- 1EcoSciences Precinct, Department of Agriculture and Fisheries, Queensland Government, Brisbane, QLD, Australia
- 2Division of Microbiology, Brewing and Biotechnology, School of Biosciences, University of Nottingham, Nottingham, United Kingdom
This study describes the development and use of bacteriophage cocktails to control Campylobacter in broiler chickens, in a commercial setting, in Queensland Australia, following the birds from farm to the processing plant. The components of the bacteriophage cocktails were selected to be effective against the maximum number of Campylobacter jejuni and Campylobacter coli isolates encountered on SE Queensland farms. Farms were identified that had suitable Campylobacter target populations and phage were undetectable 1 week prior to the intended treatment. Cocktails of phages were administered at 47 days of age. Groups of study birds were slaughtered the following day, on-farm, at the end of flock transport to the plant, and at processing (approximately 28 h post-treatment). On Farm A, the phage treatment significantly reduced Campylobacter levels in the ceca at the farm in the range of 1–3 log10 CFU/g (p = 0.007), compared to mock treated controls. However, individual birds sampled on farm (1/10) or following transport (2/10) exhibited high cecal Campylobacter counts with low phage titers, suggesting that treatment periods > 24 h may be required to ensure phage replication for effective biocontrol in vivo. At the time of the trial the control birds in Farm B were phage positive despite having been negative one week earlier. There was no significant difference in the cecal Campylobacter counts between the treatment and control groups following treatment but a fall of 1.7 log10 CFU/g was observed from that determined from birds collected the previous week (p = 0.0004). Campylobacter isolates from both farms retained sensitivity to the treatment phages. These trials demonstrated bacteriophages sourced from Queensland farms have the potential to reduce intestinal Campylobacter levels in market ready broiler chickens.
Introduction
Campylobacter infection is one of the most frequently reported causes of food-borne enteritis in Australia and worldwide (Kaakoush et al., 2015). Australia began a National Notifiable Diseases Surveillance System in 1991 and since then, the number of cases of human infection has steadily risen to 137.5/100,000, in 20181. This incidence rate is higher than many other parts of the world (Kaakoush et al., 2015) and it is estimated that only around 10% of Campylobacter infection cases are recorded (Hall et al., 2008). Thus, Campylobacter has a significant impact on the health and economic prosperity of the Australia population.
Consumption of poultry meat has been identified as an important risk factor for human infection from source attribution studies (Mughini Gras et al., 2012; Ravel et al., 2017). Campylobacter spp., particularly Campylobacter jejuni and to a lesser extent Campylobacter coli are ubiquitous in the intestinal contents of broiler chickens (European Food Safety Authority [EFSA] and European Centre for Disease Prevention and Control [ECDC], 2018). During slaughter and processing, poultry carcasses frequently become contaminated with Campylobacter from the intestinal contents, providing a reservoir for human infection (European Food Safety Authority [EFSA], 2010). Consequently, managing Campylobacter numbers in poultry hosts, on-farm, is a promising strategy to reduce disease burden. A 3 log10 reduction in Campylobacter numbers in the intestines of infected birds at slaughter, could potentially contribute to a 90% reduction in public health risks (Crotta et al., 2017). European studies indicate that on-farm interventions can be very effective with a 1.0 log10 reduction in fecal count supported by a 1.0 log10 reduction in contamination of the exterior of chickens (during processing) could result in a 90% reduction of human infections (Havelaar et al., 2007). Biocontrol using bacteriophages has the potential to control Campylobacter numbers in highly contaminated flocks (Crotta et al., 2017).
Biocontrol using bacteriophages has been exploited for controlling other food-borne pathogens such as Salmonella, Escherichia coli O157:H7 and Listeria monocytogenes (Goodridge and Bisha, 2011). Bacteriophages have been shown to be naturally present in poultry environments in the United Kingdom and Australia along with their host campylobacters (Connerton et al., 2004; Loc Carrillo et al., 2007; Owens et al., 2013). Poultry farms are a natural source of phages from which to develop appropriate on-farm treatments as their use will not introduce any agent that is not already frequently encountered (Atterbury et al., 2005; El-Shibiny et al., 2005). These considerations are of commercial importance against a background of consumer anxiety regarding the adoption of intervention strategies against campylobacters from poultry (MacRitchie et al., 2014). Experimental studies have demonstrated the potential to use phages to reduce C. jejuni in broiler chicken ceca (Loc Carrillo et al., 2005; El-Shibiny et al., 2009) and the surface of chicken skin (Atterbury et al., 2003; Goode et al., 2003). Bacteriophages can be used to reduce Campylobacter either on-farm or on the processed product (Connerton et al., 2011). A one log10 reduction in the numbers of C. jejuni and C. coli in feces has been reported (Carvalho et al., 2010). The use of phages on-farm is a welfare friendly option for the biological control of Campylobacter that can be adopted from a logistic perspective for use with commercial poultry.
Phage selection, method, and timing of application on commercial farms are important criteria to achieve meaningful reductions in terms of the risk to the consumer. Wagenaar et al. (2005) have demonstrated 1–3 log10 reductions in Campylobacter counts at various points in the rearing cycle. Loc Carrillo et al. (2005) have also demonstrated log reductions (0.5 and 5 log10 CFU/g) over an extended treatment period (5 days) on experimentally infected birds (25 days old). Similarly, Campylobacter reductions in ceca have been demonstrated over a shorter period (i.e., 2 log10 CFU/g reduction over 48 h) in experimentally inoculated broilers (El-Shibiny et al., 2009). Treatment of naturally colonized commercial birds has been demonstrated to produce significant reductions in Campylobacter levels of 3.2 log10 CFU/g of ceca from one of the three commercial broiler houses involved in the trial (Kittler et al., 2013).
