfq on oprD::lacZ translation was less pronounced (Figure 1A; 1.5-fold difference between PAO1 and PAO1Δhfq harboring pME1615PtacoprD::lacZ) than on opdP::lacZ translation (Figure 1B; 10-fold difference between PAO1 and PAO1Δhfq harboring pME1615PtacopdP::lacZ). The differential translation rates observed for oprD::lacZ and opdP::lacZ in the wild-type strain and the corresponding hfq deletion strains coincided with a ∼2–3-fold and ≥10-fold higher transcript abundance of oprD and opdP, respectively, in PAO1 and PAO1hfq during cultivation in different media (Supplementary Table S2). As anticipated, the complementation of hfq by ectopic expression of a plasmid borne hfqFlag allele in strain PAO1Δhfq resulted again in repression of the oprD::lacZ and opdP::lacZ reporter genes (Figures 1C,D), respectively.
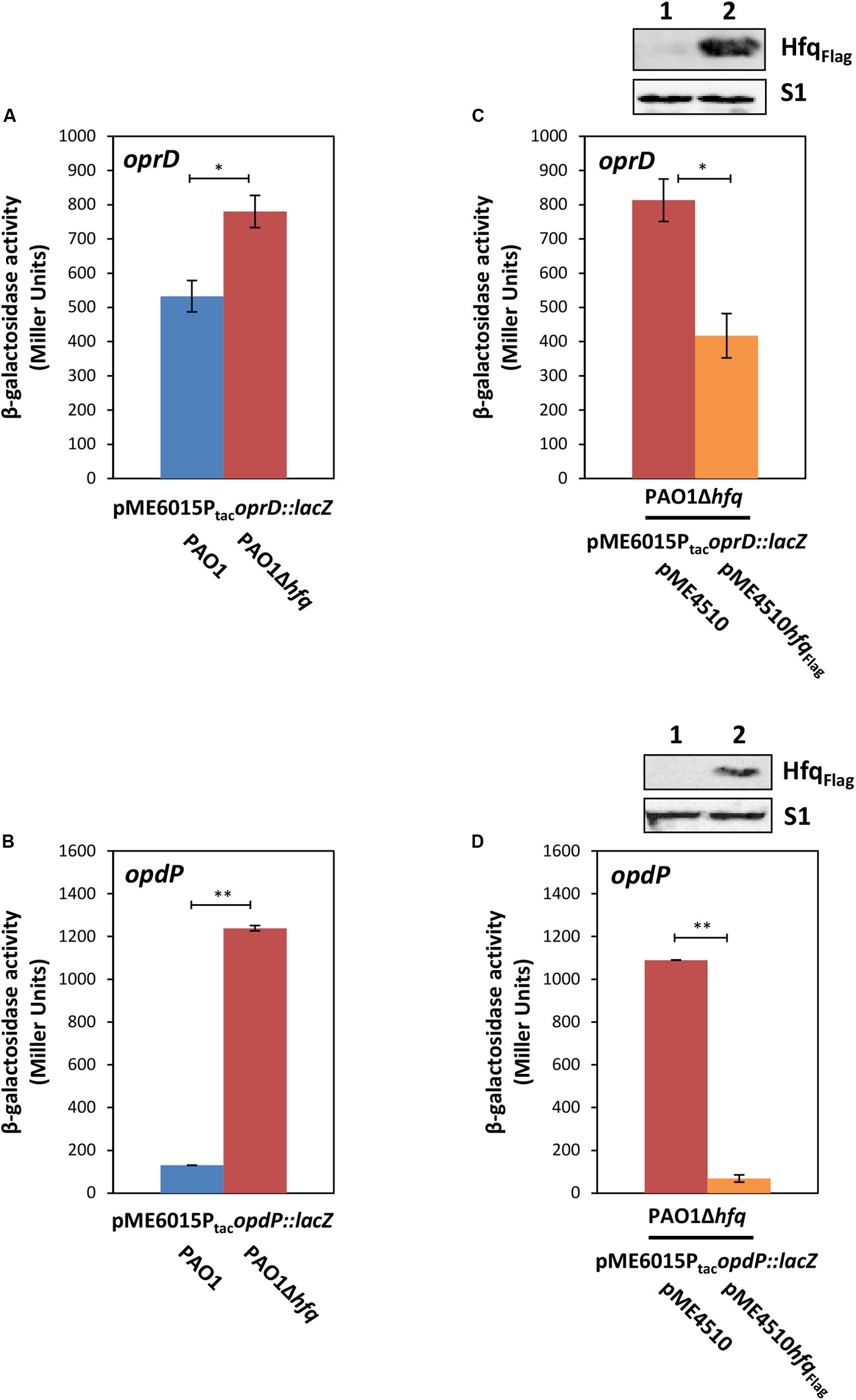
Figure 1. Hfq negatively regulates oprD and opdP translation. (A,B) The strains PAO1 (blue bar) and PAO1Δhfq (red bar) harboring either plasmid pME6015PtacoprD::lacZ (A) or pME6015PtacopdP::lacZ (B) were grown in LB medium. Samples were withdrawn at an OD600 of 2.0. The bars represent the β-galactosidase values conferred by the oprD::lacZ translational fusion gene encoded by plasmid pME6015PtacoprD::lacZ (A) and by the opdP::lacZ translational fusion gene encoded by plasmid pME6015PtacopdP::lacZ (B), respectively. The error bars represent standard deviations from two independent experiments. The strains PAO1Δhfq(pME4510) (red bar) and PAO1Δhfq(pME4510hfqFlag) (orange bar) harboring either plasmid pME6015PtacoprD::lacZ (C) or pME6015Ptac opdP::lacZ (D) were grown in LB medium. Samples were withdrawn at an OD600 of 2.0. The bars represent the β-galactosidase values conferred by the oprD::lacZ translational fusion gene (C) and by the opdP::lacZ translational fusion gene (D), respectively, in the presence or absence of ectopic hfqFlag expression. The error bars represent standard deviations from two independent experiments. Statistical analyses were performed in Excel with a two tailed distributed Student’s T-test of two sample arrays with unequal variance. ns P > 0.05, *P ≤ 0.05, and **P ≤ 0.01. (C,D: top panels), Hfq and S1 levels in strains PAO1Δhfq(pME4510) (lane 1) and PAO1Δhfq(pME4510hfqFlag) (lane 2) harboring either plasmid pME6015PtacoprD::lacZ (C) or pME6015PtacopdP::lacZ (D). The Hfq levels were determined by Western-blot analyses using anti-Hfq antibodies. Immunodetection of ribosomal protein S1 served as a loading control.
As the mRNA sequences of the TIRs of the oprD and opdP genes in the clinical isolate PA14 showed only minor differences to PAO1 (Winsor et al., 2016), the same experiments were performed with the clinical isolate PA14 and the corresponding PA14Δhfq mutant harboring plasmids pME1615PtacoprD::lacZ and pME1615PtacopdP::lacZ, respectively, after growth in synthetic cystic fibrosis medium (SCFM) containing 100 μM FeSO4 (Palmer et al., 2007; Tata et al., 2016). The outcome of these experiments was comparable with the results obtained for PAO1 after growth in LB medium (Supplementary Figures S3A–D).
Binding of Hfq to the Translation Initiation Regions of oprD and opdP
To test whether Hfq binds to the TIRs of oprD and opdP, a co-immunoprecipitation assay with Hfq specific antibodies was performed to identify protein bound RNA fragments. Subsequent RNA-seq revealed a distinct sub-sequence upstream of the oprD start codon (Figure 2A). This region contains several ARN motifs, which have been shown to bind to the distal side of Hfq (Link et al., 2009). The sub-sequence also comprises the ErsA and Sr0161 interaction sites (Figure 2A) (Zhang et al., 2017).
