- 1Shanxi Key Laboratory of Ecological Restoration on Loess Plateau, Institute of Loess Plateau, Shanxi University, Taiyuan, China
- 2Department of Biology, Georgia Southern University, Statesboro, GA, United States
Copper mining and the byproducts associated with the industry have led to serious pollution in the Loess Plateau of China. There is a potential in improving the ecological restoration efficiency of such degraded land through combining microbial and plant remediation approaches. However, the community structure and function of phyllosphere and rhizosphere microorganisms and their response to plant development in copper tailings dams are poorly understood. This study investigated the impact of the phyllosphere and rhizosphere microbial communities on Bothriochloa ischaemum during three distinct plant development stages: seedling, tiller, and mature. The relative species abundance and Shannon index of bacterial communities of the rhizosphere during the seedling and tiller stages were distinct from that in the mature stage. Dominant bacteria at the level of phyla, such as Proteobacteria, Cyanobacteria, Actinobacteria, and Bacteroidetes, followed distinct patterns associated with plant development in the phyllosphere, but the predominant bacteria were similar in the rhizosphere. Redundancy analysis showed that aboveground total nitrogen and the carbon and nitrogen ratio of this plant species significantly affected phyllosphere bacterial community structure, whereas soil water content, soil nutrients, electrical conductivity, and salinity significantly affected rhizosphere bacterial community structure. Moreover, keystone phyllosphere and rhizosphere bacterial species differed significantly. This study sheds new light on understanding the dynamic relationship of phyllosphere and rhizosphere bacterial communities during plant development in copper tailings. These results are beneficial to the development and utilization of beneficial microbial communities at different stages of development, which might help to reclaim and stabilize tailings more effectively.
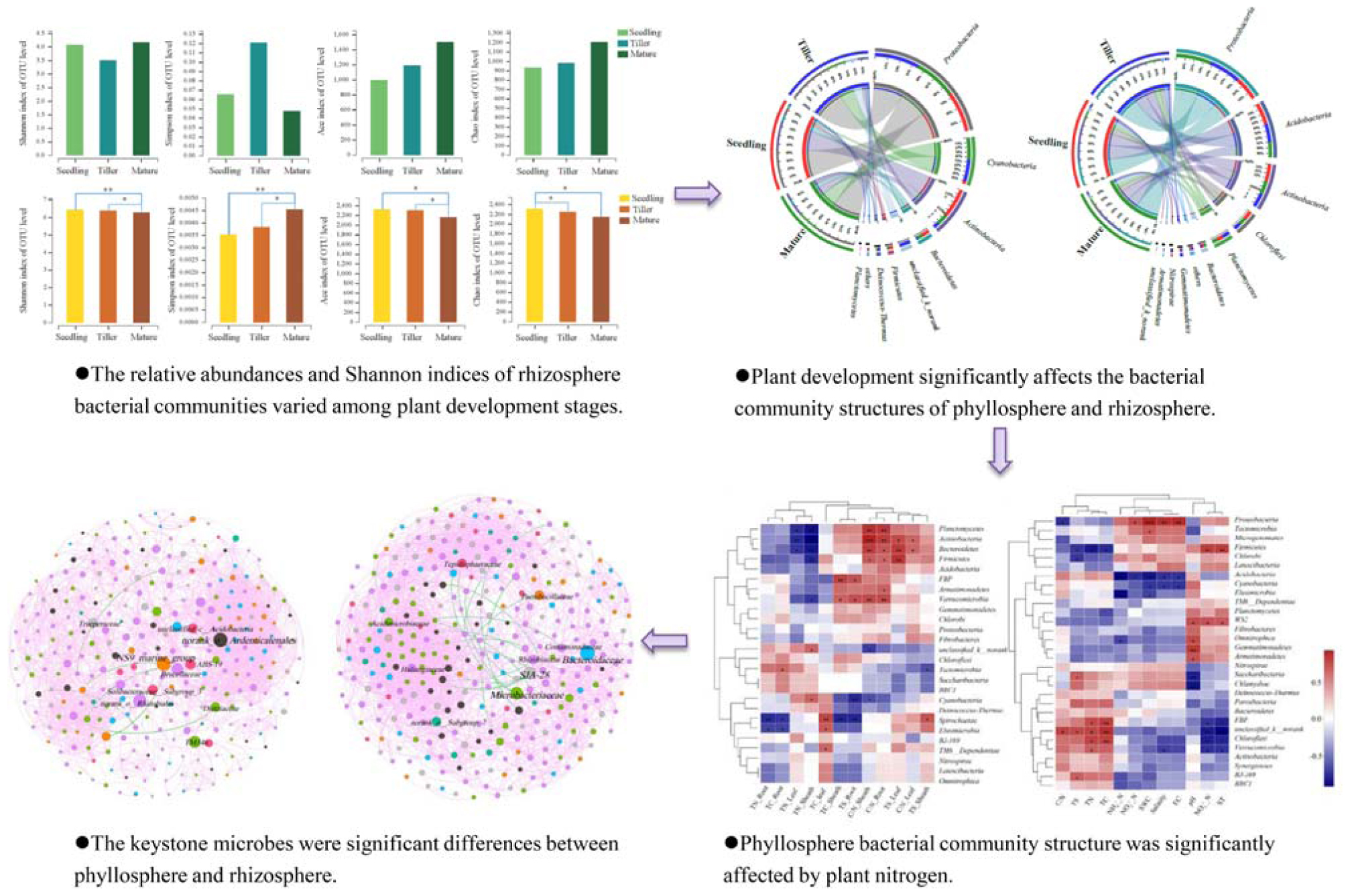
Graphical Abstract. The characteristics of phyllosphere and rhizosphere bacterial communities during the development of Bothriochloa ischaemum in a copper tailings.
Key Points:
(1) The phyllosphere bacterial community yields more unique OTUs than the rhizosphere community.
(2) Phyllosphere bacterial community structure is significantly affected by plant nitrogen content.
(3) Rhizosphere bacterial community structure is mainly affected by soil water content, soil nutrients, electrical conductivity, and salinity.
(4) Keystone phyllosphere and rhizosphere microbes significantly differ.
Introduction
Soil contamination is a key issue of the mining industry. There are many metal mines in China which produce vast waste byproducts, consequently causing severe environmental pollution (Wong, 2003; Jia et al., 2017). The mutualistic symbiosis between microorganisms and plants promotes plant growth, aids in the absorption of water and nutrients, fortifies heavy metal resilience, and increases heavy metal absorption by plants while increasing overall plant biomass, thus improving the phytoremediation process (Lazaridou et al., 2011). Both plants and their associative microbial communities are able to remediate heavy metal contamination. Consequently, the application potential of combining both approaches to increase heavy metal absorption and transformation efficiency in contaminated soil is prodigious (Conesa et al., 2007; He et al., 2007).
The phyllosphere naturally contains a diverse and abundant community of non-pathogenic microbes (Vorholt, 2012). Conservative estimates indicate that 6.4 × 108 km2 of global leaf surfaces harbor nearly 1026 bacteria, being the most bounteous and predominant colonists of the phyllosphere (Lindow and Brandl, 2003). The interaction between bacteria species and plants can be negative, causing disease, or positive, in that they can produce or modify hormones and metabolites or by interfering with pathogen growth. Their interactions can also be neutral and commensal (Lindow and Brandl, 2003). It has been shown that these bacterial populations are sufficiently large to play a dominant role in both vegetative growth (Chinnadurai et al., 2009; Janarthine and Eganathan, 2012) and vegetative resistance to pathogens (Costa et al., 2008; Balintkurti et al., 2010). However, to date most relevant research on phyllosphere bacteria has been concerned with the role of individual bacterium in protecting plants by suppressing pathogens (Costa et al., 2008) as well as how bacterial communities in the phyllosphere respond to climate change (Ren et al., 2014). Many factors control phyllosphere microbiota composition. For instance, environmental variables are dynamic, while host genotype and geographical location are more stable and constant (Vorholt, 2012). Although previous studies have identified certain factors (e.g., pH, heavy mental, and soil moisture) that have important impacts on structuring microbiota (Jia et al., 2018), results between studies still conflict.
Because root exudates are microbes’ primary source of nutrients, microbial interaction is particularly active in the rhizosphere, being the driver of both population density and activity (Raaijmakers et al., 2009; Bakker, 2012). Root-associated microbial communities are important in soil ecosystems, impacting plant growth and health, as well as influencing many soil biochemical processes (Zhao et al., 2015). Studies have shown that phyllosphere communities that reside on soil grown plants are similar to soil communities under natural conditions (Hardoim et al., 2012; Bodenhausen et al., 2013; Perazzolli et al., 2014).
Some plants including bean, soybean, maize, cabbage, cowpea, cotton, and Arabidopsis have exhibited age-related resistance (Marie-Pierre and Eric, 2010). Many studies have demonstrated that plant development can promote changes in microbial communities, but these studies have not determined which microbes or how such microbes contribute to observable changes. In some cases, host genotype is the primary factor that influences phyllosphere microbiome composition (Costa et al., 2012; Kim et al., 2012), although location has been shown in other cases to have the most significant impact on the composition of communities (Redford et al., 2010; Finkel et al., 2011; Rastogi et al., 2012). Season-dependent microbial communities that typically reside on perennial plants are similar from year to year (Redford and Fierer, 2009), while other microbial communities that reside on perennial plants change significantly, exhibiting significant seasonal variability (Jackson and Denney, 2011). Micallef et al. (2009) observed that rhizosphere microbial communities of Arabidopsis vary during the development of this plant species for which bulk density varies more significantly in communities during the early stages of plant development, decreasing as plants mature. Similarly, cultivar-dependent microbial communities have been shown to reside in the rhizosphere of young potato plants; however, such differences vanish as plants mature (Zgül et al., 2011).