The present study was designed to provide a better understanding of the application of phages for typical Australian commercial farm settings. Campylobacter counts were assessed at the end of rearing on the farm, after transport, and on carcasses post processing.
Methodology
Campylobacter Strains
Campylobacter strains used as bacteriophage hosts were as follows: C. jejuni NCTC 12662, C. jejuni NC3142 (Farm C isolate sourced from re-use litter in 2011), and C. coli NC2934 (Farm C isolate sourced from non-reused litter in 2011). These were grown on Blood agar No 2 (Oxoid CM0271) with 5% (v/v) lyzed horse blood added (LHB; Oxoid Australia), for 24 h at 42°C, under microaerobic conditions, produced by using Campygen gas packs (Oxoid, CN0025A; Basingstoke, United Kingdom). Isolates used for resistance testing were sourced from chicken ceca and carcasses pre- and post-initiation of the study.
Bacteriophage Isolation, Characterization, and Selection
Bacteriophages used in this study were isolated between 2012 and 2015, from samples of: cecal contents, litter, carcass rinses, and soil from Queensland broiler chicken farms and pig effluent. For direct isolation from cecal contents, a 10% suspension in SM buffer (100 mM NaCl; 8 mM MgSO4.7H2O; 0.01% gelatin; 50 mM Tris-HCl, pH7.5), incubated over-night at 4°C, centrifuged at 15,000 g for 10 min at 4°C, filtered through 0.2 μm filters (Minisart; Sartorius) then 100 μl added containing 200 μl of Campylobacter host strains (108 CFU/ml) and incubated at 42°C for 30 min to allow phage to bind to host. The proportion of sample to broth was as follows: cecal contents and pig effluent 1:4; litter 1:6; soil 1:3, and carcass rinse 1:2. The suspension was the added to 5 ml of molten 0.6% NZCYM (Difco, Beckton Dickinson, United States) soft agar overlay at 48°C, poured onto 1% NZCYM plates and allowed to set. The plates were then dried with lids partially open and then incubated at 42°C for 24 h, under microaerobic conditions (Connerton et al., 2004). Isolated plaques were collected using a pipette tip and suspended in 100 μl of SM buffer. Each single plaque was propagated three times to ensure that the isolates represented a single clone. In addition, an enrichment technique using modified Preston broth (Bolton et al., 1983) was used. The base Preston broth was prepared from Nutrient Broth Number 2 (NB2; Oxoid CM0067), 5% (v/v) LHB (Oxoid), with Campylobacter growth supplement (Oxoid, SR0232) and Preston Campylobacter selective supplement (Oxoid SR0117). This was modified for phage enrichment by addition of 10 mM MgSO47H2O and 1 mM CaCl2 to stabilize phage capsids (Adams, 1959). Fifty microliters of overnight cultures of C. jejuni 12662, C. jejuni NC3142, and C. coli NC2934 (that had been grown in NB2 with 5% LHB (v/v) and incubated overnight at 42°C) were added to the diluted samples. The enrichment broth containing sample and host bacteria were incubated at 42°C for 24 h under microaerobic conditions. After incubation, centrifugation, and filtration, phages were isolated using the soft agar technique as described above for direct isolation, using all three hosts on separate plates. Approximately 600 bacteriophages were isolated using these methods from using either direct isolation or enrichment. A total of 128 (from the 600) phages were screened (described below) against 486 Campylobacter isolates using multiple combinations to narrow down representation to a 17-member phage panel. A further two phages from Queensland pig farm effluent were also included based on their lytic activity to arrive at a 19-member cocktail candidate panel. The Campylobacter isolates used, represented a mix of C. jejuni and C. coli, sourced from 17 South East Queensland farms across 36 farm samplings that occurred from 2009 to 2013.
Screening of Farm Campylobacter Isolates Against Phage Cocktail Candidates
The lytic activities of 19 phage cocktail candidates were tested against 241 representative Campylobacter isolates sourced between 2012 and 2016 from nine different Queensland farms. The 19 phage cocktail candidates, diluted to contain 106 PFU/ml were dispensed as 10 μl droplets, onto test bacterial lawns prepared as above. Following incubation, strains were scored as sensitive to a bacteriophage, if clear lysis or semi-clear lysis was observed following incubation.
Pre-screening of Birds to Enable Farm Selection
Six farms all having birds of roughly the same age were pre-screened approximately 1 week before the end of the growth cycle. Three chickens were randomly picked from two to four sheds. The criteria for final selection was that the farm should have a high-level resident Campylobacter population that was sensitive to more than one of the 19 selected phage candidates, and that the digesta samples were negative for indigenous phage. The absence of indigenous phage was considered a prerequisite because a previous survey demonstrated that such phage can effect mean Campylobacter populations (Atterbury et al., 2005). Birds were euthanized at the farm and the ceca were removed before transport on ice to the laboratory within 3–4 h. For Campylobacter enumeration, serial dilutions of cecal contents were prepared in MRD then 100 μl of each dilution was spread in triplicate, onto mCCDA (Oxoid CM0739) plates, containing selective supplement (Oxoid SR0155), and then incubated at 37°C for 48 h under microaerobic conditions as above. Ten well-separated Campylobacter colonies per shed were randomly picked across the three samples and re-streaked for purity. Their lysis profiles were then assessed against the 19 phage cocktail candidates (described above). Two farms which fulfilled the three criteria were selected (Farm A and Farm B) and the most appropriate phages selected to form a cocktail to administer to the test birds on these farms. These were PH5, PH8, PH11, and PH13 for Farm A and PH18 and PH19 for Farm B.