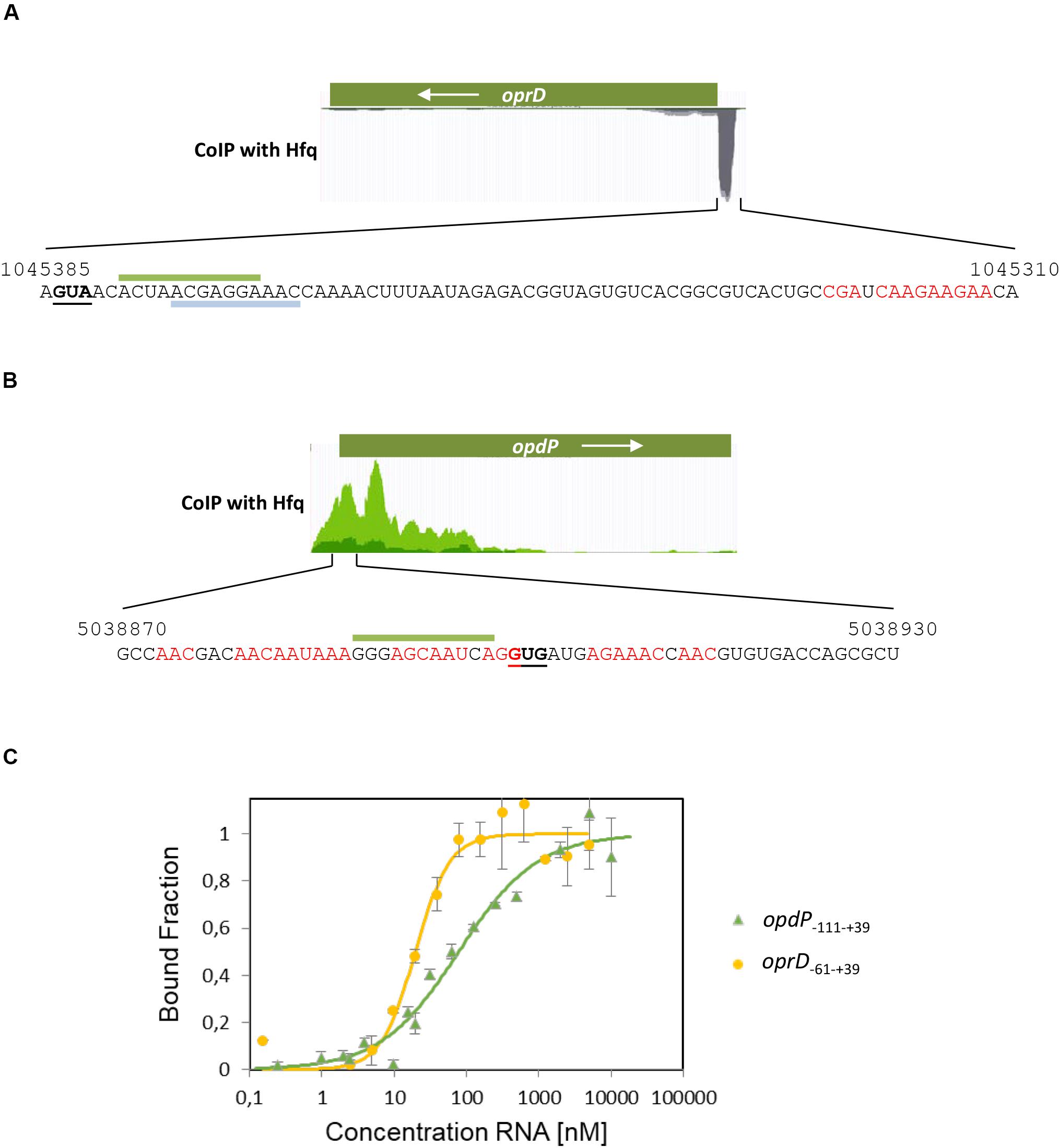
Figure 2. Co-immunoprecipitation of oprD and opdP mRNA fragments with Hfq. Hfq-bound RNAs were isolated from lysates of the PAO1 culture after co-immunoprecipitation with Hfq-specific antibodies as described in section “Materials and Methods.” The identity of Hfq-bound RNAs in the supernatant was revealed by RNA-seq. (A,B) The read coverage visualized in the Genome Browser is shown for oprD (A) and opdP (B), respectively. The sequences of the co-immunoprecipitated mRNA fragments corresponding to the TIR of oprD (A) and opdP (B), respectively, are shown below. The numbers refer to the PAO1 genome coordinates (http://www.pseudomonas.com) (Winsor et al., 2016). The start codons of oprD and opdP are underlined. Putative Hfq binding sites are indicated in red. The interacting sequences for EsrA and Sr0161 in the oprD TIR (A) (Zhang et al., 2017) are indicated by gray and green bars, respectively. The putative interacting sequence for Sr0161 in the opdP TIR (B) is indicated by a green bar (B) (Zhang et al., 2017). (C) Microscale thermophoresis reveals the Kd of Hfq for oprD–61 –+ 39 RNA (yellow circles) and opdP–111 –+ 39 RNA (green triangles) with 19.7 ± 2.8 nM and 78.1 ± 1.97 nM, respectively. Increasing amounts of the non-labeled in vitro transcribed oprD–61 –+ 39 and opdP–111 –+ 39 fragments were added to 56 nM fluorescently labeled Hfq protein. The dissociation constant (Kd) of oprD–61 –+ 39 and opdP–111 –+ 39 was determined as described in section “Materials and Methods,” and was expressed as mean EC50 ± EC50 confidence interval of two independent experiments. Thermophoresis/T-jump analysis is shown. LED power of 50% and MST power of 40% were used.
