- 1School of Biology and Environmental Science, Earth Institute, University College Dublin, Dublin, Ireland
- 2School of Life Sciences, University of Essex, Colchester, United Kingdom
- 3Teagasc Environment Research Centre, Wexford, Ireland
- 4Soil Biology Group, Wageningen University & Research, Wageningen, Netherlands
- 5SRUC, Crop & Soil Systems Research Group, Edinburgh, United Kingdom
The fate of future food productivity depends primarily upon the health of soil used for cultivation. For Atlantic Europe, increased precipitation is predicted during both winter and summer months. Interactions between climate change and the fertilization of land used for agriculture are therefore vital to understand. This is particularly relevant for inorganic phosphorus (P) fertilization, which already suffers from resource and sustainability issues. The soil microbiota are a key indicator of soil health and their functioning is critical to plant productivity, playing an important role in nutrient acquisition, particularly when plant available nutrients are limited. A multifactorial, mesocosm study was established to assess the effects of increased soil water availability and inorganic P fertilization, on spring wheat biomass, soil enzymatic activity (dehydrogenase and acid phosphomonoesterase) and soil bacterial community assemblages. Our results highlight the significance of the spring wheat rhizosphere in shaping soil bacterial community assemblages and specific taxa under a moderate soil water content (60%), which was diminished under a higher level of soil water availability (80%). In addition, an interaction between soil water availability and plant presence overrode a long-term bacterial sensitivity to inorganic P fertilization. Together this may have implications for developing sustainable P mobilization through the use of the soil microbiota in future. Spring wheat biomass grown under the higher soil water regime (80%) was reduced compared to the constant water regime (60%) and a reduction in yield could be exacerbated in the future when grown in cultivated soil that have been fertilized with inorganic P. The potential feedback mechanisms for this need now need exploration to understand how future management of crop productivity may be impacted.
Introduction
Soil is an invaluable commodity urgently in need of protection if we are to support the growing global human population (Arneth et al., 2019). This is particularly poignant in the face of climate change, as 95% of our food comes directly or indirectly from soil (FAO, 2015; Spanner, 2015), with a 70% increase in demand predicted by 2050 (Dubey et al., 2019). Grassland cultivation has been a tool for increasing land used for agricultural purposes (Qi et al., 2012) and management of these systems has steadily intensified since the 1960’s (Isselstein et al., 2005). As this looks set to continue, optimizing future grassland management, whilst sustaining a healthy soil environment is therefore vital (Lemanceau et al., 2015).
A key component to sustaining soil health is through the soil microbiome (Glick, 2018; Dubey et al., 2019). As the drivers of biogeochemical nutrient cycling, microbes disproportionately influence soil processes, plants and wider ecosystem functioning (Falkowski et al., 2008; Jansson and Hofmockel, 2019), and can form a range of symbioses (Lambers et al., 2009). However, climate change impacts on microbial communities, and the repercussions this may cause for humanity are not well understood, despite recent efforts to promote awareness (Cavicchioli et al., 2019; Hartman and Tringe, 2019; Jansson and Hofmockel, 2019; Timmis et al., 2019).
Across Atlantic Europe, robust increases in heavy precipitation are predicted for both winter and summer months (IPCC, 2014). With increased rainfall and flooding, land degradation (Arneth et al., 2019) and soil erosion can be exaggerated, particularly for agricultural soils where management has weakened structure (St Clair and Lynch, 2010). Excessive periods of waterlogging can increase nutrient loss via overland flow (Di and Cameron, 2002; McDowell et al., 2003), impact root development and soil nutrient availability (St Clair and Lynch, 2010; Hamonts et al., 2013). As a result, fertilization of soil and the feedback onto plant productivity will likely be effected by heightened periods of rainfall. For inorganic phosphorus (P) fertilization, this is particularly concerning due to the political, environmental and economic issues already dominating P resource availability and production (Elser et al., 2007; EU Commission, 2011; Ceci et al., 2018).
As concerns surrounding inorganic P availability within soil have increased, research exploring the soil microbiota as a sustainable tool for P mobilization has also increased. Whilst some studies report an insensitivity of soil microbiota to conservative application rates of inorganic P (Beauregard et al., 2010; Wakelin et al., 2012; Gosling et al., 2013; Chen et al., 2014), bacterial communities appear particularly responsive when soils are unfertilized (Mander et al., 2012; Tan et al., 2013; Randall et al., 2019). This has revealed bacterial taxa with potential roles in improving soil P mobilization (Mander et al., 2012; Lakshmanan et al., 2014; Randall et al., 2019), however, study of microbial responses to elevated soil moisture content and P fertilization within temperate regions is unexplored.
To-date, soil microbial studies concerning increased soil moisture focus largely on the effect of re-wetting arid soils and the microbiome of flooded paddy fields (Fierer et al., 2003; Austin et al., 2004; Huxman et al., 2004; Liu et al., 2009). In such scenarios, microbial community structure and activity profiles have been found to significantly differ between flooded and unflooded soils (Kimura and Asakawa, 2006; Breidenbach and Conrad, 2014), with reductions in microbial biomass, aerobic bacterial and mycorrhizal fungal PLFA biomarkers also being observed (Unger et al., 2009). In addition, microbial functional responses have shown an increased abundance of denitrifiers in soil exposed to elevated water availability (Liu et al., 2014), as well as increased production of the greenhouse gases (GHG) CH4 and N2O, to which microbes contribute significantly (Ferré et al., 2012; Tete et al., 2015). With more frequent and heavier precipitation events, conditions imposed upon temperate regions may select for soil anaerobic bacteria and archaea, the likes which dominate paddy fields post flooding (Liesack et al., 2000; Reim et al., 2012). Such consequences may impact future GHG emissions, as demonstrated when temperate coastal grasslands flood (Gebremichael et al., 2017).
The aim of this study was to test the hypothesis that a long-term difference in inorganic P fertilization, combined with a short-term difference in soil water availability and plant presence will significantly affect soil bacterial community composition, plant biomass and soil enzymatic activity (acid phosphomonoesterase and dehydrogenase activity). To achieve this, a mesocosm, growth chamber study was conducted.
Materials and Methods
Soil Sampling
Soil was sampled from a long established (44 years), inorganic P grazed field trial located in Co. Wexford, Ireland (52°16′ N, 06°30′ W, during August 2020). Details are documented by Randall et al. (2019). Soil from three continually unfertilized (0 kg P ha–1 y–1) (P0) and three continually fertilized field plots, (30 kg P ha–1 y–1) (P30) was sampled (Supplementary Figure 1), ensuring consistent soil texture (Tunney et al., 2010). For each field plot, 25 soil cores were sampled from the top 20 cm of the soil profile using a Dutch auger (4 cm diameter) during August 2012. A composite sample was then made for each field plot by sieving (2 mm) the 25 cores and homogenizing, producing six composite soil samples. Baseline biochemical and physico-chemical properties were then determined. The remainder of each composite sample was stored in separate containers and left to settle in the dark for 1 week at 15°C (Zeng, 2017; Comeau et al., 2018; Randle-Boggis et al., 2018).
Experimental Set-Up
Mesocosms were constructed from plastic trunking (100 cm × 3.6 cm × 3.6 cm) (Radionics Ltd., Dublin). After 1 week of incubating the soil in the dark, the soil water holding capacity (WHC) of each composite field sample (3 × P0 and 3 × P30) was determined (see Supplementary Material). The overall experimental design is presented in Figure 1.
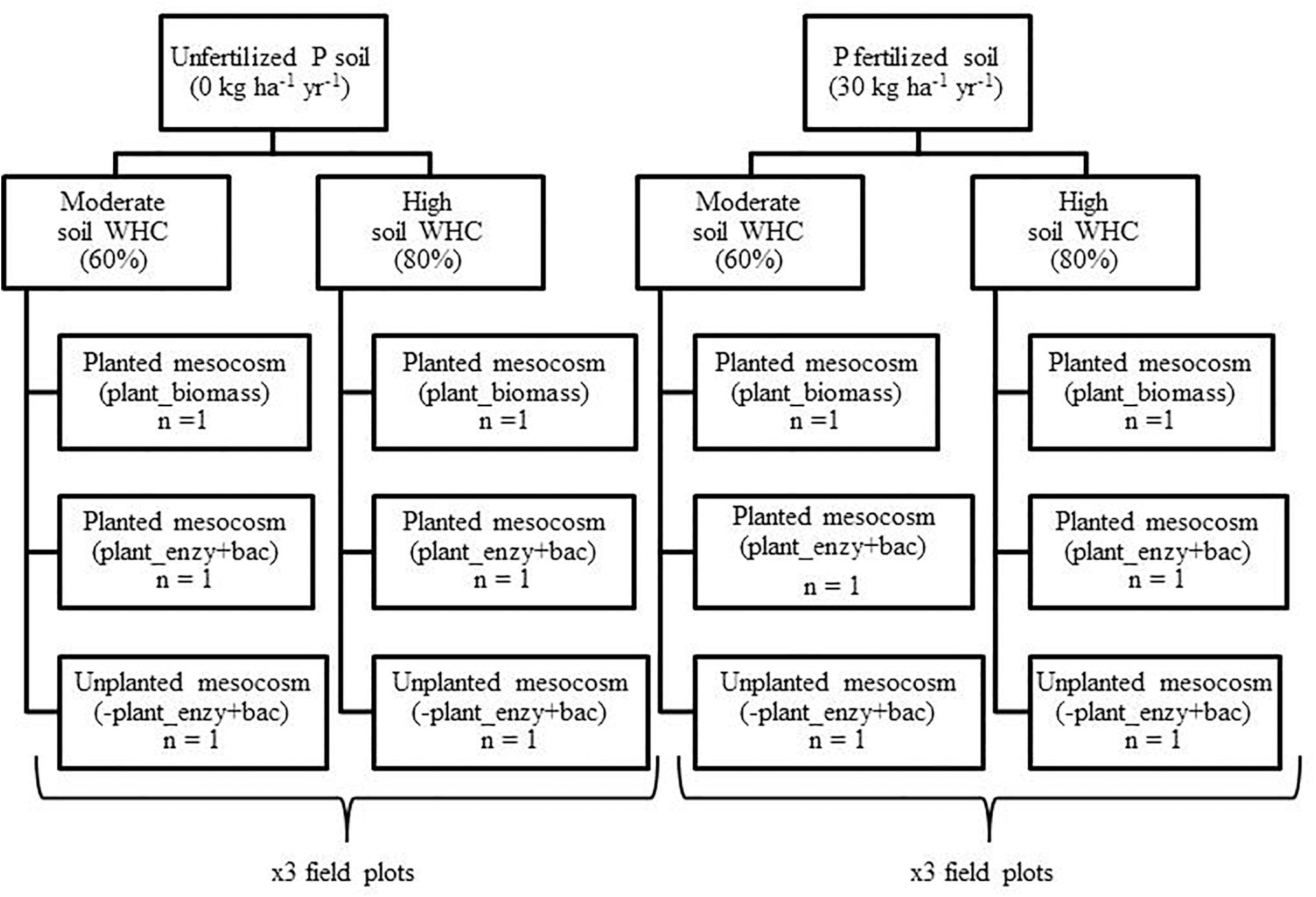
Figure 1. Design of the mesocosm growth chamber experiment. Field soil was sampled from a long-term inorganic phosphorus (P) field trial (44 years), from unfertilized (P0) (n = 3) and fertilized (P30) (30 kg ha–1 y–1) (n = 3) field plots. Soil water holding capacity (WHC) was then manipulated to either 60% or 80% and was maintained. Mesocosms were either sown with an individual seed of spring wheat (T. aestivum), Trappe variety (Goldcrop Ltd., Co. Cork, Ireland), or remained unplanted, serving as negative plant controls. The experiment ran for four months within a growth chamber.
For each of the six composite samples (3 × P0 and 3 × P30), the following protocol was performed; soil was packed into six pre-weighed mesocosms at a bulk density of 1.1 g cm–3 (Dijkstra and Cheng, 2007). For ease, the addition of soil was made in 10 cm sub-sections D1 (0–10 cm) to D8 (70–80 cm) (see Supplementary Material).