Although many studies have shown that plant development affects plant associated microbial structures under natural conditions, there have only been limited studies conducted to date on the response of phyllosphere and rhizosphere bacterial communities to plant development in areas of environmental pollution. Moreover, a comprehensive comparison between phyllosphere and rhizosphere bacterial communities and their variable driving factors across plant development stages are extremely limited. Accordingly, this study investigated the structure and function of phyllosphere and rhizosphere bacterial communities associated with Bothriochloa ischaemum during three different plant development stages: seedling, tiller, and mature. The objectives of this study were (1) to survey phyllosphere and rhizosphere bacterial community composition during three distinct physiological developmental stages; (2) to examine the dynamic relationship of phyllosphere and rhizosphere bacterial communities during plant development; and (3) to test whether the driving factors of these phyllosphere and rhizosphere bacterial communities differ.
Materials and Methods
Site Description
Construction on the Shibahe tailings dam (latitude 35°15′∼35°17′ N, longitude 118°38′∼111°39′ E) commenced in 1969, and the dam is part of the Northern Copper Mine, situated in southern Shanxi Province, China. Currently, this copper tailings dam is comprised of 14 sub-dams. The study area is marked by four distinct seasons subject to a continental “monsoon” climate wherein annual mean temperature = 14°C, annual precipitation = ∼780 mm, and frost free days = greater than 200 d (Jia et al., 2017).
Plant and Soil Sampling
We selected the No. 536 sub-dam of the Shibahe tailings dam in July 2017 for investigation. This sub-dam, in its twentieth year of restoration, was used for sampling during the three selected plant growth stages (seedling, tiller, and mature). The dominant plant species in this sub-dam was B. ischaemum. We randomly collected shoots and roots from B. ischaemum specimens as well as rhizosphere soil samples in three 1 m × 1 m sample plots, and each sample plot was spaced greater than 50 m apart. In each plot, 60 leaf samples were selected. The leaves were sealed in sterile plastic bags using tweezers sterilized in ethanol. For the plant samples, one subsample was used for physiochemical properties and the other was transported to the laboratory where it was stored (−20°C) in advance of high-throughput sequencing. For rhizosphere soil samples, visible roots and any residue were removed prior to homogenizing the soil fraction of each sample. Fresh soil samples were divided into two subsamples after sifting through a 2 mm sieve. The first subsample was stored (4°C) in advance of determining the physiochemical properties while the second was stored (−20°C) in advance of DNA extraction.
Plant and Soil Chemical Properties
We measured the total carbon (TC), total nitrogen (TN), and total sulfur (TS) content of plant and soil samples using an elemental analyzer (vario EL/MACRO cube, Elementar, Hanau, Germany) (Tables 1, 2). Soil water (1:2.5 mass/volume) suspensions were shaken for 30 min in advance of measuring soil pH. Gravimetric analysis was used to measure soil moisture. We measured ammonium nitrogen (NH4+-N), nitrate nitrogen (NO3+-N), and nitrite nitrogen (NO2+-N) in soil using the Automatic Discrete Analyzer (CleverChem 380, DeChem-Tech, GmbH, Hamburg, Germany) (Table 2). The intra-group differences between the samples were smaller than the inter-group differences, which could reflect the overall situations of plant and soil.
DNA Extraction, PCR Amplification, and Miseq Sequencing
Thirty leaf samples were randomly collected from each plot. These leaf samples were washed three times in a sterile phosphate buffer solution (PBS: NaCl, KCl, Na2HPO4, and KH2PO4) before being filtered through a sterile membrane filter (0.2 μm pore size) (Millipore, Jinteng, Tianjin, China). These filtered samples used to extract microbial DNA were sealed in sterile centrifuge tubes. The EZNA® Soil DNA Kit (Omega Bio-tek, Norcross, GA, United States) was used to extract plant and soil microbial DNA under the manufacturer’s protocol. Extracted DNA was quantified using a NanoDrop ND-1000 UV-Vis Spectrophotometer (NanoDrop Technologies, Wilmington, DE, United States). Amplification of the V4-V5 hyper variable region of the 16S rRNA bacterial gene was conducted using primers 515F (5′-GTGCCAGCMGCCGCGG-3′) and 907R (5′- CCGTCAATTCMTTTRAGT TT-3′). Sequencing was conducted at Shanghai Majorbio Bio-pharm Technology (Shanghai, China) using the MiSeq platform (Illumina, Inc., United States). The bacterial sequences have been deposited in the SRA of the NCBI database under the SRA accession: PRJNA600330.
Statistical Analysis
SPSS 20 was used to calculate the data that we derived from the aforementioned analyses, while Circos software was used to build the Circos graph (Krzywinski and Schein, 2009). Heatmapping of the top ten genera in each sample was conducted using the R packages. Principal component analysis (PCA) and redundancy analysis (RDA) were used to analyze relationships between bacteria and environmental factors using Canoco 5.0 (Microcomputer Power, United States). We used one-way analysis of variance (ANOVA) to determine the differences in environmental parameters, alpha diversity (α-diversity) indices, and the relative species abundance of the dominant bacterial species among the three selected plant growth stages. Additionally, we used the Mantel test to determine correlations between bacterial communities and environmental variables. We used the interactive platform Gephi to explore and visualize networks (Bastian et al., 2009). Structural equation models (SEM) was analyzed with AMOS 13.0.
Results
Overall Taxonomic Distribution and Bacterial Diversity
We obtained a total of 705,846 high-quality sequence reads of an average length of 396 bp across all samples. A total of 1,982 bacterial OTUs from phyllosphere and 2,909 bacterial OTUs from rhizosphere soil samples were determined based on 97% sequence similarity. Taxonomically classified OTUs were all associated with 32 phyla, 80 classes, 70 orders, 319 families, and 563 genera in rhizosphere soil samples. For phyllosphere samples, taxonomically classified OTUs were associated with 26 phyla, 59 classes, 140 orders, 287 families, and 589 genera. The Venn diagram revealed that 480 OTUs were common to all phyllosphere bacterial communities while 1,837 were common to all rhizosphere bacterial communities (Supplementary Figure S1). Phyllosphere bacterial communities yielded a greater number of unique OTUs compared to rhizosphere bacterial communities during each plant development stage (Supplementary Figure S1). The relative abundance (Ace and Chao1) and diversity (Shannon and Simpson) index values of the phyllosphere and rhizosphere bacterial communities revealed the occurrence of dynamic change during the three plant development stages (Figure 1).
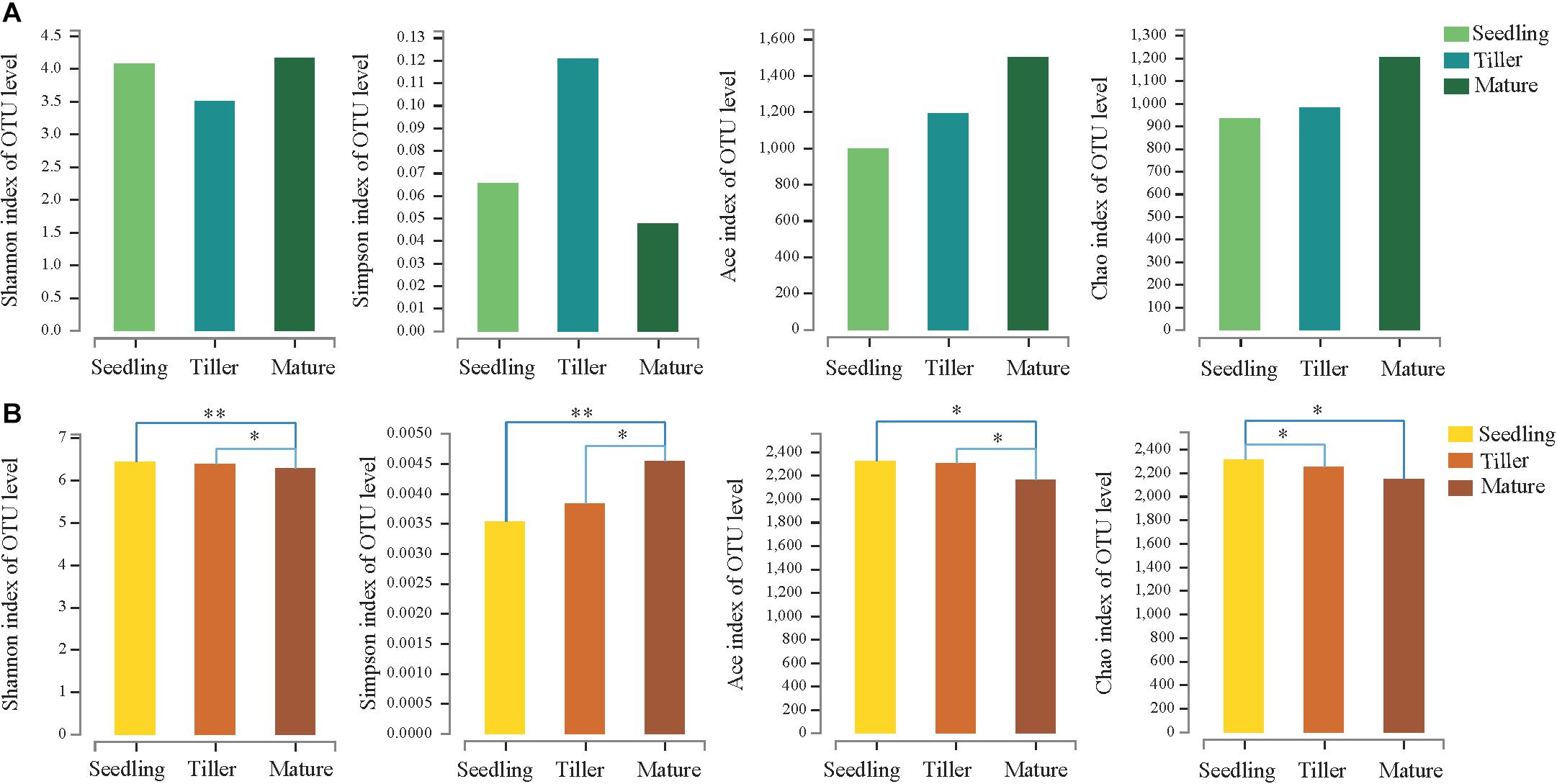
Figure 1. Estimated values of phyllosphere (A) and rhizosphere (B) bacterial community relative abundances and diversity index. Asterisks indicate significant differences among plant development stages, ∗∗P < 0.01, ∗P < 0.05.
