- 1Institute of Plant Biology, Biological Research Centre, Szeged, Hungary
- 2Department of Medical Chemistry, Faculty of Medicine, University of Szeged, Szeged, Hungary
- 3Institute of Biochemistry, Biological Research Centre, Szeged, Hungary
- 4Department of Biotechnology, Faculty of Science and Informatics, University of Szeged, Szeged, Hungary
- 5MTA-SZTE Biomimetic Systems Research Group, University of Szeged, Szeged, Hungary
- 6Institute of Biophysics, Biological Research Centre, Szeged, Hungary
- 7Institute of Molecular Biology, Biocenter, Medical University of Innsbruck, Innsbruck, Austria
Because of enormous crop losses worldwide due to pesticide-resistant plant pathogenic fungi, there is an increasing demand for the development of novel antifungal strategies in agriculture. Antifungal proteins (APs) and peptides are considered potential biofungicides; however, several factors limit their direct agricultural application, such as the high cost of production, narrow antifungal spectrum, and detrimental effects to plant development and human/animal health. This study evaluated the safety of the application of APs and peptides from the ascomycete Neosartorya fischeri as crop preservatives. The full-length N. fischeri AP (NFAP) and novel rationally designed γ-core peptide derivatives (PDs) γNFAP-opt and γNFAP-optGZ exhibited efficacy by inhibiting the growth of the agriculturally relevant filamentous ascomycetes in vitro. A high positive net charge, however, neither the hydrophilicity nor the primary structure supported the antifungal efficacy of these PDs. Further testing demonstrated that the antifungal activity did not require a conformational change of the β-pleated NFAP or the canonically ordered conformation of the synthetic PDs. Neither hemolysis nor cytotoxicity was observed when the NFAP and γNFAP-opt were applied at antifungally effective concentrations in human cell lines. Similarly, the Medicago truncatula plants that served as toxicity model and were grown from seedlings that were treated with NFAP, γNFAP-opt, or γNFAP-optGZ failed to exhibit morphological aberrations, reduction in primary root length, or the number of lateral roots. Crop protection experiments demonstrated that NFAP and associated antifungal active γ-core PDs were able to protect tomato fruits against the postharvest fungal pathogen Cladosporium herbarum.
Introduction
The delicate balance between the demand for food of the increasing world population and global agricultural production is easily disturbed by several different factors that cause enormous crop losses resulting in serious economic and societal impacts (Savary et al., 2012). One of these factors is postharvest plant pathogenic fungi. Fungi threaten the global food supply every year as they are destructive pathogens of agriculturally important plants in fields and spoilage agents of crops during storage (Almeida et al., 2019). Based on the available data from 2010, it is estimated that the amount of the five most important crops (viz., rice, wheat, maize, potato, and soybean) destroyed by fungal infection or contamination that year would be enough to feed 8.5% of the world’s population and would support increasing global calorie consumption for decades (Fisher et al., 2012). In the 20th century, the main strategy for elevating productivity was breeding high yield or fungal pathogen-resistant cultivars in parallel with the application of chemical fungicides in the fields (Savary et al., 2012). Despite these efforts, agricultural fungal damage has steadily been increasing over the last few decades (Fisher et al., 2012). This is likely because fungal pathogens adapt easily to resistant cultivars or quickly develop resistance against novel chemical fungicides (McDonald and Stukenbrock, 2016). Climate change (Elad and Pertot, 2014), global trade, and the transport of agricultural products (Jeger et al., 2011) are facilitating the rapid dispersion of highly virulent and fungicide-resistant plant pathogens or foodborne strains globally. This problem is further exacerbated by the fact that very few new fungicides have been introduced to the global market recently. High research cost, coupled with the rapid emergence of resistant strains, is discouraging investment in the development of new fungicides (Borel, 2017). The application of traditional chemical pesticides in the fields and during storage is still the most common plant and crop protection strategy despite the abovementioned disadvantages (Bardin et al., 2015). Therefore, substantial demand exists for the discovery of novel, cost-effective, and potent fungicides or crop preservatives with a lower risk of developing resistance.
The features of the intensively studied extracellular, small, cysteine-rich, and cationic antifungal proteins (APs) from ascomycetes may meet these requirements and possess potential for utilization as a biofungicide (Leiter et al., 2017). APs exhibit remarkable stability within harsh environmental conditions and are resistant to protease degradation owing to their compact β-fold and disulfide bond-stabilized tertiary structure (Galgóczy et al., 2010). Different APs from Penicillium or Aspergillus spp. can effectively inhibit the growth of several plant pathogens (Vila et al., 2001; Moreno et al., 2003, 2006; Theis et al., 2005; Barna et al., 2008; Garrigues et al., 2018; Tóth et al., 2020) and foodborne and postharvest mycotoxigenic fungi (Barakat et al., 2010a, b; Delgado et al., 2015, 2017, 2018; Garrigues et al., 2016). Topical application of the Aspergillus giganteus AP (AFP) (Moreno et al., 2003) and the Penicillium chrysogenum AP (PAF) (Barna et al., 2008) on plant leaves proved to protect Pelargonium, barley and wheat, respectively, against fungal pathogens without causing any detrimental effects to the host. Laboratory studies further demonstrated that the AFP (Barakat et al., 2010a) and the P. chrysogenum AP (PgAFP) (Delgado et al., 2018) decreased the level of mycotoxin contamination caused by Fusarium species and Aspergillus parasiticus on stored barley and dry-fermented food products, respectively. Furthermore, expression systems using generally recognized as safe (GRAS) microorganisms, such as Pichia pastoris (López-García et al., 2010; Virágh et al., 2014) and P. chrysogenum (Sonderegger et al., 2016; Garrigues et al., 2018; Tóth et al., 2018), are already available to produce correctly folded and functional APs in high amounts.
Synthetic antifungally active peptide derivatives (PDs) spanning distinct motifs of the full-length APs are also considered as promising biofungicides. Functional mapping of Penicillium digitatum AP B (AfpB) (Garrigues et al., 2017), Neosartorya (Aspergillus) fischeri AP 2 (NFAP2) (Tóth et al., 2018), and P. chrysogenum PAF (Sonderegger et al., 2018) demonstrated that their synthetic PDs spanning the cationic, surface-exposed loop regions exhibited remarkable in vitro antifungal activity. One of these regions is localized at the evolutionary conserved, so-called γ-core motif (GXC-X[3–9]-C). This motif is present in extracellular, cysteine-rich antimicrobial peptides and proteins from all biological kingdoms (Yount and Yeaman, 2006), and the amino acid constitution determines the efficacy of the antifungal plant defensins (Sagaram et al., 2011). In our previous study, we introduced a synthetic PD of the native PAF γ-core with anti-Candida activity and demonstrated that amino acid substitutions that elevate the positive net charge and hydrophilicity increased the antifungal potency (Sonderegger et al., 2018). And very recently, we described the potential of the P. chrysogenum PAF, its designed variant PAFopt, and γ-core peptide Pγopt in plant protection (Tóth et al., 2020).
The present study provides further evidence for the applicability of APs and PDs in agriculture for use as a potential biofungicide or biopreservative agent. We characterized the full-length NFAP from N. (A.) fischeri NRRL 181 and its PD γNFAP spanning the native NFAP γ-core and used the de novo rationally designed variant γNFAP-opt for the in vitro antifungal efficacy trial against phytopathogenic ascomycetes. Furthermore, we investigated the physicochemical properties, the structural flexibility influencing the antifungal activity, cytotoxicity on mammalian cell lines and plant seedlings, and their crop protection ability.
Materials and Methods
Strains, Cell Lines, and Media
The fungal strains that were tested for antifungal susceptibility are listed in Table 1. They were maintained on potato dextrose agar (PDA, Sigma–Aldrich, St. Louis, MO, United States) slants at 4°C, and susceptibility tests were performed in 10-fold diluted potato dextrose broth (0.1 × PDB, Sigma–Aldrich, St. Louis, MO, United States). Then, the cytotoxicity of NFAP and the PDs was investigated with the following human cell lines THP-1 monocyte cells and HT-29 colonic epithelial cells maintained in RPMI-1640 medium [no 4-(2-hydroxyethyl)-1-piperazineethanesulfonic acid (HEPES), phenol red; Gibco, Thermo Fisher Scientific, Waltham, MA, United States] and HaCaT keratinocyte cells maintained in Dulbecco’s modified Eagle’s medium (DMEM) (high glucose, HEPES, phenol red; Gibco, Thermo Fisher Scientific, Waltham, MA, United States). These media were supplemented with 10% (v/v) fetal bovine serum (Gibco Thermo Fisher Scientific, Waltham, MA, United States) and 1% (v/v) antibiotic/antimycotic solution containing 10,000 U ml–1 of penicillin, 10,000 μg ml–1 of streptomycin, and 25 μg ml–1 of amphotericin B (Gibco, Thermo Fisher Scientific, Waltham, MA, United States). Human cell lines were cultured at 37°C, 5% (v/v) CO2 added to the air.
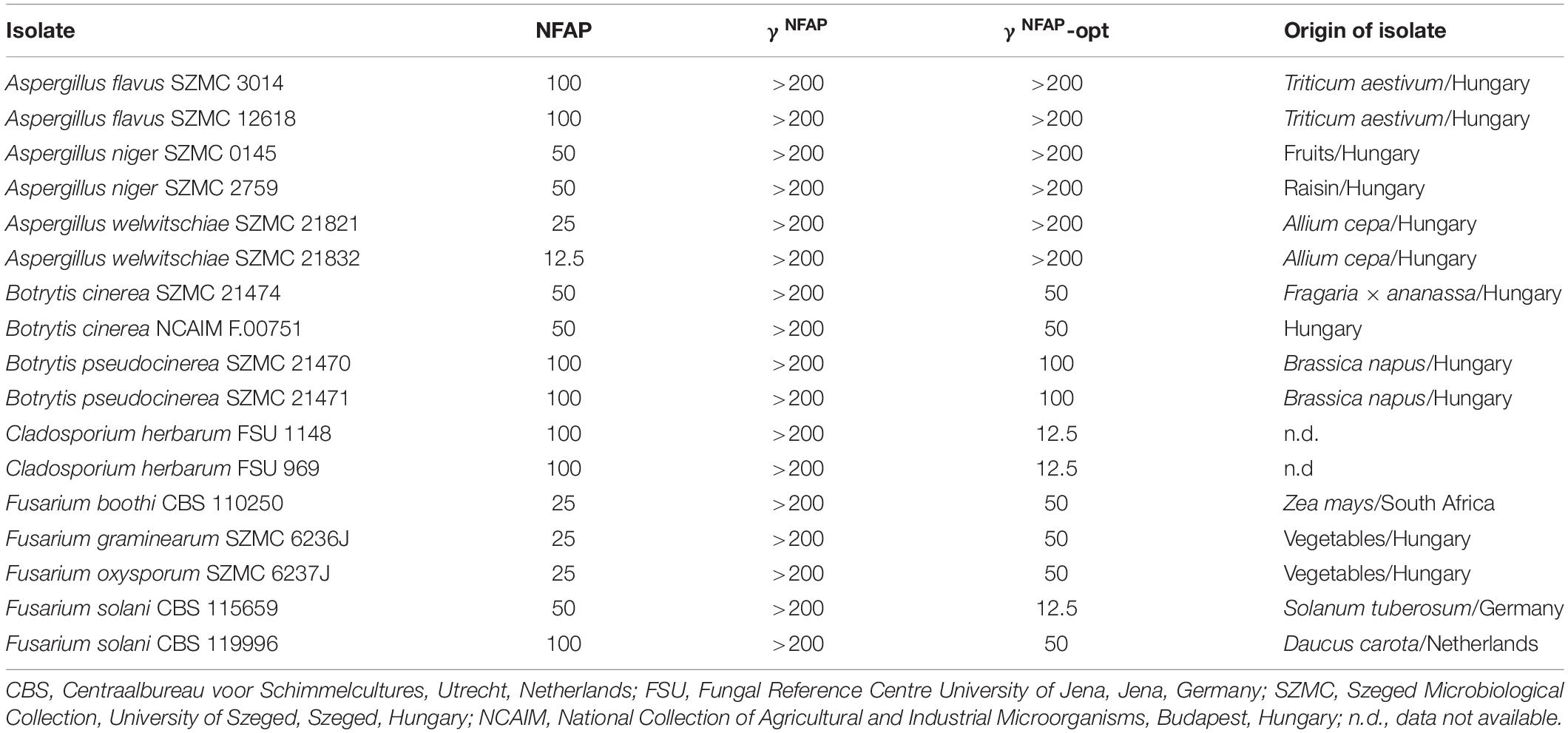
Table 1. Minimal inhibitory concentrations (μg ml–1) of Neosartorya fischeri antifungal protein (NFAP), γNFAP, and γNFAP-opt peptides against plant pathogenic filamentous ascomycetes.