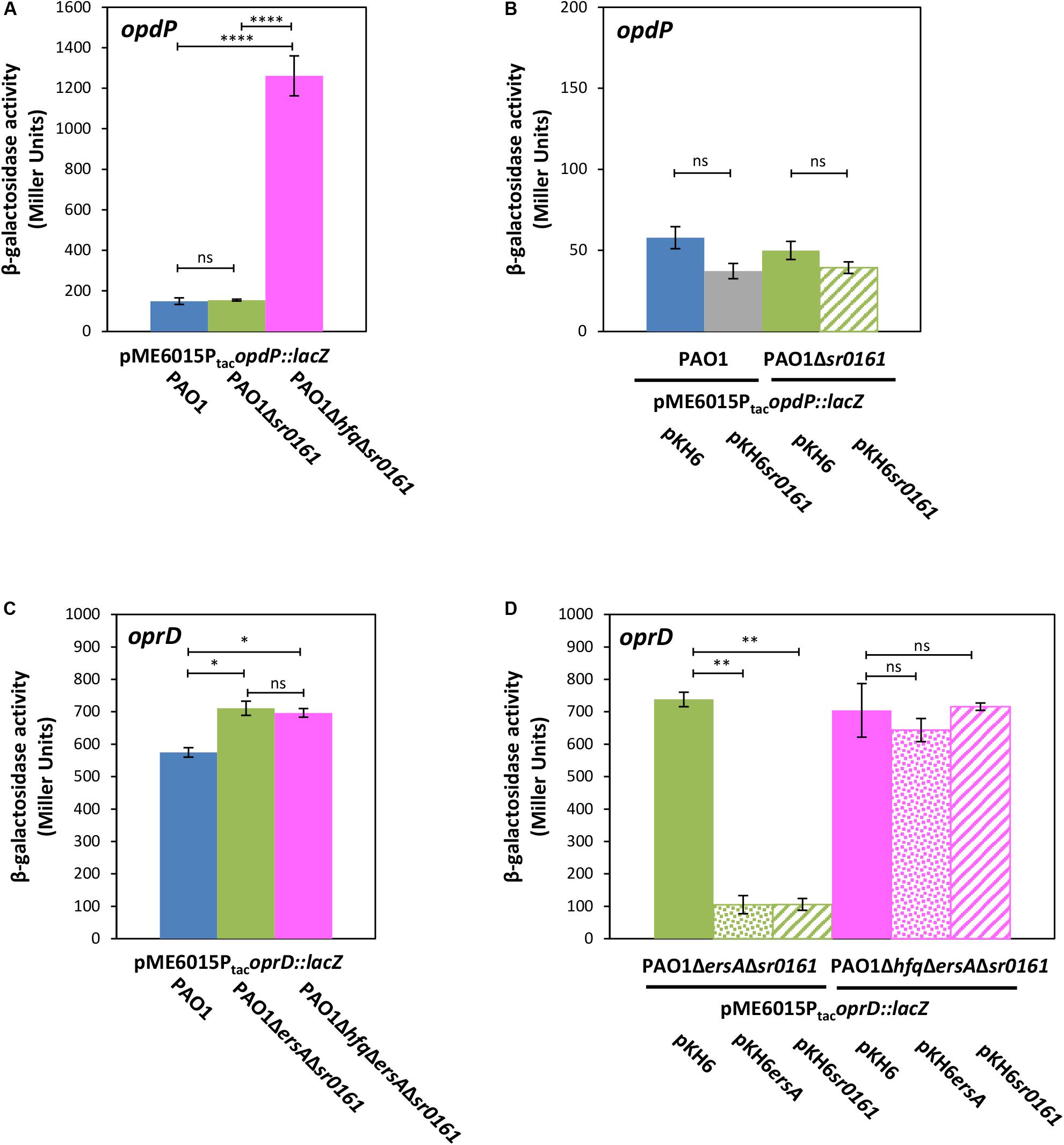
Figure 3. The sRNAs Sr0161 does not regulate opdP translation. (A) The strains PAO1 (blue bar), PAO1Δsr0161 (green bar), and PAO1ΔhfqΔsr0161 (pink bar) harboring plasmid pME6015PtacopdP::lacZ were grown in LB medium. Samples were withdrawn at an OD600 of 2.0. The bars represent the β-galactosidase values conferred by the opdP::lacZ translational fusion gene encoded by plasmid pME6015PtacopdP::lacZ. (B) The strains PAO1 (pKH6) (blue bar), PAO1(pKH6sr0161) (gray bar), PAO1Δsr0161(pKH6) (green bar), and PAO1Δsr0161(pKH6sr0161) (striped green bar) harboring plasmid pME6015PtacopdP::lacZ were grown in LB medium supplemented with 0.2% arabinose to induce sRNA gene expression from plasmid pKH6sr0161. Samples were withdrawn at an OD600 of 2.0. The bars represent the β-galactosidase values conferred by the opdP::lacZ translational gene fusion encoded by plasmid pME6015PtacopdP::lacZ. (C) The strains PAO1 (blue bar), PAO1ΔersAΔsr0161 (green bar) and PAO1ΔhfqΔersAΔsr0161 (pink bar) harboring plasmid pME6015PtacoprD::lacZ were grown in LB medium. Samples were withdrawn at an OD600 of 2.0. The bars represent the β-galactosidase values conferred by the oprD::lacZ translational fusion gene encoded by plasmid pME6015PtacoprD::lacZ. (D) Ectopic expression of ersA and sr0161 in strains PAO1ΔersAΔsr0161 (green bars) and PAO1ΔhfqΔersAΔsr0161 (pink bars) harboring plasmid pME6015PtacoprD::lacZ. The β-galactosidase values conferred by the oprD::lacZ translational fusion gene in either strain in the absence of ersA/sr0161 expression (solid bars) and in the presence of ersA (dotted bars) or sr0161 (striped bars) are indicated by bars. Statistical analyses were performed with AGOVA test with post hoc multiple comparison as described in section “Materials and Methods.” ns (non-significant); *P < 0.05, **P < 0.01, ***P < 0.001, ****P < 0.0001.
The co-immunoprecipitated RNA sub-sequence including the start codon of opdP comprised as well several ARN-triplets and overlaps with the predicted Sr0161 binding-site (Figure 2B) (Zhang et al., 2017).
The binding affinity of Hfq for the respective co-immunoprecipitated oprD and opdP sub-sequences was determined by MST. Hfq exerted a high affinity for the oprD–61 –+ 39 sub-sequence (Kd = 19.7 ± 2.8 nM) and a somewhat lower affinity for the opdP–111 –+ 39 sub-sequence (Kd = 78.1 ± 1.97 nM) (Figure 2C).
At this junction it seems worth noting that binding of Hfq to the TIRs of either oprD or opdP is anticipated regardless of whether Hfq/sRNA-mediated regulation is the underlying mechanism of translational repression, or whether this occurs through Hfq/Crc repressive complexes.
The sRNA Sr0161 Does Not Impact Translation of opdP
Next, we re-examined whether sRNA Sr0161, which was identified by GRIL-seq and suggested to base-pair with the opdP TIR (Zhang et al., 2017) can translationally regulate opdP. The strains PAO1 and PAO1Δsr0161 harboring plasmid pME6016PtacopdP::lacZ were grown in LB medium to an OD600 of 2.0. The β-galactosidase activities conferred by the translational opdP::lacZ reporter gene were indistinguishable in either strain (Figure 3A). In addition, ectopic expression of Sr0161 in PAO1 and PAO1Δsr0161 harboring plasmid pME6016PtacopdP::lacZ did not result in significant translational repression of the opdP::lacZ reporter gene (Figure 3B), arguing against translational regulation of opdP by Sr0161. However, the β-galactosidase activity conferred by the opdP::lacZ reporter gene encoded by plasmid pME6015PtacopdP::lacZ was approximately 10-times higher in strain PAO1ΔhfqΔsr0161 when compared with strains PAO1 and PAO1Δsr0161 (Figure 3A), suggesting that Hfq regulates opdP either with the aid of an hitherto unknown sRNA or directly by a Hfq/Crc repressive complex.
On the other hand, our studies with the constructed PAO1ΔersAΔsr0161(pME6015PtacoprD::lacZ) double mutant and with the PAO1hfqΔersAΔsr0161(pME6015PtacoprD::lacZ) triple mutant strain showed that the absence of the sRNAs leads to de-repression of oprD::lacZ translation in both strains (Figure 3C), whereas ectopic overexpression of either sRNA restored translational repression of oprD::lacZ in strain PAO1ΔersAΔsr0161(pME6015PtacoprD::lacZ) to the same level but not in strain PAO1ΔhfqΔersAΔsr0161 (pME6015PtacoprD::lacZ) (Figure 3D). Thus, the latter studies corroborate and extend the observations of Zhang et al. (2017) in that (i) EsrA and Sr0161 negatively regulate oprD translation and that (ii) not only Sr0161 operates in a Hfq-dependent manner but also EsrA.