Comparison Between Bacterial Communities Among Plant Development Stages
The dominant bacterial species in samples from the phyllosphere were Proteobacteria, Cyanobacteria, Actinobacteria, and Bacteroidetes (Supplementary Figure S2A). Cyanobacteria increased from 6.9% (seedling) to 47% (mature), and Actinobacteria decreased from 63% (seedling) to 11% (tiller) (Figure 2A). The five prevailing bacterial phyla found in rhizosphere samples were Proteobacteria, Acidobacteria, Actinobacteria, Chloroflexi, and Planctomycetes, and they were present in greater than 90% of bacterial sequences during plant development stages (Figure 2B). Additionally, there was an increase in the relative abundance of Proteobacteria along with plant development in rhizosphere samples, while the relative abundance decreased in Acidobacteria as plant development continued to progress.
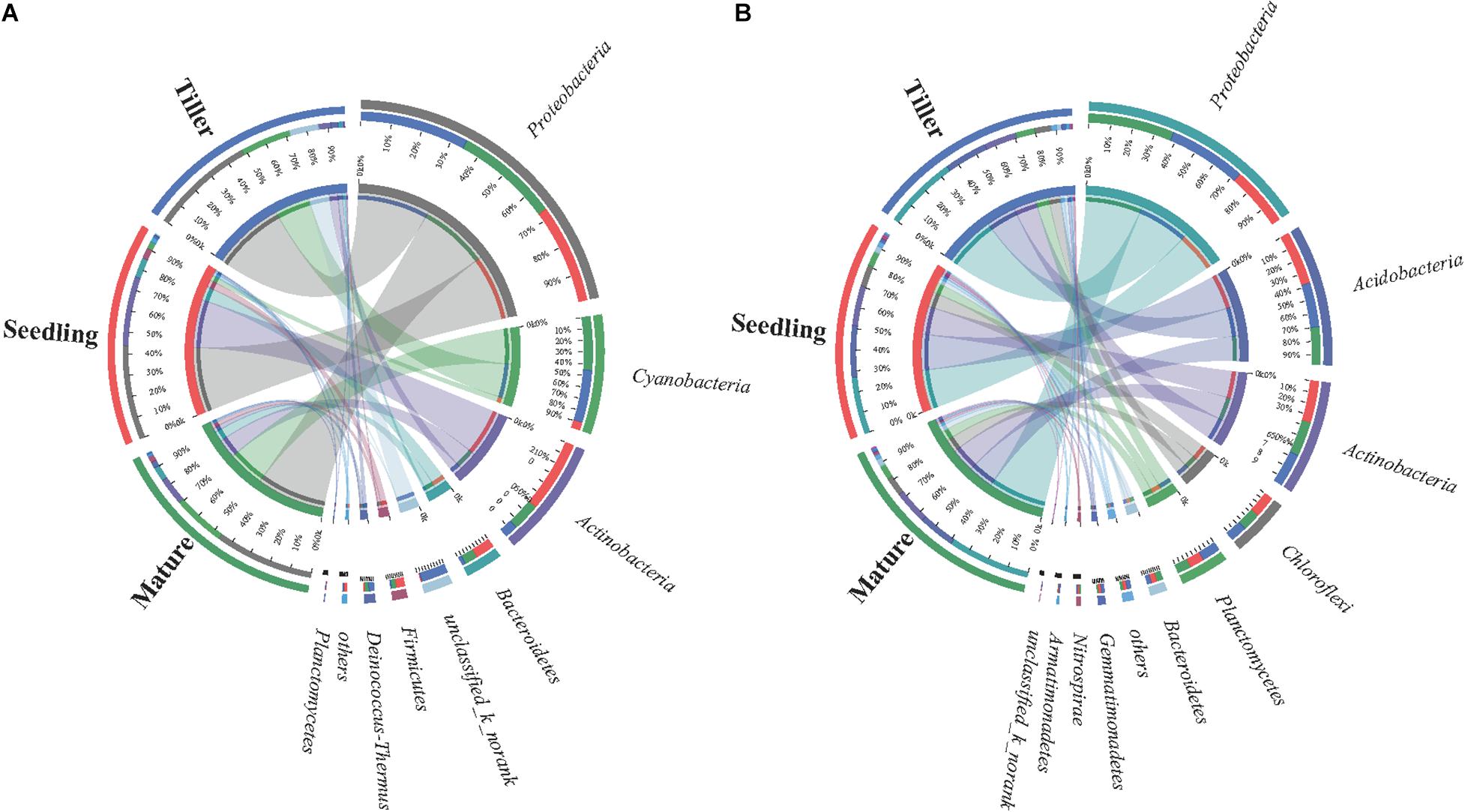
Figure 2. Distribution of phyllosphere (A) and rhizosphere (B) bacterial communities for each sample at phylum level. The width of the bars from each phylum indicate the relative abundance of that phylum in the sample.
The Bray-Curtis distance analysis based on PCA and ANOSIM was conducted to determine the dissimilarity of bacterial communities among each plant development stage (Supplementary Figure S3). The results indicated that the first two axes (PC1 and PC2) explained 40.74 and 42.19%, respectively, of total variation in phyllosphere and rhizosphere bacteria communities during three plant development stages. ANOSIM revealed significant differences in both phyllosphere (Bray–Curtis ANOSIM = 0.885; P = 0.003) and rhizosphere (Bray–Curtis ANOSIM = 0.893; P = 0.006) bacterial community structure among the different plant growth stages.
The top 10 dominant phyla and genera are shown in Figure 3. For the phyllosphere bacterial community, significant differences were found in Actinobacteria, Bacteroidetes, Firmicutes, and Planctomycetes at a phylum level, and significant differences were found in Paracoccus, Microbacterium, and norank_f_Mitochondria at a genus level among the plant development stages (Figures 3A,B). For the rhizosphere bacterial community, Proteobacteria, Acidobacteria, Gemmatimonadetes, and unclassified_k__norank exhibited significant differences at a phylum level, and norank_o_Subgroup_7, RB41, and Pseudarthrobacter were distinct at a genus level among the plant development stages (Figures 3C,D).
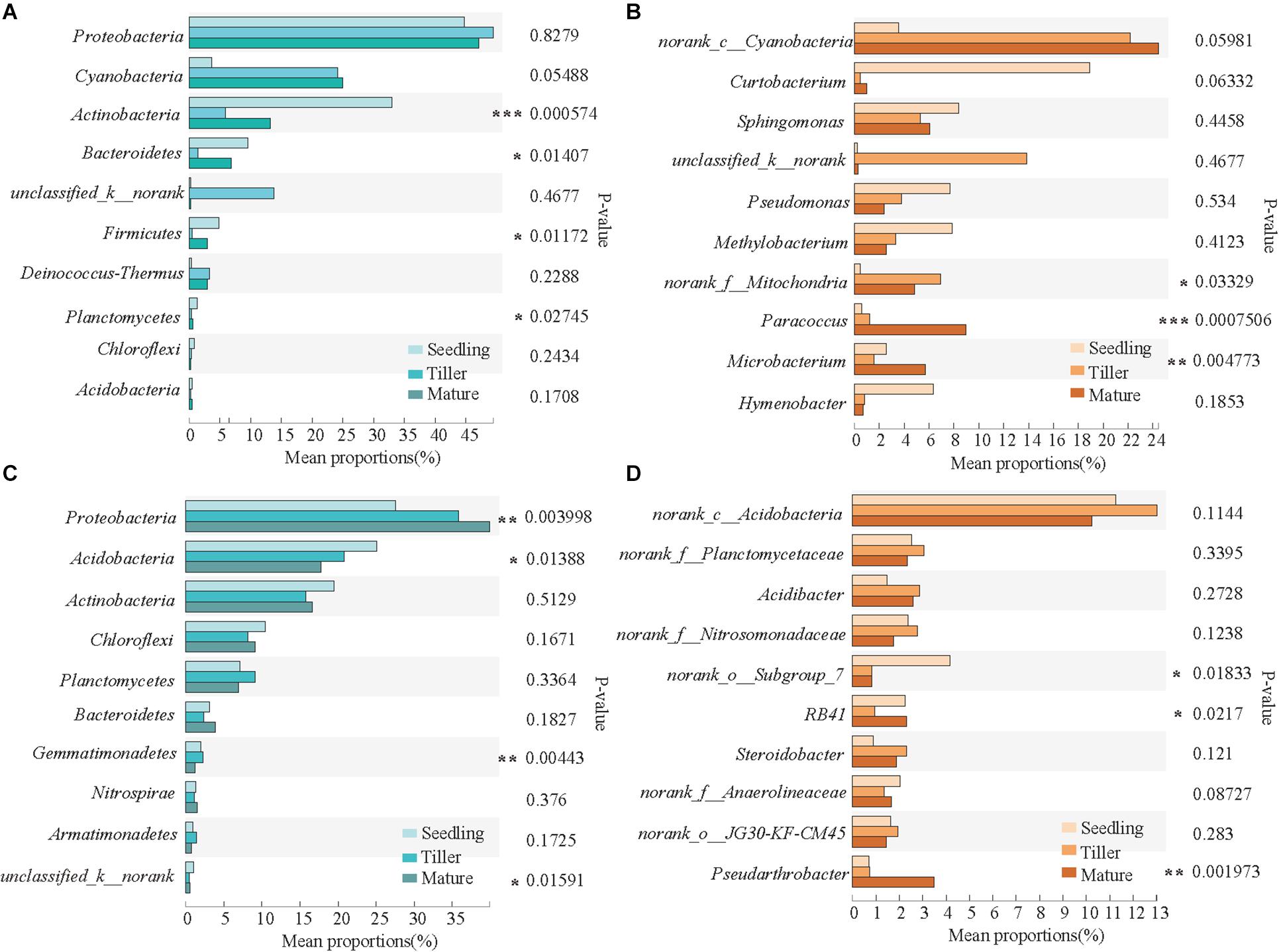
Figure 3. Relative abundances of top 10 bacterial phyla and genera that showed significant differences among phyllosphere (A,B) and rhizosphere (C,D) samples from the seedling, tiller, and mature stages. A one-way ANOVA was used to evaluate the significance of differences between the indicated groups. *P < 0.05; **P < 0.01; and ***P < 0.001.