Protein Production and Peptide Synthesis
Recombinant NFAP was produced in P. chrysogenum and purified as described previously (Sonderegger et al., 2016). Solid-phase peptide synthesis applying 9-fluorenylmethyloxycarbonyl chemistry was used to generate γ-core PDs of NFAP, according to Sonderegger et al. (2018).
In silico Analyses
Physicochemical properties of the NFAP and γ-core PDs were predicted in silico. Molecular weight (Mw), isoelectric point (pI), and the grand average of hydropathy (GRAVY) value were calculated by the ExPASy ProtParam tool (Gasteiger et al., 2005). The total net charge at pH = 7.0 was estimated using the Protein Calculator v3.4 server (The Scripps Research Institute1).
In vitro Antifungal Susceptibility Tests
The broth microdilution susceptibility testing method, according to Tóth et al. (2016), was applied to determine the minimal inhibitory concentrations (MICs) of NFAP and the γ-core PDs against phytopathogenic ascomycetes. One hundred microliters of NFAP or γ-core PD solution (0.39–400 μg ml–1 in twofold dilutions in 0.1 × PDB) were mixed with 100 μl of 2 × 105 conidia ml–1 in 0.1 × PDB in a flat-bottom 96-well microtiter plate (TC Plate 96 Well, Suspension, F; Sarstedt, Nümbrecht, Germany). The medium (0.1 × PDB) without NFAP or the γ-core PDs was added to the conidial suspension to serve as the untreated growth control. Fresh 200 μl 0.1 × PDB was used for the background calibration. The plates were incubated statically for 72 h at 25°C, and then, the absorbance (OD620) of each well was measured after shaking for 5 s with a microtiter plate reader operating in well-scanning mode (SPECTROstar Nano, BMG Labtech, Ortenberg, Germany). The absorbance of the untreated control represented 100% growth for the MIC calculation. MIC was defined as the lowest AP/PD concentration at which growth was ≤ 5% in comparison with the untreated control.
Growth percentages for Cladosporium herbarum FSU 1148 below the MIC were calculated in comparison with the untreated control to reveal the dose-dependent activity of NFAP and the PDs. In this case, the absorbance of the untreated control culture represented 100% growth for the calculation.
Susceptibility tests were repeated at least two times, including three technical replicates.
Microscopy
Morphological changes that occurred in the C. herbarum FSU 1148 conidia in the presence of NFAP and γ-core PDs were visualized by light microscopy (Axiovert 40 CFL; Zeiss, Oberkochen, Germany) and photographed by a microscope camera (AxioCam ICc 1; Zeiss, Oberkochen, Germany). ZEN 2.3 software (blue edition; Zeiss, Oberkochen, Germany) was used for image processing.
Electronic Circular Dichroism Spectroscopy
The conformational changes in the structure of NFAP and the γ-core PDs were investigated upon binding to the fungal target cells. Electronic circular dichroism (ECD) spectroscopy application for C. herbarum was performed with slight modifications as described previously for Candida albicans cells (Kovács et al., 2019). Briefly, C. herbarum FSU 1148 conidia were washed three times and suspended in ddH2O or an aqueous solution of 100 μg ml–1 NFAP or γ-core PD at a final concentration of 107 conidia ml–1. ECD spectroscopic measurements of these samples and an aqueous solution of 100 μg ml–1 NFAP and PDs were performed in the 185–260 nm wavelength range using a Jasco-J815 spectropolarimeter (JASCO, Tokyo, Japan). Spectra were collected at 25°C with a scan speed of 100 nm s–1 using a 0.1-cm path length quartz cuvette. Spectra presented are the accumulations of 10 scans for each sample. Spectrum acquisitions were conducted following 0, 4, and 24 h of incubation of the samples at 25°C and shaking at 210 r min−1. Following the spectroscopic measurements, the conidia in the samples were tested for their ability to germinate. To this end, they were washed and suspended in spore buffer [0.9% (m/v) NaCl, 0.01% (v/v) Tween 80], and then, they were streaked in appropriate dilutions (105–102 conidia ml–1 in PDB) onto PDA plates in three technical replicates for each sample dilution. The colony-forming units (CFUs) were determined following incubation at 25°C for 72 h. This experiment was repeated twice.
Cell Toxicity Tests With Human Cell Lines
The potential toxic effects of NFAP and γ-core PDs on the human cell lines were investigated with the application of the CCK8 cell proliferation and cytotoxicity assay kit (Dojindo Molecular Technologies Inc.; Rockville, MD, United States) following the manufacturer’s instructions with slight modifications. Briefly, 20,000 cells per well were preincubated statically in 100 μl (HaCaT and HT-29) or 80 μl (THP-1) of the maintaining medium without phenol red (Gibco, Thermo Fisher Scientific, Waltham, MA, United States) in a flat-bottom 96-well microtiter plate (TC Plate 96 Well, Standard, F; Nümbrecht, Germany) for 24 h in a humidified incubator at 37°C and 5% (v/v) CO2 in the air to determine the viability of the cells. Subsequently, the medium from the HaCaT and HT-29 cells was replaced with fresh medium supplemented with NFAP (400–100 μg ml–1 in twofold dilution) or γNFAP-opt or γNFAP-optGZ (25–6.25 μg ml–1 in twofold dilution). In non-adherent THP-1 cells, 20 μl of NFAP, γNFAP-opt, or γNFAP-optGZ were diluted and then RPMI-640 (without phenol red) was added to reach a final concentration of 50–200 μg ml–1 of NFAP or 6.25–25 μg ml–1 of γNFAP-opt or γNFAP-optGZ. Then, the plates were incubated for an additional 24 h under the same conditions. Untreated cells (in 100 μl of the respective medium) were used as a viability control, whereas cells treated with 100 μl 50% (v/v) ethanol for only 10 min before measurement served as the dead control. The viability measurement involved the following: the media of adherent HaCaT and HT-29 cells were replaced with the maintaining media without phenol red. Ten microliters of CCK-8 solution was gently mixed by pipetting into each well. Following 2 h the HaCaT and HT-29 or at 4 h THP-1 were incubated at the above-described conditions, the absorbance (OD405) was measured using a microplate reader (Hidex Sense Microplate Reader, Turku, Finland). Calculation of cell viabilities included the absorbance of the untreated control, which was assumed to represent 100% growth. Fresh medium without phenol red (100 μl) was used for the background calibration.
The hemolytic potentials of NFAP and the γ-core PDs were tested on Columbia blood agar plates (VWR; Radnor, PA, United States) using disc diffusion as described previously (Sonderegger et al., 2018). Sterile filter discs (Ø 6 mm) were placed on the agar plates, and 10 μl of an aqueous solution of NFAP (4 mg ml–1) or γNFAP-opt or γNFAP-optGZ (500 μg ml–1) was dropped onto them. Sterile ddH2O and 20% (v/v) Triton X-100 were used as negative and positive lysis controls, respectively. The plates were incubated for 24 h at 37°C before the plates were checked for the presence of clear zones around the filter discs.
Toxicity Tests With Plant Seedlings
Medicago truncatula, a fast-growing, small legume, easily cultivable on water agar in Petri dishes (Barker et al., 2006), was an appropriate model organism to investigate the harmful effects of APs and PDs on the growing plants. The NFAP and γ-core PDs were tested for potential toxic effects to the M. truncatula A-17 seedlings. Seeds were washed with 96% (v/v) sulfuric acid for 5 min, followed by washing with 0.1% (w/v) mercuric chloride solution for 3 min at room temperature and three times with cold ddH2O. Then, the seeds were left to germinate on 1% (w/v) water agar (Agar HP 696; Kalys, Bernin, France) for 3 days at 4°C in the dark. Seedlings with 3–4-mm-long primary roots were selected and transferred to square plates (120 mm × 120 mm × 17 mm Bio-One Square Petri Dishes with Vents, Greiner, Sigma–Aldrich, St. Louis, MO, United States) on fresh 1% (w/v) water agar lining them up at a 20-mm distance from the top. From this line, the bottom part of the plate was covered with aluminum foil to keep the root region in the dark. The apical region of the primary root was treated daily by dripping 20 μl drops of 400 μg ml–1 NFAP or 25 μg ml–1 γ-core PD in sterile ddH2O for 10 days. Plates were incubated in a humid (60%) plant growth chamber at 23°C under continuous illumination (1200 lux). The primary root length was measured, and the number of lateral roots was counted following the treatment period. Sterile ddH2O- and 70% (v/v) ethanol-treated seedlings were used as the growth or dead controls, respectively. Toxicity tests were repeated at least two times, and 12 seedlings were involved in each treatment.