Farm Trials
Descriptions of the two test farms and conditions employed during the trial are presented in Table 1. For each of the two selected farms, two groups of randomly picked 30 birds (phage treated and placebo groups) were segregated into two pens, within the chicken barn using wire mesh, but all other farming conditions remained same as the rest of barn. All the birds were 47 days of age at this point. Phage or placebo were administered by oral gavage, 1 day prior to transport to the processing plant. The placebo group of chickens received 3 ml of sterile tap water while the phage treatment group were given 3 ml of 107 PFU/ml of each phage combined in sterile tap water. Food and water were withdrawn 8 h prior to collection according to normal farm practice. The following day, when the main cohort were collected for transport to the processing plant, 10 birds from each group were euthanized at the farm. A further 10 birds were euthanized following transport (which took approximately 4 h) and the carcasses of the final group were processed by the plant and collected before the chlorine rinse stage.
Sample Preparation and Enumeration of Campylobacter
Chicken ceca were removed aseptically and transported to the laboratory on ice. The ceca were chopped into fine material, from which 10 g samples were aseptically blended using a homogenizer for 1 min, with a diluent consisting of NB2 with LHB 5% (v/v). A 10 cm length of the ileum was measured from the ileal–cecal junction similarly transported on ice to laboratory. The total ileum contents were weighed, and appropriate 10-fold dilutions prepared. Carcasses were removed prior to chlorination and spin-chilling and placed in a sterile bag with 200 ml of 0.1% peptone (Oxoid LP0037) and shaken for 2 min in a shaker designed for the purpose. Ten-fold dilutions were prepared using Preston broth, and Campylobacter enumerated using a three tube, MPN technique for Farm B (Chinivasagam et al., 2009). Briefly the dilution tubes were incubated at 42°C for 48 h under microaerobic conditions, then sub-cultured on mCCDA and further incubated at 42°C for 48 h under microaerobic conditions, and finally scored as positive or negative. The MPN was calculated using tables with correction for dilution.
Enumeration of Phage in Ceca, Ileum and on Carcasses
Bacteriophages were enumerated from cecal and ileum contents by decimal dilution in SM buffer as described above. For carcass rinses, the suspension used for Campylobacter enumeration was diluted 1:1 in SM buffer and then treated as for the ceca and ileum samples.
Statistical Analysis
Data were analyzed using Prism8 (GraphPad Software, San Diego, CA, United States). The Shapiro–Wilks test of normality was employed on all log10-transformed bacterial counts or phage titers. Non-parametric tests were used as indicated when the data did not conform to normality at a significance level of 0.05. Spearman’s rank correlation coefficients were calculated using Prism8 to assess rank correlations between two variables from non-parametric data.
Results
Diversity of the 19-Phage Panel Against a Selection of Farm Campylobacter Isolates
The selected cocktail candidates demonstrated diversity in their lytic activity against farm Campylobacter isolates. Among the 241 farm Campylobacter isolates compared, 200 were identified as C. jejuni, 39 were C. coli, and two were not speciated. One hundred and eighty-eight of the Campylobacter strains (78%) were sensitive to at least one member of the bacteriophage panel. The results for each bacteriophage are shown in Table 2.
This analysis revealed that bacteriophages PH18 and PH19 had the broadest lytic activity, and although effective against most C. jejuni strains appeared to be more efficacious against C. coli. In contrast, some bacteriophages, for example, bacteriophages PH1, PH7, PH19, and PH17, were active against multiple C. jejuni strains, but did not lyze any of the C. coli isolates tested.
Selection of Suitable Locations for On-Farm Treatment Trials
Table 3 presents the Campylobacter and phage counts and Campylobacter species identity for 18 sheds screened across six potential farms. The lytic profiles for each of the 10 isolates tested were similar indicating that a single Campylobacter type was dominant at that point in the rearing cycle. All isolates tested from these six farms represented both C. jejuni and C. coli, with C. jejuni being dominant (Table 3) Rejected farms Farm D and F had a phage presence (though those farms met the other phage candidate selection criteria). The criteria for Campylobacter were met across all six farms which ranged from log10 7.00 to 9.00 CFU/g. For Farm A, all 10 isolates were sensitive to 13 out of the 19 candidate phages with only one phage having no activity (presented in Supplementary Table S1). Phages PH5, PH8, PH11, and PH13 were selected from the 13 candidates for the Farm A trial. For Farm B, Phages PH1–PH17 showed no activity against any of the 10 test isolates, but all the isolates were sensitive to PH18 and PH19 (Supplementary Table S1), which were therefore selected for Farm B trial.
Enumeration of Campylobacter and Phages From Intestinal Contents of Birds On-Farm and at the Plant
Campylobacter and phages were enumerated from cecal and ileal samples of the birds before transport and after transport, but only phages were enumerated from the processed carcasses of Farm B, due to high levels of competitive flora overgrowing the Campylobacter selective medium. Unlike the control log10 CFU/g Campylobacter counts from the ceca, the counts for the phage-treated birds were not normally distributed from Farm A (Shapiro–Wilk test). The median for the control was 6.91 log10 CFU/g compared to 5.79 log10 CFU/g for the phage treated birds (Figure 1A, Farm A). Non-parametric analysis indicates the phage treated cecal Campylobacter counts were significantly lower than controls (p = 0.007; Mann–Whitney U-test). The data feature an outlier of 8.36 log10 CFU/g that was investigated by reference to repeat enumeration data at 24 h and the phage titer recorded for the bird. The cecal Campylobacter count was confirmed and included in the analysis, but it was noted that the phage titer for the sample was the lowest recorded and may represent a situation where the phages have not efficiently replicated throughout the digesta in the treatment period. Following transport to the plant, no significant difference (p = 0.242; Mann–Whitney U-test) was observed between the Campylobacter counts in phage-treated and control birds (Figure 1A; Farm A), although the median for the control was greater at 7.39 log10 CFU/g compared to 6.49 log10 CFU/g for the phage treated birds. The Campylobacter counts for the phage-treated data also contained two high cecal counts > 8.0 log10 CFU/g that correspond with low phage titers. Analysis of the data using the Spearman’s rank correlation coefficient indicates a strong negative correlation is evident between the Campylobacter count and the phage titer in the treatment birds (rs = −0.746; p = 0.02). Figure 1B shows the titers of bacteriophages recovered on-farm and at the plant for Farm A (left panel). As expected, for the control birds from Farm A, bacteriophages were not detected (below limit of detection). There was no significant difference between farm and plant for those birds that had been treated with bacteriophage with median values of 5.6 and 5.0 log10 PFU/g cecal contents, respectively.