Relationship Between Bacterial Community Structure and Plant and Soil Characteristics
The experiment evaluated the effect of these ecological factors on the top 10 bacterial phyla in phyllosphere and rhizosphere communities using RDA and found a correlation among plant and soil bacterial community structure and characteristics (Figure 4). Results showed that 76.89% of variation in phyllosphere bacteria could be explained by leaf properties (Figure 4A). Phyllosphere bacterial community structure was significantly affected by plant N (including leaf TN, sheath TN, and root TN) as well as the C/N of leaves and sheaths (Figure 4A). Soil properties could explain 91.79% variability in rhizosphere bacterial community structure (Figure 4B). Six soil characteristics were chosen for RDA after removing redundant variables. As shown in Figure 4B, soil water content (SWC) (P = 0.015), soil TC (P = 0.005), NO4+-N (P = 0.028), C/N (P = 0.003), electrical conductivity (EC) (P = 0.002), and salinity (P = 0.002) significantly affected the bacterial community structure of the rhizosphere. Both the physiological properties of plant species and soil properties had significant effects on phyllosphere (Mantel test, P = 0.008 and r = 0.531) and rhizosphere (Mantel test, P = 0.001 and r = 0.658) bacterial communities, respectively.
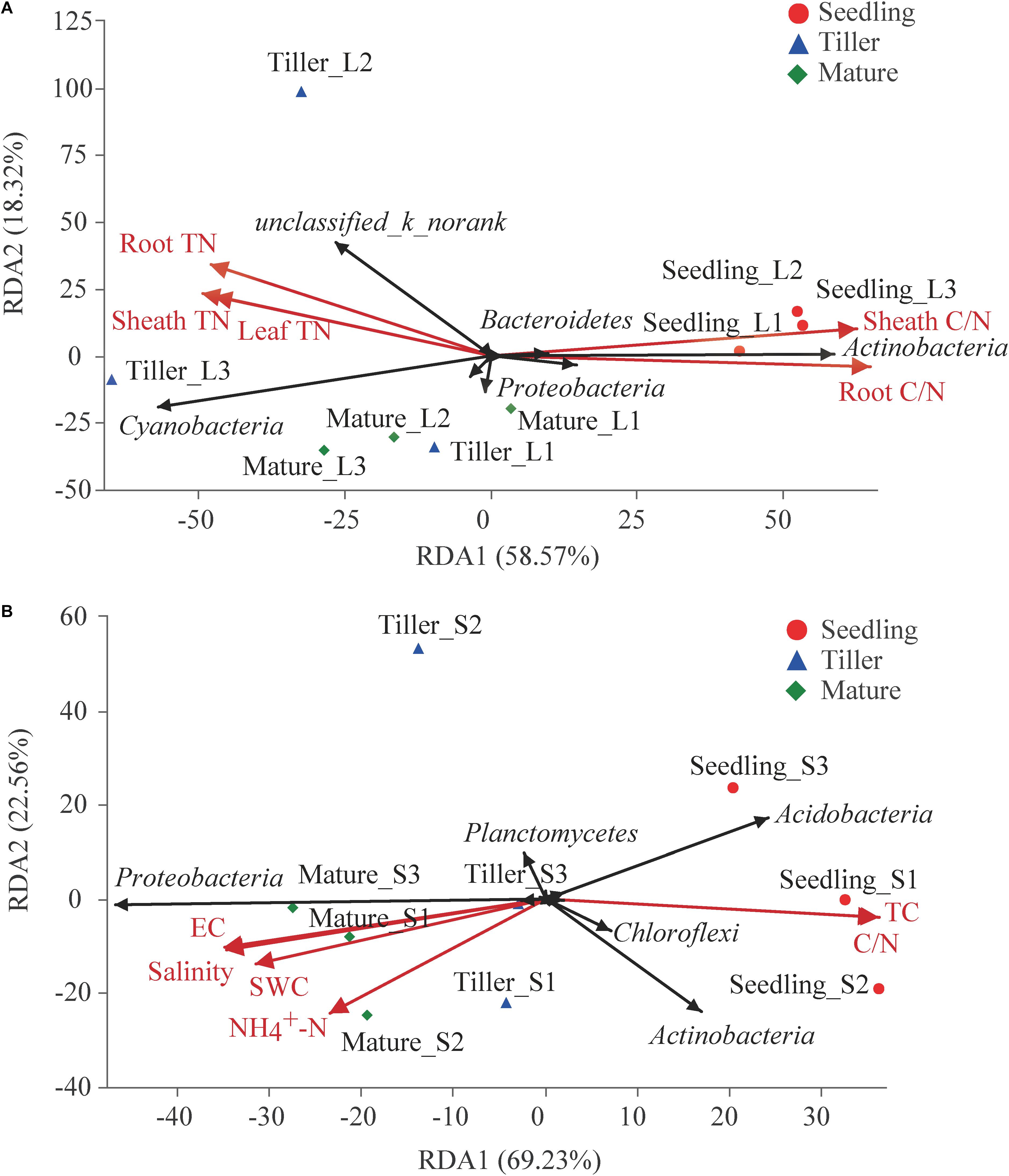
Figure 4. Redundancy analysis (RDA) analysis of the top 10 bacterial phyla and leaf or soil characteristics. Phyllosphere and rhizosphere bacterial communities are shown in (A,B), respectively. The values of axes 1 and 2 are the percentages explained by the corresponding axis.
The correlation heatmap showed that the relationship between bacterial phyla and plant properties or environmental factors differed for the phyllosphere and rhizosphere (Figure 5). For the phyllosphere, the phyla Actinobacteria and Bacteroidetes exhibited significant positive correlations to the C/N of leaves, sheaths, and roots. Moreover, Actinobacteria, Bacteroidetes, and Planctomycetes exhibited extremely significant negative correlations to the TN of leaves and sheaths (Figure 5A). For the rhizosphere bacterial community, the abundance of Proteobacteria is extremely positive correlated to SWC, salinity, and EC, and significant negative correlated to the C/N of soil (Figure 5B). Soil properties and aboveground prosperities were the prominent factors influencing phyllosphere bacterial diversity. Also, root properties affected phyllosphere bacterial diversity, while the impacts of the plant development processes on the rhizosphere bacterial community were not observed (Figure 6).