Crop Protection Experiments
The crop protection abilities of NFAP and γ-core PDs were tested on the tomato fruits. The conidial suspension and NFAP and γ-core PD solutions were prepared in 0.1 × PDB. Tomato fruits (“On The Vine Red” variety) were purchased from a local organic farm (Szeged, Hungary). The surface of tomato fruits was sterilized via rinsing three times with 20% ethanol (v/v) and then sterile ddH2O. Tomato fruits were stung in 3 mm depth at three points near the stalk. Then, (i) infection control, 10 μl of the conidial suspension of C. herbarum FSU 1148 (107 conidia ml–1), (ii) toxicity testing, 10 μl of the MIC of NFAP or γ-core PDs (100 and 12.5 μg ml–1, respectively), (iii) crop protection investigation 10 μl C. herbarum FSU 1148 conidial suspension (107 conidia ml–1) containing the MIC of NFAP or γ-core PDs, and (iv) for the uninfected control 10 μl 0.1 × PDB were pipetted into the holes, and the treated specimens were then left to dry at room temperature. Unwounded tomato fruits were used as the untreated controls. Incubation included the following: the tomato fruits were kept in a sterile plastic box, humidified with wet paper towels for 7 days at 23°C (average room temperature in shops). Following this incubation period, the tomato fruits were axially cut in half, and then, the presence and depth of the fungal infection were assessed.
Statistical Analysis
Microsoft Excel 2016 software (Microsoft, Edmond, WA, United States) was used to check the normal distribution of our datasets. Levene’s test (Homogeneity of Variance Calculator-Levene’s Test2) was applied to reveal whether the variances of two samples are approximately equal or not. If equal variance was assumed, Microsoft Excel 2016 software was used also to calculate the standard deviations and to determine the significance values (two-sample t-test). Significance was defined as P ≤ 0.05 based on the following: ∗P ≤ 0.05, ∗∗P ≤ 0.005, and ∗∗∗P ≤ 0.0001. If the two datasets failed in the test of homogeneity, the two-tailed Mann–Whitney U-test (Mann–Whitney U Test Calculator3) was applied to reveal the significance. Significance was defined as ∗P < 0.05 in this case.
Results
Peptide Design
Physicochemical properties of the NFAP and PDs are summarized in Table 2. The native γ-core motif of NFAP (GECFTKDNTC) is located in the first loop region. It is negatively charged (net charge is −1.1 at pH = 7.0) and slightly hydrophilic (GRAVY = −0.840) (Table 2). The PD γNFAP spanning the native NFAP γ-core motif was designed based on our recent findings regarding the stability and antifungal efficacy of the γ-core peptides Pγ and Pγopt from native P. chrysogenum PAF (Sonderegger et al., 2018). The synthetic γNFAP is almost neutral (net charge is −0.1 at pH = 7.0) and hydrophilic (GRAVY = −1.5000) and contains three additional amino acids at the N-terminus and ends in an extra C-terminal lysine residue. The applied N-terminal acetylation and C-terminal amidation mimicked the propagating native protein backbone, neutral terminals, and provided stability against proteolysis. Previously, we observed that cyclization of the Pγ through the disulfide bridge formation impaired antifungal efficacy (Sonderegger et al., 2018); thus, all cysteines in γNFAP possessed free sulfhydryl (–SH) groups (Table 2). Specific amino acids were substituted in γNFAP to create the PDγNFAP-opt exhibiting an elevated positive net charge (+5.8 at pH = 7.0) and increased hydrophilicity (GRAVY = −2.264) (Table 2). Then, we investigated whether the net charge or the hydrophilicity influenced the antifungal activity of γNFAP-opt and synthesized two different variants. In the γNFAP-optChZ, amino acid substitutions reduced the net charge from +5.8 to neutral (−0.1 at pH = 7.0) but maintained the GRAVY (Table 2). In contrast, the GRAVY was reduced to −0.557, whereas the net charge remained unchanged (+ 5.8 at pH = 7.0) in the γNFAP-optGZ variant (Table 2).
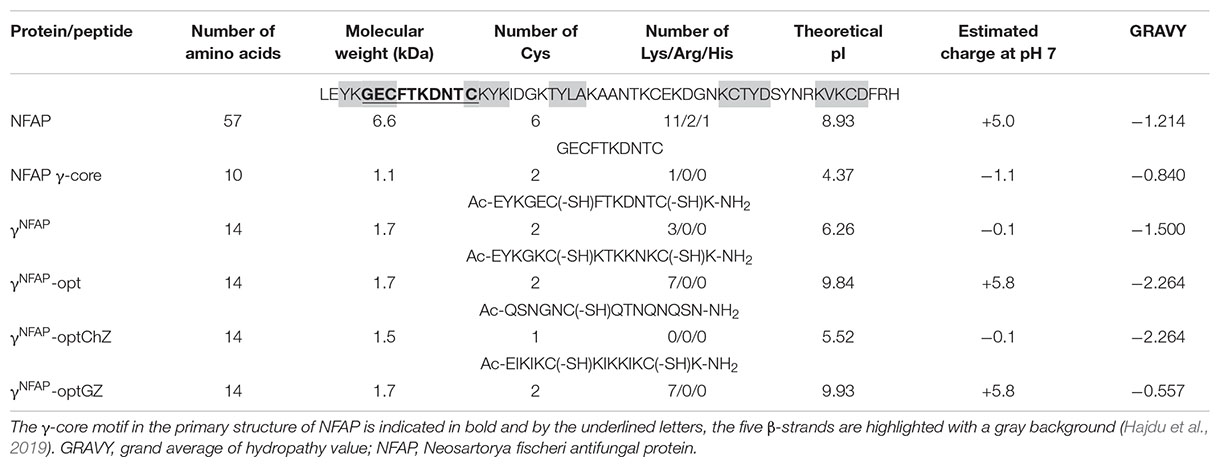
Table 2. Amino acid sequence and in silico predicted physicochemical properties of mature Neosartorya fischeri antifungal protein (NFAP) and peptide derivatives.
In vitro Antifungal Activity of Neosartorya fischeri Antifungal Protein and γ-Core Peptide Derivatives on Plant Pathogenic Ascomycetes
The antifungal activity and spectrum of NFAP and the γ-core PDs were investigated in a broth microdilution susceptibility assay. The results are summarized in Table 1. NFAP inhibited the growth of all tested preharvest or postharvest plant pathogenic ascomycetous isolates with various MICs ranging from 12.5 to 100 μg ml–1. In contrast, γNFAP was ineffective (MIC > 200 μg ml–1) in the investigated concentration range. The γNFAP-opt effectively inhibited the growth of Botrytis (MIC range: 50–100 μg ml–1), Cladosporium (MIC range: 25–100 μg ml–1), and Fusarium (MIC range: 12.5–50 μg ml–1) isolates; however, aspergilli were not inhibited (MIC > 200 μg ml–1).
Physicochemical Determinants for the Antifungal Efficacy of γ-Core Peptide Derivatives
To understand whether the hydrophilicity or the net charge determines the high antifungal activity of γNFAP-opt, the PD variants γNFAP-optChZ and γNFAP-optGZ (Table 2) were also subjected to antifungal susceptibility test against C. herbarum FSU 1148. The neutral and hydrophilic γNFAP-optChZ was inactive at 12.5 μg ml–1, which corresponded to the MIC of γNFAP-opt. Fungal growth was visible in the presence of γNFAP-optChZ, and the fungal germlings resembled those of the untreated control (Figure 1A). In contrast, the positively charged but less hydrophilic γNFAP-optGZ inhibited the germination of C. herbarum FSU 1148 conidia at the same MIC as γNFAP-opt (12.5 μg ml–1) (Figure 1A). These results suggested that the positive net charge, not the hydrophilicity of these γ-core peptides, played a major role in antifungal efficacy. Furthermore, this experiment also indicated that NFAP induced severe morphological changes in the C. herbarum hyphae, which resulted in a multibranched phenotype when applied at concentrations below the MIC (12.5 μg ml–1). In contrast, γNFAP did not exhibit any morphological effects on this test fungus at this concentration (Figure 1A). Application of the twofold dilution series of NFAP and the γ-core PDs showed that the full-length protein acts in a dose-dependent manner, whereas the synthetic γ-core peptides γNFAP-opt and γNFAP-optGZ did not show any significant inhibitory effects on the fungal growth below their MIC (Figure 1B).
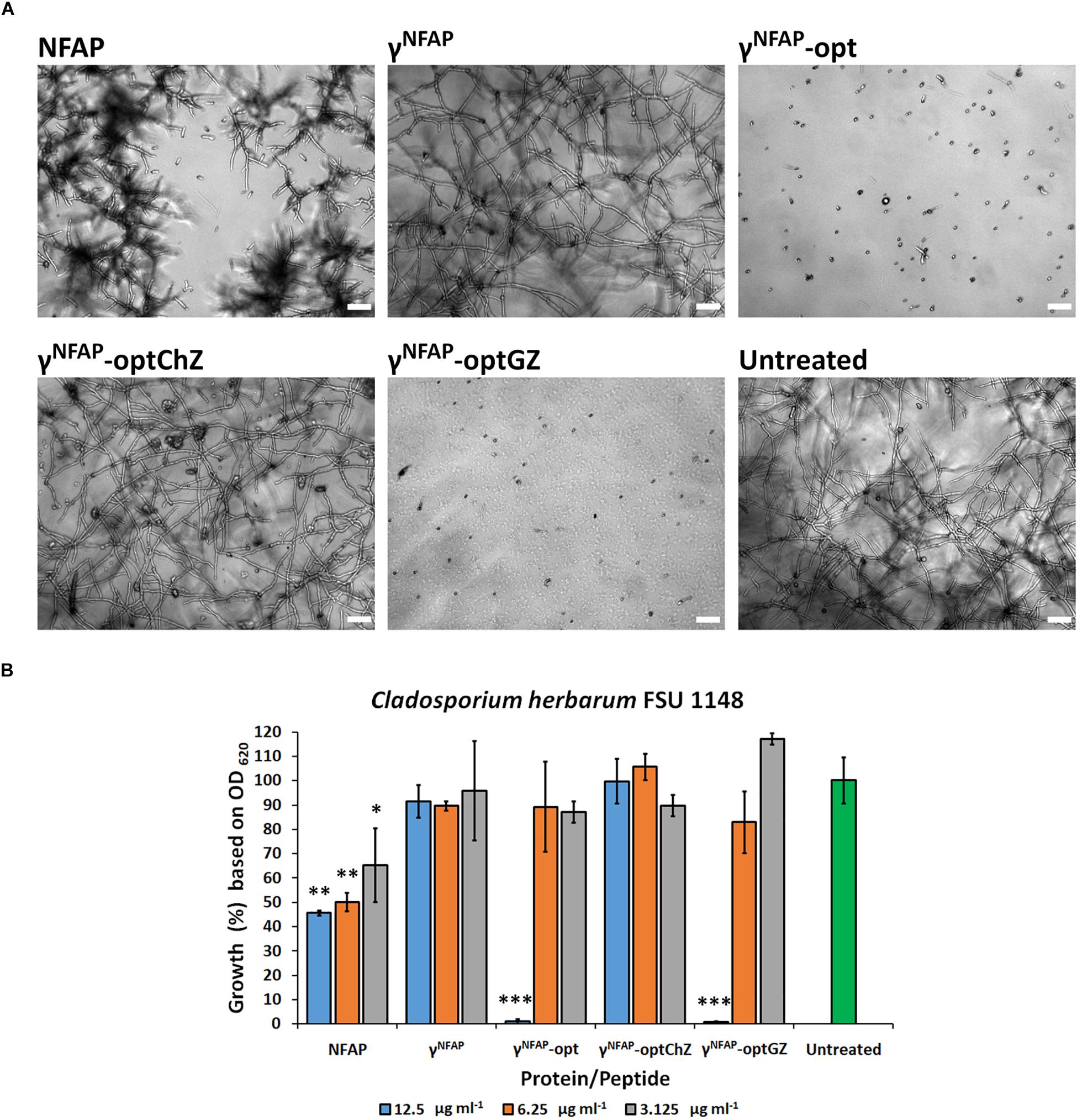
Figure 1. (A) Phenotype of the Cladosporium herbarum FSU 1148 hyphae evolved from conidia treated with 12.5 μg ml–1 Neosartorya fischeri antifungal protein (NFAP) and the γ-core peptide derivatives (PDs) γNFAP, γNFAP-opt, γNFAP-optChZ, and γNFAP-optGZ at 25°C for 72 h. Scale bars represent 25 μm. (B) Growth percentages of C. herbarum FSU 1148 in the presence of different concentrations of NFAP and the γ-core PDs at 25°C for 72 h. Significant differences from two-sample t-test in (B) are indicated with ∗(P ≤ 0.05), ∗∗(0.0001 < P ≤ 0.005), and ∗∗∗(P ≤ 0.0001) in comparison of each treatment with the untreated control sample.