Within these six mesocosms, three then had the soil WHC adjusted to 60%, to reflect a moderate soil WHC (60%). The remaining three mesocosms had the soil WHC adjusted to 80%, representing a high soil water treatment (80%) (Chen et al., 2007; Dijkstra and Cheng, 2007; Wang et al., 2016). The final weight of each mesocosm was recorded and was maintained for the duration of the experiment using distilled water (Dijkstra and Cheng, 2007; Lazcano et al., 2014) (see Supplementary Material). The mesocosms detachable front cover provided access for watering and sampling (Supplementary Figure 2).
A single seed of spring wheat (T. aestivum) was then sown into two mesocosms, 5 cm below the surface. Two planted mesocosms were required so one could be used to determine total root biomass (+Plant_biomass), whilst the other was used to determine bulk soil enzymatic activity and bacterial community composition (+Plant_enzy + bac) (Figure 1). The third mesocosm remained unplanted to serve as a negative plant control. This set-up was repeated for the remaining five composite field samples, with the final design consisting of 36 mesocosms.
Growth Chamber Conditions
The experiment ran for a 4 month period and was conducted in a controlled growth chamber (Series 3; Temperature Applied Sciences Ltd.). Chamber parameters were set at 75% humidity with a diurnal light intensity program (16 h daylight, 8 h night) (Haworth et al., 2011, 2013). The temperature regime reflects the 1.5°C increase the IPCC wish to cap global warming (IPCC, 2018). A 1.5°C increase was added to the previous year’s (2011) mean monthly temperatures (March–June) recorded for the area (Met Éireann, 2011). The block temperatures used to simulate the 4 month growing season were, 8.3, 10.0, 12.5, and 16°C.
Experimental Sampling Protocol
After 4 months, all 36 mesocosms were destructively sampled. At this stage, the plants had reached the end of their vegetative growth stage (Zadoks et al., 1974). All sampling was conducted under sterile conditions, with the front of the trunking removed to access soil within each mesocosm. All planted mesocosms were carefully inspected to identify depth of root growth within each of the 10 cm sub-sections (D1–D8). Across all planted mesocosms, roots were present up to sub-section D5.
Plant Biomass (+plant_biomass)
To determine the shoot and root biomass of spring wheat, one planted mesocosm per treatment, per original field plot was sampled (Figure 1, +plant_biomass). The intact plant was carefully removed from the soil. Shoots and roots were severed and the fresh weights of both were recorded. Roots and shoots were then individually oven dried for 48 h at 60°C to determine the dry weight.
Soil Bacterial Communities and Bulk Soil Enzymatic Activity (+plant_enzy + bac, –plant_enzy + bac)
The remaining planted and all unplanted mesocosms were sampled to determine bacterial community composition and bulk soil enzymatic activity (Figure 1, +plant_enzy + bac and –plant_enzy + bac).
For planted mesocosms, sampling was conducted separately for each sub-section where roots were present (D1–D5). Planted bulk and rhizosphere soil was also sampled within each sub-section for molecular characterization of bacterial communities. Rhizosphere soil was considered as soil adhering to root section post 1 min of shaking by hand. The remaining soil within the mesocosm was considered as the bulk soil. In unplanted mesocosms, the equivalent sub-sections (D1–D5) were sampled, with all soil considered as bulk. Soil samples intended for molecular analysis were then frozen (−20°C).
At the same time, additional bulk soil samples were collected within planted (+plant_enzy + bac) and unplanted (–plant_enzy + bac) mesocosm sub-sections (D1–D5) to determine both dehydrogenase and acid phosphomonoesterase activity and soil physico-chemcial properties.
Soil Biochemical and Physico-Chemical Properties
For details of how to determine the gravimetric soil water content and WHC please refer to the Supplementary Material.
Soil pH
Soil pH was determined using a soil: deionized water ratio of 1:2 (w/v) (McCormack, 2002), using 5 g of fresh sieved soil and measured using a WTW pH526 pH meter (Labsource, United States).
Soil Available Inorganic Phosphorus
Soil available inorganic P was determined using the Morgan’s extraction method (McCormack, 2002) as described by Massey et al. (2012) and measured spectrophotometrically at 880 nm using the phosphomolybdate method (Murphy and Riley, 1962).
Dehydrogenase Activity
1 g of the freshly sampled soil was used to determine dehydrogenase activity, using the method described by Alef and Nannipieri (1995). The optical density of the supernatant was measured at 546 nm using a spectrophotometer (Helios Unicam, United Kingdom). Dehydrogenase activity was calculated using standard curves constructed with known concentrations of TPF and expressed as μg TPF g–1 dry soil 24 h–1.
Acid Phosphomonoesterase Activity
1 g of the freshly sampled soil was used to determine acid phosphomonoesterase activity using a modified method of Tabatabai and Bremner (1969), with the toluene step removed. The optical density of the supernatant was measured at 400 nm using a spectrophotometer (Helios Unicam, United Kingdom). Acid phosphomonoesterase activity was calculated with respect to standard curves from known concentrations of p-nitrophenol and expressed as μg p-NP g–1 soil h–1.
DNA Extraction and 16S rRNA Bacterial Amplicon Library Creation
DNA extractions followed a modified version of the method by Griffiths et al. (2000) (Storey et al., 2014). Triplicate extractions were performed for each biological sample and pooled. DNA was extracted from the D1 section and the deepest section containing roots (D5). For planted mesocosms (+plant_enzy + bac), DNA was extracted from bulk and rhizosphere soil from both the D1 and D5 sub-sections. For the unplanted mesocosms (–plant_enzy + bac), DNA was extracted from soil within D1 and D5 sub-sections. Library preparation for high throughput sequencing (HTS) followed Randall et al. (2019).
Bioinformatic Pipeline for High Throughput Sequencing (HTS) Data
After downloading the raw sequencing reads, the forward and reverse reads were combined into contigs using Mothur (Schloss et al., 2009) and uploaded to the NCBI SRA (PRJNA610548). Read processing and quality control (QC) followed Randall et al. (2019). After QC, identical sequences were grouped into “unique” sequences. Chimeric sequences were identified using the UCHIME algorithm within Mothur with 142,556 chimeric sequences removed. From 3,467,214 contigs, 1,723,604 paired end reads were constructed and 516,752 unique sequences detected. 201,430 sequences were assigned to operational taxonomic units (OTUs) using the “cluster” command and the average neighbor algorithm. OTU-based analyses were performed using a cutoff of 0.03. Taxonomy was assigned to the aligned sequences by comparing data to the SILVA database for bacteria (arb-silva.de/silva-license-information) and relative abundances were calculated.
Statistical Data Analysis
All univariate analyses were performed using Minitab v15 (Minitab Ltd.). For the analysis of baseline soil biochemical and physico-chemical parameters (pH, available inorganic P, dehydrogenase and acid phosphomonoesterase activity) the design was an independent between-subjects design, with inorganic P fertilization rate (P0 vs. P30) as the independent variable.
Univariate data from the experiment was analyzed by applying general linear models (GLM) (Minitab Ltd.) to test main and interactive effects of the independent variables, inorganic P fertilization rate (P0 vs. P30), soil water content (60% vs. 80% soil WHC) and where appropriate, plant (unplanted vs. planted) on the dependent variables; spring wheat shoot and root biomass and bulk soil dehydrogenase and acid phosphomonoesterase activity. Significance levels were set at the 0.05 probability level.
Multivariate analyses to test for differences in beta diversity of bacterial assemblages were performed using PRIMER-E v6.1.10 with the PERMANOVA +add-on package (PRIMER-E Ltd., Plymouth, United Kingdom) (Anderson, 2001). The relative abundance data for bacterial communities were initially transformed to the 4th root (Clarke and Warwick, 2001). The dataset was sub-divided and analyzed to answer specific questions for paired samples (i.e., D1 vs. D5 sub-sections and bulk vs. rhizosphere soil) and Bray-Curtis resemblance matrices were generated (Clarke et al., 2006). Data was tested for homogeneity of variances at the lowest level of factor combinations using the PermDisp function in PRIMER-E and permutation analysis of variance (PERMANOVA) were conducted, applying 9,999 permutations of residuals under a reduced model.
Results
Baseline Soil Characteristics
Prior to the experimental manipulation, baseline field soil characteristics were determined (Table 1). A significant main effect of inorganic P fertilization rate was detected for soil pH, available inorganic P concentration and acid phosphomonoesterase activity (Table 1). Across all the plots, unfertilized (P0) soil had significantly lower pH [t(6) = 23.09, p = 0.003] and available inorganic P [t(6) = 123.00, p = 0.001] compared to fertilized (P30) soil (Table 1).
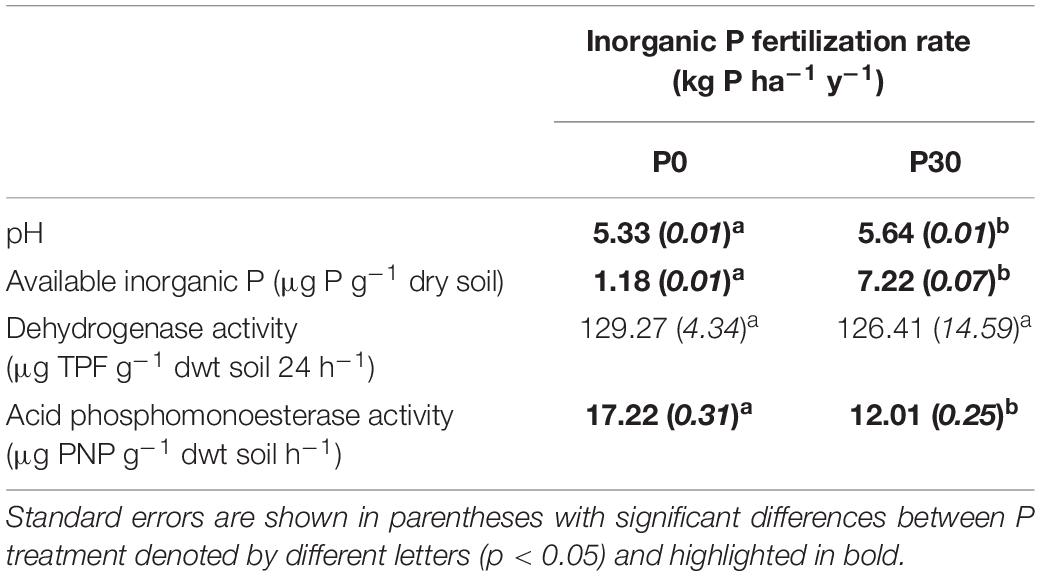
Table 1. Mean baseline soil pH, available inorganic phosphorus (P) concentrations and enzymatic activities measured in unfertilized (P0) (n = 3) and fertilized (P30) field soil (n = 3) from a long running (44 years) P fertilization trial (prior to experimental manipulation).
For baseline enzymatic activity measurements, whilst dehydrogenase activity did not significantly differ between unfertilized (P0) and fertilized (P30) soil (Table 1), acid phosphomonoesterase activity was significantly higher in the unfertilized (P0) soil compared to the fertilized (P30) soil [t(6) = 5.00, p = 0.003] (Table 1).
Aboveground and Belowground Spring Wheat Biomass at Harvest
Both the shoot [F(1,12) = 30.50, p = 0.001] and root spring wheat biomass [F(1,12) = 62.64, p = 0.001] were significantly impacted by the main effect of soil water availability, whilst an independent effect of P fertilization was not observed (Figure 2). For the roots, significantly lower biomass was observed for spring wheat grown under the higher water (80%) compared to the moderate soil water treatment (60%), regardless of inorganic P fertilization (Figure 2B).