Crc Impacts Hfq-Mediated opdP Regulation but Not on Hfq/EsrA/Sr0161-Mediated Riboregulation of oprD
Previous studies have shown that both, Hfq and Crc, are required for tight translational repression of mRNAs, which are subject to CCR (Sonnleitner and Bläsi, 2014; Moreno et al., 2015). Although the presence of Crc did not significantly enhance the affinity of Hfq for a RNA substrate, the simultaneous interactions of Crc with both binding partners resulted in an Hfq/Crc/RNA assembly with increased stability when compared with the Hfq/RNA complex alone (Sonnleitner et al., 2018). In other words, Crc can be regarded a co-repressor in Hfq/Crc repressive complexes. A recent structural study provided a rationale for the increased stability of the Hfq/Crc/RNA assembly by showing that the Hfq binding site on mRNA is sandwiched between both binding partners (Pei et al., 2019), which in turn can be readily reconciled with an increased translational repression observed for Hfq/Crc regulated genes in the presence of Crc (wild type strain) when compared to an isogenic crc deletion strain (Sonnleitner and Bläsi, 2014). Therefore, the following experiments were performed with the rationale that Crc should impact translation of genes that are assumed to be directly controlled by a Hfq/Crc repressive complex such as opdP rather than genes subject to canonical riboregulation such as oprD. Translation of opdP and oprD was monitored in strains PAO1 and PAO1Δcrc transformed with either plasmid pME6015PtacopdP::lacZ or pME6015PtacoprD::lacZ. As shown in Figure 4A, the β-galactosidase activity conferred by the opdP::lacZ translation was increased in the absence of Crc, i.e., the presence of Crc resulted in a stronger repression of opdP::lacZ. In contrast, the β-galactosidase activity conferred by the oprD::lacZ reporter gene was unaffected by Crc (Figure 4B). In line, ectopic expression of crc-flag resulted in repression of opdP::lacZ in strain PAO1Δcrc(pME6015PtacopdP::lacZ) (Figure 4C), whereas ectopic expression of crc-flag did not significantly change oprD::lacZ translation in strain PAO1Δcrc(pME6015PtacoprD::lacZ) (Figure 4D). These data strongly suggested that Crc contributes to Hfq-mediated direct translational regulation of opdP, i.e., that its translation is controlled by a Hfq/Crc repressive complex. As translational regulation of oprD was rather independent of Crc, these studies further support the notion that translational regulation of oprD is only subject to Hfq/EsrA/Sr0161-mediated riboregulation.
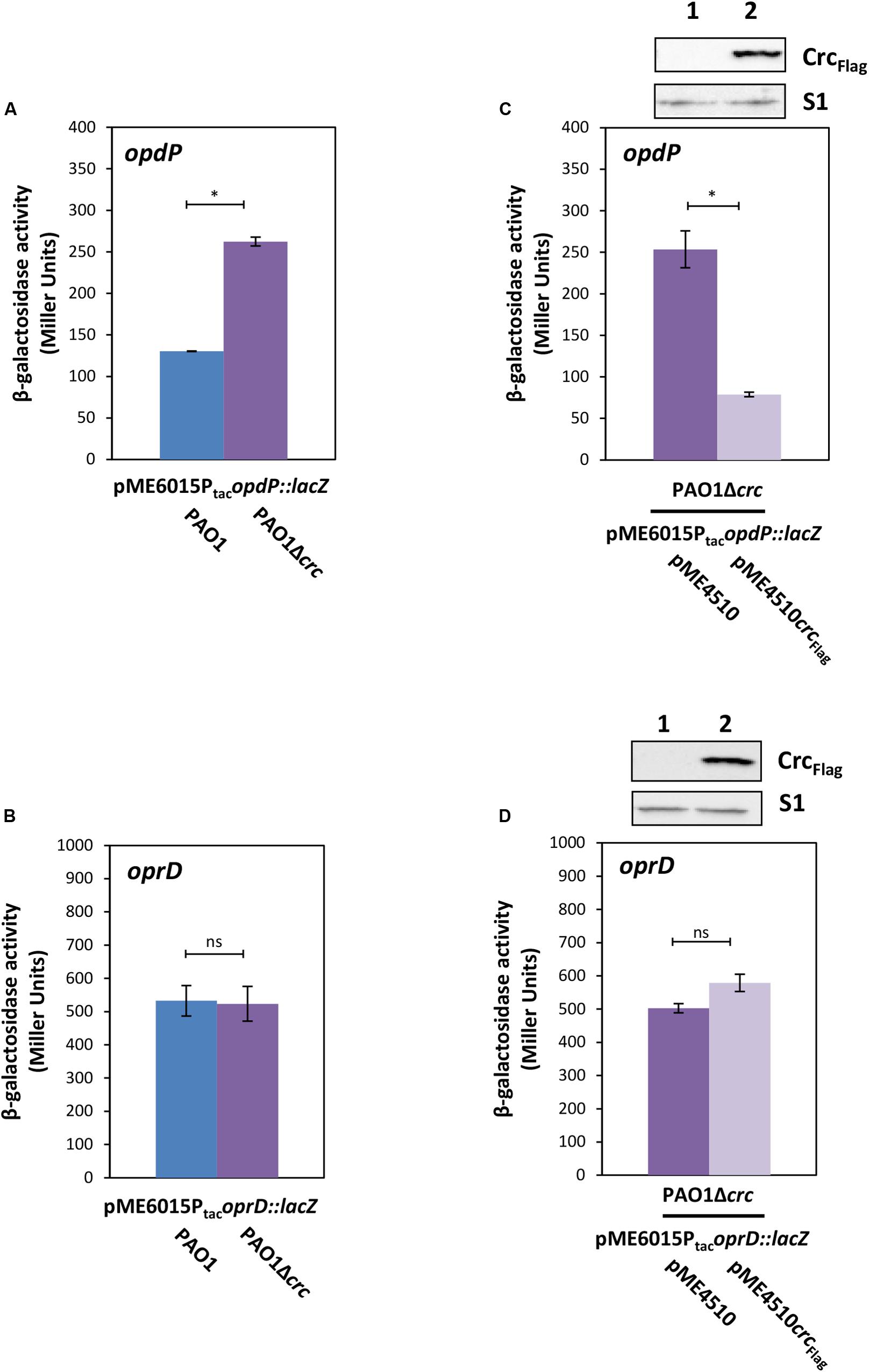
Figure 4. Crc impacts on opdP but not on oprD translation. (A,B) The strains PAO1 (blue bar) and PAO1Δcrc (purple bar) harboring either plasmid pME6015PtacopdP::lacZ (A) or pME6015PtacoprD::lacZ (B) were grown in LB medium. Samples were withdrawn at an OD600 of 2.0. The bars represent the β-galactosidase values conferred by the opdP::lacZ translational fusion gene encoded by plasmid pME6015PtacopdP::lacZ (A) and by the oprD::lacZ translational fusion gene encoded by plasmid pME6015PtacoprD::lacZ (B). The error bars represent standard deviations from two and three independent experiments, respectively. (C,D) The strains PAO1Δcrc(pME4510) (purple bar) and PAO1Δcrc(pME4510crcFlag) (light purple bar) harboring either plasmid pME6015Ptac opdP::lacZ (C) or pME6015PtacoprD::lacZ (D) were grown in LB medium. Samples were withdrawn at an OD600 of 2.0. The bars represent the β-galactosidase values conferred by the opdP::lacZ translational fusion gene encoded by plasmid pME6015PtacopdP::lacZ (C) and by the oprD::lacZ translational fusion gene encoded by plasmid pME6015PtacoprD::lacZ (D), respectively, in the presence or absence of ectopic crcFlag expression. The error bars represent standard deviations from two independent experiments. Statistical analyses were performed in Excel with a two tailed distributed Student’s T-test of two sample arrays with unequal variance. ns P > 0.05, *P ≤ 0.05. Top panels, Crc–Flag and S1 levels in strains PAO1Δcrc(pME4510) (lane 1) and PAO1Δcrc(pME4510crcFlag) (lane 2) harboring either plasmid pME6015PtacopdP::lacZ (C) or pME6015PtacoprD::lacZ (D). The Crc levels were determined by Western-blot analyses using anti-Crc antibodies. Immunodetection of ribosomal protein S1 served as a loading control.