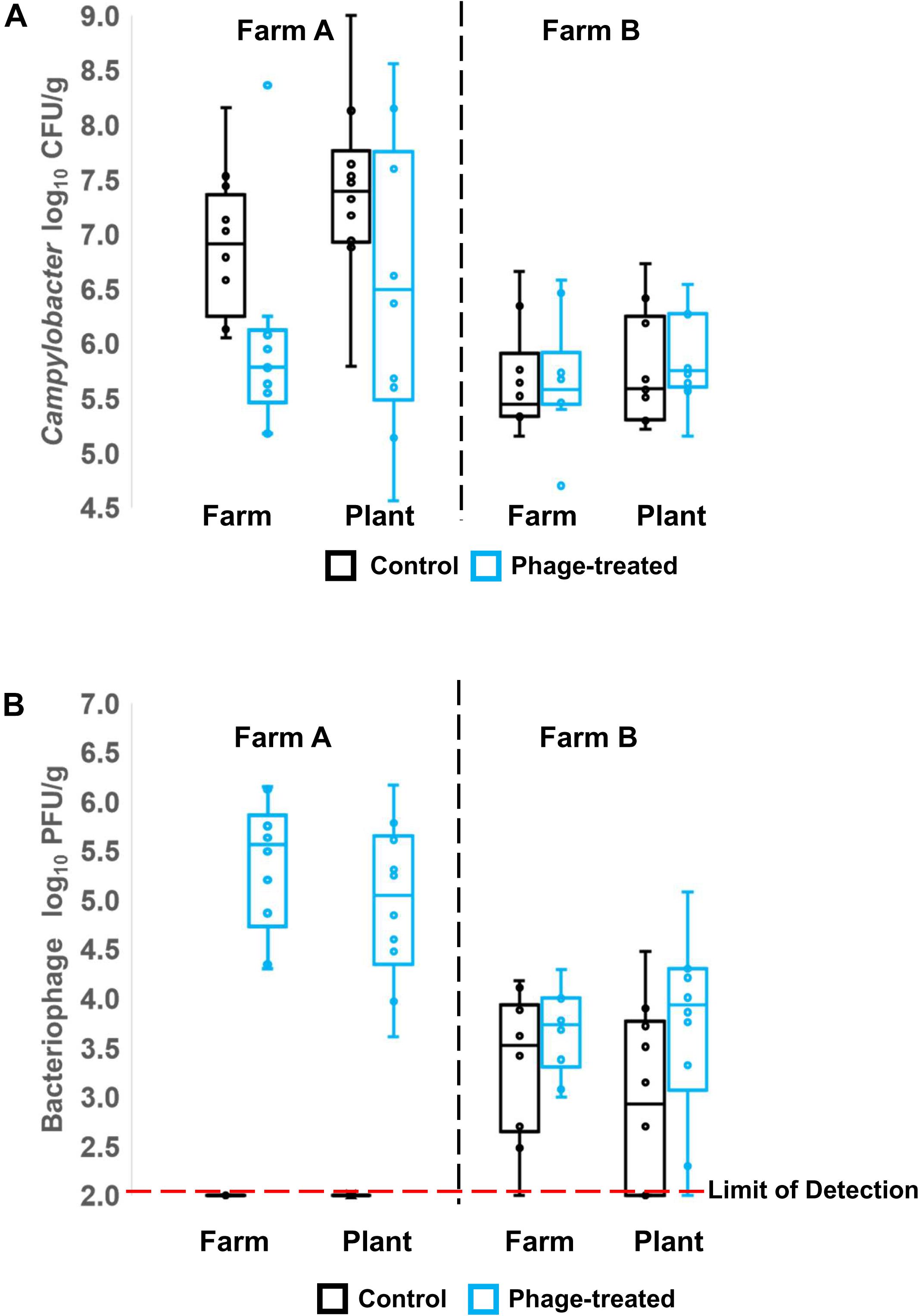
Figure 1. Box Whisper plots showing: (A) enumeration of Campylobacter in cecal contents, in control and phage-treated birds, comparing values on the farm and at the plant (after transport); (B) enumeration of bacteriophage in cecal contents, in control and phage-treated birds, comparing values on the farm and at the plant (after transport).
Figure 1A (right panel) shows the Campylobacter enumeration data for the cecal contents of birds from Farm B, on-farm and after transport to the plant. Campylobacter counts in the control and phage-treated birds either on farm (p = 0.373; Mann–Whitney U-test) or after transport (p = 0.384; Mann–Whitney U-test) were not significantly different. Enumeration of Campylobacter from the ileum did not show any significant difference at either farm or plant (data not shown). However, the phage titer data for farm B were unexpected in that control birds were colonized by phage despite having been administered a placebo and had been selected as phage-negative at pre-screen 1 week earlier (Figure 1B, right panel). The difference in the mean Campylobacter counts from Farm B at pre-screen and the experimental values of the control birds recorded on-farm represents a significant reduction of 1.7 log10 CFU/g (p = 0.006; Mann–Whitney U-test).