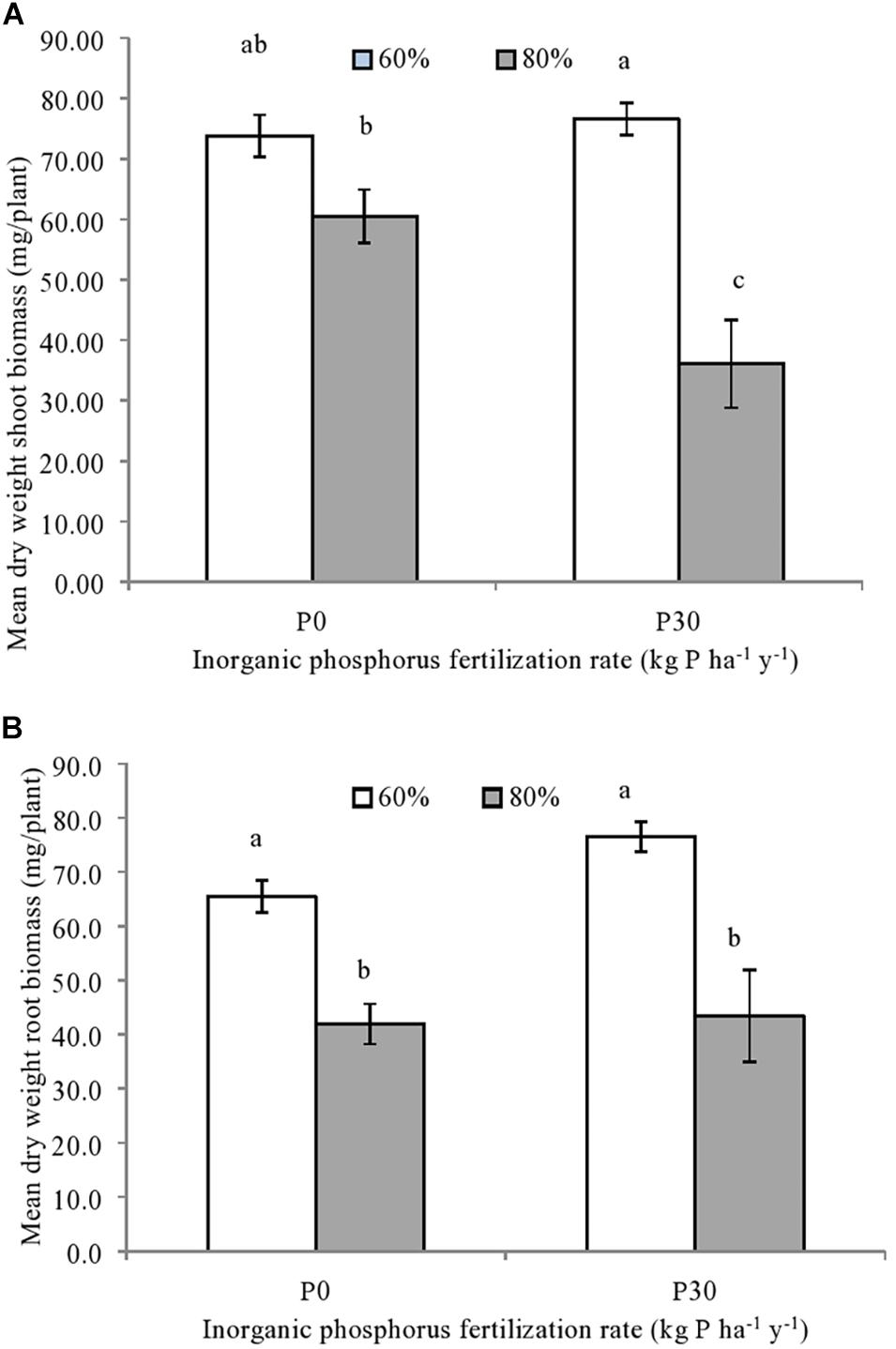
Figure 2. (A) Mean shoot and (B) root biomass of individually sown spring wheat (T. aestivum), grown within mesocosms using field soil sampled from a long-term inorganic phosphorus (P) fertilization experiment (44 years). Soil used was sampled from field plots that were either unfertilized (P0) (n = 3) or fertilized at a rate of 30 kg P ha–1 y–1 (P30) (n = 3). Soil water holding capacity was altered to 60% or 80% and was maintained within a growth chamber for four months. Error bars represent standard error of the mean. Significant differences in mean dry weight biomass are indicated by differences in letters (p < 0.05).
As for roots, regardless of P fertilization, shoot biomass was significantly reduced when grown under the higher soil water treatments (80%) compared to the moderate treatment (60%). An additive reduction in shoot biomass was due to the significant interaction between inorganic P fertilization rate and soil water availability [F(1,12) = 7.29, p = 0.027] (Figure 2A). This meant that while wheat plants grown in the fertilized soil under the higher soil water treatment (P30-80%) had the lowest shoot biomass (Figure 2A), shoot biomass from unfertilized soil exposed to same water regime (P0-80%) was significantly lower than fertilized soil maintained at the moderate water content (P30-60%) (Figure 2A).
Soil Bacterial Community Responses at Harvest
A Generally Consistent Soil Bacterial Microbiome
No main effect of positioning along the developing root system of spring wheat was detected for bacterial community structure (D1 vs. D5) (Supplementary Table 1). Subsequent analysis of bacterial beta diversity was performed on the sub-section D5 only. Within this sub-section, further comparison between the bulk and rhizosphere soil found the main effect of soil region did not significantly alter bacterial communities (Supplementary Table 2). Data used for further analysis of bacterial beta diversity was therefore from D5 rhizosphere samples and was considered to be representative of bacterial communities within planted soil.
A Consistent Interaction Between Soil Water Availability and Plant for Bacterial Beta Diversity
Across the experiment, soil water availability, inorganic P fertilization rate and plant presence had consistent and significant independent main effects on the bacterial community structure at multiple taxonomic levels (Table 2). Analysis of the 2nd order interactive terms revealed an additionally consistent and significant interaction between soil water availability and plant presence (Table 2). The 3rd order interaction was not found to be significant (Table 2).
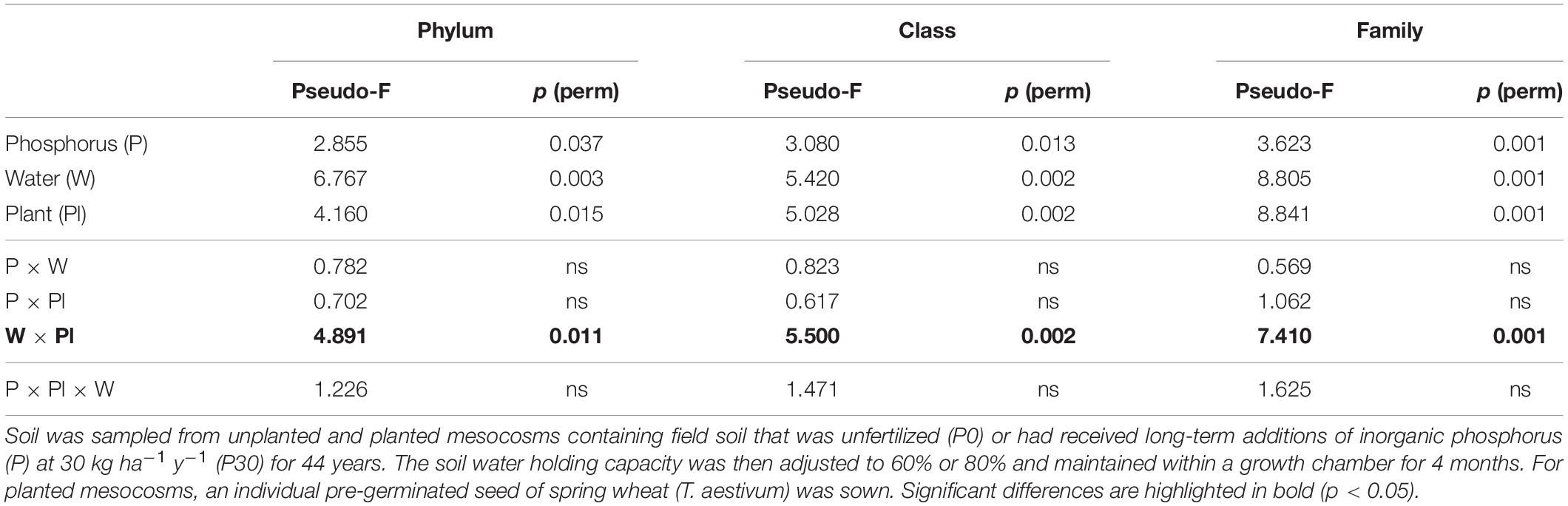
Table 2. PERMANOVA results testing for differences between experimental treatments within the D5 sub-section of mesocosms.
The significant 2nd order interaction between plant presence and soil water was driven by plant presence. The interaction was driven by plant presence, where bacterial community structure differed significantly between planted and unplanted soil only when soil water conditions were moderate (60%), irrespective of long-term inorganic P fertilization (Supplementary Table 3). Additionally, the water regime only significantly impacted bacterial beta diversity when soil was planted. This effect was again regardless of inorganic P fertilization rate (Supplementary Table 4).
Individual Responses of Bacterial Taxa
The top three dominant phyla across all treatments were the Firmicutes (35.3%), Acidiobacteria (16.9%), and Proteobacteria (13.3%) (Supplementary Table 5). On average 104 (±18) bacterial families were identified across all experimental treatments ranging from 85 in P0-60% unplanted soil to 143 in P0-60% planted rhizosphere soil.
Based on the relative abundance data, the top 25 most relatively abundant bacterial families detected across the experiment are presented in Supplementary Table S6. Within this top 25, the individual responses of six bacterial families were found to significantly respond to experimental treatments. These families were, Bacillaceae 1, Clostridiaceae 1, Subdivision3 family incertae sedis, Gp7 family incertae sedis, Thermomonosporaceae, and Planococcaceae (Figure 3).
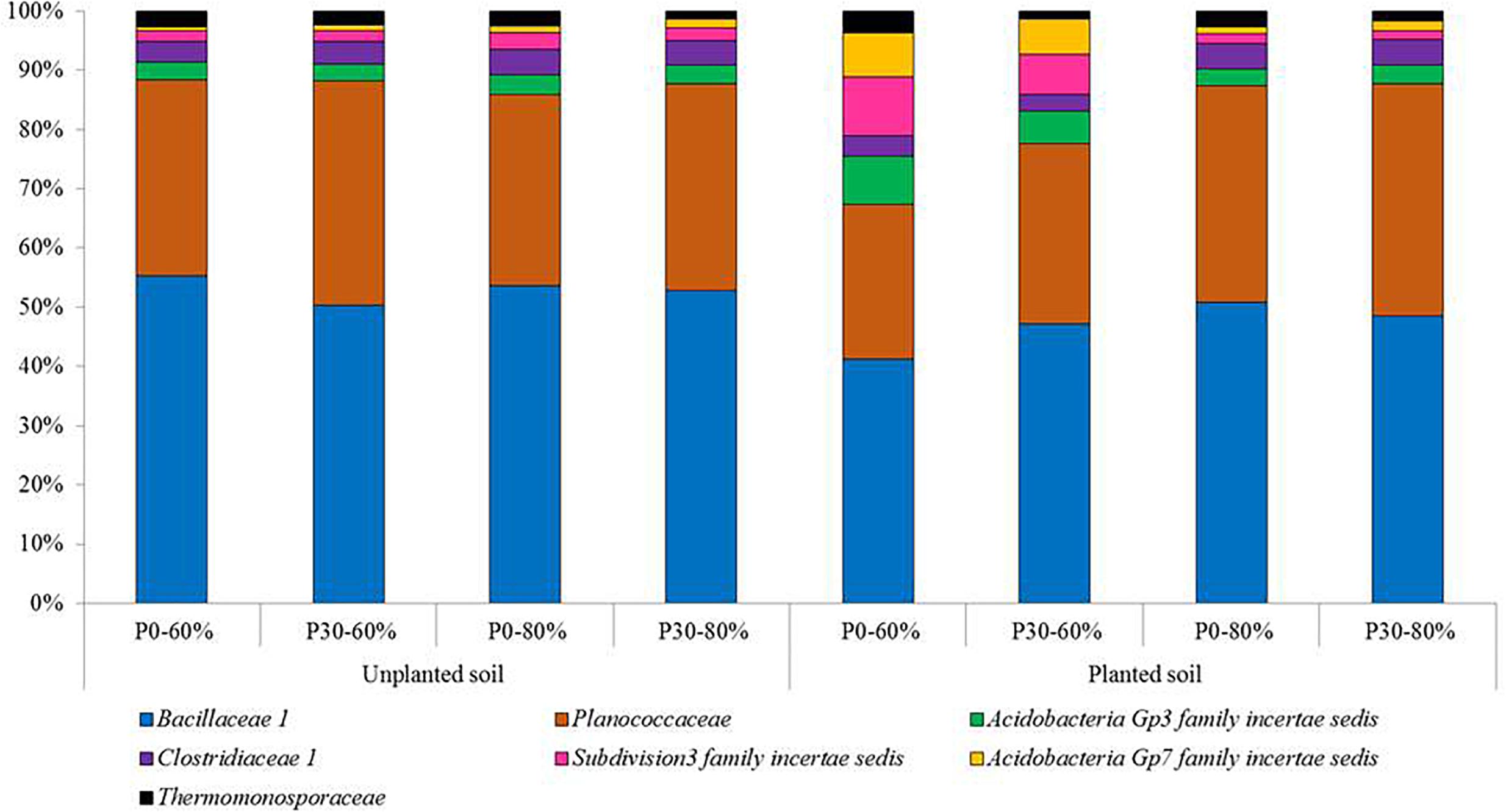
Figure 3. The mean relative abundances of bacterial families significantly responding to experimental treatments from the top 25 most relatively abundant. Experimental treatments are unplanted and planted soil sampled from mesocosms using field soil that was unfertilized (P0) (n = 3) or had received long-term additions of inorganic phosphorus (P) at 30 kg ha–1 y–1 (P30) (n = 3) (44 years). The soil water holding capacity was then adjusted to 60% or 80% and was maintained within a growth chamber for four months. For planted mesocosms, an individual pre-germinated seed of spring wheat (T. aestivum) was sown and the experiment ran for 4 months within a growth chamber. Samples were sent for targeted amplicon sequencing of the bacterial 16S rRNA gene.