The Structure–Function Relation of Neosartorya fischeri Antifungal Protein and γ-Core Peptide Derivatives
Several antimicrobially active peptides exerted their effect through the disruption of cell membranes in the sensitive microorganisms. Such mechanisms required extensive conformational changes or peptide oligomerization (Cosentino et al., 2016; Sani and Separovic, 2016; Kumar et al., 2018). ECD spectroscopy was an effective method to monitor such structural changes in the presence of a sensitive microbe (Avitabile et al., 2014). Following 24-h co-incubation of NFAP and C. herbarum FSU 1148 conidia did not alter the ECD spectrum of the protein, which indicated that no conformational changes are induced by this interaction with the conidia (Figure 2), whereas the antifungal effect was evident, as indicated by the decreased colony-forming abilities of the conidia following treatment (Table 3). The ECD spectrum of NFAP in the presence of conidia was very similar to that measured previously for pure aqueous solutions of NFAP (Sonderegger et al., 2016), which indicated an intact, disulfide-bridged, β-pleated conformation (Figure 2). Nevertheless, the treatment with 100 μg ml–1 NFAP reduced the CFU from 2.2 ± 0.7 × 106 to 1.5 ± 0.7 × 106 (Table 3). Similarly, no conformational changes were observed with any of the antifungal active NFAP γ-core PDs tested. Based on their ECD spectra, γNFAP-opt and γNFAP-optGZ were unordered peptides in aqueous solution (Figure 2). No indication of the ordered structure formation was observed, following 24 h of incubation with C. herbarum FSU 1148 conidia (Figure 2). However, again, γNFAP-opt and γNFAP-optGZ significantly reduced the CFU from 2.2 ± 0.7 × 106 to 1.3 ± 0.6 × 105 (P = 0.00512) and 0.7 ± 0.6 × 105 (P = 0.00512), respectively (Table 3). These results indicated that a canonically ordered conformation is not required for the antifungal effect of the studied peptides. Furthermore, no conformational change could be detected with γNFAP and γNFAP-optChZ by ECD spectroscopy (data not shown), and expectedly, these PDs did not significantly reduce the ability for colony establishment of C. herbarum conidia (Table 3).
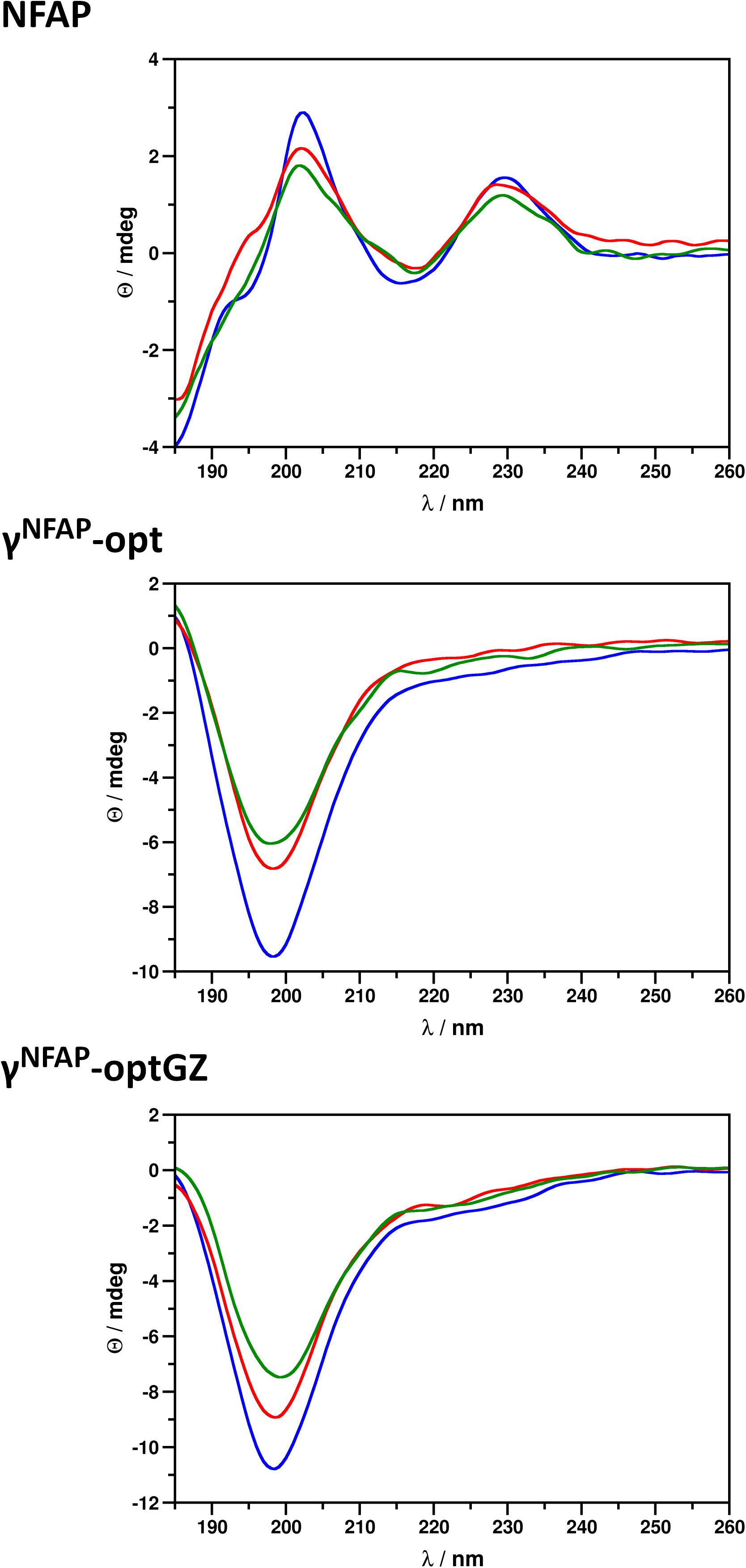
Figure 2. Electronic circular dichroism (ECD) spectra of Neosartorya fischeri antifungal protein (NFAP), γNFAP-opt, and γNFAP-optGZ peptides in ddH2O (blue) and in the presence of Cladosporium herbarum FSU 1148 conidia immediately after exposure (red) to and after 24 h of co-incubation (green) with 100 μg ml–1 NFAP, γNFAP-opt, or γNFAP-optGZ.
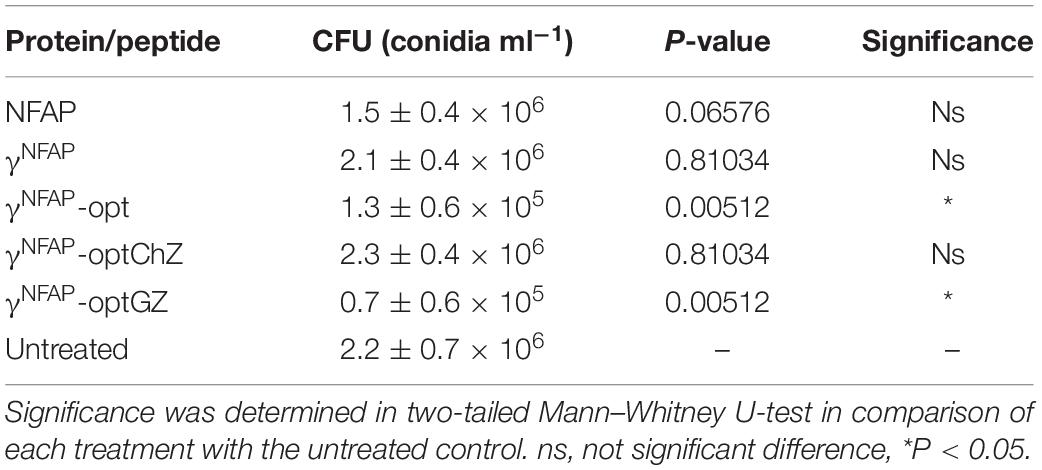
Table 3. Colony-forming unit (CFU) of Cladosporium herbarum FSU 1148 conidia treated with 100 μg ml–1 Neosartorya fischeri antifungal protein (NFAP), γNFAP-opt, and γNFAP-optGZ peptides.
Cytotoxic Potential of Neosartorya fischeri Antifungal Protein and γ-Core Peptide Derivatives on Human Cell Lines
One of the requirements for new fungicides designed for agricultural applications is their harmlessness in the host. As a proof-of-principle, we tested the cytotoxic potential of NFAP and the two antifungally active γ-core PDs γNFAP-opt and γNFAP-optGZ on human keratinocytes and the colonic epithelial cells. These cell types were in direct contact with APs and PDs if applied as biofungicides, and the treated agricultural products were considered for human consumption. Keratinocytes are the predominant cell type in the epidermis, whereas colonic epithelial cells play a role in nutrient absorption and the innate and adaptive mucosal immunity. Monocytes were also subjected to toxicity tests. They are important parts of the human body’s defense system against infectious organisms and non-self-molecules.
NFAP and γNFAP-opt did not reduce the viability of the human cell lines in the tested concentration range up to their 2 × MIC (Figures 3A,B). Interestingly, higher viability than the untreated control was detected for the keratinocytes in the presence of 12.5 and 6.25 μg ml–1 γNFAP-opt (Figure 3B). In contrast, a significant reduction in the viability of the keratinocytes exposed to 25 and 12.5 μg ml–1 γNFAP-optGZ in comparison with the untreated control was observed (Figure 3C). The viability of the other cell lines was not significantly affected by this peptide in the tested concentration range (Figure 3C). The cell membrane disruption ability of NFAP and the antifungally active PDs was investigated on erythrocytes. None of the tested proteins and peptides, NFAP, γNFAP-opt, and γNFAP-optGZ, caused hemolysis (Figure 3D).
