- 1School of Pharmacy, Nanjing University of Chinese Medicine, Nanjing, China
- 2Jiangsu Engineering Research Centre for Efficient Delivery System of TCM, School of Pharmacy, Nanjing University of Chinese Medicine, Nanjing, China
- 3Jiangsu Key Laboratory of Pediatric Respiratory Disease, Nanjing University of Chinese Medicine, Nanjing, China
Intestinal microbiota has been extensively studied in the context of host health benefit, and it has recently become clear that the gut microbiota influences drug pharmacokinetics and correspondingly efficacy. Intestinal microbiota dysbiosis is closely related with liver cirrhosis, especially the depletion of Lactobacillus. Therefore, the bioavailability of orally administered glycyrrhizic acid (GL) was speculated to be influenced under a pathological state. In the present study, L. murinus was isolated and screened for GL bioconversion capacity in vitro. Compared with Lactobacillus rhamnosus and Lactobacillus acidophilus, L. murinus was chosen for further investigation because it has the highest biotransformation rate. Our results showed that L. murinus could significantly improve the translocation of GL on Caco-2 cell models. Meanwhile, L. murinus was observed to have the ability to bind with the surface of Caco-2 cells and prominently downregulate the transporter gene expression level of multidrug resistance gene 1 (MDR1) and multidrug resistance protein 2 (MRP2), which were involved in the efflux of drugs. Furthermore, L. murinus was selected to be orally administred into rats in healthy and liver cirrhosis groups by a daily gavage protocol. Our data highlighted that supplements of L. murinus significantly improved the bioavailability of orally administered GL in rats, especially under a pathological condition, which may provide a novel strategy for improving the clinical therapeutic effect of liver protective drugs.
Introduction
The human microbiome is composed of trillions of bacteria, viruses, and fungi, whose collective genome contains at least 100-fold more genes than the complete human genome (Stojancevic et al., 2014). By far, the organ colonized with the largest microbial population is the gastrointestinal (GI) tract, which contains over 70% of all the microbes in the human body (Sekirov et al., 2010). The intestinal microflora that form a complex ecosystem play a key role in the modulation of human health and diseases. Additionally, gut microbiota has also exhibited the ability to metabolize endogenous and exogenous substances, especially orally administered drugs; more than 50 Food and Drug Administration (FDA)-approved drugs have been reported to have clinical interaction with gut microbiota (Wilson and Nicholson, 2017; Zhang et al., 2018). Increasing evidence showed that gut microbiota could directly affect the metabolism of drugs and alter their pharmacological effects.
In general, the oral route of drug administration is still the easiest and convenient way for drug application (Sastry et al., 2000). The liver has long been considered to be the major organ for drug metabolism, which is mainly responsible for the hydrophobic xenobiotic conversion of drugs into hydrophilic products (Kim, 2015), while the GI microflora mainly metabolize the phytochemicals into hydrophobic compounds through various microbial enzymes (Choi et al., 2018). More importantly, metabolizing drugs through the gut microbiota prior to absorption can alter the systemic bioavailability of certain drugs. Most herbal medicines are extracted by water or ethanol to generate decorations, infusions, or tincture for oral administration (Xu et al., 2017). A wide range of herbal medicine components, including ginsenosides from Ginseng (Kim, 2018), astilbin from Smilax glabra Roxb (Zhao et al., 2014), and berberine from Coptis chinensis (Feng R. et al., 2015), inevitably come into the GI tract. Orally administered herbal medicines are metabolized by the gut microbial enzyme before their absorption into the blood. However, studies on the impact of the gut microbial on drug metabolism and efficacy are still insufficient.