Main Effects
For Subdivision3 family incertae sedis, the main effects of inorganic P fertilization, soil water availability and plant presence all had significant main effects on the relative abundance of this bacterial family (Table 3). For Gp7 family incertae sedis, the relative abundance of this family significantly responded to the two main effects of soil water availability and plant presence (Table 3). Different main effects were significant for Bacillaceae 1 and Thermomonosporaceae, with the plant being significant for Bacillaceae 1 and inorganic P fertilization significant for Thermomonosporaceae (Table 3). Clostridiaceae 1 and Planococcaceae were not significantly influenced by any of the three experimental treatments as main effects.
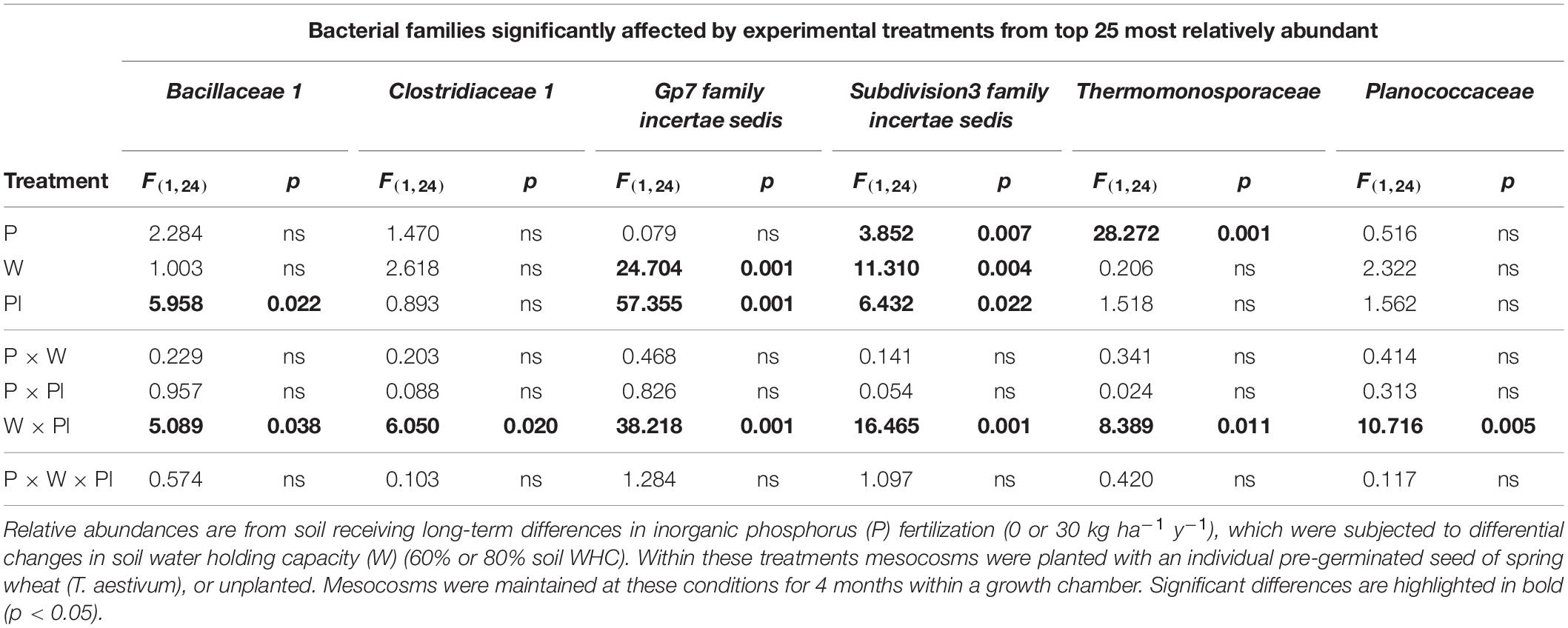
Table 3. Test statistics and p value summary table for bacterial families within the top 25 most relatively abundant that were significantly affected by main and interactive terms across the experiments.
Interactive Effects
Despite an absence of significant main effects for Clostridiaceae 1 and Planococcaceae, all six bacterial families, (Bacillaceae 1, Clostridiaceae 1, Subdivision3 family incertae sedis, Gp7 family incertae sedis, Thermomonosporaceae, and Planococcaceae) were all significantly affected by a consistent 2nd order interaction between soil water availability and plant presence (Table 3).
For the Bacillaceae 1, Planococcaceae and Clostridiaceae 1 these results manifested themselves by a reduced relative abundance in planted soil maintained at the moderate soil water content (60%) compared to equivalent planted soil exposed to the higher soil water content (80%), regardless of inorganic P fertilization rate. In addition, these three families reduced in relative abundance significantly compared to most unplanted treatments (Supplementary Table 6).
For Subdivision3 family incertae sedis and Gp7 family incertae sedis, the opposite response was generally observed, with their relative abundances increasing in the planted soil maintained at the moderate soil water content (60%) compared to other treatments including the unplanted treatments (Supplementary Table 6).
For the Thermomonosporaceae family, whilst not consistently significant across all P30 treatments, a significant reduction in this bacterial family was observed within some fertilized (P30) soil when compared to unfertilized soils (Supplementary Table 6).
Soil Enzymatic Activity at Harvest
The activity measurements of D1 and D5 bulk soil at the end of the experiment for dehydrogenase and acid phosphomonoesterase activity are presented in Figure 4.
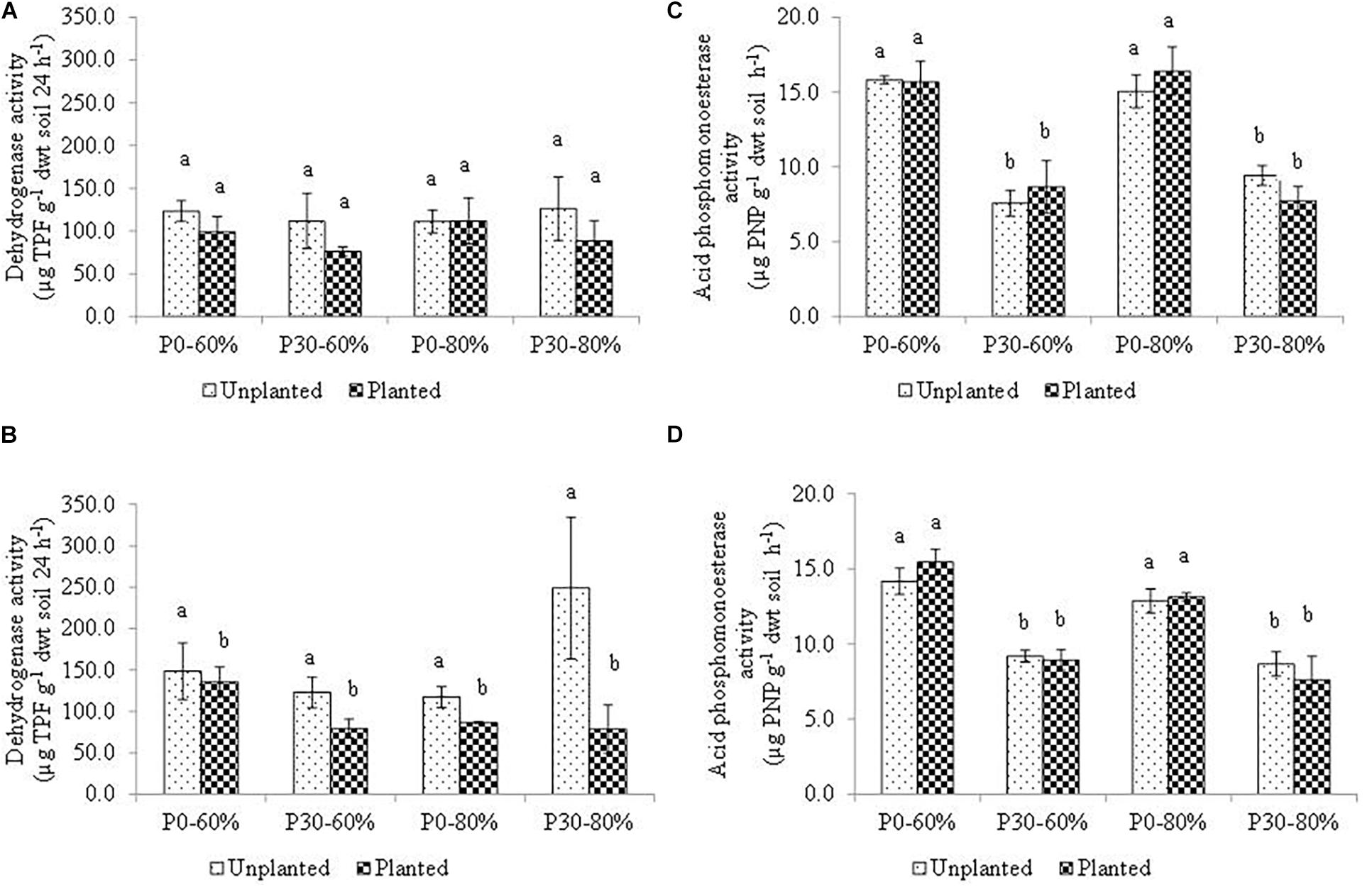
Figure 4. Mean dehydrogenase activity measured in bulk soil within the (A) D1 and (B) D5 and mean acid phosphomonoesterase measured in bulk soil within the (C) D1 and (D) D5 sub-sections of sampled mesocosm. Activity was measured after four months incubation in a growth chamber. Soil used in the experiment originated from an established inorganic phosphorus (P) fertilization field trial (44 years) which had either remained unfertilized (P0) or had received 30 kg ha–1 y–1 (P30) of inorganic P. Soil water holding capacity was altered to 60% and 80% and was maintained within a growth chamber for four months. Error bars represent standard error of the mean (n = 3). Significant differences are indicated by letters (p < 0.05).
Dehydrogenase Activity Along the Soil Profile
Within the top sub-section of the mesocosms, (D1), mean soil dehydrogenase activity did not differ significantly across the experimental treatments as a result of main treatment effects, or due to any of the 2nd, or even the 3rd order interaction (Table 4 and Figure 4A).
At the lower mesocosm sub-section (D5), however, dehydrogenase activity differed significantly due to the main effect of the plant (Table 4), with activity of the unplanted mesocosms showing heightened activity (Figure 4B).
Acid Phosphomonoesterase Activity Along the Soil Profile
For acid phosphomonoesterase activity, a significant independent effect of inorganic P fertilization rate was detected, regardless of position descending the soil profile within the mesocosms (D1 and D5) (Figures 4C,D). The result mirrors the baseline field observation prior to experimental manipulation (Table 1). As a result of this sole main effect, a significantly lower acid phosphomonoesterase activity was determined for all fertilized (P30) treatments, compared to all unfertilized (P0) treatment regardless of water regime and plant presence (Figures 4C,D).