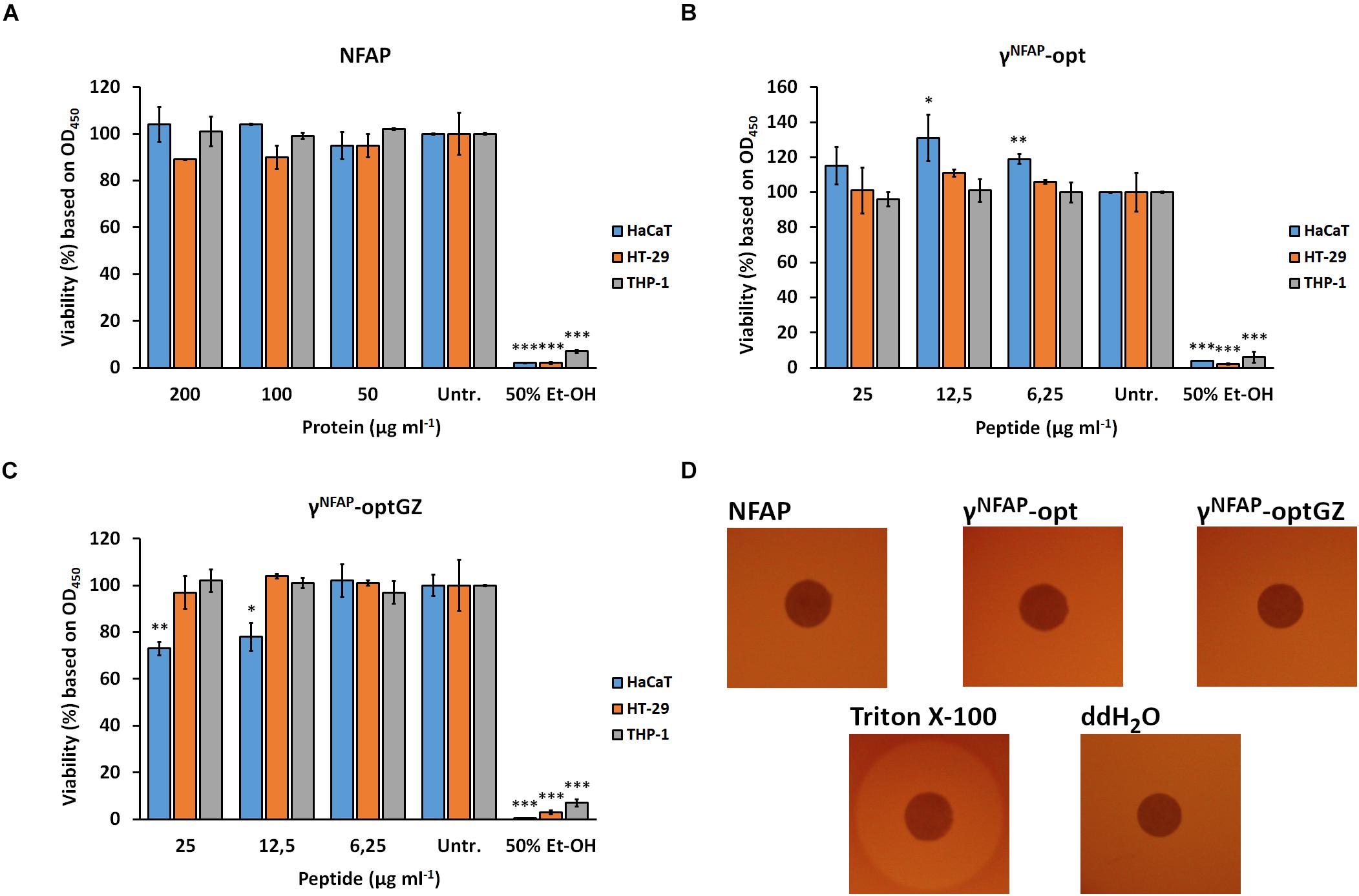
Figure 3. Viability of keratinocytes (HaCaT), colonic epithelial cells (HT-29), and monocytes (THP-1) after treatment with different concentrations of (A) Neosartorya fischeri antifungal protein (NFAP), (B) γNFAP-opt, or (C) γNFAP-optGZ peptides for 24 h and with 50% (v/v) ethanol (Et-OH) for 10 min in comparison with the untreated control (Untr.). (D) Hemolytic activity of 40 μg NFAP, 25 μg γNFAP-opt and γNFAP-optGZ peptides on Columbia blood agar plates after incubation for 24 h at 37°C. Triton X-100 [20% (v/v)] and ddH2O were used as the positive and negative controls, respectively. Significant differences from two-sample t-test in (A–C) are indicated with ∗(P ≤ 0.05), ∗∗(0.0001 < P ≤ 0.005), and ∗∗∗(P ≤ 0.0001) in comparison of each treatment with the untreated control sample.
Cytotoxic Potential of Neosartorya fischeri Antifungal Protein and γ-Core Peptide Derivatives on Plant Seedling
The potential of NFAP and the antifungally active γ-core PDs γNFAP-opt and γNFAP-optGZ to induce morphological aberration and retardation in growing plants was investigated using M. truncatula A-17 seedlings. Treatment with 400 μg ml–1 NFAP and 25 μg ml–1 γNFAP-opt and γNFAP-optGZ did not cause any changes to the plant morphology (Figure 4A). Furthermore, no significant changes in the primary root length and the number of evolved lateral roots were observed following the treatment period (Figure 4B).
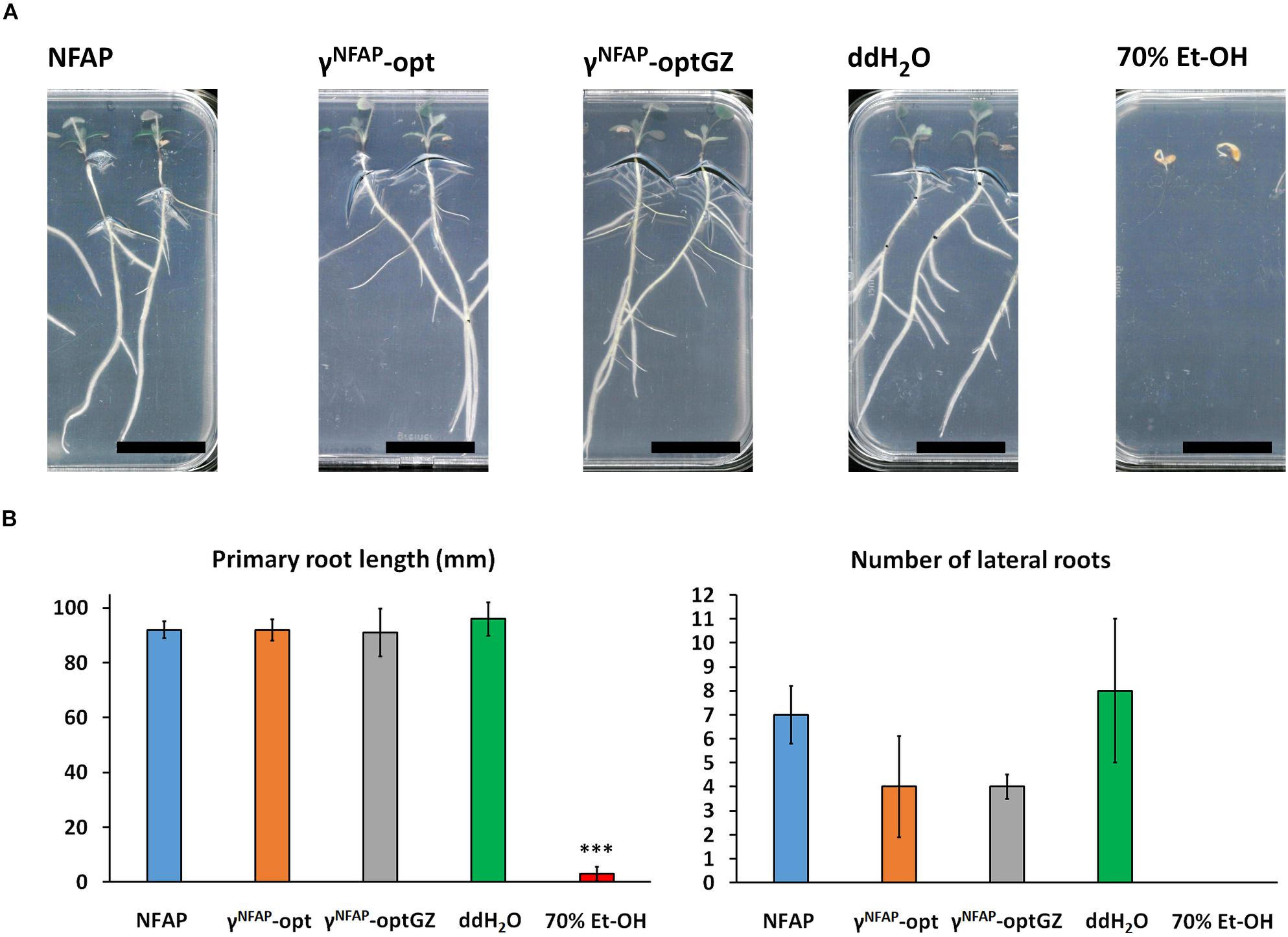
Figure 4. Vegetative growth and development of Medicaco truncatula A-17 roots. (A) Phenotype of M. truncatula A-17 plants grown from seedlings and (B) the length of the evolved primary roots and number of lateral roots after treatment with 400 μg ml–1 Neosartorya fischeri antifungal protein (NFAP), 25 μg ml–1 γNFAP-opt, and 25 μg ml–1 γNFAP-optGZ peptides for 10 days at 23°C under continuous illumination of the shoot system (1200 lux) in comparison with ddH2O- and 70% (v/v) Et-OH-treated controls. Significant difference from two-sample t-test in (B) is indicated with ∗∗∗(P ≤ 0.0001) in comparison of each treatment to the ddH2O-treated control.
Crop Protection Ability of Neosartorya fischeri Antifungal Protein and γ-Core Protein Derivatives
The ability of NFAP and the antifungally active γ-core PDs to protect crops was studied on tomato fruits against C. herbarum FSU 1148. This fungus is known as a postharvest spoilage agent of fresh fruits and vegetables, including tomatoes under storage conditions, especially when the vegetable surface was damaged (Snowden, 1992). Control treatments with NFAP, γNFAP-opt, and γNFAP-optGZ did not cause any decay on the surface of the tomato fruits (data not shown). The same was observed when the fruits were treated with 0.1 × PDB (0.1 × PDB in Figure 5), the medium used for the infection. C. herbarum infection, instead, was established within the applied incubation period at the sting points and the deeper tissues (Cherb in Figure 5). Application of NFAP, γNFAP-opt, and γNFAP-optGZ at their MIC inhibited the development of decay. No intensive fungal growth was observed on the surface or in deeper tissues of the tomato fruits (Cherb + NFAP, Cherb + γNFAP-opt, and Cherb + γNFAP-optGZ in Figure 5).