Glycyrrhizic acid (GL) is the major bioactive triterpene glycoside of the Chinese herb gancao, which has a variety of biological activities, such as anti-inflammatory, antioxidation, antiviral, immunoregulation, and liver protection (Armanini et al., 2002; Hosseinzadeh and Nassiri-Asl, 2015; Han et al., 2017). However, GL is difficult to absorb after oral administration because of its large relative molecular mass and polarity. GL needs to be deglycosylated into glycyrrhetinic acid (GA) by various gut microbiota, which is then absorbed into the blood to show efficacy (Akao, 2000). Additionally, our previous research work showed that the relative abundance of Lactobacillus was extremely decreased in the liver cirrhosis group compared with the healthy group. Accordingly, it was speculated that GL bioavailability was influenced by a shortage of Lactobacillus under a pathological state (Yuan et al., 2019). Recent research work showed that the bioavailability of orally administered drugs was directly influenced by the disturbance of gut microbiota. Escherichia coli Nissle 1917 could lead to a 43% increase of amiodarone area under the curve (AUC) compared with that in the control samples (Matuskova et al., 2014), while Lactobacillus reuteri K8 could decrease orally administered acetaminophen to 68.4% compared with that in the normal control group (Kim et al., 2018). Nevertheless, the knowledge of the effects of administration of Lactobacillus on GL pharmacokinetics has not been investigated, especially under a pathological state, which may provide new strategies to improve the therapeutic efficacy of GL.
Therefore, in this current study, three lactobacilli for GL biotransformation were firstly screened. Lactobacillus murinus was isolated from healthy rats’ feces and identified by 16S rDNA sequencing. L. murinus was considered as the predominant Lactobacillus species in rat gut, one of the most frequently found Lactobacillus strains. Lactobacillus acidophilus and Lactobacillus rhamnosus were both reported as beneficial probiotics, and L. acidophilus has been proven to play an important role in the deglucosylation step of glycoconjugated phytochemicals (Theilmann et al., 2017). Meanwhile, L. rhamnosus was demonstrated to have the ability for GL bioconversion in our previous research work. Therefore, the two common and available lactobacilli L. acidophilus and L. rhamnosus were chosen for the GL bioconversion compared with L. murinus in our study. Due to having the highest GL biotransformation rate as well as itself originating from the rat gut, L. murinus was selected for further research. Additionally, the beneficial properties of L. murinus on GL bioabsorption were primarily investigated in vitro. Moreover, the potential effects of selected L. murinus on GL bioavailability of healthy and liver cirrhosis rat models were explored.
Materials and Methods
Chemicals and Reagents
GL (≥98% purity) and GA (≥98% purity) were purchased from Yuanye Bio-Technology Co. (Shanghai, China). Acetonitrile and methanol were purchased from Merck KGaA (Darmstadt, Germany). Formic acid (FA) was bought from ACS (Wilmington, United States), and distilled water was prepared using a Milli-Q purification system (Millipore, Billerica, MA, United States). CCl4 and olive oil were purchased from Macklin Biochemical Technology Co. (Shanghai, China). An alanine aminotransferase (ALT) assay kit and aspartate aminotransferase (AST) assay kit were purchased from Jiancheng Bioengineering Institute (Nanjing, China). A MiniBEST Universal RNA Extraction Kit, PrimeScriptTM RT Reagent Kit with gDNA Eraser (Perfect Real Time), and SYBR® Premix Ex TaqTM (Tli RNaseH Plus) were purchased from Takara Bio (Tokyo, Japan). All mobile-phase solvents were of HPLC grade, and all reagents were of analytical reagent grade.
Animals
Specific pathogen-free Sprague-Dawley rats (180–220 g) were purchased from the Experimental Animal Center of Zhejiang Province (Zhejiang, China), housed under controlled condition (light/dark, every 12 h; temperature, 20–22°C; and humidity, 50 ± 10%) and acclimated for 1 week before the experiments. Rats were fed standard laboratory chow and water ad libitum. The protocols for animal studies were performed according to the National Institutes of Health Guide for the Care and Use of Laboratory Animals and were approved by the Animal Studies Ethics Committee of Nanjing University of Chinese Medicine.