Discussion
The mesocosm study provides insight into complex above and belowground plant-soil-microbial interactions to increased soil water availability and inorganic P fertilization rate. Whilst studies have attempted to model wheat productivity to a range of climate change scenarios (Southworth et al., 2002; Tao and Zhang, 2013; Mohan, 2014; Váry et al., 2015), study of the spring wheat-soil microbiome in this context is unexplored.
Soil used within the study was sampled from an established (44 year), inorganic P fertilization field trial, where differences in total and available inorganic P, soil microbial biomass P and bacterial community structure have been documented (Griffiths et al., 2012; Chen et al., 2014; Randall et al., 2019). The aim of this study was to determine if this effect persisted when combined with a short-term change in soil water availability, whilst exploring the role of the spring wheat rhizosphere as a mediator. Whilst large scale field studies are desirable, parameters such as soil water availability are difficult to control in situ. Mesocosm studies therefore allow such variables to be controlled (Williams and Rice, 2007). Albeit not representative of realistic field scenarios, this study lays foundations for future research, in an area currently understudied.
Soil by its very nature is heterogeneous, as such, equal soil WHC and moisture content between our field plots was unlikely. To control this aspect of the experiment, the soil WHC of each field plot was adjusted to two equal levels (60% and 80%), using an experimental approach typical of similar studies (Barratt et al., 2003; Uhlírová et al., 2005; Chen et al., 2007; Dijkstra and Cheng, 2007). Selection of the moderate soil water treatment (60% WHC) simulated “optimal” moisture content for aerobic microbial processes (Papendick and Campbell, 1981). The 80% soil WHC was selected to represent heightened periods of rainfall, as opposed to flooding events (100% WHC) (Barratt et al., 2003).
Baseline Soil Enzymatic Activity and Soil Characteristics
Despite a lower soil pH in the P0 soils, the average pH of both unfertilized (5.33) and fertilized (P30) (5.64) soils was acidic. This is important, as soil pH is an important driver of inorganic P availability within soils (Alt et al., 2011; Brod et al., 2015) and can be a significant driver of changes in soil microbial communities (Griffiths et al., 2011; Zhalnina et al., 2014).
Measures of soil enzymatic activity can serve as bioindicators of changes within the soil (Henry, 2012; Stone et al., 2016). The two enzymes assayed in the current study provide indication of the P mineralizing (acid phosphomonoesterase) and aerobically active (dehydrogenase) potential of soil (Nannipieri et al., 2011). The significant difference in baseline activity of acid phosphomonoesterase between unfertilized (P0) and P30 fertilized field plots supports the use of this enzymes as an indicator of soil P status. Typically activity increases when available inorganic P concentrations are low (Olander and Vitousek, 2000; Shi et al., 2013; Turner and Joseph Wright, 2014), which is supported by the low concentrations detected at the unfertilized (P0) plots in the current study. The similarity in baseline measurements of dehydrogenase activity between the unfertilized and fertilized (P30) field plots suggests soil aerobic activity has not impacted by long-term differences in soil P status due to P fertilization. Whilst an insensitivity of this enzyme is not uncommon (Chu et al., 2007; Shi et al., 2013), opposing responses have been noted with P fertilization (Beauregard et al., 2010; Luo et al., 2015).
Bulk Soil Enzymatic Activity at Experimental Harvest
Elevated soil water availability has the ability to impact a large portion of the soil microbial community. Waterlogging events have been shown to decrease redox potential, O2 diffusion (Sharma and Swarup, 1988; Yaduvanshi et al., 2012) and concentration to 2% (Belford et al., 1980). As a result, changes to soil processes such as enzyme activities are likely. In the mesocosm experiment, however, the unaltered activity of acid phosphomonoesterase measurements, suggests an insensitivity to elevated water availability compared to long-term differences in soil P status.
As a measure of the aerobic potential of soil, it may be expected for dehydrogenase activity to be negatively impacted by increased soil water availability. Here we failed to observe this. Instead a significant reduction in dehydrogenase activity was measured at the root tip for all planted soil. Whilst difficult to untangle, the rhizosphere can significantly facilitate changes in the soil environment (Luster et al., 2009; Neumann et al., 2009; Philippot et al., 2013), perhaps relevant for dehydrogenase, which is not solely microbial, as is acid phosphomonoesterase. Future work should adopt a molecular functional approach, targeting genes associated with microbial P cycling (Fraser et al., 2014; Ragot et al., 2016) and anaerobic conditions such as methanogenesis (Angel et al., 2011). This will provide a refined measure of the microbial contribution to important soil processes as opposed to broader enzyme responses.
Response of Spring Wheat Biomass at Experimental Harvest
Typically, metrics used to determine wheat quality and grain yield (grain weight and kernels per ear head) develop during the post vegetative growth stage (Hütsch et al., 2019). Nonetheless, the end of the vegetative growth period can provide significant indication of downstream wheat development (Santos and Diola, 2015). It is therefore important to understand how the vegetative growth of spring wheat can be impacted as it may be useful for early stage wheat management.
Known to be sensitive to changes in climate (Dubey et al., 2019), both waterlogging (Araki et al., 2012; Yaduvanshi et al., 2012) and short-term flooding (Sharma and Swarup, 1988) can impact wheat development. This result was mirrored in the current study at the early growth stage. As a globally significant crop, wheat is used within 21% of food sources (Wu et al., 2004; Ortiz et al., 2008), with recent figures of European production reaching 142.7 million tonnes (Eurostat, 2019). Importantly the interaction between P fertilization and soil water content in the current study, may indicate a negative impact on yield as future precipitation increases across Atlantic Europe (IPCC, 2014). Naturally, this requires testing at a larger scale and over longer periods, but we may see costs for the agri-sector, particularly for soils fertilized by inorganic P, such as cultivated grasslands.
Response of Bacterial Communities at Experimental Harvest
Plants significantly contribute and mediate C translocation belowground, via the roots and into the rhizosphere (Lakshmanan et al., 2014). This introduction can influence soil heterotrophic respiration, microbial community composition and activity (Hinsinger et al., 2009; Ai et al., 2013; Skwarylo-Bednarz and Krzepilko, 2013; Nie et al., 2014). By sampling at two proximities away from the root system of spring wheat, the ability of the rhizosphere to shape bacterial communities was assessed. Studies comparing bulk and rhizosphere microbial community assemblages from the same samples often observe differences in structure (Ai et al., 2013; Bakker et al., 2015; Praeg et al., 2019). In the current study, however, this was not so. As demonstrated by the effect of cabbage age in shaping bacterial beta diversity (O’Brien et al., 2018), the experimental and plant establishment time may have influenced this. The need for future studies to run for longer, capturing the entire crop growing season is additionally cemented by this.
Despite no distinction between bulk and rhizosphere bacterial community structure during the 4 month experiment, planted and unplanted soil showed clear distinctions. The significant ability of spring wheat to influence this microbial group is clear, and it is only through the plant that soil water content becomes significant. This result demonstrates the complexity of plant-soil-microbial interactions which will require consideration in future crop production (de Vries and Wallenstein, 2017). It also suggests a heightened sensitivity of wheat associated bacterial communities to soil water content, compared to those within unplanted soil. Under drought experiments, it appears bacteria are sensitive to water fluctuations, more so than fungal communities (Borowik and Wyszkowska, 2016), likely driven by changes in root exudation (de Vries et al., 2018; Gargallo-Garriga et al., 2018; Naylor and Coleman-Derr, 2018). Exploration of both root exudation and the wider soil microbiome within the context of our research is therefore welcomed. By demonstrating a strong water-plant interaction within the current study, we have also shown how elevated soil water availability can override the effect of long-term differences in inorganic P fertilization on soil bacterial community structure (Griffiths et al., 2012; Chen et al., 2014; Randall et al., 2019). The use of key bacterial taxa found within unfertilized (P0) soils with potential roles as sustainable modes of P mobilization (Owen et al., 2015; Stamenković et al., 2018) may therefore be impacted under wetter conditions.
Response of Individual Bacterial Taxa
Collectively, the individual responses of the bacterial families support the significant influence of the spring wheat rhizosphere in shaping the wider bacterial community. The individual responses also support the observation that the plant effect is significantly mediated by the soil water content. These significant responses of individual bacterial taxa may therefore be indicative of changes to communities within future temperate cultivated grassland soils.
Whilst Bacillaceae and Clostridiaceae are commonly found in soil (Mandic-Mulec et al., 2015), the comparatively lower relative abundances of these families and Planococcaceae in planted soil, at moderate (60%) soil water regimes suggests a potential suppression due to this treatment. Members within the Bacillaceae family are mostly aerobic or facultative anaerobic chemoorganotrophs, phylogenetically similar to the Bacillaceae family (Shivaji et al., 2014). The Clostridiaceae, however, are strict anaerobes (Mandic-Mulec et al., 2015). Organisms belonging to both Bacillaceae and Clostridiaceae families can exhibit increased tolerance to environmental stressors. This is due to their endospore forming capabilities (Nessner Kavamura et al., 2013), with Clostridiaceae important within the pre-treatment consortia of anaerobic digesters to enhance CH4 production from plant waste (Suksong et al., 2019). Our response within temperate grassland soil for this family is complemented by significantly greater relative abundance within both anoxic regions of rice paddy fields (Lüdemann et al., 2000), but also upon soil re-wetting events (Liesack et al., 2000; Noll et al., 2005; Reim et al., 2017).
Conversely, the two bacterial families that significantly increased within the same treatment (60% planted soil) belong to the phylum Acidobacteria. Acidobacteria are commonly profiled in grassland soil (Quaiser et al., 2003; da Rocha et al., 2013) and whilst relatively little is known about this diverse phylum, Acidobacteria are detected across a range of habitats (Quaiser et al., 2003; Naether et al., 2012) and are highly abundant (Janssen, 2006), particularly in acidic soils (Jones et al., 2009; Griffiths et al., 2011; Dedysh et al., 2017) as used in the current study. Our results reflect this phylum’s ability to dominate environments with reduced substrate inputs such as the P0 soil (Naether et al., 2012), but they do not fully support it, due to an insensitivity to P fertilization. Previous studies have noted both positive and negative responses by members of the Acidobacteria to soil moisture (Naether et al., 2012). The negative response to heightened water availability observed in planted mesocosms suggests a potentially important sensitivity.
Finally, varied response in the relative abundance of the family Thermomonosporaceae across treatments suggests a complex interaction with plant and soil water availability, influenced by P fertilization or other soil parameters. Unfortunately, limited knowledge surrounds Thermomonosporaceae in terms of responses to environmental perturbations, however, the phylum Actinobacteria, to which it belongs is associated with increased solubilization of inorganic P (Mander et al., 2012). The general decreased relative abundance of Thermomonosporaceae to high P fertilization may support this, however, it is not a significant observation.
Conclusion
This study has attempted to address a knowledge gap in soil-microbial-climate change research that is relevant for Atlantic Europe. Clearly much work is still needed in this area, but, the fact a relatively short-term increase in soil water availability and plant significantly overrode effects of long-term inorganic P fertilization is interesting for the future health of temperate cultivated grassland soils.
Data Availability Statement
The datasets generated for this study can be found in the NCBI SRA repository, with accession number PRJNA610548.
Author Contributions
KR designed the experiment. Design, sampling logistics and sampling were discussed with ED, NC, BG, FB, and RC as was analyses and manuscript preparation. KR set-up and sampled the experiment. KR also conducted the lab work, with SS helping with NGS library preparation and bioinformatics. KR conducted main portion of the bioinformatics for NGS data and all statistical analyses.
Funding
This research was conducted through the Earth and Natural Sciences Doctoral Studies Program, funded under the Program for Research in Third-Level Institutions, and co-funded under the European Regional Development Fund.
Conflict of Interest
The authors declare that the research was conducted in the absence of any commercial or financial relationships that could be construed as a potential conflict of interest.
Acknowledgments
We are grateful to technical staff at Teagasc Environment Research Centre. In addition we are grateful to Dr. David Wall for helping with the initial growth chamber set-up.