Enumeration of Campylobacter on Carcasses
The Campylobacter numbers recovered from carcasses comparing treated and control birds from Farm B were not significantly different (p = 0.406; Mann–Whitney U-test), although six carcasses were below detection limit of <6000 organisms per carcass. Bacteriophage were detected at low titer (<100 PFU/carcass) from four carcasses of Farm B, but not detected on any carcasses from Farm A.
Continued Sensitivity to Isolates to Cocktail Before and After Phage-Treatment
The lytic profile of the cocktail phages to Campylobacter strains isolated after phage treatment, from both farms were unchanged compared to those isolated before treatment (Table 4). Development of resistance to the treatment phages was therefore not detected.
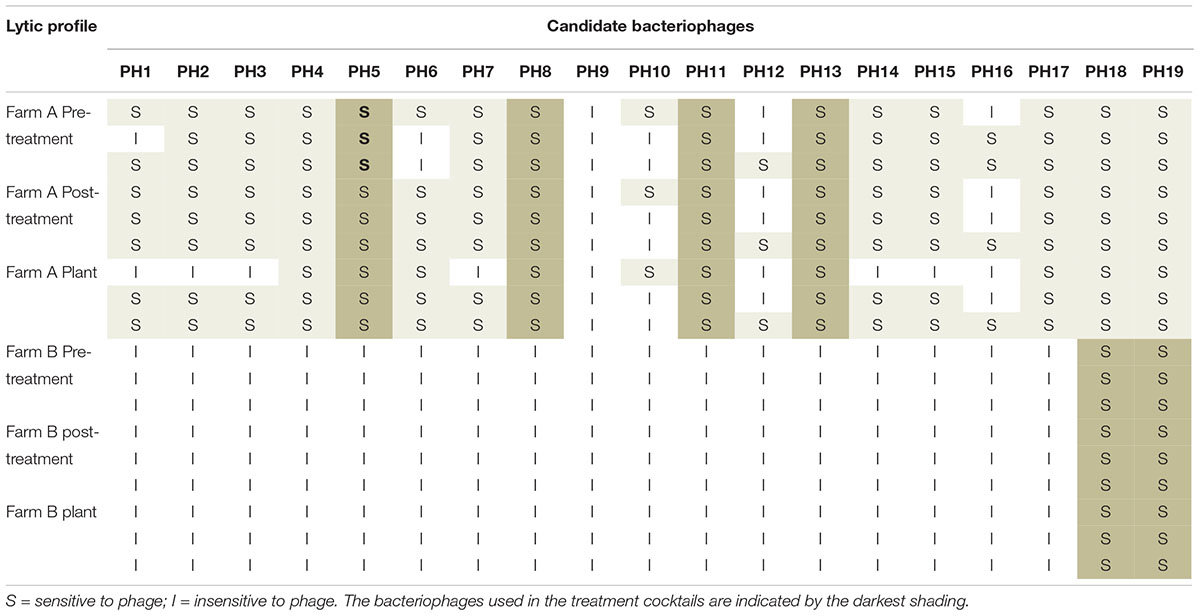
Table 4. Lytic profiles of Campylobacter isolates from Farm A and Farm B, to the candidate bacteriophage pre-and post-treatment, and after transport to the plant.
Discussion
One of the reasons that phage therapy was superseded by antibiotic therapies is that utilizing a biological agent requires care in selection of the appropriate agent and in application. However, the subtlety of being able to target pathogenic species within a complex microbiota, without causing dysbiosis represents a major advantage. Campylobacters are not overt pathogens of chickens so the ability to target the zoonotic component of the microbiota is a key advantage to the welfare of the bird and the quality of the product (Richards et al., 2019). However, for success the bacteriophages must be virulent against the target bacteria, and able to reach the target in sufficient quantities to affect a change in the host bacterial population. Exposure time is also a key consideration for a self-amplifying antimicrobial. Phages must have enough time to achieve a titer that will enable their access to all host-rich environments within the gut to kill the target bacteria. Intestinal transit will limit the exposure time to high titers, which will limit the development of resistance in the target populations. Experiments in the laboratory have gone a long way to understanding what is required for successful reduction of Campylobacter in chickens but studies are required to assess operation in commercial settings where the birds are subjected to feed withdrawal and the stress of transportation.
Selecting of the optimum phages from a panel that are active against this dominant Campylobacter is key to a successful intervention. Data from Campylobacter and non-Campylobacter studies suggest that the optimal number of phages in a cocktail is between two and four (for examples, see Carvalho et al., 2010; Fischer et al., 2013; Manohar et al., 2019). For Farm A, where 17 phages were active against the host bacteria, using four as the maximum was a logical choice. However, for Farm B, only two of the candidate phages had activity to the Campylobacter host, so only two phages were used in the cocktail. The use of the four-phage cocktail on Farm A brought about a significant decline in the Campylobacter count compared to control birds within the ceca that represents the major reservoir of intestinal contamination.