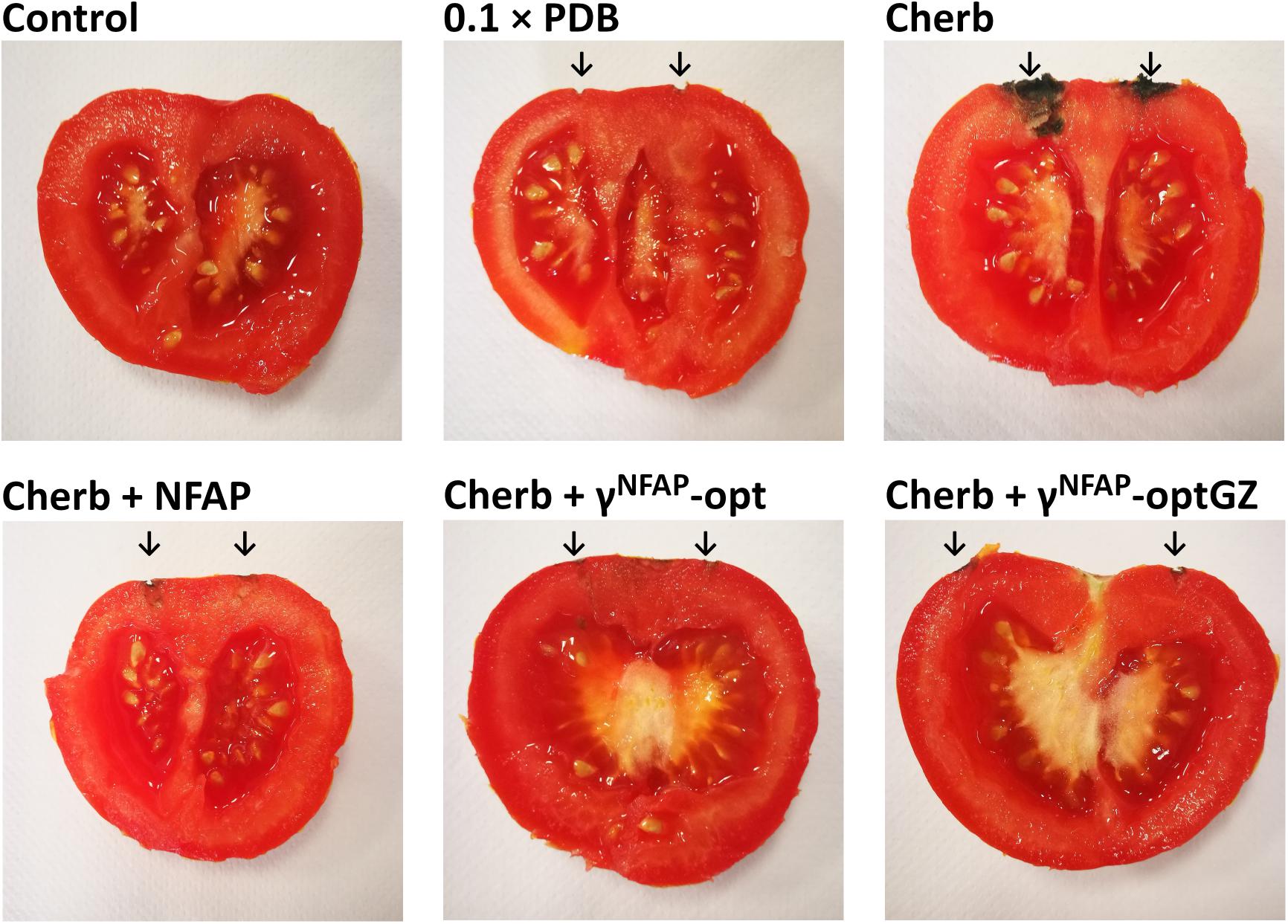
Figure 5. The biopreservation potential of Neosartorya fischeri antifungal protein (NFAP) (Cherb + NFAP) and γ-core peptide derivatives (PDs) (Cherb + γNFAP-opt, Cherb + γNFAP-optGZ) of postharvest tomato fruits infected with Cladosporium herbarum FSU 1148 after incubation at 23°C for 7 days. The controls were uninfected but treated with medium (0.1 × PDB) or infected with C. herbarum FSU 1148 (Cherb) but without AP or PD treatment. Infected tomatoes were treated with 100 μg ml–1 NFAP, 12.5 μg ml–1 γNFAP-opt, or γNFAP-optGZ, respectively. Unwounded tomato fruits without infection and treatment were used as natural decay controls (Control). The sites of the infections and treatments are indicated with the black arrow.
Discussion
Several APs and antimicrobial peptides from various studies are already in consideration for agricultural application as protective or preservative agents against microbial infections or contamination of plants or stored crops. Their features, such as heat tolerance, a relatively broad antimicrobial spectrum, and low toxicity to plant and mammalian cells, render them to be promising candidates as biopesticides. Primarily, they are applied as recombinant antimicrobial peptides expressed by transgenic plants to confer disease protection (Meng et al., 2010). However, diverse, non-comprehensive international regulations regarding the agricultural application of genetically modified (GM) plants (Tagliabue, 2017) and the prevalence of an anti-GM organism attitude held by policy makers and the general public highly limit the cultivation of these breeds (Lucht, 2015). Furthermore, high production costs, limited information about the antifungal spectrum, the long-term toxic effects regarding plant development and human health are currently obstructing the direct topical application of antifungal peptides and proteins for use as biofungicides or bioprotective agents (Jung and Kang, 2014). The present study provided evidence that AP and the PD applications could be used as a safe and effective topical biofungicide.
The recombinant NFAP produced by the GRAS organism P. chrysogenum effectively inhibited the in vitro growth of agriculturally important ascomycetes such as Aspergillus, Botrytis, Cladosporium, and Fusarium isolates (Table 2). Aspergillus and Fusarium are well-known plant pathogens and infectious agents of stored crops; they produce mycotoxins that pose a severe threat to both human and animal health (Alshannaq and Yu, 2017). Cladosporium spp. are spoilage agents of freshly harvested vegetables and cause significant economic losses every year (De Lucca, 2007). Botrytis spp. are aggressive pathogens of the upper parts of numerous plants in the preharvest and postharvest stages (Elad et al., 2007). Recently, several different resistance mechanisms to the chemical fungicides have emerged in the genera Aspergillus, Fusarium (Lucas et al., 2015; Hawkins et al., 2019), and Botrytis (Rupp et al., 2017). NFAP and γNFAP-opt represent potential alternatives to combat chemical fungicide-resistant strains based on our susceptibility test results.
Interestingly, NFAP did not inhibit the germination of C. herbarum conidia when it was applied at sublethal concentrations (below the MIC) but affected the hyphal morphology (Figure 1). NFAP induced extensive hyperbranching, similarly to the report on the effect of the morphogenic defensin MsDef1 from Medicago sativa (Sagaram et al., 2011) or PAF from P. chrysogenum (Kaiserer et al., 2003). We have previously observed this phenomenon with Aspergillus niger (Kovács et al., 2011) and Aspergillus nidulans (Virágh et al., 2015; Galgóczy et al., 2017) when treated with NFAP. These results evidence that NFAP is a morphogenic AP.
The synthetic γ-core PDs of NFAP, γNFAP-opt, and γNFAP-optGZ (Table 2), with a positive net charge, exhibited potent antifungal activity, whereas the PD γNFAP-optChz with a neutral net charge but the same GRAVY (Table 2) was ineffective against plant pathogenic ascomycetes (Tables 1, 3 and Figure 1). The authors observed a similar correlation between the positive net charge and antifungal efficacy in a previous study with the anti-yeast NFAP2. A PD spanning the neutral NFAP2 γ-core loop region did not inhibit the growth of Candida cells. In contrast, a PD derived from the center of the positively charged N-terminal loop region of NFAP2 showed anti-Candida activity, whereby the inhibitory potential was independent of the primary structure (Tóth et al., 2018). Another example was reported by Garrigues et al. (2017), who observed that synthetic peptides derived from P. digitatum AfpB inhibited the growth of filamentous fungi when they exhibited a high positive net charge. The potential to improve the antifungal efficacy of the peptides by rational design was demonstrated with the peptide Pγ spanning the positively charged P. chrysogenum PAF γ-core along with the variant Pγopt. This latter PD inhibited the growth of the C. albicans cells more efficiently than the native Pγ because of the elevated positive net charge; however, this occurred independently from the primary structure (Sonderegger et al., 2018). All of these reports so far were evidence that the overall positive net charge was the primary and the most important feature that determined the efficacy of antifungal PDs, irrespective of hydrophilicity, amino acid composition, or primary structure.
Electronic circular dichroism spectroscopy excluded any conformational changes to NFAP to be mandatory for the inhibition of the conidial germination (Figure 2 and Table 3). The same was described before for the potent anti-yeast protein NFAP2 in the presence of the C. albicans cells (Kovács et al., 2019). Both proteins shared a similar ECD spectrum, which was characteristic of the small, disulfide-bridged, β-pleated antifungal proteins (Sonderegger et al., 2016; Tóth et al., 2016). We also found that the NFAP γ-core PDs were unordered in the pure aqueous solution, and their antifungal activity was independent of the ordered structure (Figure 2 and Table 3). These observations are parallel with reports of the antifungal activity of the PDs of AfpB (Garrigues et al., 2017) and PAF (Sonderegger et al., 2018) that showed unordered structures under the same experimental setup as the current study utilized. In contrast, membrane disruptive antibacterial peptides underwent a remarkably conformational change when they exerted inhibitory activity on the sensitive bacterial cells (Avitabile et al., 2014). Differences in the mechanistic mode of action between the antifungal and antibacterial peptides or between the structure of the fungal and bacterial cell membrane could explain these contradictions in observations.
The suitability of APs to efficiently protect agriculturally important plants and crops against fungal infection and contamination by direct topical application was proved previously (Moreno et al., 2003; Theis et al., 2005; Barna et al., 2008; Barakat et al., 2010a, b; Barakat, 2014; Tóth et al., 2020). However, the introduction of APs into the biopesticide market requires high ecological compatibility and tolerance by the host (humans, animals, and plants). As for the harmlessness in humans and animals, our proof-of-principle experiments indicated that NFAP and the PD γNFAP-opt were not cytotoxic against keratinocytes, colonic epithelial cells, and monocytes and were not hemolytic in the antifungal effective concentration (Figure 3). Therefore, this N. fischeri protein and peptide exhibited harmless activity in vitro similar to other members of the fungal APs, such as PAF and AFP (Szappanos et al., 2005, 2006; Tóth et al., 2020), PAF γ-core PDs (Sonderegger et al., 2018; Tóth et al., 2020), and NFAP2 (Kovács et al., 2019). Importantly, experiments with a murine model for pulmonary fungal infection and fungal vulvovaginitis further proved the safety in vivo for PAF (Palicz et al., 2013, 2016) and NFAP2 (Kovács et al., 2019), respectively.