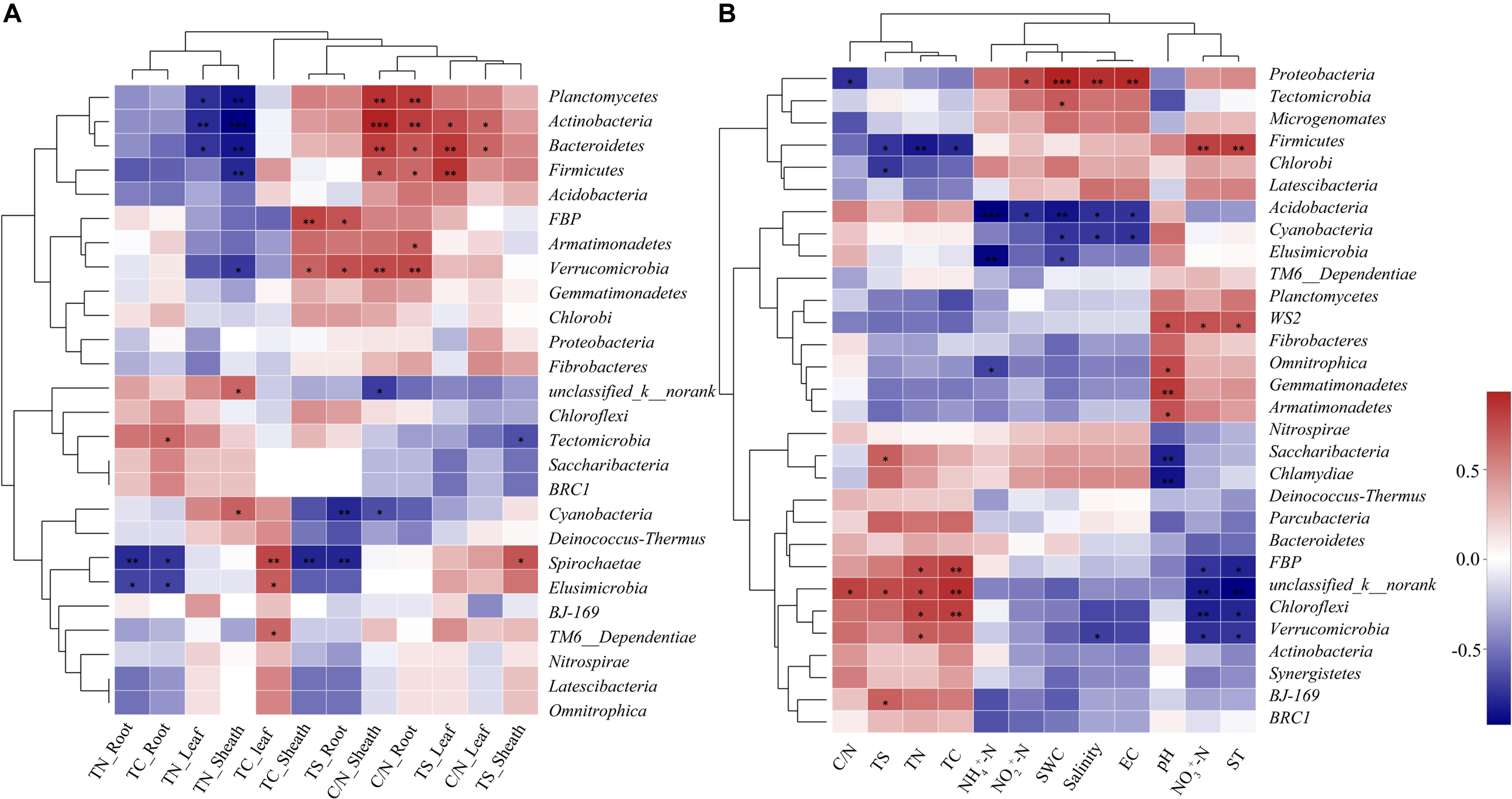
Figure 5. Correlation heat map of the top thirty phyla and soil properties and vegetation. X and Y axis are environmental factors and phyla. R in different colors to show, the right side of the legend is the color range of different r values. *P < 0.05; **P < 0.01; and ***P < 0.001. Phyllosphere and rhizosphere bacterial communities are shown in (A, B), respectively.
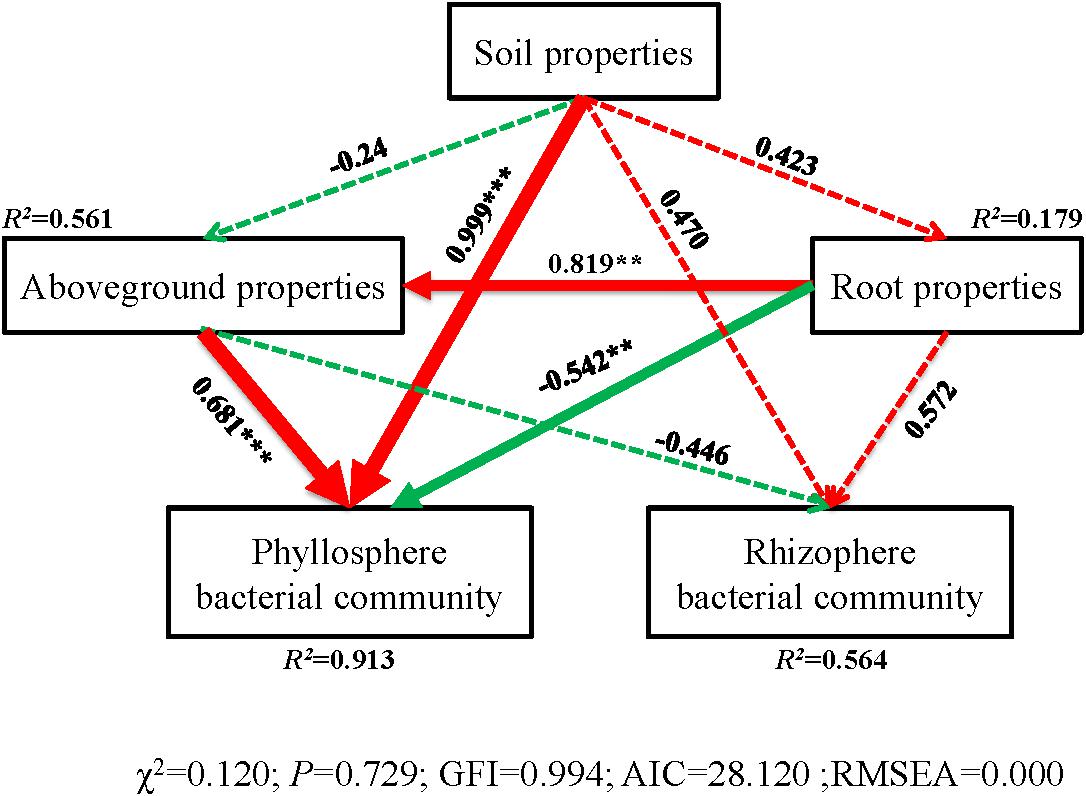
Figure 6. Structural equation model (SEM) illustrating the effects of soil properties on physicochemical characteristics (aboveground and root) and bacterial communities of phyllosphere and rhizophere. Continuous and dashed arrows represent the significant and non-significant relationships, respectively. Adjacent number that are labeled in the same direction as the arrow represents path coefficients, and the width of the arrow is in proportion to the degree of path coefficients. Green and red arrows indicate positive and negative relationships, respectively. R2 values indicate the proportion of variance explained by each variable. Significance levels are denoted with **P < 0.01 and ***P < 0.001. Standardized total effects (direct plus indirect effects) calculated by the SEM are displayed below the SEM. The low chi-square (χχ2), nonsignificant non-significant probability level (P > 0.05), high goodness-of-fit index (GFI > 0.90), low Akaike information criteria (AIC), and low root-mean-square errors of approximation (RMSEA < 0.05) listed below the SEMs indicate that our data matches the hypothetical models.
The phyllosphere bacterial network in this study consisted of 282 nodes and 2,319 edges, and the network diameter and density were 10 and 0.059, respectively (Figure 7). The rhizosphere bacterial network consisted of 315 nodes and 2,845 edges, and the network diameter and density were 7 and 0.057, respectively. Both indicated that the coexistence among bacterial communities was greater than their exclusion in the phyllosphere (99.74%) and the rhizosphere (99.4%). Bacteroidetes, Chloroflexi, and Proteobacteria were the key bacterial phyla in the phyllosphere, while Actinobacteria, Bacteroidetes, and Chlorobi played critical roles in the rhizosphere (Table 3). Bacteroidaceae and Microbacteriaceae yielded the highest betweenness centrality (BC) values within the rhizosphere bacterial network, while they were also critical in maintaining the structure and function of the bacterial communities (Figure 7).
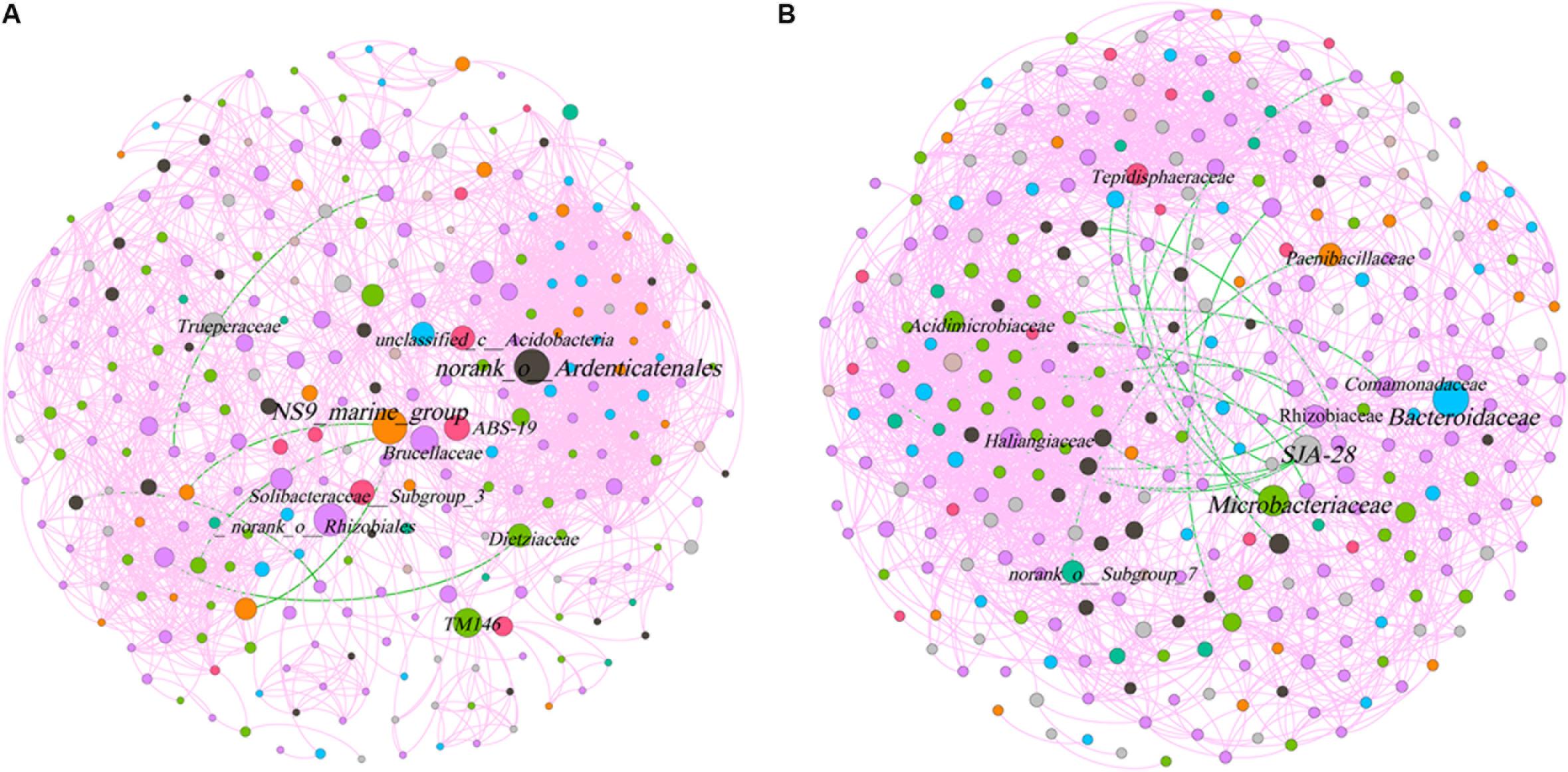
Figure 7. Co-occurrence network of microbial taxa on phyllosphere (A) and rhizosphere (B). Nodes represent bacteria families, whereas pink and green edges, respectively, represent positive and negative connections between pairs of species.