High CrcZ Levels Increase oprD and opdP Translation
Transcription of the Hfq titrating RNA CrcZ is known to be induced by less preferred carbon sources such as mannitol (Sonnleitner et al., 2009). Therefore, we next tested whether the susceptibility toward imipenem is increased in an oprD deletion strain during growth in the presence of mannitol (high levels of CrcZ; Sonnleitner et al., 2009) when compared to the presence of succinate (low levels of CrcZ; Sonnleitner et al., 2009), i.e., whether translational repression of opdP by Hfq/Crc is relieved in the presence of CrcZ and in the absence of CCR. The disk diffusion assay revealed extended growth inhibition zones for the oprD mutant strain during growth in mannitol (Table 1), and therefore increased susceptibility to the tested antibiotic, consistent with the notion that opdP translation is controlled by Hfq/Crc. The same experiment conducted with an opdP deletion strain revealed a comparable result, indicating that CrcZ also interferes with Hfq/EsrA/Sr0161-mediated riboregulation of oprD. In support, ectopic overexpression of crcZ from plasmid pMMBcrcZ in strains PAO1(pME6015PtacoprD::lacZ) (Supplementary Figure S4A) and PAO1(pME6015PtacopdP::lacZ) (Supplementary Figure S4B) confirmed that high levels of CrcZ increase both oprD and opdP translation. Again, these findings are in line with a reduced minimal inhibitory concentration (MIC) of imipenem upon ectopic overexpression of crcZ in PAO1 (Supplementary Figure S4C).
Discussion
The outer membrane porins OprD and OpdP are required for the uptake of carbapenems (Tamber and Hancock, 2006). Here, we have verified and shown that Hfq is involved in negative translational riboregulation of oprD by the sRNAs EsrA and Sr0161. Moreover, we have provided evidence for Hfq/Crc-mediated regulation of opdP. Hence, the increased susceptibility of P. aeruginosa hfq deletion mutants toward imipenem (Pusic et al., 2018) can be rationalized at the molecular level by these studies. However, we cannot exclude that hitherto unknown Hfq-mediated regulatory circuits additionally impact oprD and opdP regulation.
Zhang et al. (2017) observed no specific amplicons of chimeras formed by Sr0161 and oprD mRNA in a Δhfq strain. In addition, they showed that the oprD mRNA levels are increased in a Δhfq strain. These experiments indicated that Sr0161-dependent negative regulation of oprD is Hfq dependent (Zhang et al., 2017). Using the triple mutant strain PAO1ΔhfqΔersAΔsr0161(pME6015PtacoprD::lacZ), we have shown directly that translational repression of oprD::lacZ upon ectopic expression of either esrA or sr0161 depends on Hfq (Figure 3D). Ectopic expression of Crc in strain PAO1Δcrc(pME6015PtacoprD::lacZ) insignificantly increased translation of oprD::lacZ (Figure 4D) rather than decreased it. This observation is consistent with our previous results in that over-expression of crc can interfere with Hfq-mediated riboregulation (Sonnleitner et al., 2018), and would argue against negative regulation of oprD translation by a repressive Hfq/Crc complex. In this context, it is also worth noting that the absence of Hfq in strain PAO1ΔhfqΔersAΔsr0161(pME6015PtacoprD::lacZ) (Figure 3C) did not result in increased de-repression of oprD::lacZ translation when compared with strain PAO1ΔersAΔsr0161(pME6015PtacoprD::lacZ) (Figure 3C), again indicating that direct translational repression of oprD by Hfq/Crc does not occur in addition to Hfq/EsrA/Sr0161-mediated riboregulation (Figure 5A). This hypothesis also agrees with other studies (Corona et al., 2018), which indicated that oprD is not post-transcriptionally regulated by Crc.
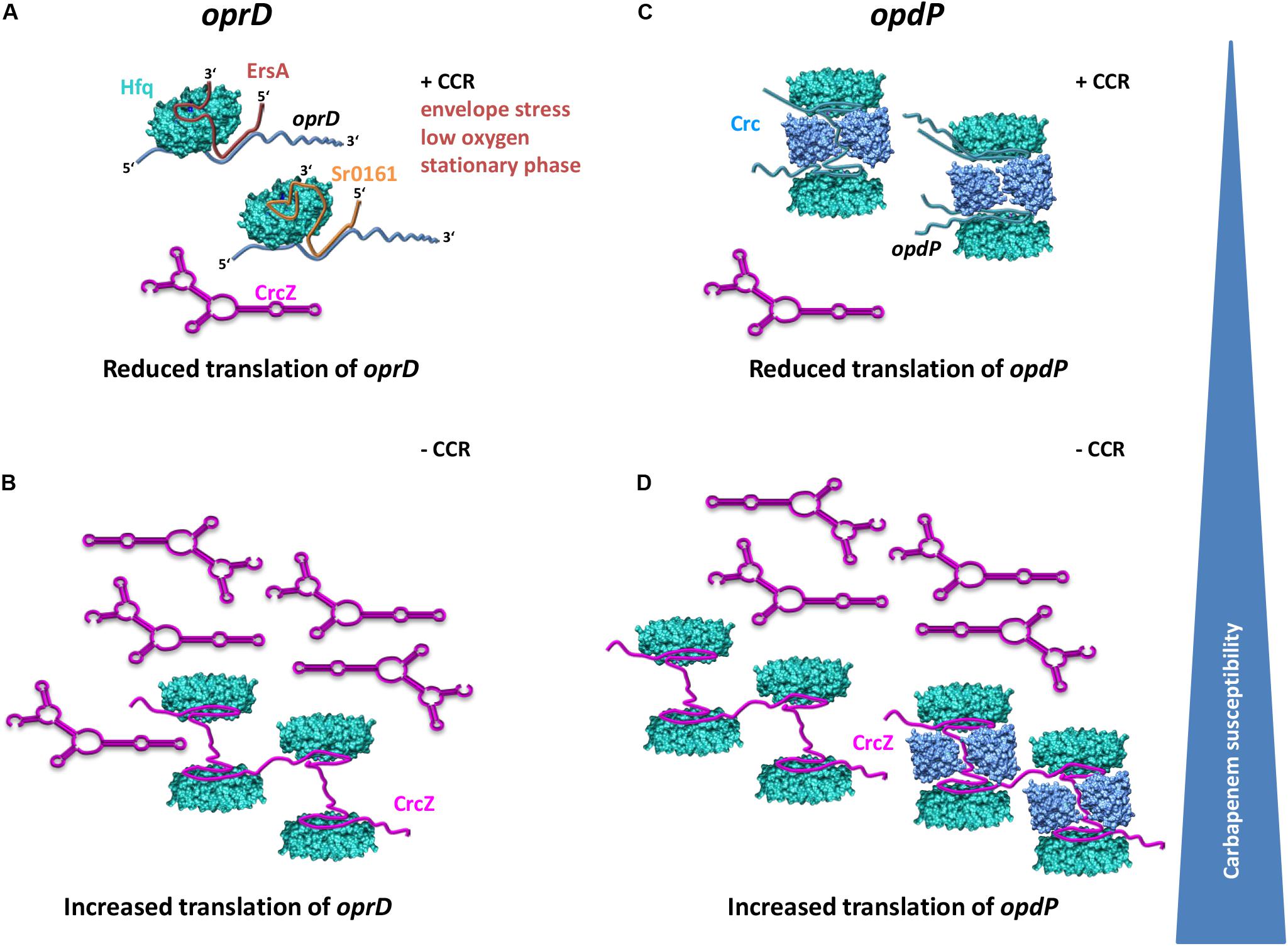
Figure 5. Model for distinctive translational regulation of oprD and opdP by Hfq/EsrA/Sr0161 and Hfq/Crc, respectively. (A) Under conditions of envelope stress, low oxygen and during stationary phase, EsrA is synthesized and represses translation of oprD in a Hfq dependent manner if the levels of the Hfq-titrating CrcZ RNA are low as it is the case during CCR (+CCR) (Sonnleitner et al., 2009). The environmental cues leading to synthesis of Sr0161, the Hfq-dependent second negative regulator of oprD, are unknown. (B) Alleviation of CCR results in increased levels of the RNA CrcZ, which will titrate Hfq and result in increased translation of oprD. (C) When CCR is in place, translation of opdP is reduced by a repressive Hfq/Crc complex. (D) Alleviation of CCR results in CrcZ synthesis and thus in titration of Hfq and/or Hfq/Crc complexes, which in turn will relieve translational repression of oprD. Translational repression of oprD by Hfq/ErsA/Sr0161 and of opdP by Hfq/Crc, respectively, will lead to reduced influx of carbapenems and thus increased resistance toward these antibiotics. In opposite, titration of Hfq and or Hfq/Crc by the RNA CrcZ will lead to increased translation of both, oprD and opdP concomitantly with an increased influx of and susceptibility to carbapenems.