Once the birds had been transported to the processing plant, it is possible that the increases in the mean Campylobacter counts for both phage-treated and control birds were due to transport stress. Increases in Campylobacter count following transport have been noted previously for cecal content and fecal matter, which have been postulated to arise as a result of the effects of transport on peristaltic movements of the chicken gut (Stern et al., 1995; Whyte et al., 2001). This was not manifest as a consistent increase in all birds, but it appeared that some birds were more affected than others, illustrated by the wide range of counts in the phage-treated birds including some that were very high at approximately 8 log10 CFU/g and some that were below 6 log10 CFU/g. The data do, however, demonstrate a strong negative correlation between the Campylobacter count and the phage titer. The high Campylobacter counts in some of the phage-treated birds may therefore represent birds where the phages have not attained a great enough titer in the ceca to affect a reduction in the population. All birds were treated similarly so the failure is likely due to low host concentrations encountered in the amplification phase, for example, in the intestinal tract prior to reaching the ceca. Success under these circumstances would then be reliant on titer amplification and dissemination in the ceca, which is subject to regular cecal evacuation. The treatment period to affect the Campylobacter reductions in as many birds as possible needs to be increased from 24 h as the phage may not have sufficient time to achieve the titer and/or the required dispersion within the intestine. However, in the majority of the phage treated birds of Farm A, the phage did replicate and were effective at reducing Campylobacter numbers in the cecal contents.
The main difference from experimental models is that birds become naturally infected with Campylobacter strains that provoke competition with each other. Although dominant types can emerge that predominate in surveys (El-Shibiny et al., 2005). Similarly, biosecurity measures applied on a commercial farm may not prevent the incursion of native phages from the environment. Phage isolation studies report variable frequencies of between 20 and 50% recovery from chicken sources, which suggests phages are not always present in Campylobacter infected flocks (Atterbury et al., 2005; El-Shibiny et al., 2005; Owens et al., 2013). Campylobacters exposed to phage can become resistant to the infecting phage; however, there is frequently an associated cost in competitive fitness (Connerton et al., 2004; Scott et al., 2007). Thus, selection favors phage-sensitive hosts when phages are not present or are at low titers. Commercial farms are challenged by multiple campylobacters that undergo succession, and one facet of the competition is the evasion of phage. A longitudinal study across successive flocks of a Campylobacter and phage infected barn resulted in the elimination of the phage when a new phage insensitive strain entered the environment to dominate (Connerton et al., 2004). This scenario is consistent with evidence from Farm B that became colonized by a previously undetectable phage that proliferated on sensitive Campylobacter populations in the final week of rearing after the pre-screen. However, we also acknowledge that the presence of an indigenous phage, albeit not detected at pre-screen, may also have affected the narrow choice of treatment phage able to lyse campylobacters from Farm B. A similar situation was also reported by Kittler et al. (2013), in one of three sites investigated. The infiltrating phages clearly spread rapidly throughout the flock and it seems likely that they reduced the numbers of Campylobacter in cecal contents of both control and test birds, either before or concurrently, with the phage intervention. At the time of sampling, the average Campylobacter number in the cecal contents was 5.6 log10 CFU/g in contrast to 7.8 log10 CFU/g, when pre-screened, 1 week earlier, with no phages detected. While this makes the results difficult to interpret with respect to the treatment phage, it sheds light on the process of concurrent phage infection that are part of the natural Campylobacter–phage interactions occurring in the intestinal tracts of farm chickens, and confirms earlier reports that the presence of phage in broiler flocks can reduce cecal Campylobacter population levels (Atterbury et al., 2005). Reduced concentrations of the target bacteria below the phage proliferation threshold will also diminish the likelihood of in situ amplification of the treatment phage and the effectiveness of the intervention (Cairns et al., 2009). These observations suggest that the selection of the treatment phage should also take in to account the action of concurrent phage infections, which may make phage intervention more effective if optimized correctly.
The development of resistance to phage treatment is often cited as a negative aspect of phage intervention but resistance to the phage cocktails selected was not detected on either farm. This may have been partly because the time between intervention and slaughter was less than 24 h, which reduces the time for resistant strains to emerge although this was also minimized by using cocktails of phages rather than just one. Increasing the time between treatment and slaughter from 24 to 2–4 days may increase the effectiveness of treatment (Loc Carrillo et al., 2005; Wagenaar et al., 2005; El-Shibiny et al., 2009; Kittler et al., 2013) but may also lead to the emergence of resistance. The short exposure of the birds to the phage cocktail treatment may have contributed to low phage titers observed and the strong correlation with the Campylobacter counts observed on Farm A in the absence of emerging phage resistant populations. Phage resistant mutants were also absent amongst the campylobacters recovered from birds of Farm B that had been pre-exposed to indigenous phage. A combination of competition and exposure to new virulent phage may have eliminated any resistant types in the treatment group. However, phage insensitive isolates were also not evident from the control group Campylobacter isolates. The residual population surviving intestinal colonization by the indigenous phage may not have been exposed to the phage to become resistant, and similarly were either not exposed to the treatment phage or not exposed to sufficient phage titer to affect a further reduction in the colonization level. This study highlights a general need to understand system specific phage–host interactions that are likely critical for successful treatment outcomes.
Data Availability Statement
The datasets generated for this study are available on request to the corresponding author.
Ethics Statement
The experimental design was approved by the Animal Ethics Committee of the Department of Agriculture and Fisheries Queensland before commencing farm screening and experimental intervention trials. Broiler chickens were reared in accordance with the National Animal Welfare Standards for the Chicken Meat Industry (available at: chicken.org.au/wp-content/uploads/2017/09/ME-083-Chicken-Standards-The-Standards-3.pdf) and the National Farm Biosecurity Manual for chicken growers (available at: chicken.org.au/wp-content/uploads/2017/09/National-Farm-Biosecurity-Manual-for-Chicken-Growers-Feb-2010-web.pdf).
Author Contributions
HC and IC designed the experiments. LM executed the farm trials, WE, LM, CW, and HC executed the lab experiments. HC, PC, IC, and DM analyzed the data. HC, PC, and IC prepared the manuscript.
Funding
This study received funding from Poultry CRC. The funder was not involved in the study design, collection, analysis, interpretation of data, the writing of this article or the decision to submit it for publication.