Supplementary Material
The Supplementary Material for this article can be found online at: https://www.frontiersin.org/articles/10.3389/fmicb.2020.00682/full#supplementary-material
References
Ai, C., Liang, G., Sun, J., Wang, X., He, P., and Zhou, W. (2013). Different roles of rhizosphere effect and long-term fertilization in the activity and community structure of ammonia oxidizers in a calcareous fluvo-aquic soil. Soil Biol. Biochem. 57, 30–42. doi: 10.1016/j.soilbio.2012.08.003
Alef, K., and Nannipieri, P. (eds). (1995). “7 – Enzyme activities,” in Methods in Applied Soil Microbiology and Biochemistry. London: Academic Press, 311–373. doi: 10.1016/B978-012513840-6/50022-7
Alt, F., Oelmann, Y., Herold, N., Schrumpf, M., and Wilcke, W. (2011). Phosphorus partitioning in grassland and forest soils of Germany as related to land-use type, management intensity, and land use–related pH. J. Plant Nutr. Soil Sci. 174, 195–209. doi: 10.1002/jpln.201000142
Anderson, M. (2001). A new method for non-parametric multivariate analysis of variance. Austral. Ecol. 26, 32–46. doi: 10.1111/j.1442-9993.2001.01070.pp.x
Angel, R., Claus, P., and Conrad, R. (2011). Methanogenic archaea are globally ubiquitous in aerated soils and become active under wet anoxic conditions. ISME J. 6:847. doi: 10.1038/ismej.2011.141
Araki, H., Hossain, M. A., and Takahashi, T. (2012). Waterlogging and hypoxia have permanent effects on wheat root growth and respiration. J. Agron. Crop Sci. 198, 264–275. doi: 10.1111/j.1439-037X.2012.00510.x
Arneth, A., Barbosa, H., Benton, T., Calvin, K., Calvo, E., Connors, S., et al. (2019). “Climate change and land: summary for policymakers,” in An IPCC Special Report on Climate Change, Desertification, Land Degradation, Sustainable Land Management, Food Security, and Greenhouse Gas Fluxes in Terrestrial Ecosystems, 43. Geneva: IPCC. doi: 10.4337/9781784710644
Austin, A. T., Yahdjian, L., Stark, J. M., Belnap, J., Porporato, A., Norton, U., et al. (2004). Water pulses and biogeochemical cycles in arid and semiarid ecosystems. Oecologia 141, 221–235. doi: 10.1007/s00442-004-1519-1
Bakker, M., Chaparro, J., Manter, D., and Vivanco, J. (2015). Impacts of bulk soil microbial community structure on rhizosphere microbiomes of Zea mays. Plant Soil 392, 115–126. doi: 10.1007/s11104-015-2446-0
Barratt, S. R., Ennos, A. R., Greenhalgh, M., Robson, G. D., and Handley, P. S. (2003). Fungi are the predominant micro-organisms responsible for degradation of soil-buried polyester polyurethane over a range of soil water holding capacities. J. Appl. Microbiol. 95, 78–85. doi: 10.1046/j.1365-2672.2003.01961.x
Beauregard, M. S., Hamel, C., Atul-Nayyar, and St-Arnaud, M. (2010). Long-Term phosphorus fertilization impacts soil fungal and bacterial diversity but not AM fungal community in Alfalfa. Microb. Ecol. 59, 379–389. doi: 10.1007/s00248-009-9583-z
Belford, R. K., Cannell, R. Q., Thomson, R. J., and Dennis, C. W. (1980). Effects of waterlogging at different stages of development on the growth and yield of peas (Pisum sativum L.). J. Sci. Food Agric. 31, 857–869. doi: 10.1002/jsfa.2740310902
Borowik, A., and Wyszkowska, J. (2016). Soil moisture as a factor affecting the microbiological and biochemical activity of soil. Plant Soil Environ. 62, 250–255. doi: 10.17221/158/2016-PSE
Breidenbach, B., and Conrad, R. (2014). Seasonal dynamics of bacterial and archaeal methanogenic communities in flooded rice fields and effect of drainage. Front. Microbiol. 5:752. doi: 10.3389/fmicb.2014.00752
Brod, E., Øgaard, A., Hansen, E., Wragg, D., Haraldsen, T., and Krogstad, T. (2015). Waste products as alternative phosphorus fertilisers part I: inorganic P species affect fertilisation effects depending on soil pH. Nutr. Cycling Agroecosyst. 103, 167–185. doi: 10.1007/s10705-015-9734-1
Cavicchioli, R., Ripple, W. J., Timmis, K. N., Azam, F., Bakken, L. R., Baylis, M., et al. (2019). Scientists’ warning to humanity: microorganisms and climate change. Nat. Rev. Microbiol. 17, 569–586. doi: 10.1038/s41579-019-0222-5
Ceci, A., Pinzari, F., Russo, F., Maggi, O., and Persiani, A. M. (2018). Saprotrophic soil fungi to improve phosphorus solubilisation and release: In vitro abilities of several species. AMBIO 47, 30–40. doi: 10.1007/s13280-017-0972-0
Chen, M.-M., Zhu, Y.-G., Su, Y.-H., Chen, B.-D., Fu, B.-J., and Marschner, P. (2007). Effects of soil moisture and plant interactions on the soil microbial community structure. Eur. J. Soil Biol. 43, 31–38. doi: 10.1016/j.ejsobi.2006.05.001
Chen, X., Daniell, T. J., Neilson, R., O’Flaherty, V., and Griffiths, B. S. (2014). Microbial and microfaunal communities in phosphorus limited, grazed grassland change composition but maintain homeostatic nutrient stoichiometry. Soil Biol. Biochem. 75, 94–101. doi: 10.1016/j.soilbio.2014.03.024
Chu, H., Lin, X., Fujii, T., Morimoto, S., Yagi, K., Hu, J., et al. (2007). Soil microbial biomass, dehydrogenase activity, bacterial community structure in response to long-term fertilizer management. Soil Biol. Biochem. 39, 2971–2976. doi: 10.1016/j.soilbio.2007.05.031
Clarke, K. R., Somerfield, P. J., and Chapman, M. G. (2006). On resemblance measures for ecological studies, including taxonomic dissimilarities and a zero-adjusted Bray-Curtis coefficient for denuded assemblages. J. Exp. Mar. Biol. Ecol. 330, 55–80. doi: 10.1016/j.jembe.2005.12.017
Clarke, K. R., and Warwick, R. M. (2001). Changein Marine Communities: An Approach to Statistical Analysis and Interpretation, 2nd Edn. Plymouth: PRIMER-E Ltd.
Comeau, L.-P., Lai, D. Y. F., Cui, J. J., and Hartill, J. (2018). Soil heterotrophic respiration assessment using minimally disturbed soil microcosm cores. MethodsX 5, 834–840. doi: 10.1016/j.mex.2018.07.014
da Rocha, U., Plugge, C. M., George, I., van Elsas, J. D., and van Overbeek, L. S. (2013). The rhizosphere selects for particular groups of acidobacteria and verrucomicrobia. PLoS One 8:e82443. doi: 10.1371/journal.pone.0082443
de Vries, F. T., Griffiths, R. I., Bailey, M., Craig, H., Girlanda, M., Gweon, H. S., et al. (2018). Soil bacterial networks are less stable under drought than fungal networks. Nat. Commun. 9:3033. doi: 10.1038/s41467-018-05516-7
de Vries, F. T., and Wallenstein, M. D. (2017). Below-ground connections underlying above-ground food production: a framework for optimising ecological connections in the rhizosphere. J. Ecol. 105, 913–920. doi: 10.1111/1365-2745.12783
Dedysh, S. N., Kulichevskaya, I. S., Huber, K. J., and Overmann, J. (2017). Defining the taxonomic status of described subdivision 3 Acidobacteria: proposal of Bryobacteraceae fam. nov. Int. J. Syst. Evol. Microbiol. 67, 498–501. doi: 10.1099/ijsem.0.001687
Di, H. J., and Cameron, K. C. (2002). Nitrate leaching in temperate agroecosystems: sources, factors and mitigating strategies. Nutr. Cycling Agroecosyst. 64, 237–256. doi: 10.1023/A:1021471531188
Dijkstra, F. A., and Cheng, W. (2007). Moisture modulates rhizosphere effects on C decomposition in two different soil types. Soil Biol. Biochem. 39, 2264–2274. doi: 10.1016/j.soilbio.2007.03.026
Dubey, A., Malla, M. A., Khan, F., Chowdhary, K., Yadav, S., Kumar, A., et al. (2019). Soil microbiome: a key player for conservation of soil health under changing climate. Biodivers. Conserv. 28, 2405–2429. doi: 10.1007/s10531-019-01760-5
Elser, J. J., Bracken, M. E. S., Cleland, E. E., Gruner, D. S., Harpole, W. S., Hillebrand, H., et al. (2007). Global analysis of nitrogen and phosphorus limitation of primary producers in freshwater, marine and terrestrial ecosystems. Ecol. Lett. 10, 1135–1142. doi: 10.1111/j.1461-0248.2007.01113.x
EU Commission (2011). COMMUNICATION FROM THE COMMISSION TO THE EUROPEAN PARLIAMENT, THE COUNCIL, THE EUROPEAN ECONOMIC AND SOCIAL COMMITTEE AND THE COMMITTEE OF THE REGIONS. Horizon 2020 - The Framework Programme for Research and Innovation. Brussels: EU Commission.
Eurostat (2019). The EU in the World – Agriculture, Forestry and Fisheries. Available online at: https://ec.europa.eu/eurostat/statistics-explained/index.php?title=The_EU_in_the_world_-_agriculture,_forestry_and_fisheries#Agricultural_area (accessed November 15, 2019).
Falkowski, P. G., Fenchel, T., and Delong, E. F. (2008). The microbial engines that drive Earth’s biogeochemical cycles. Science 320, 1034–1039. doi: 10.1126/science.1153213
FAO (2015). Soil is a Non-renewable Resource. Rome: Food and Agriculture Organization of the United Nations, 2–3.