Zhang et al. (2017) reported that deletion and over-expression of sr0161 resulted in increased and decreased opdP mRNA levels, respectively, which was indicative for Sr0161-dependent regulation of opdP. However, our experiments performed with the translational opdP::lacZ reporter gene did not reveal direct evidence for an involvement of the sRNA Sr0161 in opdP translation (Figures 3A,B). Hence, we can only speculate that the observations made by Zhang et al. (2017) result from indirect effects. It is also worth noting that the putative Hfq and Sr0161 binding sites appear to overlap (Figure 2B). Thus, Hfq would be assumed to compete with sRNA binding rather than support the interaction with opdP. Our results are rather consistent with a model wherein a repressive Hfq/Crc complex prevents opdP translation when CCR is in place (Figure 5C). In support, our studies showed that opdP::lacZ translation is de-repressed and repressed in the absence of Crc and upon ectopic expression of crc-flag, respectively.
Hfq is titrated by the regulatory RNA CrcZ, which abrogates its function in Hfq-Crc-mediated translational repression during CCR (Sonnleitner and Bläsi, 2014) as well as in Hfq/sRNA-mediated riboregulation (Sonnleitner and Bläsi, 2014; Sonnleitner et al., 2017). Previous studies have shown that the levels of CrcZ are comparatively low when the cells are cultured in the presence of succinate (CCR) when compared with cells cultured in the presence of mannitol (no CCR) (Sonnleitner et al., 2009; Valentini et al., 2014). The model shown in Figure 5 therefore specifies that in the presence of low levels of CrcZ (succinate; CCR), the translation of both, oprD and opdP, is negatively regulated by Hfq/EsrA/Sr0161-mediated riboregulation (Figure 5A) and by Hfq/Crc repressive complexes (Figure 5C), respectively. In opposite, increasing levels of CrcZ (mannitol; no CCR) are anticipated to result in titration of Hfq, and in translation of oprD and opdP (Figures 5B,D). Hence, the differential imipenem susceptibility (Table 1) during growth on succinate and mannitol can thus be rationalized in light of Hfq-dependent oprD/opdP regulation and of the antagonizing function of the RNA CrcZ on Hfq regulatory complexes.
Data Availability Statement
The datasets presented in this study can be found in online repositories. The names of the repository/repositories and accession number(s) can be found in the article/ Supplementary Material.
Author Contributions
UB, ES, and PP conceived and designed the experiments. ES and PP performed the experiments. ES, PP, UB, and MW analyzed the data. ES and UB wrote the manuscript.
Funding
The work was supported by the Austrian Science Fund (www.fwf.ac.at/en) through project P28711-B22 (UB and ES) and through the Special Research Program RNA-REG F43, subproject AF4311 (UB). PP was supported through the FWF funded doctoral program RNA-Biology W-1207.
Conflict of Interest
The authors declare that the research was conducted in the absence of any commercial or financial relationships that could be construed as a potential conflict of interest.
Acknowledgments
We are grateful to Dr. S. Lory and Dr. Y. F. Zhang, Harvard Medical School, and to Dr. A. A. Miller, AstraZeneca Infection Innovative Medicines, United States, for providing plasmids and strains as well as to Beatrice Krennmayr and Dorothea A. Heitzinger for excellent technical assistance.
Supplementary Material
The Supplementary Material for this article can be found online at: https://www.frontiersin.org/articles/10.3389/fmicb.2020.01001/full#supplementary-material
Footnotes
References
Bassetti, M., Vena, A., Croxatto, A., Righi, E., and Guery, B. (2018). How to manage Pseudomonas aeruginosa infections. Drugs Context 7:212527. doi: 10.7573/dic.212527
Breidenstein, E. B., de la Fuente-Nunez, C., and Hancock, R. E. (2011). Pseudomonas aeruginosa: all roads lead to resistance. Trends Microbiol. 19, 419–426. doi: 10.1016/j.tim.2011.04.005
Buehrle, D. J., Shields, R. K., Clarke, L. G., Potoski, B. A., Clancy, C. J., and Nguyen, M. H. (2017). Carbapenem-resistant Pseudomonas aeruginosa bacteremia: risk factors for mortality and microbiologic treatment failure. Antimicrob. Agents Chemother. 61:1216. doi: 10.1128/AAC.01243-1216
Castanheira, M., Deshpande, L. M., Costello, A., Davies, T. A., and Jones, R. N. (2014). Epidemiology and carbapenem resistance mechanisms of carbapenem-non-susceptible Pseudomonas aeruginosa collected during 2009-11 in 14 European and Mediterranean countries. J. Antimicrob. Chemother. 69, 1804–1814. doi: 10.1093/jac/dku048
Chalhoub, H., Saenz, Y., Rodriguez-Villalobos, H., Denis, O., Kahl, B. C., Tulkens, P. M., et al. (2016). High-level resistance to meropenem in clinical isolates of Pseudomonas aeruginosa in the absence of carbapenemases: role of active efflux and porin alterations. Int. J. Antimicrob. Agents 48, 740–743. doi: 10.1016/j.ijantimicag.2016.09.012
Chevalier, S., Bouffartigues, E., Bodilis, J., Maillot, O., Lesouhaitier, O., Feuilloley, M. G. J., et al. (2017). Structure, function and regulation of Pseudomonas aeruginosa porins. FEMS Microbiol. Rev. 41, 698–722. doi: 10.1093/femsre/fux020
Corona, F., Reales-Calderon, J. A., Gil, C., and Martinez, J. L. (2018). The development of a new parameter for tracking post-transcriptional regulation allows the detailed map of the Pseudomonas aeruginosa Crc regulon. Sci. Rep. 8:16793. doi: 10.1038/s41598-018-34741-34749
Ducret, V., Gonzalez, M. R., Scrignari, T., and Perron, K. (2016). OprD repression upon metal treatment requires the RNA chaperone Hfq in Pseudomonas aeruginosa. Genes 7:82. doi: 10.3390/genes7100082
Eren, E., Vijayaraghavan, J., Liu, J., Cheneke, B. R., Touw, D. S., Lepore, B. W., et al. (2012). Substrate specificity within a family of outer membrane carboxylate channels. PLoS Biol. 10:e1001242. doi: 10.1371/journal.pbio.1001242
Ferrara, S., Carloni, S., Fulco, R., Falcone, M., Macchi, R., and Bertoni, G. (2015). Post-transcriptional regulation of the virulence-associated enzyme AlgC by the sigma(22) -dependent small RNA ErsA of Pseudomonas aeruginosa. Environ. Microbiol. 17, 199–214. doi: 10.1111/1462-2920.12590
Fritzenwanker, M., Imirzalioglu, C., Herold, S., Wagenlehner, F. M., Zimmer, K. P., and Chakraborty, T. (2018). Treatment options for carbapenem- resistant gram-negative infections. Dtsch. Arztebl. Int. 115, 345–352. doi: 10.3238/arztebl.2018.0345