Bacterial Strains and Culture Condition
L. rhamnosus and L. acidophilus were purchased from the China General Microbiological Culture Collection Center (CGMCC) under accession numbers 1.8882 and 1.1878. L. murinus was isolated from healthy rats’ feces. All cultures were grown in Man, Rogosa, and Sharpe (MRS) broth (Solarbio, Beijing, China) and propagated twice in MRS broth prior to use. All strains were grown overnight at 37°C in an anaerobic culture tank (volume: 2.5 L, Mitsubishi, Tokyo, Japan) with an anaerobic gas bag.
Caco-2 Cell Culture
Caco-2 cells were preserved in our own laboratory. Caco-2 cells were maintained at 37°C under atmospheric conditions with 5% CO2. Cells were cultured in Dulbecco’s modified Eagle medium (DMEM, catalog number: SH30022.01; HyClone) supplemented with 10% heat-inactivated fetal bovine serum (Gibco), 1% MEM non-essential amino acids (catalog number: 321-011-EL; Wisent), and 1% penicillin–streptomycin (catalog number: SV30010.01; HyClone). The medium was changed every 3 days. When the cells reached 80–90% confluence, the cells were collected through trypsin digestion with 0.25% trypsin–EDTA (Yeasen, Shanghai, China).
LC–MS/MS Methods
LC–MS/MS analyses were performed using a Waters HPLC system (Milford, MA, United States) coupled to a Waters Xevo TQD triple-quadrupole mass spectrometer (Milford, MA, United States). The mass spectrometer was operated using an electrospray atmospheric pressure ionization source in negative ion mode (ESI-) with multiple reaction monitoring (MRM). The analytical column was an ACQUITY UPLC® BEH C18, 2.1 × 100 mm, 1.7 μM (Milford, MA, United States), and column temperature was maintained at 40°C. The mobile phase consisted of (A) 0.4% FA in distilled water and (B) acetonitrile. A gradient program was used as follows: 0–1 min, 70–20% A; 1–3 min, 20% A; 3–3.5 min, 20–70% A; 3.5–4.5 min, 70% A. The flow rate was 0. ml/min, and the sample injection volume was 10 μl.
Sensitivity of MRM was optimized by infusing a mixture of GL and GA, 1 μg/ml each, in the mobile phase. The capillary voltage was maintained at 2.66 kV, and the cone was set to 60 V. The desolvation and ion source temperatures were 300 and 150°C, respectively. The drying gas flow was set at 50 L/min. To assay all analytes, both quadrupoles were maintained at unit resolution, and the transitions (precursor to daughter) monitored were m/z 821.3 → 851 for GL and m/z 469.1 → 470 for GA. MRM data were acquired, and the chromatograms were integrated using a MassLynx V4.1 workstation.
Transwell Experimentation and Sample Preparation
Cells were seeded onto 12-well Transwell plates (0.4-μM-pore-size polyester membrane insert, catalog number: 3460; Corning) at a concentration of 1.5 × 105 cells/cm2. Cells were cultured for 21 days as previously described, and the medium was replaced every 2 days. Transepithelial electric resistance (TEER) assays were undertaken to confirm Caco-2 monolayer integrity. The cell monolayers were considered tight enough for the transport experiments when the TEER value was above 300 Ω⋅cm2. Prior to absorption experimentation, apical and basolateral compartments were washed three times and incubated in Hanks’ balanced salt solution (catalog number: RNBF9191; Sigma) at 37°C. Cells were treated apically with 1,000 μM GL or 10 μM GA in the presence or absence of 109 colony-forming unit (CFU)/ml of L. murinus. Basolateral sampling (100 μl) with replacement was performed at 2 h.
The samples were diluted with methanol and vortex-mixed for 5 min and then centrifuged at 14,000 rpm for 7 min. The supernatant was injected into the LC–MS/MS analysis system. Apparent permeability coefficients (Papp) were calculated using the equation following:
where dQ/dt is the transport rate on the receiver side (μg/s), A is the membrane surface area of the inset (1.1 cm2), and C0 is the initial drug concentration in the donor compartment (μg/ml).