So far, no adverse effects of the fungal APs and PDs on plants or fruits have been reported in the literature (Vila et al., 2001; Moreno et al., 2003, 2006; Theis et al., 2005; Barakat, 2014; Garrigues et al., 2018); however, little information is available on the induction of morphological aberrations when growing seedlings with these biomolecules. Exceptions are AFP that did not affect the growth of the tomato plant roots (Theis et al., 2005) or PAF and its derived variant and PD that did neither harm the leaves of barley (Barna et al., 2008) nor those of tomatoes nor affected the seedlings of M. truncatula (Tóth et al., 2020). In the present study, the vegetative growth and the morphology of the roots of the M. truncatula seedlings were not disturbed by NFAP (Figure 4). We, therefore, suggested that most APs and PDs originating from ascomycetes acted specifically on the fungal cells and were not harmful to the plant cells.
The crop-protective potential of AFP as a topical postharvest preservative has been proven against different Fusarium spp. on postharvest barley (Barakat et al., 2010b) and against Alternaria alternata on tomatoes, mango fruits (Barakat et al., 2010a), and banana (Barakat, 2014). One important benefit of the AFP treatment was a significant reduction in the mycotoxin burden of the crop (Barakat et al., 2010a). NFAP and the γ-core PDs γNFAP-opt and γNFAP-optGZ similarly proved effective as biopreservatives for tomato fruits as they prevented the contamination by the postharvest mold C. herbarum (Figure 5) equally, which suggested a promising novel compound for the prevention of mycotoxin contamination in food products.
Conclusion
Taken together, our study demonstrated that NFAP and rationally designed synthetic PD γNFAP-opt are promising candidates for biopreservation in agriculture and food industry. However, further studies that focus on their environmental impact and address their pharmacokinetic properties in the human body are essential to push forward their applicability.
Data Availability Statement
The datasets generated for this study are available on request to the corresponding author.
Author Contributions
IN, GT, GR, FM, and LG conceived and supervised the study, designed the experiments, and edited the manuscript. GV, FM, and LG performed peptide design. GV performed peptide synthesis. LT and HF performed protein preparation, in vitro antifungal susceptibility tests, and plant toxicity assay and analyzed the related data. AB and HF performed ECD spectroscopy and analysis of the related data. LT and ÉB performed cell viability assay and analyzed the related data. LT and LG performed crop protection experiments and analysis of the related data. LT, AB, IN, GT, GR, FM, and LG wrote the manuscript and made manuscript revisions. All authors read and approved the submitted version.
Funding
LG was financed by the Postdoctoral Excellence Program (PD 131340) and the bilateral Austrian-Hungarian Joint Research Project (ANN 131341) of the Hungarian National Research, Development and Innovation Office (NKFI Office). This work was supported by the Austrian Science Fund FWF (I3132-B21) to FM. Research of LG has been supported by the János Bolyai Research Scholarship of the Hungarian Academy of Sciences. Present work of LG was supported by the ÚNKP-19-4 New National Excellence Program of the Ministry for Innovation and Technology. This work was supported by the following grants TUDFO/47138-1/2019-ITM FIKP, GINOP-2.3.2-15-2016-00014, 20391-3/2018/FEKUSTRAT to GV, and GT. Work of LT was supported by the NTP-NFTÖ-18 Scholarship.
Conflict of Interest
The authors declare that the research was conducted in the absence of any commercial or financial relationships that could be construed as a potential conflict of interest.
Footnotes
- ^ http://protcalc.sourceforge.net
- ^ https://www.socscistatistics.com/tests/levene/default.aspx
- ^ https://www.socscistatistics.com/tests/mannwhitney/default2.aspx
References
Almeida, F., Rodrigues, M. L., and Coelho, C. (2019). The still underestimated problem of fungal diseases worldwide. Front. Microbiol. 10:214. doi: 10.3389/fmicb.2019.00214
Alshannaq, A., and Yu, J. H. (2017). Occurrence, toxicity, and analysis of major mycotoxins in food. Int. J. Environ. Res. Public Health 14:E632. doi: 10.3390/ijerph14060632
Avitabile, C., D’Andrea, L. D., and Romanelli, A. (2014). Circular dichroism studies on the interactions of antimicrobial peptides with bacterial cells. Sci. Rep. 4:4293. doi: 10.1038/srep04293
Barakat, H. (2014). Bio-control of Alternaria alternata during banana storage by purified AFP using isoelectric focusing technique. Food Nutr. Sci. 5, 1482–1495. doi: 10.4236/fns.2014.515161
Barakat, H., Spielvogel, A., Hassan, M., El-Desouky, A., El-Mansy, H., Rath, F., et al. (2010a). The antifungal protein AFP from Aspergillus giganteus prevents secondary growth of different Fusarium species on barley. Appl. Microbiol. Biotechnol. 87, 617–624. doi: 10.1007/s00253-010-2508-4
Barakat, H., Stahl, U., and El-Mansy, H. (2010b). Application for the Antifungal Protein From Aspergillus Giganteus: Use With Selected Foodstuffs As Regards To Quality And Safety. Saarbrucken: VDM Verlag Dr. Müller.
Bardin, M., Ajouz, S., Comby, M., Lopez-Ferber, M., Graillot, B., Siegwart, M., et al. (2015). Is the efficacy of biological control against plant diseases likely to be more durable than that of chemical pesticides? Front. Plant Sci. 6:566. doi: 10.3389/fpls.2015.00566
Barker, D., Pfaff, T., Moreau, D., Groves, E., Ruffel, S., Lepetit, M., et al. (2006). “Growing M. truncatula: choice of substrates and growth conditions,” in Medicago Truncatula Handbook, eds E.-P. Journet and U. Mathesius (Ardmore: The Samuel Roberts Noble Fondation), 1–25.
Barna, B., Leiter, E., Hegedus, N., Bíró, T., and Pócsi, I. (2008). Effect of the Penicillium chrysogenum antifungal protein (PAF) on barley powdery mildew and wheat leaf rust pathogens. J. Basic Microbiol. 48, 516–520. doi: 10.1002/jobm.200800197
Cosentino, K., Ros, U., and García-Sáez, A. J. (2016). Assembling the puzzle: oligomerization of α-pore forming proteins in membranes. Biochim. Biophys. Acta 1858, 457–466. doi: 10.1016/j.bbamem.2015.09.013
De Lucca, A. J. (2007). Harmful fungi in both agriculture and medicine. Rev. Iberoam Micol. 24, 3–13.
Delgado, J., Acosta, R., Rodríguez-Martín, A., Bermúdez, E., Núñez, F., and Asensio, M. A. (2015). Growth inhibition and stability of PgAFP from Penicillium chrysogenum against fungi common on dry-ripened meat products. Int. J. Food Microbiol. 205, 23–29. doi: 10.1016/j.ijfoodmicro.2015.03.029
Delgado, J., Owens, R. A., Doyle, S., Núñez, F., and Asensio, M. A. (2017). Quantitative proteomics reveals new insights into calcium-mediated resistance mechanisms in Aspergillus flavus against the antifungal protein PgAFP in cheese. Food Microbiol. 66, 1–10. doi: 10.1016/j.fm.2017.03.015
Delgado, J., Rodríguez, A., García, A., Núñez, F., and Asensio, M. A. (2018). Inhibitory effect of PgAFP and protective cultures on Aspergillus parasiticus growth and aflatoxins production on dry-fermented sausage and cheese. Microorganisms 6:69. doi: 10.3390/microorganisms6030069
Elad, Y., and Pertot, I. (2014). Climate change impact on plant pathogens and plant diseases. J. Crop. Improv. 28, 99–139. doi: 10.1080/15427528.2014.865412
Elad, Y., Williamson, B., Tudzynski, P., and Delen, N. (2007). “Botrytis spp. and diseases they cause in agricultural systems – An introduction,” in Botrytis: Biology, Pathology and Control, eds Y. Elad, B. Williamson, P. Tudzynski, and N. Delen (Dordrecht: Springer), 1–8.
Fisher, M. C., Henk, D. A., Briggs, C. J., Brownstein, J. S., Madoff, L. C., McCraw, S. L., et al. (2012). Emerging fungal threats to animal, plant and ecosystem health. Nature 484, 186–194. doi: 10.1038/nature10947
Galgóczy, L., Borics, A., Virágh, M., Ficze, H., Váradi, G., Kele, Z., et al. (2017). Structural determinants of Neosartorya fischeri antifungal protein (NFAP) for folding, stability and antifungal activity. Sci. Rep. 7:1963. doi: 10.1038/s41598-017-02234-w
Galgóczy, L., Kovács, L., and Vágvölgyi, C. S. (2010). “Defensin-like antifungal proteins secreted by filamentous fungi,” in Current Research, Technology and Education Topics in Applied Microbiology and Microbial Biotechnology, Vol. 1., Microbiology Book Series-Number 2, ed. A. Méndez-Vilas (Bajadoz: Formatex), 550–559.
Garrigues, S., Gandía, M., Borics, A., Marx, F., Manzanares, P., and Marcos, J. F. (2017). Mapping and identification of antifungal peptides in the putative antifungal protein AfpB from the filamentous fungus Penicillium digitatum. Front. Microbiol. 8:592. doi: 10.3389/fmicb.2017.00592
Garrigues, S., Gandía, M., Castillo, L., Coca, M., Marx, F., Marcos, J. F., et al. (2018). Three antifungal proteins from Penicillium expansum: different patterns of production and antifungal activity. Front. Microbiol. 9:2370. doi: 10.3389/fmicb.2018.02370
Garrigues, S., Gandía, M., and Marcos, J. F. (2016). Occurrence and function of fungal antifungal proteins: a case study of the citrus postharvest pathogen Penicillium digitatum. Appl. Microbiol. Biotechnol. 100, 2243–2256. doi: 10.1007/s00253-015-7110-3
Gasteiger, E., Hoogland, C., Gattiker, A., Duvaud, S., Wilkins, M. R., Appel, R. D., et al. (2005). “Protein identification and analysis tools on the ExPASy server,” in The Proteomics Protocols Handbook, ed. J. M. Walker (Totowa, NJ: Humana Press), 571–607. doi: 10.1385/1-59259-584-7:531
Hajdu, D., Huber, A., Czajlik, A., Tóth, L., Kele, Z., Kocsubé, S., et al. (2019). Solution structure and novel insights into phylogeny and mode of action of the Neosartorya (Aspergillus) fischeri antifungal protein (NFAP). Int. J. Biol. Macromol. 129, 511–522. doi: 10.1016/j.ijbiomac.2019.02.016
Hawkins, N. J., Bass, C., Dixon, A., and Neve, P. (2019). The evolutionary origins of pesticide resistance. Biol. Rev. Camb. Philos. Soc. 94, 135–155. doi: 10.1111/brv.12440
Jeger, M., Pautasso, M., and Stack, J. (2011). “Climate, globalization, and trade: impacts on dispersal and invasion of fungal plant pathogens,” in Fungal Diseases - An Emerging Threat to Human, Animal, and Plant Health, Workshop Summary, eds L. A. Olsen, E. R. Choffnes, D. A. Relman, and L. Pray (Washington, DC: The National Academies Press), 273–296.