Conflict of Interest
The authors declare that the research was conducted in the absence of any commercial or financial relationships that could be construed as a potential conflict of interest.
Acknowledgments
We are grateful to Margaret MacKenzie and Kelly McTavish from the integrator company for providing industry access to enable the performance of these trials and logistical support with planning and executing these trials on farm and the plant. We acknowledge the support of Katherine McGlasshan for lab experiments during both farm trials. We acknowledge the contributions of the farmers for providing access and support to maintain the trial conditions.
Supplementary Material
The Supplementary Material for this article can be found online at: https://www.frontiersin.org/articles/10.3389/fmicb.2020.00632/full#supplementary-material
Footnotes
References
Atterbury, R. J., Connerton, P. L., Dodd, C. E., Rees, C. E., and Connerton, I. F. (2003). Application of host-specific bacteriophages to the surface of chicken skin leads to a reduction in recovery of Campylobacter jejuni. Appl. Environ. Microbiol. 69, 6302–6306. doi: 10.1128/aem.69.10.6302-6306.2003
Atterbury, R. J., Dillon, E., Swift, C., Connerton, P. L., Frost, J. A., Dodd, C. E., et al. (2005). Correlation of Campylobacter bacteriophage with reduced presence of hosts in broiler chicken ceca. Appl. Environ. Microbiol. 71, 4885–4887. doi: 10.1128/aem.71.8.4885-4887.2005
Bolton, F. J., Coates, D., Hinchliffe, P. M., and Robertson, L. (1983). Comparison of selective media for isolation of Campylobacter jejuni/coli. J. Clin. Pathol. 36, 78–83. doi: 10.1136/jcp.36.1.78
Cairns, B. J., Timms, A. R., Jansen, V. A., Connerton, I. F., and Payne, R. J. (2009). Quantitative models of in vitro bacteriophage-host dynamics and their application to phage therapy. PLoS Pathog. 5:e1000253. doi: 10.1371/journal.ppat.1000253
Carvalho, C., Susano, M., Fernandes, E., Santos, S., Gannon, B., Nicolau, A., et al. (2010). Method for bacteriophage isolation against target Campylobacter strains. Lett. Appl. Microbiol. 50, 192–197.
Chinivasagam, H. N., Tran, T., Maddock, L., Gale, A., and Blackall, P. J. (2009). Mechanically ventilated broiler sheds: a possible source of aerosolized Salmonella, Campylobacter, and Escherichia coli. Appl. Environ. Microbiol. 75, 7417–7425. doi: 10.1128/aem.01380-09
Connerton, P. L., Loc Carrillo, C. M., Swift, C., Dillon, E., Scott, A., Rees, C. E., et al. (2004). Longitudinal study of Campylobacter jejuni bacteriophages and their hosts from broiler chickens. Appl. Environ. Microbiol. 70, 3877–3883. doi: 10.1128/aem.70.7.3877-3883.2004
Connerton, P. L., Timms, A. R., and Connerton, I. F. (2011). Campylobacter bacteriophages and bacteriophage therapy. J. Appl. Microbiol. 111, 255–265. doi: 10.1111/j.1365-2672.2011.05012.x
Crotta, M., Georgiev, M., and Guitian, J. (2017). Quantitative risk assessment of Campylobacter in broiler chickens – Assessing interventions to reduce the level of contamination at the end of the rearing period. Food Control 75, 29–39. doi: 10.1016/j.foodcont.2016.12.024
El-Shibiny, A., Connerton, P. L., and Connerton, I. F. (2005). Enumeration and diversity of campylobacters and bacteriophages isolated during the rearing cycles of free-range and organic chickens. Appl. Environ. Microbiol. 71, 1259–1266. doi: 10.1128/aem.71.3.1259-1266.2005
El-Shibiny, A., Scott, A., Timms, A., Metawea, Y., Connerton, P., and Connerton, I. (2009). Application of a group II Campylobacter bacteriophage to reduce strains of Campylobacter jejuni and Campylobacter coli colonizing broiler chickens. J. Food Prot. 72, 733–740. doi: 10.4315/0362-028x-72.4.733
European Food Safety Authority [EFSA] (2010). Scientific Opinion on Quantification of the risk posed by broiler meat to human campylobacteriosis in the EU. EFSA J. 8:1437. doi: 10.2903/j.efsa.2010.1437
European Food Safety Authority [EFSA], and European Centre for Disease Prevention and Control [ECDC] (2018). The European Union summary report on trends and sources of zoonoses, zoonotic agents and food-borne outbreaks in 2017. EFSA J. 16:5500. doi: 10.2903/j.efsa.2018.5500
Fischer, S., Kittler, S., Klein, G., and Glünder, G. (2013). Impact of a single phage and a phage cocktail application in broilers on reduction of Campylobacter jejuni and development of resistance. PLoS One 8:e78543. doi: 10.1371/journal.pone.0078543
Goode, D., Allen, V. M., and Barrow, P. A. (2003). Reduction of experimental Salmonella and Campylobacter contamination of chicken skin by application of lytic bacteriophages. Appl. Environ. Microbiol. 69, 5032–5036. doi: 10.1128/aem.69.8.5032-5036.2003
Goodridge, L. D., and Bisha, B. (2011). Phage-based biocontrol strategies to reduce foodborne pathogens in foods. Bacteriophage 1, 130–137. doi: 10.4161/bact.1.3.17629
Hall, G., Yohannes, K., Raupach, J., Becker, N., and Kirk, M. (2008). Estimating community incidence of Salmonella, Campylobacter, and Shiga toxin-producing Escherichia coli infections, Australia. Emerg. Infect. Dis. 14, 1601–1609.