Caco-2 and Bacterial Cell Co-culture
L. murinus from stationary-phase cultures were washed by phosphate-buffered saline (PBS), following centrifugation at 2,500 × g for 5 min and resuspension in DMEM. Approximately 109 CFUs were added to each well. Caco-2 cells cultured in 12-well plates (Corning) were prepared 24 h prior to the adhesion assay. The cultures were washed three times by PBS, replenished with serum- and antibiotic-free medium (DMEM) to eliminate any interference, and incubated for 24 h. On the day of the assay, the co-cultures were washed four times with PBS, a bacteria-supplemented medium was added to each well, and plates were incubated at 37°C in a 5% CO2 atmosphere for 3 h.
Scanning Electron Microscopy
The Caco-2 monolayers cells were grown and maintained in a method similar to that mentioned above in 12-well tissue culture plates. After the incubation of bacteria with Caco-2 cells for 3 h, the cell monolayer with adhered bacteria was washed four times with PBS to remove the unbound bacteria. Later on, they were fixed with 2.5% glutaraldehyde and incubated at 4°C overnight. Alcohol dehydration in ascending gradation was performed, and the slides were coated with gold plating and observed under a scanning electron microscopy (SEM) (Hitachi SU8010, Japan).
RNA Extraction and Quantitative Real-Time PCR
Total RNA was isolated from Caco-2 samples using a MiniBEST Universal RNA Extraction Kit and reverse-transcribed into cDNA using a PrimeScriptTM RT Reagent Kit with gDNA Eraser according to the manufacturer’s protocol. RNA and cDNA extracts were stored until use at −80 and −20°C, respectively. Custom primers were listed in Supplementary Table 1. Primers were characterized by melting curve analysis, agarose gel electrophoresis, and DNA sequencing and synthesized at GenScript Co. (Nanjing, China). Quantitative RT-PCR was performed on a CFX96 real-time PCR detection system (Bio-Rad, United States) using SYBR® Premix Ex TaqTM (Tli RNaseH Plus), Bulk. The relative expression of each gene was measured in triplicate via the comparative cycle threshold method, and we used the housekeeping gene GAPDH as the internal standard.
Experimental Design and Model of Liver Fibrosis
We designed the following liver fibrosis experimental protocol as shown in Figure 4A. Rats were randomized into four groups (n = 6 for each group) as follows: the control group treated with normal saline (control group), supplementation with L. murinus (control + L. murinus group), the CCl4-induced fibrosis group treated with normal saline (NS group), and supplementation with L. murinus (NS + L. murinus group). Liver fibrosis was induced via an intraperitoneal injection of a 50% (V/V) CCl4 solution in olive oil twice per week at a dose of 1 ml/kg for 6 weeks. In the control rats, CCl4 was replaced with olive oil (Shi et al., 2017). At the seventh week of the experiment, animals in the NS and control groups were treated with normal saline at a dose of 1 ml, and animals in other groups were treated with a dose of 1 ml L. murinus by gavage once daily for 1 week. Blood was taken from the orbital vein, and blood samples were centrifuged at 3,000 × g for 15 min at room temperature to separate the serum for analysis. The serum levels of ALT and AST were assessed using an assay kit according to the manufacturer’s directions.
Pharmacokinetic Experiments
Rats pretreated with L. murinus or normal saline (n = 6) were administered an oral (10 mg/kg) dose of GL dissolved in 0.5% sodium carboxymethyl cellulose (CMC-Na) (5 mg/ml) 24 h after completing the seven consecutive daily treatment with physiological saline (control) or L. murinus dissolved in physiological saline (1 × 109 CFU/mouse). Whole-blood samples were taken from the orbital vein into tubes with heparin sodium at 0.17, 0.33, 0.5, 0.75, 1, 2, 4, 6, 8, 12, 24, 48, 72 h after oral administration (Zhou et al., 2013; Wang et al., 2017). Plasma was harvested by centrifugation at 8,000 rpm for 10 min and stored at −20°C until analyzed.