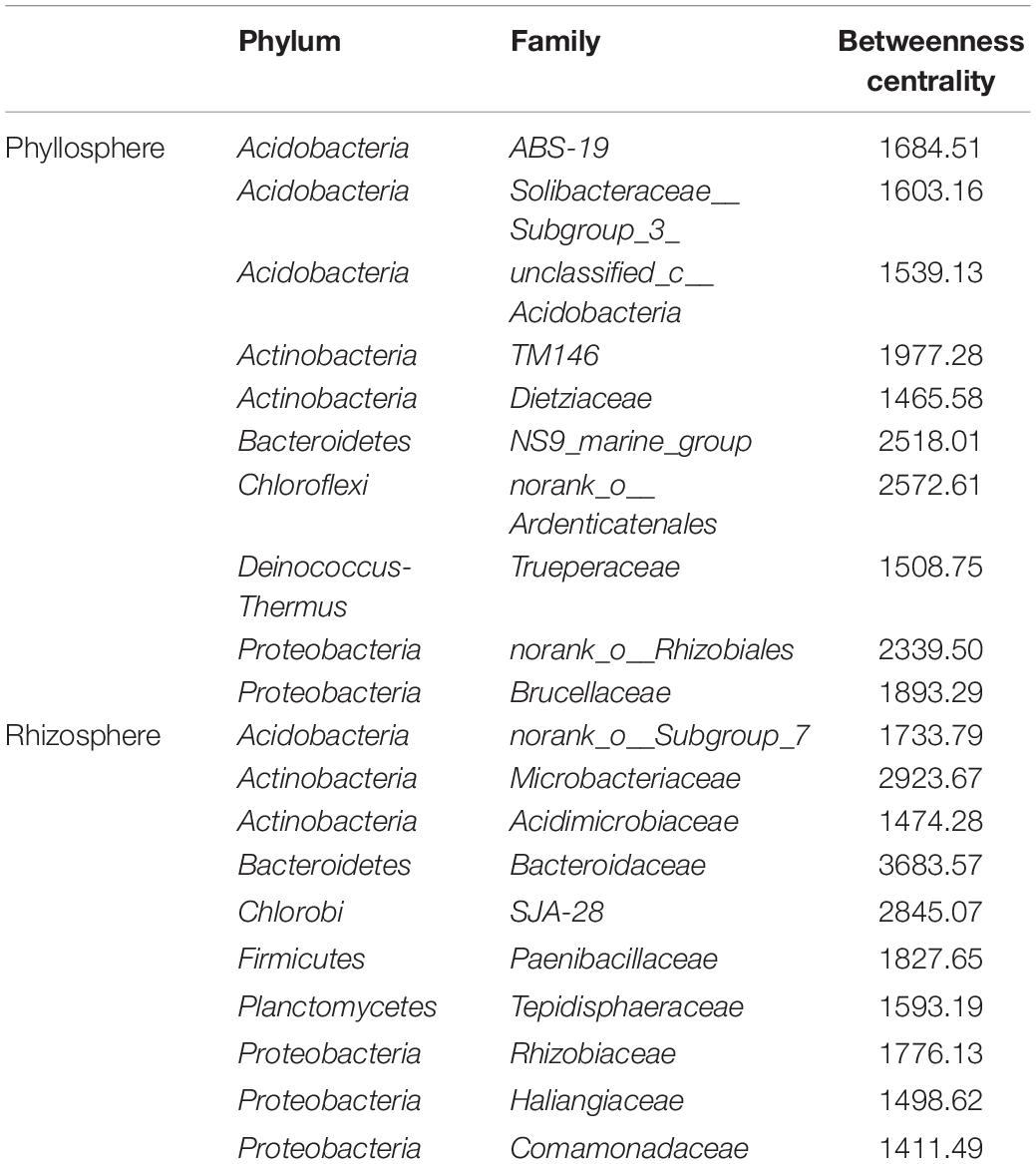
Table 3. Dominant keystone species (top 10) from phyllosphere and rhizosphere bacterial community structures.
Functional Features of Phyllosphere and Rhizosphere Bacterial Communities
We used the PICRUSt10 program to better understand the important role that bacteria play in the phyllosphere and rhizosphere, and we obtained a microbial COG profile and analyzed functional microbial features during three plant development stages (Supplementary Figure S4). The microbiomes exhibited distinct functional features associated with phyllosphere and rhizosphere bacterial communities. These functional features included amino acid transport and its metabolic processes, energy production and conversion, transcription, nucleotide transport and its metabolic processes, and carbohydrate transport and its metabolic processes (Supplementary Figure S4).
Discussion
This study investigated the dynamic relationship of phyllosphere and rhizosphere bacterial communities during the plant development. The relative abundance and Shannon indices showed that rhizosphere bacterial communities during the seedling and tiller stages were significantly more diverse than those during the mature stage, while changes in the Simpson index had the opposite effect (Figure 1). These results agreed with previous studies, namely, that the rhizosphere microbial community of Arabidopsis differed significantly during the seedling stage compared to the other two stages (Chaparro et al., 2014). There were no significant differences in the relative abundance and diversity of the phyllosphere bacterial community during these three stages (Figure 1). Similarly, we observed no distinguishable trends in patterns of change in bacterial diversity during plant growth stages, and this is consistent with a report from a previous study (Redford and Fierer, 2009), demonstrating that there were no discernible patterns of change in phyllosphere bacterial diversity. For phyllosphere microbiomes of trees or perennial plant species, less migration from soil could simply be the result of distance or the longer time required for the host to adapt (Kim et al., 2012).
The dominant phyllosphere bacterial phyla varied among the plant growth stages, but the dominant bacteria during the three plant development stages were similar in the rhizosphere (Supplementary Figure S2). This was consistent with results from earlier studies on other plant species deduced from pyrosequencing, such as lettuce (Rastogi et al., 2012), fresh spinach (Lopezvelasco et al., 2011), and tree species (Redford et al., 2010). The role of Bacteroidetes in the phyllosphere has yet to be clarified although one study has indicated that it is critical to nutrient turnover (Yousuf et al., 2012). Moreover, bacterial species that belong to the Bacteroidetes phylum comprise of genes associated with denitrification, suggesting a potential role in N cycling (Spanning et al., 2005). During the seedling stage, Cyanobacteria and Actinobacteria showed different dynamic change trends. Recently, Actinobacteria has been correlated to disease suppressive soils (Rodrigo et al., 2011). Moreover, Cyanobacteria is known to colonize plant roots (Gantar et al., 2010; Lundberg et al., 2012), which can encourage plant growth (Prasanna et al., 2009). Meanwhile, Cyanobacteria, owing to its N-fixation ability, are a key source of inorganic N for plants (Franche et al., 2009). In future studies, Cyanobacteria and Actinobacteria could be inoculated in the process of bioremediation, and studied for their effects on plant growth and physiology, so as to better restore contaminated sites via a combined microbial and plant interaction.
The predominant bacterial components of samples during the plant development stages were similar to that of the rhizosphere (Supplementary Figure S2B). In contrast to previous studies, our more detailed examination of rhizosphere microbial communities during plant development stages revealed the formation of a central microbiome composed of bacteria from various phyla (Actinobacteria, Bacteroidetes, Proteobacteria, Chloroflexi, Firmicutes, Gemmatimonadetes, Nitrospirae, Planctomycetes, and Verrucomicrobia) (Bulgarelli et al., 2012; Lundberg et al., 2012), and Actinobacteria was observed to significantly change during plant development (Chaparro et al., 2014). Proteobacteria was the prevailing bacterial phylum found in both the phyllosphere and rhizosphere, which indicated that members of this phylum could be involved in both the physiological and the ecological development of B. ischaemum in contaminated environments. One study reported that Proteobacteria was the most stress-tolerant phylum under conditions of heavy soil contamination (Eva-Maria et al., 2011). It has also been suggested that numerous heavy metal oxidase genes encoded by Proteobacteria resist heavy metal contamination (Altimira, 2012). Futhermore, Proteobacteria has previously exhibited considerable diversity in morphology, physiology, and metabolic processes (Zavarzin et al., 1991), suggesting that this phylum can adapt to different phyllosphere environments by physiological and metabolic regulation. For the rhizosphere bacterial community, Proteobacteria and Acidobacteria exhibited significant differences at a phylum level (Figure 3). It is accepted among researchers that plant species can adopt a microbial subset at various growth stages. The role of Acidobacteria, an abundant bacterial phylum (Barns et al., 1999), is important in C cycling, because of its capacity in degrading complex polysaccharides, such as cellulose and lignin, derived from plants (Ward et al., 2009). Moreover, these results reinforced findings from previous studies that reported that temporal variability in phyllosphere and rhizosphere bacterial community composition was not random. During the different growth stages, variation in the structure and composition of bacterial communities can be explained to some extent by differences in abiotic environments, including climate conditions and plant and soil chemical characteristics.