Hancock, R. E., and Brinkman, F. S. (2002). Function of pseudomonas porins in uptake and efflux. Annu. Rev. Microbiol. 56, 17–38. doi: 10.1146/annurev.micro.56.012302.160310
Hancock, R. E., and Woodruff, W. A. (1988). Roles of porin and beta-lactamase in beta-lactam resistance of Pseudomonas aeruginosa. Rev. Infect. Dis. 10, 770–775. doi: 10.1093/clinids/10.4.770
Hmelo, L. R., Borlee, B. R., Almblad, H., Love, M. E., Randall, T. E., Tseng, B. S., et al. (2015). Precision-engineering the Pseudomonas aeruginosa genome with two-step allelic exchange. Nat. Protoc. 10, 1820–1841. doi: 10.1038/nprot.2015.115
Hoffmann, S., Otto, C., Kurtz, S., Sharma, C. M., Khaitovich, P., Vogel, J., et al. (2009). Fast mapping of short sequences with mismatches, insertions and deletions using index structures. PLoS Comput. Biol. 5:e1000502. doi: 10.1371/journal.pcbi.1000502
Isabella, V. M., Campbell, A. J., Manchester, J., Sylvester, M., Nayar, A. S., Ferguson, K. E., et al. (2015). Toward the rational design of carbapenem uptake in Pseudomonas aeruginosa. Chem. Biol. 22, 535–547. doi: 10.1016/j.chembiol.2015.03.018
Ishikawa, H., Otaka, H., Maki, K., Morita, T., and Aiba, H. (2012). The functional Hfq-binding module of bacterial sRNAs consists of a double or single hairpin preceded by a U-rich sequence and followed by a 3’ poly(U) tail. RNA 18, 1062–1074. doi: 10.1261/rna.031575.111
Kambara, T. K., Ramsey, K. M., and Dove, S. L. (2018). Pervasive targeting of nascent transcripts by Hfq. Cell Rep. 23, 1543–1552. doi: 10.1016/j.celrep.2018.03.134
Kavita, K., de Mets, F., and Gottesman, S. (2018). New aspects of RNA-based regulation by Hfq and its partner sRNAs. Curr. Opin. Microbiol. 42, 53–61. doi: 10.1016/j.mib.2017.10.014
Linares, J. F., Moreno, R., Fajardo, A., Martinez-Solano, L., Escalante, R., Rojo, F., et al. (2010). The global regulator Crc modulates metabolism, susceptibility to antibiotics and virulence in Pseudomonas aeruginosa. Environ. Microbiol. 12, 3196–3212. doi: 10.1111/j.1462-2920.2010.02292.x
Link, T. M., Valentin-Hansen, P., and Brennan, R. G. (2009). Structure of Escherichia coli Hfq bound to polyriboadenylate RNA. Proc. Natl. Acad. Sci. U.S.A. 106, 19292–19297. doi: 10.1073/pnas.0908744106
Martin, M. (2011). Cutadapt removes adapter sequences from high-throughput sequencing reads. EMBnet. J. 17:10. doi: 10.14806/ej.17.1.200
McDougall, D. A., Morton, A. P., and Playford, E. G. (2013). Association of ertapenem and antipseudomonal carbapenem usage and carbapenem resistance in Pseudomonas aeruginosa among 12 hospitals in Queensland. Austr. J. Antimicrob. Chemother. 68, 457–460. doi: 10.1093/jac/dks385
Mikulecky, P. J., Kaw, M. K., Brescia, C. C., Takach, J. C., Sledjeski, D. D., and Feig, A. L. (2004). Escherichia coli Hfq has distinct interaction surfaces for DsrA, rpoS and poly(A) RNAs. Nat. Struct. Mol. Biol. 11, 1206–1214. doi: 10.1038/nsmb858
Miller, J. H. (1972). Experiments in Molecular Genetics. Cold Spring Harbor, NY: Cold Spring Harbor Laboratory.
Moreno, R., Hernandez-Arranz, S., La Rosa, R., Yuste, L., Madhushani, A., Shingler, V., et al. (2015). The Crc and Hfq proteins of Pseudomonas putida cooperate in catabolite repression and formation of ribonucleic acid complexes with specific target motifs. Environ. Microbiol. 17, 105–118. doi: 10.1111/1462-2920.12499
Murina, V., Lekontseva, N., and Nikulin, A. (2013). Hfq binds ribonucleotides in three different RNA-binding sites. Acta Crystallogr. D Biol. Crystallogr. 69(Pt 8), 1504–1513. doi: 10.1107/S090744491301010X
Ochs, M. M., Lu, C. D., Hancock, R. E., and Abdelal, A. T. (1999). Amino acid-mediated induction of the basic amino acid-specific outer membrane porin OprD from Pseudomonas aeruginosa. J. Bacteriol. 181, 5426–5432.
Otaka, H., Ishikawa, H., Morita, T., and Aiba, H. (2011). PolyU tail of rho-independent terminator of bacterial small RNAs is essential for Hfq action. Proc. Natl. Acad. Sci. U.S.A. 108, 13059–13064. doi: 10.1073/pnas.1107050108
Palmer, K. L., Aye, L. M., and Whiteley, M. (2007). Nutritional cues control Pseudomonas aeruginosa multicellular behavior in cystic fibrosis sputum. J. Bacteriol. 189, 8079–8087. doi: 10.1128/JB.01138-1137
Panja, S., Schu, D. J., and Woodson, S. A. (2013). Conserved arginines on the rim of Hfq catalyze base pair formation and exchange. Nucleic Acids Res. 41, 7536–7546. doi: 10.1093/nar/gkt521
Papp-Wallace, K. M., Endimiani, A., Taracila, M. A., and Bonomo, R. A. (2011). Carbapenems: past, present, and future. Antimicrob. Agents Chemother. 55, 4943–4960. doi: 10.1128/AAC.00296-211
Pei, X. Y., Dendooven, T., Sonnleitner, E., Chen, S., Blasi, U., and Luisi, B. F. (2019). Architectural principles for Hfq/Crc-mediated regulation of gene expression. eLife 8:43158. doi: 10.7554/eLife.43158
Peng, Y., Curtis, J. E., Fang, X., and Woodson, S. A. (2014). Structural model of an mRNA in complex with the bacterial chaperone Hfq. Proc. Natl. Acad. Sci. U.S.A. 111, 17134–17139. doi: 10.1073/pnas.1410114111
Pusic, P., Sonnleitner, E., Krennmayr, B., Heitzinger, D. A., Wolfinger, M. T., Resch, A., et al. (2018). Harnessing metabolic regulation to increase Hfq-dependent antibiotic susceptibility in Pseudomonas aeruginosa. Front. Microbiol. 9:2709. doi: 10.3389/fmicb.2018.02709
Quinn, J. P., Dudek, E. J., DiVincenzo, C. A., Lucks, D. A., and Lerner, S. A. (1986). Emergence of resistance to imipenem during therapy for Pseudomonas aeruginosa infections. J. Infect. Dis. 154, 289–294. doi: 10.1093/infdis/154.2.289
Rietsch, A., Vallet-Gely, I., Dove, S. L., and Mekalanos, J. J. (2005). ExsE, a secreted regulator of type III secretion genes in Pseudomonas aeruginosa. Proc. Natl. Acad. Sci. U.S.A. 102, 8006–8011. doi: 10.1073/pnas.0503005102
Robinson, K. E., Orans, J., Kovach, A. R., Link, T. M., and Brennan, R. G. (2014). Mapping Hfq-RNA interaction surfaces using tryptophan fluorescence quenching. Nucleic Acids Res. 42, 2736–2749. doi: 10.1093/nar/gkt1171
Sacha, P., Wieczorek, P., Hauschild, T., Zorawski, M., Olszanska, D., and Tryniszewska, E. (2008). Metallo-beta-lactamases of Pseudomonas aeruginosa–a novel mechanism resistance to beta-lactam antibiotics. Folia Histochem. Cytobiol. 46, 137–142. doi: 10.2478/v10042-008-0020-29
Sambrook, J., Fritsch, E. F., and Maniatis, T. (1989). Molecular Cloning: A Laboratory Manual. Cold Spring Harbor, NY: Cold Spring Harbor Laboratory Press.