Blood Sample Preparation
A 100 μl plasma sample was deproteinized with 1,000 μl methanol containing 10 μl internal standard (2 μg/ml). The sample was vortex-mixed for 5 min and then centrifuged at 14,000 rpm for 7 min. The supernatant was evaporated to dryness under nitrogen gas and redissolved in 80 μl mobile phase. After centrifugation at 14,000 rpm for 7 min, an aliquot of 10 μl of each sample was injected into the LC–MS/MS analysis system.
Pharmacokinetic Analysis
After analyzing plasma samples, we obtained the plasma concentration–time profiles. The maximum plasma concentration (Cmax) and the time taken to reach max (Tmax) for GL or GA were estimated directly from the plasma concentration–time profiles. The Drug and Statistics v3.0 (Drug Clinical Research Center of Shanghai University of Traditional Chinese Medicine, Shanghai, China) was used with a non-compartmental statistical model to determine other kinetic parameters of the plasma samples.
Statistical Analysis
GraphPad Prism 5 software (GraphPad Software, San Diego, CA, United States) was used to perform statistical analysis. The data were presented as mean ± standard derivation if normally distributed, and statistical significance was analyzed by Student’s t-test or ANOVA. Otherwise, the values were presented as median (25th and 75th) and analyzed by a non-parametric test (Mann–Whitney test). P < 0.05 was considered as statistically significant.
Results
In vitro Screening of Lactobacillus for GL Biotransformation
Lactobacillus is largely considered to play a beneficial role for the host’s health; moreover, in our previous research work, Lactobacillus was significantly reduced in the pathological condition of rat liver cirrhosis, which was speculated to have an effect on GL bioavailability. In order to investigate the relationship between Lactobacillus and GL bioabsorption, we firstly sought to isolate the Lactobacillus from healthy rats’ feces on an MRS medium. The strain was identified as L. murinus by 16S rDNA sequencing in Figure 1A. Next, we cultured L. murinus and two other common and available lactobacilli, L. rhamnosus and L. acidophilus, with GL in vitro and screened for the GL biotransformation ability. All three Lactobacillus species can bioconvert the GL to GA, and the UPLC/MS results of GL biotransformation by L. murinus were analyzed as the representative for the other two lactobacilli. Our results showed that GL could be partially bioconverted to GA in vitro by L. murinus (Figures 1B,C), and there are no GA produced without the addition of L. murinus,which demonstrated that GL could not convert to GA spontaneously (Figure 1D). Among the three lactobacilli, L. murinus has the highest transformation rate, about 8.3% in 24 h culture, compared with the other two Lactobacillus strains (Figure 1E). L. murinus was selected for further research.