Jung, Y.-J., and Kang, K.-K. (2014). Application of antimicrobial peptides for disease control in plants. Plant Breed. Biotech. 2, 1–13. doi: 10.9787/PBB.2014.2.1.001
Kaiserer, L., Oberparleiter, C., Weiler-Goerz, R., Burgstaller, W., Leiter, E., and Marx, F. (2003). Characterization of the Penicillium chrysogenum antifungal protein PAF. Arch. Microbiol. 180, 204–210. doi: 10.1007/s00203-003-0578-8
Kovács, L., Virágh, M., Takó, M., Papp, T., Vágvölgyi, C., and Galgóczy, L. (2011). Isolation and characterization of Neosartorya fischeri antifungal protein (NFAP). Peptides 32, 1724–1731. doi: 10.1016/j.peptides.2011.06.022
Kovács, R., Holzknecht, J., Hargitai, Z., Papp, C., Farkas, A., Borics, A., et al. (2019). In vivo applicability of Neosartorya fischeri antifungal protein 2 (NFAP2) in treatment of vulvovaginal candidiasis. Antimicrob. Agents Chemother. 63:e1777. doi: 10.1128/AAC.01777-18
Kumar, P., Kizhakkedathu, J. N., and Straus, S. K. (2018). Antimicrobial peptides: diversity, mechanism of action and strategies to improve the activity and biocompatibility in vivo. Biomolecules 8:E4. doi: 10.3390/biom8010004
Leiter, É, Gáll, T., Csernoch, L., and Pócsi, I. (2017). Biofungicide utilizations of antifungal proteins of filamentous ascomycetes: current and foreseeable future developments. Biocontrol 62, 125–138. doi: 10.1007/s10526-016-9781-9
López-García, B., Moreno, A. B., San Segundo, B., De los Ríos, V., Manning, J. M., Gavilanes, J., et al. (2010). Production of the biotechnologically relevant AFP from Aspergillus giganteus in the yeast Pichia pastoris. Protein Expr. Purif. 70, 206–210. doi: 10.1016/j.pep.2009.11.002
Lucas, J. A., Hawkins, N. J., and Fraaije, B. A. (2015). The evolution of fungicide resistance. Adv. Appl. Microbiol. 90, 29–92. doi: 10.1016/bs.aambs.2014.09.001
Lucht, J. M. (2015). Public acceptance of plant biotechnology and GM crops. Viruses 7, 4254–4281. doi: 10.3390/v7082819
McDonald, B. A., and Stukenbrock, E. H. (2016). Rapid emergence of pathogens in agro-ecosystems: global threats to agricultural sustainability and food security. Philos. Trans. R. Soc. Lond. B Biol. Sci. 371:20160026. doi: 10.1098/rstb.2016.0026
Meng, S., Xu, H., and Wang, F. (2010). Research advances of antimicrobial peptides and applications in food industry and agriculture. Curr. Protein Pept. Sci. 11, 264–273. doi: 10.2174/138920310791233369
Moreno, A. B., Del Pozo, A. M., Borja, M., and Segundo, B. S. (2003). Activity of the antifungal protein from Aspergillus giganteus against Botrytis cinerea. Phytopathology 93, 1344–1353. doi: 10.1094/PHYTO.2003.93.11.1344
Moreno, A. B., Martínez Del Pozo, A., and San Segundo, B. (2006). Biotechnologically relevant enzymes and proteins. Antifungal mechanism of the Aspergillus giganteus AFP against the rice blast fungus Magnaporthe grisea. Appl. Microbiol. Biotechnol. 72, 883–895. doi: 10.1007/s00253-006-0362-1
Palicz, Z., Gáll, T., Leiter, E., Kollár, S., Kovács, I., Miszti-Blasius, K., et al. (2016). Application of a low molecular weight antifungal protein from Penicillium chrysogenum (PAF) to treat pulmonary aspergillosis in mice. Emerg. Microbes Infect. 5:e114. doi: 10.1038/emi.2016.116
Palicz, Z., Jenes, A., Gáll, T., Miszti-Blasius, K., Kollár, S., Kovács, I., et al. (2013). In vivo application of a small molecular weight antifungal protein of Penicillium chrysogenum (PAF). Toxicol. Appl. Pharmacol. 269, 8–16. doi: 10.1016/j.taap.2013.02.014
Rupp, S., Weber, R. W., Rieger, D., Detzel, P., and Hahn, M. (2017). Spread of Botrytis cinerea strains with multiple fungicide resistance in German horticulture. Front. Microbiol. 7:2075. doi: 10.3389/fmicb.2016.02075
Sagaram, U. S., Pandurangi, R., Kaur, J., Smith, T. J., and Shah, D. M. (2011). Structure-activity determinants in antifungal plant defensins MsDef1 and MtDef4 with different modes of action against Fusarium graminearum. PLoS One 6:e18550. doi: 10.1371/journal.pone.0018550
Sani, M. A., and Separovic, F. (2016). How membrane-active peptides get into lipid membranes. Acc. Chem. Res. 49, 1130–1138. doi: 10.1021/acs.accounts.6b00074
Savary, S., Ficke, A., Aubertot, J. N., and Hollier, C. (2012). Crop losses due to diseases and their implications for global food production losses and food security. Food Sec. 4, 519–537. doi: 10.1007/s00203-017-1426-6
Snowden, A. (1992). “Solanaceous fruit vegetables,” in Post-Harvest Diseases and Disorders of Fruits and Vegetables, ed. A. Snowden (London: CRC Press), 53–93.
Sonderegger, C., Galgóczy, L., Garrigues, S., Fizil, A., Borics, A., Manzanares, P., et al. (2016). A Penicillium chrysogenum-based expression system for the production of small, cysteine-rich antifungal proteins for structural and functional analyses. Microb. Cell Fact. 15:192. doi: 10.1186/s12934-016-0586-4
Sonderegger, C., Váradi, G., Galgóczy, L., Kocsubé, S., Posch, W., Borics, A., et al. (2018). The evolutionary conserved γ-core motif influences the anti-Candida activity of the Penicillium chrysogenum antifungal protein PAF. Front. Microbiol. 9:1655. doi: 10.3389/fmicb.2018.01655
Szappanos, H., Szigeti, G. P., Pál, B., Rusznák, Z., Szucs, G., Rajnavölgyi, E., et al. (2005). The Penicillium chrysogenum-derived antifungal peptide shows no toxic effects on mammalian cells in the intended therapeutic concentration. Naunyn Schmiedebergs Arch. Pharmacol. 371, 122–132. doi: 10.1007/s00210-004-1013-7
Szappanos, H., Szigeti, G. P., Pál, B., Rusznák, Z., Szucs, G., Rajnavölgyi, E., et al. (2006). The antifungal protein AFP secreted by Aspergillus giganteus does not cause detrimental effects on certain mammalian cells. Peptides 27, 1717–1725. doi: 10.1016/j.peptides.2006.01.009
Tagliabue, G. (2017). The EU legislation on “GMOs” between nonsense and protectionism: an ongoing schumpeterian chain of public choices. GM Crops Food 8, 57–73. doi: 10.1080/21645698.2016.1270488
Theis, T., Marx, F., Salvenmoser, W., Stahl, U., and Meyer, V. (2005). New insights into the target site and mode of action of the antifungal protein of Aspergillus giganteus. Res. Microbiol. 156, 47–56. doi: 10.1016/j.resmic.2004.08.006
Tóth, L., Boros, É, Poór, P., Ördög, A., Kele, Z., Váradi, G., et al. (2020). The potential use of the Penicillium chrysogenum antifungal protein PAF, the designed variant PAFopt and its γ-core peptide Pγopt in plant protection. Microb. Biotechnol. [Epub ahead of print], doi: 10.1111/1751-7915.13559
Tóth, L., Kele, Z., Borics, A., Nagy, L. G., Váradi, G., Virágh, M., et al. (2016). NFAP2, a novel cysteine-rich anti-yeast protein from Neosartorya fischeri NRRL 181: isolation and characterization. AMB Express 6:75. doi: 10.1186/s13568-016-0250-8
Tóth, L., Váradi, G., Borics, A., Batta, G., Kele, Z., Vendrinszky, M., et al. (2018). Anti-candidal activity and functional mapping of recombinant and synthetic Neosartorya fischeri antifungal protein 2 (NFAP2). Front. Microbiol. 9:393. doi: 10.3389/fmicb.2018.00393
Vila, L., Lacadena, V., Fontanet, P., Martinez del Pozo, A., and San Segundo, B. (2001). A protein from the mold Aspergillus giganteus is a potent inhibitor of fungal plant pathogens. Mol. Plant Microbe Interact. 14, 1327–1331. doi: 10.1094/MPMI.2001.14.11.1327
Virágh, M., Marton, A., Vizler, C., Tóth, L., Vágvölgyi, C., Marx, F., et al. (2015). Insight into the antifungal mechanism of Neosartorya fischeri antifungal protein. Protein Cell 6, 518–528. doi: 10.1007/s13238-015-0167-z
Virágh, M., Vörös, D., Kele, Z., Kovács, L., Fizil, A., Lakatos, G., et al. (2014). Production of a defensin-like antifungal protein NFAP from Neosartorya fischeri in Pichia pastoris and its antifungal activity against filamentous fungal isolates from human infections. Protein Expr. Purif. 94, 79–84. doi: 10.1016/j.pep.2013.11.003
Keywords: Neosartorya fischeri antifungal protein, γ-core peptides, biofungicide, cytotoxicity, crop protection
Citation: Tóth L, Váradi G, Boros É, Borics A, Ficze H, Nagy I, Tóth GK, Rákhely G, Marx F and Galgóczy L (2020) Biofungicidal Potential of Neosartorya (Aspergillus) Fischeri Antifungal Protein NFAP and Novel Synthetic γ-Core Peptides. Front. Microbiol. 11:820. doi: 10.3389/fmicb.2020.00820
Received: 24 January 2020; Accepted: 06 April 2020;
Published: 13 May 2020.
Edited by:
Dilip Shah, Donald Danforth Plant Science Center, United StatesReviewed by:
Siva L. S. Velivelli, Donald Danforth Plant Science Center, United StatesFuguo Xing, Institute of Food Science and Technology (CAAS), China
Copyright © 2020 Tóth, Váradi, Boros, Borics, Ficze, Nagy, Tóth, Rákhely, Marx and Galgóczy. This is an open-access article distributed under the terms of the Creative Commons Attribution License (CC BY). The use, distribution or reproduction in other forums is permitted, provided the original author(s) and the copyright owner(s) are credited and that the original publication in this journal is cited, in accordance with accepted academic practice. No use, distribution or reproduction is permitted which does not comply with these terms.
*Correspondence: László Galgóczy, Z2FsZ29jemlAYmlvLnUtc3plZ2VkLmh1