Sauer, E., Schmidt, S., and Weichenrieder, O. (2012). Small RNA binding to the lateral surface of Hfq hexamers and structural rearrangements upon mRNA target recognition. Proc. Natl. Acad. Sci. U.S.A. 109, 9396–9401. doi: 10.1073/pnas.1202521109
Sauer, E., and Weichenrieder, O. (2011). Structural basis for RNA 3’-end recognition by Hfq. Proc. Natl. Acad. Sci. U.S.A. 108, 13065–13070. doi: 10.1073/pnas.1103420108
Schu, D. J., Zhang, A., Gottesman, S., and Storz, G. (2015). Alternative Hfq-sRNA interaction modes dictate alternative mRNA recognition. EMBO J. 34, 2557–2573. doi: 10.15252/embj.201591569
Schumacher, M. A., Pearson, R. F., Moller, T., Valentin-Hansen, P., and Brennan, R. G. (2002). Structures of the pleiotropic translational regulator Hfq and an Hfq-RNA complex: a bacterial Sm-like protein. EMBO J. 21, 3546–3556. doi: 10.1093/emboj/cdf322
Sonnleitner, E., Abdou, L., and Haas, D. (2009). Small RNA as global regulator of carbon catabolite repression in Pseudomonas aeruginosa. Proc. Natl. Acad. Sci. U.S.A. 106, 21866–21871. doi: 10.1073/pnas.pnas.0910308106
Sonnleitner, E., and Bläsi, U. (2014). Regulation of Hfq by the RNA CrcZ in Pseudomonas aeruginosa carbon catabolite repression. PLoS Genet. 10:e1004440. doi: 10.1371/journal.pgen.1004440
Sonnleitner, E., Hagens, S., Rosenau, F., Wilhelm, S., Habel, A., Jager, K. E., et al. (2003). Reduced virulence of a hfq mutant of Pseudomonas aeruginosa O1. Microb. Pathog. 35, 217–228. doi: 10.1016/s0882-4010(03)00149-149
Sonnleitner, E., Prindl, K., and Bläsi, U. (2017). The Pseudomonas aeruginosa CrcZ RNA interferes with Hfq-mediated riboregulation. PLoS One 12:e0180887. doi: 10.1371/journal.pone.0180887
Sonnleitner, E., Schuster, M., Sorger-Domenigg, T., Greenberg, E. P., and Bläsi, U. (2006). Hfq-dependent alterations of the transcriptome profile and effects on quorum sensing in Pseudomonas aeruginosa. Mol. Microbiol. 59, 1542–1558. doi: 10.1111/j.1365-2958.2006.05032.x
Sonnleitner, E., Wulf, A., Campagne, S., Pei, X. Y., Wolfinger, M. T., Forlani, G., et al. (2018). Interplay between the catabolite repression control protein Crc, Hfq and RNA in Hfq-dependent translational regulation in Pseudomonas aeruginosa. Nucleic Acids Res. 46, 1470–1485. doi: 10.1093/nar/gkx1245
Tacconelli, E., Carrara, E., Savoldi, A., Harbarth, S., Mendelson, M., Monnet, D. L., et al. (2018). Discovery, research, and development of new antibiotics: the WHO priority list of antibiotic-resistant bacteria and tuberculosis. Lancet Infect. Dis. 18, 318–327. doi: 10.1016/S1473-3099(17)30753-30753
Tamber, S., and Hancock, R. E. (2006). Involvement of two related porins, OprD and OpdP, in the uptake of arginine by Pseudomonas aeruginosa. FEMS Microbiol. Lett. 260, 23–29. doi: 10.1111/j.1574-6968.2006.00293.x
Tata, M., Wolfinger, M. T., Amman, F., Roschanski, N., Dotsch, A., Sonnleitner, E., et al. (2016). RNASeq based transcriptional profiling of Pseudomonas aeruginosa PA14 after short- and long-term anoxic cultivation in synthetic cystic fibrosis sputum medium. PLoS One 11:e0147811. doi: 10.1371/journal.pone.0147811
Trias, J., Dufresne, J., Levesque, R. C., and Nikaido, H. (1989). Decreased outer membrane permeability in imipenem-resistant mutants of Pseudomonas aeruginosa. Antimicrob. Agents Chemother. 33, 1202–1206. doi: 10.1128/aac.33.8.1202
Valentini, M., Garcia-Maurino, S. M., Perez-Martinez, I., Santero, E., Canosa, I., and Lapouge, K. (2014). Hierarchical management of carbon sources is regulated similarly by the CbrA/B systems in Pseudomonas aeruginosa and Pseudomonas putida. Microbiology 160(Pt 10), 2243–2252. doi: 10.1099/mic.0.078873-78870
Vogel, J., and Luisi, B. F. (2011). Hfq and its constellation of RNA. Nat. Rev. Microbiol. 9, 578–589. doi: 10.1038/nrmicro2615
Wienken, C. J., Baaske, P., Rothbauer, U., Braun, D., and Duhr, S. (2010). Protein-binding assays in biological liquids using microscale thermophoresis. Nat. Commun. 1:100. doi: 10.1038/ncomms1093
Winsor, G. L., Griffiths, E. J., Lo, R., Dhillon, B. K., Shay, J. A., and Brinkman, F. S. (2016). Enhanced annotations and features for comparing thousands of Pseudomonas genomes in the Pseudomonas genome database. Nucleic Acids Res. 44, D646–D653. doi: 10.1093/nar/gkv1227
Wolfinger, M. T., Fallmann, J., Eggenhofer, F., and Amman, F. (2015). ViennaNGS: a toolbox for building efficient next- generation sequencing analysis pipelines. F1000Research 4:50. doi: 10.12688/f1000research.6157.2
Keywords: Pseudomonas aeruginosa, opdP, oprD, carbapenem resistance, riboregulation, catabolite repression
Citation: Sonnleitner E, Pusic P, Wolfinger MT and Bläsi U (2020) Distinctive Regulation of Carbapenem Susceptibility in Pseudomonas aeruginosa by Hfq. Front. Microbiol. 11:1001. doi: 10.3389/fmicb.2020.01001
Received: 25 March 2020; Accepted: 24 April 2020;
Published: 26 May 2020.
Edited by:
Jose L. Martinez, Consejo Superior de Investigaciones Científicas, SpainReviewed by:
Pierre Cornelis, Vrije University Brussel, BelgiumRuggero La Rosa, Novo Nordisk Foundation Center for Biosustainability (DTU Biosustain), Denmark
Copyright © 2020 Sonnleitner, Pusic, Wolfinger and Bläsi. This is an open-access article distributed under the terms of the Creative Commons Attribution License (CC BY). The use, distribution or reproduction in other forums is permitted, provided the original author(s) and the copyright owner(s) are credited and that the original publication in this journal is cited, in accordance with accepted academic practice. No use, distribution or reproduction is permitted which does not comply with these terms.
*Correspondence: Elisabeth Sonnleitner, ZWxpc2FiZXRoLnNvbm5sZWl0bmVyQHVuaXZpZS5hYy5hdA==; Udo Bläsi, dWRvLmJsYWVzaUB1bml2aWUuYWMuYXQ=