Ferré, C., Zechmeister-Boltenstern, S., Comolli, R., Andersson, M., and Seufert, G. (2012). Soil microbial community structure in a rice paddy field and its relationships to CH4 and N2O fluxes. Nutr. Cycling Agroecosyst. 93, 35–50. doi: 10.1007/s10705-012-9497-x
Fierer, N., Schimel, J. P., and Holden, P. A. (2003). Influence of drying-rewetting frequency on soil bacterial community structure. Microb. Ecol. 45, 63–71. doi: 10.1007/s00248-002-1007-2
Fraser, T., Lynch, D. H., Entz, M. H., and Dunfield, K. E. (2014). Linking alkaline phosphatase activity with bacterial phoD gene abundance in soil from a long-term management trial. Geoderma 257. doi: 10.1016/j.geoderma.2014.10.016
Gargallo-Garriga, A., Preece, C., Sardans, J., Oravec, M., Urban, O., and Peñuelas, J. (2018). Root exudate metabolomes change under drought and show limited capacity for recovery. Sci. Rep. 8:12696. doi: 10.1038/s41598-018-30150-0
Gebremichael, A. W., Osborne, B., and Orr, P. (2017). Flooding-related increases in CO2 and N2O emissions from a temperate coastal grassland ecosystem. Biogeosciences 14, 2611–2626. doi: 10.5194/bg-14-2611-2017
Glick, B. R. (2018). Soil microbes and sustainable agriculture. Pedosphere 28, 167–169. doi: 10.1016/s1002-0160(18)60020-7
Gosling, P., Mead, A., Proctor, M., Hammond, J. P., and Bending, G. D. (2013). Contrasting arbuscular mycorrhizal communities colonizing different host plants show a similar response to a soil phosphorus concentration gradient. New Phytol. 198, 546–556. doi: 10.1111/nph.12169
Griffiths, B. S., Spilles, A., and Bonkowski, M. (2012). C:N:P stoichiometry and nutrient limitation of the soil microbial biomass in a grazed grassland site under experimental P limitation or excess. Ecol. Process. 1, 1–11. doi: 10.1186/2192-1709-1-6
Griffiths, I., Whiteley, A. S., O’Donnell, A. G., and Bailey, M. J. (2000). Rapid method for coextraction of DNA and RNA from natural environments for analysis of ribosomal DNA- and rRNA-based microbial community composition. Appl. Environ. Microbiol. 66, 5488–5491. doi: 10.1128/aem.66.12.5488-5491.2000
Griffiths, R. I, Thomson, B. C., James, P., Bell, T., Bailey, M., and Whiteley, A. S. (2011). The bacterial biogeography of British soils. Environ. Microbiol. 13, 1642–1654. doi: 10.1111/j.1462-2920.2011.02480.x
Hamonts, K., Clough, T. J., Stewart, A., Clinton, P. W., Richardson, A. E., Wakelin, S. A., et al. (2013). Effect of nitrogen and waterlogging on denitrifier gene abundance, community structure and activity in the rhizosphere of wheat. FEMS Microbiol. Ecol. 83, 568–584. doi: 10.1111/1574-6941.12015
Hartman, K., and Tringe, S. G. (2019). Interactions between plants and soil shaping the root microbiome under abiotic stress. Biochem. J. 476, 2705–2724. doi: 10.1042/BCJ20180615
Haworth, M., Elliott-Kingston, C., and McElwain, J. C. (2011). The stomatal CO2 proxy does not saturate at high atmospheric CO2 concentrations: evidence from stomatal index responses of Araucariaceae conifers. Oecologia 167, 11–19. doi: 10.1007/s00442-011-1969-1
Haworth, M., Elliott-Kingston, C., and McElwain, J. C. (2013). Co-ordination of physiological and morphological responses of stomata to elevated [CO2] in vascular plants. Oecologia 171, 71–82. doi: 10.1007/s00442-012-2406-9
Henry, H. A. L. (2012). Soil extracellular enzyme dynamics in a changing climate. Soil Biol. Biochem. 47, 53–59. doi: 10.1016/j.soilbio.2011.12.026
Hinsinger, P., Bengough, A. G., Vetterlein, D., and Young, I. M. (2009). Rhizosphere: biophysics, biogeochemistry and ecological relevance. Plant Soil 321, 117–152. doi: 10.1007/s11104-008-9885-9
Hütsch, B. W., Jahn, D., and Schubert, S. (2019). Grain yield of wheat (Triticum aestivum L.) under long-term heat stress is sink-limited with stronger inhibition of kernel setting than grain filling. J. Agron. Crop Sci. 205, 22–32. doi: 10.1111/jac.12298
Huxman, T. E., Cable, J. M., Ignace, D. D., Eilts, J. A., English, N. B., Weltzin, J., et al. (2004). Response of net ecosystem gas exchange to a simulated precipitation pulse in a semi-arid grassland: the role of native versus non-native grasses and soil texture. Oecologia 141, 295–305. doi: 10.1007/s00442-003-1389-y
IPCC (2014). “Climate change 2014: impacts, adaptation, and vulnerability. part B: regional aspects,” in Contribution of Working Group II to the Fifth Assessment Report of the Intergovernmental Panel on Climate Change, Vol. 88, eds V. R. Barros, C. B. Field, D. J. Dokken, and M. D. Mastrandre (Geneva: IPCC). (accessed January 2, 2016).
IPCC (2018). Summary for Policymakers. In: Global warming of 1.5°C. An IPCC Special Report on the Impacts of Global Warming of 1.5°C above Pre-industrial Levels and Related Global Greenhouse Gas Emission Pathways, in the Context of Strengthening the Global Response to the Threat of Climate Change, Sustainable Development, and Efforts to Eradicate Poverty. Available online at: https://www.ipcc.ch/site/assets/uploads/sites/2/2019/05/SR15_SPM_version_report_LR.pdf (accessed November 15, 2019).
Isselstein, J., Jeangros, B., and Pavlu, V. (2005). Agronomic aspects of biodiversity targeted management of temperate grasslands in Europe – A review. Agron. Res. 3, 139–151.
Janssen, P. H. (2006). Identifying the dominant soil bacterial taxa in libraries of 16S rRNA and 16S rRNA genes. Appl. Environ. Microbiol. 72, 1719–1728. doi: 10.1128/AEM.72.3.1719-1728.2006
Jansson, J. K., and Hofmockel, K. S. (2019). Soil microbiomes and climate change. Nat. Rev. Microbiol. 18, 35–46. doi: 10.1038/s41579-019-0265-7
Jones, R. T., Robeson, M. S., Lauber, C. L., Hamady, M., Knight, R., and Fierer, N. (2009). A comprehensive survey of soil acidobacterial diversity using pyrosequencing and clone library analyses. ISME J. 3, 442–453. doi: 10.1038/ismej.2008.127
Kimura, M., and Asakawa, S. (2006). Comparison of community structures of microbiota at main habitats in rice field ecosystems based on phospholipid fatty acid analysis. Biol. Fertil. Soils 43, 20–29. doi: 10.1007/s00374-005-0057-2
Lakshmanan, V., Selvaraj, G., and Bais, H. P. (2014). Functional soil microbiome: belowground solutions to an aboveground problem. Plant Physiol. 166, 689–700. doi: 10.1104/pp.114.245811
Lambers, H., Mougel, C., Jaillard, B., and Hinsinger, P. (2009). Plant-microbe-soil interactions in the rhizosphere: an evolutionary perspective. Plant Soil 321, 83–115. doi: 10.1007/s11104-009-0042-x
Lazcano, C., Barrios-Masias, F. H., and Jackson, L. E. (2014). Arbuscular mycorrhizal effects on plant water relations and soil greenhouse gas emissions under changing moisture regimes. Soil Biol. Biochem. 74, 184–192. doi: 10.1016/j.soilbio.2014.03.010
Lemanceau, P., Maron, P.-A., Mazurier, S., Mougel, C., Pivato, B., Plassart, P., et al. (2015). Understanding and managing soil biodiversity: a major challenge in agroecology. Agron. Sustain. Dev. 35, 67–81. doi: 10.1007/s13593-014-0247-0
Liesack, W., Schnell, S., and Revsbech, N. P. (2000). Microbiology of flooded rice paddies. FEMS Microbiol. Rev. 24, 625–645. doi: 10.1111/j.1574-6976.2000.tb00563.x
Liu, R.-X., He, J.-Z., and Zhang, L.-M. (2014). [Response of nitrification/denitrification and their associated microbes to soil moisture change in paddy soil]. Huan Jing ke Xue 35, 4275–4283.
Liu, W., Zhang, Z. H. E., and Wan, S. (2009). Predominant role of water in regulating soil and microbial respiration and their responses to climate change in a semiarid grassland. Global Change Biol. 15, 184–195. doi: 10.1111/j.1365-2486.2008.01728.x
Lüdemann, H., Arth, I., Liesack, W., Lu, H., Liesack, W., and Lüdemann, H. (2000). Spatial changes in the bacterial community structure along a vertical oxygen gradient in flooded paddy soil cores. Appl. Environ. Microbiol. 66, 754–762. doi: 10.1128/AEM.66.2.754-762.2000
Luo, P., Han, X., Wang, Y., Han, M., Shi, H., Liu, N., et al. (2015). Influence of long-term fertilization on soil microbial biomass, dehydrogenase activity, and bacterial and fungal community structure in a brown soil of northeast China. Ann. Microbiol. 65, 533–542. doi: 10.1007/s13213-014-0889-9
Luster, J., Göttlein, A., Nowack, B., and Sarret, G. (2009). Sampling, defining, characterising and modeling the rhizosphere—the soil science tool box. Plant Soil 321, 457–482. doi: 10.1007/s11104-008-9781-3
Mander, C., Wakelin, S., Young, S., Condron, L., and O’Callaghan, M. (2012). Incidence and diversity of phosphate-solubilising bacteria are linked to phosphorus status in grassland soils. Soil Biol. Biochem. 44, 93–101. doi: 10.1016/j.soilbio.2011.09.009
Mandic-Mulec, I., Stefanic, P., and van Elsas, J. D. (2015). Ecology of Bacillaceae. Microbiol. Spectr 3:TBS-0017-2013.
Massey, P. A., Creamer, R. E., and Ritz, P. K. (2012). INTERACTIONS BETWEEN PHOSPHORUS FERTILISATION AND SOIL BIOTA IN MANAGED GRASSLAND SYSTEMS. Available online at: http://dspace.lib.cranfield.ac.uk/handle/1826/7844 (accessed November 15, 2011).
McCormack, S. (2002). Analysis of Agricultural Materials: Methods used at Johnstown Castle Research Centre. Wexford: Johnstown Castle Research Centre, 2.
McDowell, R. W., Monaghan, R. M., and Carey, P. L. (2003). Potential phosphorus losses in overland flow from pastoral soils receiving long-term applications of either superphosphate or reactive phosphate rock. N. Z. J. Agric. Res. 46, 329–337. doi: 10.1080/00288233.2003.9513561
Met Éireann (2011). Monthly data – Johnstown Castle. Available online at: https://www.met.ie/climate/available-data/monthly-data (accessed November 7, 2011).
Mohan, D. (2014). Analysis of long-term wheat varieties for climate resilience and productivity oscillation in different environments of India. Indian J. Genet. Plant Breed. 74:430. doi: 10.5958/0975-6906.2014.00866.9
Murphy, J., and Riley, J. P. (1962). A modified single solution method for the determination of phosphate in natural waters. Anal. Chim. Acta 27, 31–36. doi: 10.1016/S0003-2670(00)88444-5
Naether, A., Foesel, B. U., Naegele, V., Wüst, P. K., Weinert, J., Bonkowski, M., et al. (2012). Environmental factors affect acidobacterial communities below the subgroup level in grassland and forest soils. Appl. Environ. Microbiol. 78, 7398–7406. doi: 10.1128/AEM.01325-12
Nannipieri, P., Giagnoni, L., Landi, L., Renella, G., Bünemann, E. K., Oberson, A., et al. (2011). Phosphorus in Action. Biological Processes in Soil Phosphorus Cycling, eds E. K. Bünemann, A. Oberson, and E. Frossard (Berlin: Springer Science & Business Media).
Naylor, D., and Coleman-Derr, D. (2018). Drought stress and root-associated bacterial communities. Front. Plant Sci. 8:2223. doi: 10.3389/fpls.2017.02223
Nessner Kavamura, V., Taketani, R. G., Lançoni, M. D., Andreote, F. D., Mendes, R., and Soares, et al. (2013). Water regime influences bulk soil and rhizosphere of Cereus jamacaru bacterial communities in the Brazilian caatinga biome. PLoS One 8:e73606. doi: 10.1371/journal.pone.0073606
Neumann, G., George, T. S., and Plassard, C. (2009). Strategies and methods for studying the rhizosphere—the plant science toolbox. Plant Soil 321, 431–456. doi: 10.1007/s11104-009-9953-9
Nie, S.-A., Xu, H.-J., Li, S., Li, H., and Su, J.-Q. (2014). Relationships between abundance of microbial functional genes and the status and fluxes of carbon and nitrogen in rice rhizosphere and bulk soils. Pedosphere 24, 645–651. doi: 10.1016/S1002-0160(14)60050-3
Noll, M., Matthies, D., Frenzel, P., Derakshani, M., and Liesack, W. (2005). Succession of bacterial community structure and diversity in a paddy soil oxygen gradient. Environ. Microbiol. 7, 382–395. doi: 10.1111/j.1462-2920.2005.00700.x
O’Brien, F. J. M., Dumont, M. G., Webb, J. S., and Poppy, G. M. (2018). Rhizosphere bacterial communities differ according to fertilizer regimes and cabbage (Brassica oleracea var. capitata L.) harvest time, but not aphid herbivory. Front. Microbiol. 9:1620. doi: 10.3389/fmicb.2018.01620
Olander, L. P., and Vitousek, P. M. (2000). Regulation of soil phosphatase and chitinase activity by N and P availability. Biogeochemistry 49, 175–190.