During the three plant stages, phyllosphere and rhizosphere bacterial communities separately correlated to plant and soil chemical characteristics (Figure 4). Phyllosphere bacterial community structure was significantly affected by plant N, which implied that the effects of N on the bacterial community were variable and likely stage dependent (Fierer et al., 2012). The structure of microbiota is typically shaped by abiotic and biotic environmental variables. Soil properties, aboveground and root prosperities were the prominent factors influencing phyllosphere bacterial diversity (Figure 6). Moreover, taxa that can take advantage of a variety or a specific subset of carbohydrates will thrive in the phyllosphere as indicated by the enrichment of carbohydrate transport and the metabolism of gene families. This could be caused by environmental conditions under which B. ischaemum is grown, namely, geographic characteristics and climatic parameters. It has been reported that Enterobacter had a negative impact on the Erwinia bacteria group during phyllosphere colonization (Hunter et al., 2010).
Keystone microbes can be generally defined as those species that have a disproportionate influence on ecosystems regardless of abundance, and they are crucial in the maintenance of the stability and the function of ecosystems as well as the resistance of system disturbances (Lupatini et al., 2014; Zhang et al., 2018). Some keystone families were identified from co-occurrence network, demonstrating the dynamics of the relationships between phyllosphere and rhizosphere bacterial communities during different stages of plant development (Figure 7). Moreover, it has been confirmed that certain taxa of low abundance paradoxically play a disproportionate role in regulating ecological functions in various habitats (Zhang et al., 2018). Keystone microbes exhibited significant differences between phyllosphere and rhizosphere bacterial communities. The key to comprehending the development of bacterial leaf communities in environments under stress is to understand the symbiotic relationships that exist between microbes that have successfully adapted to their host plants as well as plant health development (Andrews, 1992).
It is important to note that any alteration to either bacterial community structure or composition will likely have functional consequences (Peñuelas et al., 2012). Furthermore, enrichment in metabolic functions was observed in the phyllosphere and rhizosphere, which implied that microbial (bacterial) metabolism tended to be vigorous. The role of both phyllosphere and rhizosphere microbiomes are vital to plant productivity and health, being also referred to as the “second” genome of plant species (Berendsen et al., 2012; Chaparro et al., 2014). The phyllosphere and rhizosphere bacterial communities had distinct functional features. This indicated that phyllosphere and rhizosphere microbial communities select specific functions throughout plant development (Chaparro et al., 2014). These results shed new light on understanding the dynamic relationship of phyllosphere and rhizosphere bacterial communities during the development of B. ischaemum in a pollution environment. They are beneficial to the development and utilization of beneficial microbial communities at different stages of development, which might help to reclaim and stabilize tailings more effectively.
Data Availability Statement
The bacterial sequences have been deposited in the SRA of the NCBI database under the SRA accession: PRJNA600330.
Author Contributions
TJ conceived and designed the experiments. RW and YY performed the experiments. BC contributed new reagents. TJ and TW wrote the manuscript. All authors read and approved the manuscript.
Funding
This study was supported by the National Natural Science Foundation of China (Grant No. 31600308), the fund for Shanxi “1331 Project,” China (Ecological restoration of damaged soil system), Shanxi Province Science Foundation for Excellent Young Scholars (Grant No. 201901D211196), Scientific and Technological Innovation Programs of Higher Education Institutions in Shanxi (Grant No. 2019L0005), Shanxi Province Graduate Education Innovation Project (Grant No. 2019SY029), Shanxi Province Foundation for Returnees (Grant No. 2016-006), and the Higher Education Institution Project of Shanxi Province: Ecological Remediation of Soil Pollution Disciplines Group (Grant No. 20181401).
Conflict of Interest
The authors declare that the research was conducted in the absence of any commercial or financial relationships that could be construed as a potential conflict of interest.
Supplementary Material
The Supplementary Material for this article can be found online at: https://www.frontiersin.org/articles/10.3389/fmicb.2020.00869/full#supplementary-material
References
Altimira, F. (2012). Characterization of copper-resistant bacteria and bacterial communities from copper-polluted agricultural soils of central Chile. BMC Microbiol. 12:193. doi: 10.1186/1471-2180-12-193
Balintkurti, P., Simmons, S. J., Blum, J. E., Ballaré, C. L., and Stapleton, A. E. (2010). Maize leaf epiphytic bacteria diversity patterns are genetically correlated with resistance to fungal pathogen infection. Mol. Plant Microbe Int. 23, 473–484. doi: 10.1094/MPMI-23-4-0473
Barns, S. M., Takala, S. L., and Kuske, C. R. (1999). Wide distribution and diversity of members of the bacterial kingdom Acidobacterium in the environment. Appl. Environ. Microb. 65, 1731–1737.
Bastian, M., Heymann, S., and Jacomy, M. (2009). “Gephi: an open source software for exploring and manipulating networks,” in Proceedings of the Third International ICWSM Conference, Menlo Park, CA.
Berendsen, R. L., Pieterse, C. M. J., and Bakker, P. A. H. M. (2012). The rhizosphere microbiome and plant health. Trends Plant Sci. 17, 478–486. doi: 10.1016/j.tplants.2012.04.001
Bodenhausen, N., Horton, M. W., and Bergelson, J. (2013). Bacterial communities associated with the leaves and the roots of Arabidopsis thaliana. PLoS One 8:e56329. doi: 10.1371/journal.pone.0056329
Bulgarelli, D., Rott, M., Schlaeppi, K., Themaat, E. V. L. V., Ahmadinejad, N., Assenza, F., et al. (2012). Revealing structure and assembly cues for Arabidopsis root-inhabiting bacterial microbiota. Nature 488, 91–95. doi: 10.1038/nature11336
Chaparro, J. M., Badri, D. V., and Vivanco, J. M. (2014). Rhizosphere microbiome assemblage is affected by plant development. ISME J. 8, 790–803. doi: 10.1038/ismej.2013.196
Chinnadurai, C., Balachandar, D., and Sundaram, S. P. (2009). Characterization of 1-aminocyclopropane-1-carboxylate deaminase producing methylobacteria from phyllosphere of rice and their role in ethylene regulation. World J. Microb. Biot. 25, 1403–1411.
Conesa, H. M., Faz, A., and Arnaldos, R. (2007). Initial studies for the phytostabilization of a mine tailing from the cartagena-la union mining district (SE Spain). Chemosphere 66, 38–44. doi: 10.1016/j.chemosphere.2006.05.041
Costa, D. M. D., Samarasinghe, S. S. T., Dias, H. R. D., and Dissanayake, D. M. N. (2008). Control of rice sheath blight by phyllosphere epiphytic microbial antagonists. Phytoparasitica 36, 52–65.
Costa, L. E. D. O., Queiroz, M. V. D., Borges, A. C., Moraes, C. A. D., and Araújo, E. F. D. (2012). Isolation and characterization of endophytic bacteria isolated from the leaves of the common bean (Phaseolus vulgaris). Braz. J. Microbiol. 43, 1562–1575. doi: 10.1590/S1517-838220120004000041
Eva-Maria, B., Sebastian, B., Akob, D. M., Georg, B., and Kirsten, K. (2011). Heavy metal tolerance of Fe(III)-reducing microbial communities in contaminated creek bank soils. Appl. Environ. Microb. 77, 3132. doi: 10.1128/AEM.02085-10
Fierer, N., Lauber, C. L., Ramirez, K. S., Zaneveld, J., Bradford, M. A., and Knight, R. (2012). Comparative metagenomic, phylogenetic and physiological analyses of soil microbial communities across nitrogen gradients. ISME J. 6, 1007–1017. doi: 10.1038/ismej.2011.159
Finkel, O. M., Burch, A. Y., Lindow, S. E., Post, A. F., and Shimshon, B. (2011). Geographical location determines the population structure in phyllosphere microbial communities of a salt-excreting desert tree. Appl. Environ. Microb. 77:7647. doi: 10.1128/AEM.05565-11
Franche, C., Lindström, K., and Elmerich, C. (2009). Nitrogen-fixing bacteria associated with leguminous and non-leguminous plants. Plant Soil 321, 35–59.
Gantar, M., Kerby, N. W., Rowell, P., and Obreht, Z. (2010). Colonization of Wheat (Triticum vulgare L.) by N2-fixing cyanobacteria: I. A survey of soil cyanobacterial isolates forming associations with roots. New Phytol. 118, 477–483.