Havelaar, A. H., Mangen, M. J., de Koeijer, A. A., Bogaardt, M. J., Evers, E. G., Jacobs-Reitsma, W. F., et al. (2007). Effectiveness and efficiency of controlling Campylobacter on broiler chicken meat. Risk Anal. 27, 831–844. doi: 10.1111/j.1539-6924.2007.00926.x
Kaakoush, N. O., Castaño-Rodríguez, N., Mitchell, H. M., and Man, S. M. (2015). Global epidemiology of Campylobacter infection. Clin. Microbiol. Rev. 28, 687–720. doi: 10.1128/cmr.00006-15
Kittler, S., Fischer, S., Abdulmawjood, A., Glünder, G., and Klein, G. (2013). Effect of bacteriophage application on Campylobacter jejuni loads in commercial broiler flocks. Appl. Environ. Microbiol. 79, 7525–7533. doi: 10.1128/aem.02703-13
Loc Carrillo, C., Atterbury, R. J., El-Shibiny, A., Connerton, P. L., Dillon, E., Scott, A., et al. (2005). Bacteriophage therapy to reduce Campylobacter jejuni colonization of broiler chickens. Appl. Environ. Microbiol. 71, 6554–6563. doi: 10.1128/aem.71.11.6554-6563.2005
Loc Carrillo, C. M., Connerton, P. L., Pearson, T., and Connerton, I. F. (2007). Free-range layer chickens as a source of Campylobacter bacteriophage. Antonie Van Leeuwenhoek 92, 275–284. doi: 10.1007/s10482-007-9156-4
MacRitchie, L. A., Hunter, C. J., and Strachan, N. J. C. (2014). Consumer acceptability of interventions to reduce Campylobacter in the poultry food chain. Food Control 35, 260–266. doi: 10.1016/j.foodcont.2013.06.005
Manohar, P., Tamhankar, A. J., Lundborg, C. S., and Nachimuthu, R. (2019). Therapeutic characterization and efficacy of bacteriophage cocktails infecting Escherichia coli, Klebsiella pneumoniae, and Enterobacter species. Front. Microbiol. 10:574. doi: 10.3389/fmicb.2019.00574
Mughini Gras, L., Smid, J. H., Wagenaar, J. A., de Boer, A. G., Havelaar, A. H., Friesema, I. H., et al. (2012). Risk factors for campylobacteriosis of chicken, ruminant, and environmental origin: a combined case-control and source attribution analysis. PLoS One 7:e42599. doi: 10.1371/journal.pone.0042599
Owens, J., Barton, M. D., and Heuzenroeder, M. W. (2013). The isolation and characterization of Campylobacter jejuni bacteriophages from free range and indoor poultry. Vet. Microbiol. 162, 144–150. doi: 10.1016/j.vetmic.2012.08.017
Ravel, A., Hurst, M., Petrica, N., David, J., Mutschall, S. K., Pintar, K., et al. (2017). Source attribution of human campylobacteriosis at the point of exposure by combining comparative exposure assessment and subtype comparison based on comparative genomic fingerprinting. PLoS One 12:e0183790. doi: 10.1371/journal.pone.0183790
Richards, P. J., Connerton, P. L., and Connerton, I. F. (2019). Phage biocontrol of Campylobacter jejuni in chickens does not produce collateral effects on the gut microbiota. Front. Microbiol. 10:476. doi: 10.3389/fmicb.2019.00476
Scott, A. E., Timms, A. R., Connerton, P. L., El-Shibiny, A., and Connerton, I. F. (2007). Bacteriophage influence Campylobacter jejuni types populating broiler chickens. Environ. Microbiol. 9, 2341–2353. doi: 10.1111/j.1462-2920.2007.01351.x
Stern, N. J., Clavero, M. R., Bailey, J. S., Cox, N. A., and Robach, M. C. (1995). Campylobacter spp. In broilers on the farm and after transport. Poult. Sci. 74, 937–941. doi: 10.3382/ps.0740937
Wagenaar, J. A., Van Bergen, M. A., Mueller, M. A., Wassenaar, T. M., and Carlton, R. M. (2005). Phage therapy reduces Campylobacter jejuni colonization in broilers. Vet. Microbiol. 109, 275–283. doi: 10.1016/j.vetmic.2005.06.002
Keywords: bacteriophage, Campylobacter, broiler chicken, Queensland (Australia), poultry
Citation: Chinivasagam HN, Estella W, Maddock L, Mayer DG, Weyand C, Connerton PL and Connerton IF (2020) Bacteriophages to Control Campylobacter in Commercially Farmed Broiler Chickens, in Australia. Front. Microbiol. 11:632. doi: 10.3389/fmicb.2020.00632
Received: 26 September 2019; Accepted: 20 March 2020;
Published: 27 April 2020.
Edited by:
Nicolae Corcionivoschi, Agri-Food and Biosciences Institute (AFBI), United KingdomReviewed by:
Manan Sharma, United States Department of Agriculture (USDA), United StatesMariana Carmen Chifiriuc, University of Bucharest, Romania
Copyright © 2020 The State of Queensland (through the Department Agriculture and Fisheries). This is an open-access article distributed under the terms of the Creative Commons Attribution License (CC BY). The use, distribution or reproduction in other forums is permitted, provided the original author(s) and the copyright owner(s) are credited and that the original publication in this journal is cited, in accordance with accepted academic practice. No use, distribution or reproduction is permitted which does not comply with these terms.
*Correspondence: Helene N. Chinivasagam, bmFsaW5pLmNoaW5pdmFzYWdhbUBkYWYucWxkLmdvdi5hdQ==; Ian F. Connerton, aWFuLmNvbm5lcnRvbkBub3R0aW5naGFtLmFjLnVr