Ortiz, R., Sayre, K. D., Govaerts, B., Gupta, R., Subbarao, G. V., Ban, T., et al. (2008). Climate change: can wheat beat the heat? Agric. Ecosyst. Environ. 126, 46–58. doi: 10.1016/j.agee.2008.01.019
Owen, D., Williams, A. P., Griffith, G. W., and Withers, P. J. A. (2015). Use of commercial bio-inoculants to increase agricultural production through improved phosphrous acquisition. Appl. Soil Ecol. 86, 41–54. doi: 10.1016/j.apsoil.2014.09.012
Papendick, R. I., and Campbell, G. S. (1981). Theory and measurement of water potential. Water Potential Relat. Soil Microbiol. 9. doi: 10.2136/sssaspecpub9.c1
Philippot, L., Raaijmakers, J. M., Lemanceau, P., and van der Putten, W. H. (2013). Going back to the roots: the microbial ecology of the rhizosphere. Nat. Rev. Microbiol. 11, 789–799. doi: 10.1038/nrmicro3109
Praeg, N., Pauli, H., and Illmer, P. (2019). Microbial diversity in bulk and rhizosphere soil of Ranunculus glacialis along a high-alpine altitudinal gradient. Front. Microbiol. 10:1429. doi: 10.3389/fmicb.2019.01429
Qi, Y., Dong, Y., Peng, Q., Xiao, S., He, Y., Liu, X., et al. (2012). Effects of a conversion from grassland to cropland on the different soil organic carbon fractions in Inner Mongolia, China. J. Geogr. Sci. 22, 315–328. doi: 10.1007/s11442-012-0929-y
Quaiser, A., Ochsenreiter, T., Lanz, C., Schuster, S. C., Treusch, A. H., Eck, J., et al. (2003). Acidobacteria form a coherent but highly diverse group within the bacterial domain: evidence from environmental genomics. Mol. Microbiol. 50, 563–575. doi: 10.1046/j.1365-2958.2003.03707.x
Ragot, S. A., Huguenin-Elie, O., Kertesz, M. A., Frossard, E., and Bünemann, E. K. (2016). Total and active microbial communities and phoD as affected by phosphate depletion and pH in soil. Plant Soil 408, 15–30. doi: 10.1007/s11104-016-2902-5
Randall, K., Brennan, F., Clipson, N., Creamer, R., Griffiths, B., Storey, S., et al. (2019). Soil bacterial community structure and functional responses across a long-term mineral phosphorus (Pi) fertilisation gradient differ in grazed and cut grasslands. Appl. Soil Ecol. 138, 134–143. doi: 10.1016/j.apsoil.2019.02.002
Randle-Boggis, R. J., Ashton, P. D., and Helgason, T. (2018). Increasing flooding frequency alters soil microbial communities and functions under laboratory conditions. MicrobiologyOpen 7:e00548. doi: 10.1002/mbo3.548
Reim, A., Hernández, M., Klose, M., Chidthaisong, A., Yuttitham, M., and Conrad, R. (2017). Response of methanogenic microbial communities to desiccation stress in flooded and rain-fed paddy soil from Thailand. Front. Microbiol. 8:785. doi: 10.3389/fmicb.2017.00785
Reim, A., Luke, C., Krause, S., Pratscher, J., and Frenzel, P. (2012). One millimetre makes the difference: high-resolution analysis of methane-oxidizing bacteria and their specific activity at the oxic-anoxic interface in a flooded paddy soil. ISME J. 6, 2128–2139. doi: 10.1038/ismej.2012.57
Santos, F., and Diola, V. (2015). “Chapter 2 - Physiology,” in Sugarcane, eds F. Santos, A. Borém, and C. Caldas (San Diego, CA: Academic Press), 13–33. doi: 10.1016/B978-0-12-802239-9.00002-5
Schloss, P. D., Westcott, S. L., Ryabin, T., Hall, J. R., Hartmann, M., Hollister, E. B., et al. (2009). Introducing mothur: open-source, platform-independent, community-supported software for describing and comparing microbial communities. Appl. Environ. Microbiol. 75, 7537–7541. doi: 10.1128/AEM.01541-09
Sharma, D. B., and Swarup, A. (1988). Effects of short-term flooding on growth, yield and mineral composition of wheat on sodic soil under field conditions. Plant Soil 107, 137–143. doi: 10.1007/BF02371555
Shi, Y., Lalande, R., Hamel, C., Ziadi, N., Gagnon, B., and Hu, Z. (2013). Seasonal variation of microbial biomass, activity, and community structure in soil under different tillage and phosphorus management practices. Biol. Fertil. Soils 49, 803–818. doi: 10.1007/s00374-013-0773-y
Shivaji, S., Srinivas, T. N. R., and Reddy, G. S. N. (2014). “The family planococcaceae,” in The Prokaryotes: Firmicutes and Tenericutes, eds E. Rosenberg, E. F. DeLong, S. Lory, E. Stackebrandt, and F. Thompson (Berlin: Springer), 303–351. doi: 10.1007/978-3-642-30120-9_351
Skwarylo-Bednarz, B., and Krzepilko, A. (2013). Effect of varied npk fertilization on catalase activity of amaranth (Amaranthus cruentus L.). Ecol. Chem. Eng. S 20, 321–329. doi: 10.2478/eces-2013-0023
Southworth, J., Pfeifer, R. A., Habeck, M., Randolph, J. C., and Doering, O. C. (2002). Sensitivity of winter wheat yields in the Midwestern United States to future changes in climate, climate variability, and CO2 fertilization. Clim. Res. 22, 73–86. doi: 10.3354/cr022073
Spanner, J. (2015). Healthy Soils are the Basis for Healthy Food Production. Available online at: http://www.fao.org/3/a-i4405e.pdf (accessed April 3, 2015).
St Clair, S. B., and Lynch, J. P. (2010). The opening of Pandora’s Box: climate change impacts on soil fertility and crop nutrition in developing countries. Plant Soil 335, 101–115. doi: 10.1007/s11104-010-0328-z
Stamenković, S., Beškoski, V., Karabegovic, I., Lazic, M., and Nikolic, N. (2018). Microbial fertilizers: a comprehensive review of current findings and future perspectives. Spanish J. Agric. Res. 16:e09. doi: 10.5424/sjar/2018161-12117
Stone, D., Costa, D., Daniell, T. J., Mitchell, S. M., Topp, C. F. E., and Griffiths, B. S. (2016). Using nematode communities to test a European scale soil biological monitoring programme for policy development. Appl. Soil Ecol. 97, 78–85. doi: 10.1016/j.apsoil.2015.08.017
Storey, S., Ashaari, M. M., McCabe, G., Harty, M., Dempsey, R., Doyle, O., et al. (2014). Microbial community structure during fluoranthene degradation in the presence of plants. J. Appl. Microbiol. 117, 74–84. doi: 10.1111/jam.12518
Suksong, W., Kongjan, P., Prasertsan, P., and O-Thong, S. (2019). Thermotolerant cellulolytic Clostridiaceae and Lachnospiraceae rich consortium enhanced biogas production from oil palm empty fruit bunches by solid-state anaerobic digestion. Bioresour. Technol. 291, 121851. doi: 10.1016/j.biortech.2019.121851
Tabatabai, M. A., and Bremner, J. M. (1969). Use of p-nitrophenyl phosphate for assay of soil phosphatase activity. Soil Biol. Biochem. 1, 301–307. doi: 10.1016/0038-0717(69)90012-1
Tan, H., Barret, M., Mooij, M., Rice, O., Morrissey, J., Dobson, A., et al. (2013). Long-term phosphorus fertilisation increased the diversity of the total bacterial community and the phoD phosphorus mineraliser group in pasture soils. Biol. Fertil. Soils 49, 661–672. doi: 10.1007/s00374-012-0755-5
Tao, F., and Zhang, Z. (2013). Climate change, wheat productivity and water use in the North China plain: a new super-ensemble-based probabilistic projection. Agric. Forest Meteorol. 170, 146–165. doi: 10.1016/j.agrformet.2011.10.003
Tete, E., Viaud, V., and Walter, C. (2015). Organic carbon and nitrogen mineralization in a poorly-drained mineral soil under transient waterlogged conditions: an incubation experiment. Eur. J. Soil Sci. 66, 427–437. doi: 10.1111/ejss.12234
Timmis, K., Cavicchioli, R., Garcia, J. L., Nogales, B., Chavarría, M., Stein, L., et al. (2019). The urgent need for microbiology literacy in society. Environ. Microbiol. 21, 1513–1528. doi: 10.1111/1462-2920.14611
Tunney, H., Kirwan, L., Fu, W., Culleton, N., and Black, A. D. (2010). Long-term phosphorus grassland experiment for beef production – impacts on soil phosphorus levels and liveweight gains. Soil Use Manag. 26, 237–244. doi: 10.1111/j.1475-2743.2010.00292.x
Turner, B., and Joseph Wright, S. (2014). The response of microbial biomass and hydrolytic enzymes to a decade of nitrogen, phosphorus, and potassium addition in a lowland tropical rain forest. Biogeochemistry 117, 115–130. doi: 10.1007/s10533-013-9848-y
Uhlírová, E., Elhottová, D., Tríska, J., and Santrùcková, H. (2005). Physiology and microbial community structure in soil at extreme water content. Folia Microbiol. 50, 161–166. doi: 10.1007/bf02931466
Unger, I. M., Kennedy, A. C., and Muzika, R.-M. (2009). Flooding effects on soil microbial communities. Appl. Soil Ecol. 42, 1–8. doi: 10.1016/j.apsoil.2009.01.007
Váry, Z., Mullins, E., McElwain, J. C., and Doohan, F. M. (2015). The severity of wheat diseases increases when plants and pathogens are acclimatized to elevated carbon dioxide. Global Change Biol. 21, 2661–2669. doi: 10.1111/gcb.12899
Wang, Q., Zeng, Z., and Zhong, M. (2016). Soil moisture alters the response of soil organic carbon mineralization to litter addition. Ecosystems 19, 450–460. doi: 10.1007/s10021-015-9941-2
Wakelin, S., Mander, C., Gerard, E., Jansa, J., Erb, A., Young, S., et al. (2012). Response of soil microbial communities to contrasted histories of phosphorus fertilisation in pastures. Appl. Soil Ecol. 61, 40–48. doi: 10.1016/j.apsoil.2012.06.002
Williams, M. A., and Rice, C. W. (2007). Seven years of enhanced water availability influences the physiological, structural, and functional attributes of a soil microbial community. Appl. Soil Ecol. 35, 535–545. doi: 10.1016/j.apsoil.2006.09.014
Wu, D.-X., Wang, G.-X., Bai, Y.-F., and Liao, J.-X. (2004). Effects of elevated CO2 concentration on growth, water use, yield and grain quality of wheat under two soil water levels. Agric. Ecosyst. Environ. 104, 493–507. doi: 10.1016/j.agee.2004.01.018
Yaduvanshi, N. P. S., Setter, T. L., Sharma, S. K., Singh, K. N., and Kulshreshtha, N. (2012). Influence of waterlogging on yield of wheat (Triticum aestivum), redox potentials, and concentrations of microelements in different soils in India and Australia. Soil Res. 50:489. doi: 10.1071/SR11266
Zadoks, J. C., Chang, T. T., and Konzak, C. F. (1974). A decimal code for the growth stages of cereals. Weed Res. 14, 415–421. doi: 10.1111/j.1365-3180.1974.tb01084.x
Zeng, X. (2017). Differential response of microbial respiration to supplied nitrogen forms in 3 contrasting alpine meadow soils on the tibetan plateau. Bragantia 76, 416–421. doi: 10.1590/1678-4499.139
Keywords: climate change, soil moisture, phosphorus, bacteria, rhizosphere
Citation: Randall KC, Brennan F, Clipson N, Creamer RE, Griffiths BS, Storey S and Doyle E (2020) An Assessment of Climate Induced Increase in Soil Water Availability for Soil Bacterial Communities Exposed to Long-Term Differential Phosphorus Fertilization. Front. Microbiol. 11:682. doi: 10.3389/fmicb.2020.00682
Received: 26 November 2019; Accepted: 24 March 2020;
Published: 15 May 2020.
Edited by:
Manuel Delgado Baquerizo, University of Alicante, SpainReviewed by:
Jim He, University of Chinese Academy of Sciences, ChinaDima Chen, China Three Gorges University, China
Copyright © 2020 Randall, Brennan, Clipson, Creamer, Griffiths, Storey and Doyle. This is an open-access article distributed under the terms of the Creative Commons Attribution License (CC BY). The use, distribution or reproduction in other forums is permitted, provided the original author(s) and the copyright owner(s) are credited and that the original publication in this journal is cited, in accordance with accepted academic practice. No use, distribution or reproduction is permitted which does not comply with these terms.
*Correspondence: Evelyn Doyle, ZXZlbHluLmRveWxlQHVjZC5pZQ==