Hardoim, P. R., Hardoim, C. C., van Overbeek, L. S., and van Elsas, J. D. (2012). Dynamics of seed-borne rice endophytes on early plant growth stages. PLoS One 7:e30438. doi: 10.1371/journal.pone.0030438
He, Z., Xie, X., Xiao, S., Liu, J., and Qiu, G. (2007). Microbial diversity of mine water at Zhong Tiaoshan copper mine, China. J. Basic Microbiol. 47, 485–495. doi: 10.1002/jobm.200700219
Hunter, P. J., Paul, H., David, P., Whipps, J. M., and Bending, G. D. (2010). Both leaf properties and microbe-microbe interactions influence within-species variation in bacterial population diversity and structure in the lettuce (Lactuca Species) phyllosphere. Appl. Environ. Microb. 76, 8117–8125. doi: 10.1128/AEM.01321-10
Jackson, C. R., and Denney, W. C. (2011). Annual and seasonal variation in the phyllosphere bacterial community associated with leaves of the southern magnolia (Magnolia grandiflora). Microb. Ecol. 61, 113–122. doi: 10.1007/s00248-010-9742-2
Janarthine, S. R. S., and Eganathan, P. (2012). Plant growth promoting of endophytic Sporosarcina aquimarina SjAM16103 isolated from the pneumatophores of Avicennia marina L. Int. J. Microbiol. 2012:532060. doi: 10.1155/2012/532060
Jia, T., Cao, M., Jing, J., Liu, J., and Chai, B. (2017). Endophytic fungi and soil microbial community characteristics over different years of phytoremediation in a copper tailings dam of Shanxi, China. Sci. Total Environ. 574, 881–888. doi: 10.1016/j.scitotenv.2016.09.161
Jia, T., Guo, T., Cao, M., and Chai, B. (2018). Effects of heavy metals on phyllosphere and rhizosphere microbial community of Bothriochloa ischaemum. Appl. Sci. Basel 8:1419.
Kim, M., Singh, D., Lai-Hoe, A., Go, R., Rahim, R. A., Ainuddin, A. N., et al. (2012). Distinctive phyllosphere bacterial communities in tropical trees. Microb. Ecol. 63, 674–681. doi: 10.1007/s00248-011-9953-1
Krzywinski, M., and Schein, J. I. (2009). Circos: an information aesthetic for comparative genomics. Genome Res. 19, 1639–1645. doi: 10.1101/gr.092759.109
Lazaridou, E., Giannopoulou, C., Fotiadou, C., Vakirlis, E., Trigoni, A., and Ioannides, D. (2011). The potential role of microorganisms in the development of rosacea. J. Dtsch. Dermatol. Gestrol. 9, 21–25. doi: 10.1111/j.1610-0387.2010.07513.x
Lindow, S. E., and Brandl, M. T. (2003). Microbiology of the phyllosphere. Appl. Environ. Microb. 69, 1875–1883.
Lopezvelasco, G., Welbaum, G. E., Boyer, R. R., Mane, S. P., and Ponder, M. A. (2011). Changes in spinach phylloepiphytic bacteria communities following minimal processing and refrigerated storage described using pyrosequencing of 16S rRNA amplicons. J. Appl. Microbiol. 110, 1203–1214. doi: 10.1111/j.1365-2672.2011.04969.x
Lundberg, D. S., Lebeis, S. L., Sur Herrera, P., Scott, Y., Jase, G., Stephanie, M., et al. (2012). Defining the core Arabidopsis thaliana root microbiome. Nature 488:86. doi: 10.1038/nature11237
Lupatini, M., Suleiman, A. K. A., Jacques, R. J. S., Antoniolli, Z. I., de Siqueira Ferreira, A. O., Kuramae, E. E., et al. (2014). Network topology reveals high connectance levels and few key microbial genera within soils. Front. Environ. Sci. 2:10. doi: 10.3389/fenvs.2014.00010
Marie-Pierre, D., and Eric, G. (2010). Resistance to pathogens and host developmental stage: a multifaceted relationship within the plant kingdom. New Phytol. 175, 405–416. doi: 10.1111/j.1469-8137.2007.02130.x
Micallef, S. A., Sheridon, C., Shiaris, M. P., and Adán, C. C. (2009). Plant age and genotype impact the progression of bacterial community succession in the Arabidopsis rhizosphere. Plant Signal. Behav. 4, 777–780. doi: 10.1093/jxb/erp053
Peñuelas, J., Rico, L., Ogaya, R., Jump, A. S., and Terradas, J. (2012). Summer season and long-term drought increase the richness of bacteria and fungi in the foliar phyllosphere of Quercus ilex in a mixed Mediterranean forest. Plant Biol. 14, 565–575. doi: 10.1111/j.1438-8677.2011.00532.x
Perazzolli, M., Antonielli, L., Storari, M., Puopolo, G., Pancher, M., Giovannini, O., et al. (2014). Resilience of the natural phyllosphere microbiota of the grapevine to chemical and biological pesticides. Appl. Environ. Microbiol. 80, 3585–3596. doi: 10.1128/AEM.00415-14
Prasanna, R., Jaiswal, P., Nayak, S., Sood, A., and Kaushik, B. D. (2009). Cyanobacterial diversity in the rhizosphere of rice and its ecological significance. Indian J. Microbiol. 49:89. doi: 10.1007/s12088-009-0009-x
Raaijmakers, J. M., Paulitz, T. C., Steinberg, C., Alabouvette, C., and Moënneloccoz, Y. (2009). The rhizosphere: a playground and battlefield for soilborne pathogens and beneficial microorganisms. Plant Soil 321, 341–361.
Rastogi, G., Sbodio, A., Tech, J. J., Suslow, T. V., Coaker, G. L., and Leveau, J. H. J. (2012). Leaf microbiota in an agroecosystem: spatiotemporal variation in bacterial community composition on field-grown lettuce. ISME J. 6:1812. doi: 10.1038/ismej.2012.32
Redford, A. J., Bowers, R. M., Knight, R., Yan, L., and Fierer, N. (2010). The ecology of the phyllosphere: geographic and phylogenetic variability in the distribution of bacteria on tree leaves. Environ. Microbiol. 12, 2885–2893. doi: 10.1111/j.1462-2920.2010.02258.x
Redford, A. J., and Fierer, N. (2009). Bacterial succession on the leaf surface: a novel system for studying successional dynamics. Microb. Ecol. 58, 189–198. doi: 10.1007/s00248-009-9495-y
Ren, G., Zhang, H., Lin, X., Zhu, J., and Jia, Z. (2014). Response of phyllosphere bacterial communities to elevated CO2 during rice growing season. Appl. Microbiol. Biot. 98, 9459–9471. doi: 10.1007/s00253-014-5915-0
Rodrigo, M., Marco, K., Irene, D. B., Ester, D., Menno, V. D. V., Schneider, J. H. M., et al. (2011). Deciphering the rhizosphere microbiome for disease-suppressive bacteria. Science 332, 1097–1100. doi: 10.1126/science.1203980
Spanning, R., Delgado, M. J., and Richardson, D. J. (2005). The Nitrogen Cycle: Denitrification and Its Relationship to N2 Fixation. Nitrogen Fixation in Agriculture, Forestry, Ecology, and the Environment. Netherlands: Springer.
Ward, N. L., Challacombe, J. F., Janssen, P. H., Bernard, H., Coutinho, P. M., Martin, W., et al. (2009). Three genomes from the phylum Acidobacteria provide insight into the lifestyles of these microorganisms in soils. Appl. Environ. Microb. 75;2046. doi: 10.1128/AEM.02294-08
Wong, M. H. (2003). Ecological restoration of mine degraded soils, with emphasis on metal contaminated soils. Chemosphere 50, 775–780. doi: 10.1016/s0045-6535(02)00232-1
Yousuf, B., Keshri, J., Mishra, A., and Jha, B. (2012). Application of targeted metagenomics to explore abundance and diversity of CO2 -fixing bacterial community using cbbL gene from the rhizosphere of Arachis hypogaea. Gene 506, 18–24. doi: 10.1016/j.gene.2012.06.083
Zavarzin, G. A., Stackebrandt, E., and Murray, R. G. (1991). A correlation of phylogenetic diversity in the Proteobacteria with the influences of ecological forces. Can. J. Microbiol. 37, 1–6. doi: 10.1139/m91-001
Zgül, I. L., Waleed Abu, A., Joana Falc, O. S., Alexander, V. S., and Jan Dirk, V. E. (2011). Comparative analysis of bacterial communities in a potato field as determined by pyrosequencing. PLoS One 6:e23321. doi: 10.1371/journal.pone.0023321
Zhang, S., Zhou, Z., Li, Y., and Meng, F. (2018). Deciphering the core fouling-causing microbiota in a membrane bioreactor: low abundance but important roles. Chemosphere 195, 108–118. doi: 10.1016/j.chemosphere.2017.12.067
Keywords: Bothriochloa ischaemum, plant development, phyllosphere bacteria, rhizosphere bacteria, copper tailings dam
Citation: Jia T, Yao Y, Wang R, Wu T and Chai B (2020) Dynamics Relationship of Phyllosphere and Rhizosphere Bacterial Communities During the Development of Bothriochloa ischaemum in Copper Tailings. Front. Microbiol. 11:869. doi: 10.3389/fmicb.2020.00869
Received: 22 January 2020; Accepted: 14 April 2020;
Published: 28 May 2020.
Edited by:
Lei Chen, Tianjin University, ChinaReviewed by:
Guy Lanza, SUNY College of Environmental Science and Forestry, United StatesSanin Musovic, Danish Technological Institute (DTI), Denmark
Copyright © 2020 Jia, Yao, Wang, Wu and Chai. This is an open-access article distributed under the terms of the Creative Commons Attribution License (CC BY). The use, distribution or reproduction in other forums is permitted, provided the original author(s) and the copyright owner(s) are credited and that the original publication in this journal is cited, in accordance with accepted academic practice. No use, distribution or reproduction is permitted which does not comply with these terms.
*Correspondence: Tong Jia, amlhdG9uZ0BzeHUuZWR1LmNu