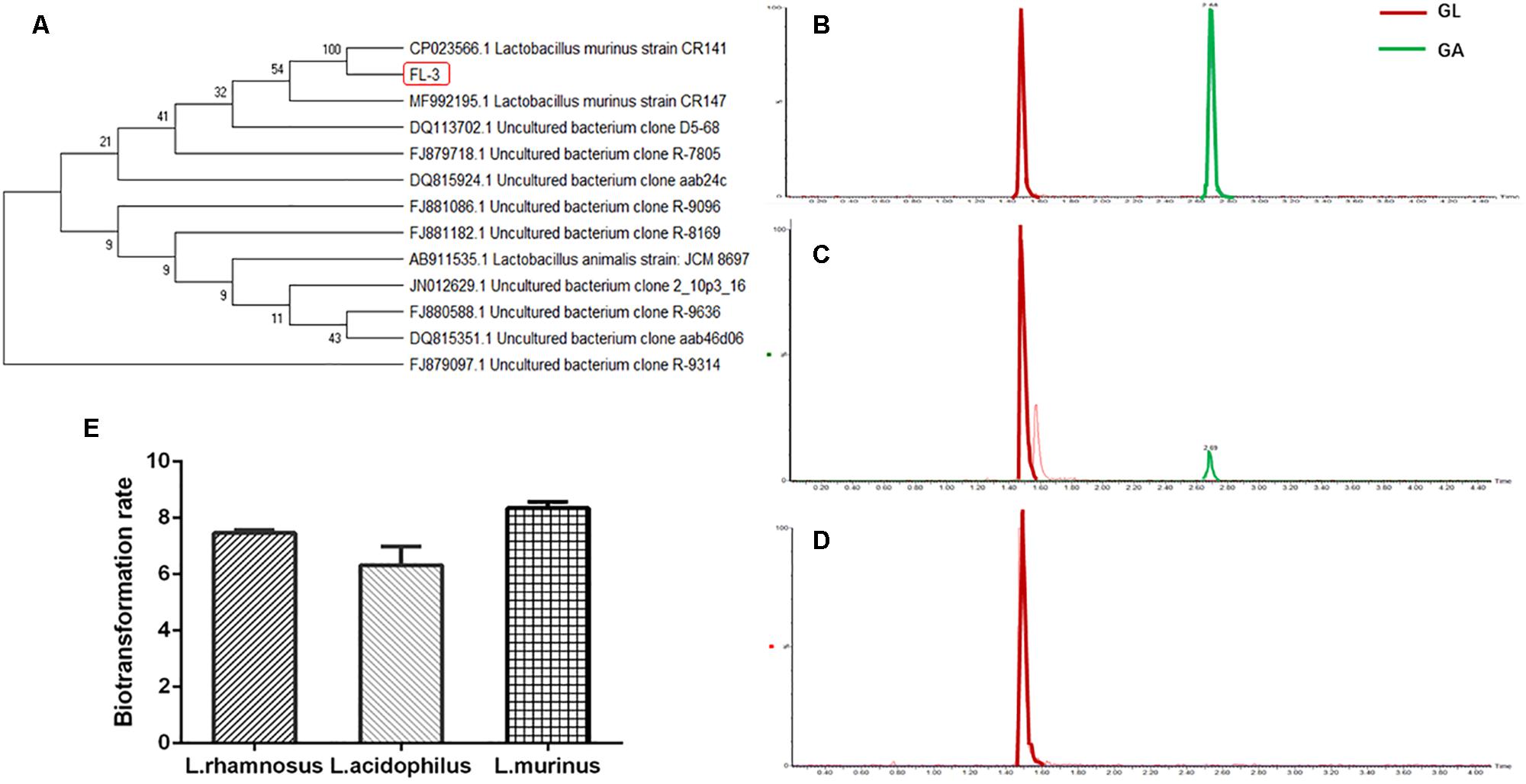
Figure 1. In vitro screening of Lactobacillus for GL biotransformation. (A) Phylogenetic relationships of the Lactobacillus isolated from healthy rats’ feces based on 16S rDNA gene sequencing. The tree was constructed using the neighbor-joining method in MEGA X. (B) UPLC–MS/MS analysis of GL and GA standard; extracted chromatograms of standard GA [M-H]– = 469; extracted chromatograms of standard GL [M-H]– = 821. (C) GL incubated with L. murinus. (D) GL incubated without L. murinus. (E) Biotransformation rate of GL screened by L. murinus, L. rhamnosus, and L. acidophilus in vitro.
L. murinus Promoted the Translocation of GL on the Caco-2 Transwell Model
Furthermore, the ability of L. murinus to mediate the absorption of GL and GA was investigated; we used the Caco-2 Transwell model of the small intense in vitro to investigate the potential effect on GL intestinal absorption by L. murinus. As shown in Figure 2A, L. murinus could significantly increase the Caco-2 apical–basolateral translocation of GL compared to un-supplemented controls. Similar to that of GL, the absorption of GA was moderately increased compared with the control group (Figure 2B). These findings support the idea that L. murinus potentially promotes the absorption of GL and GA.
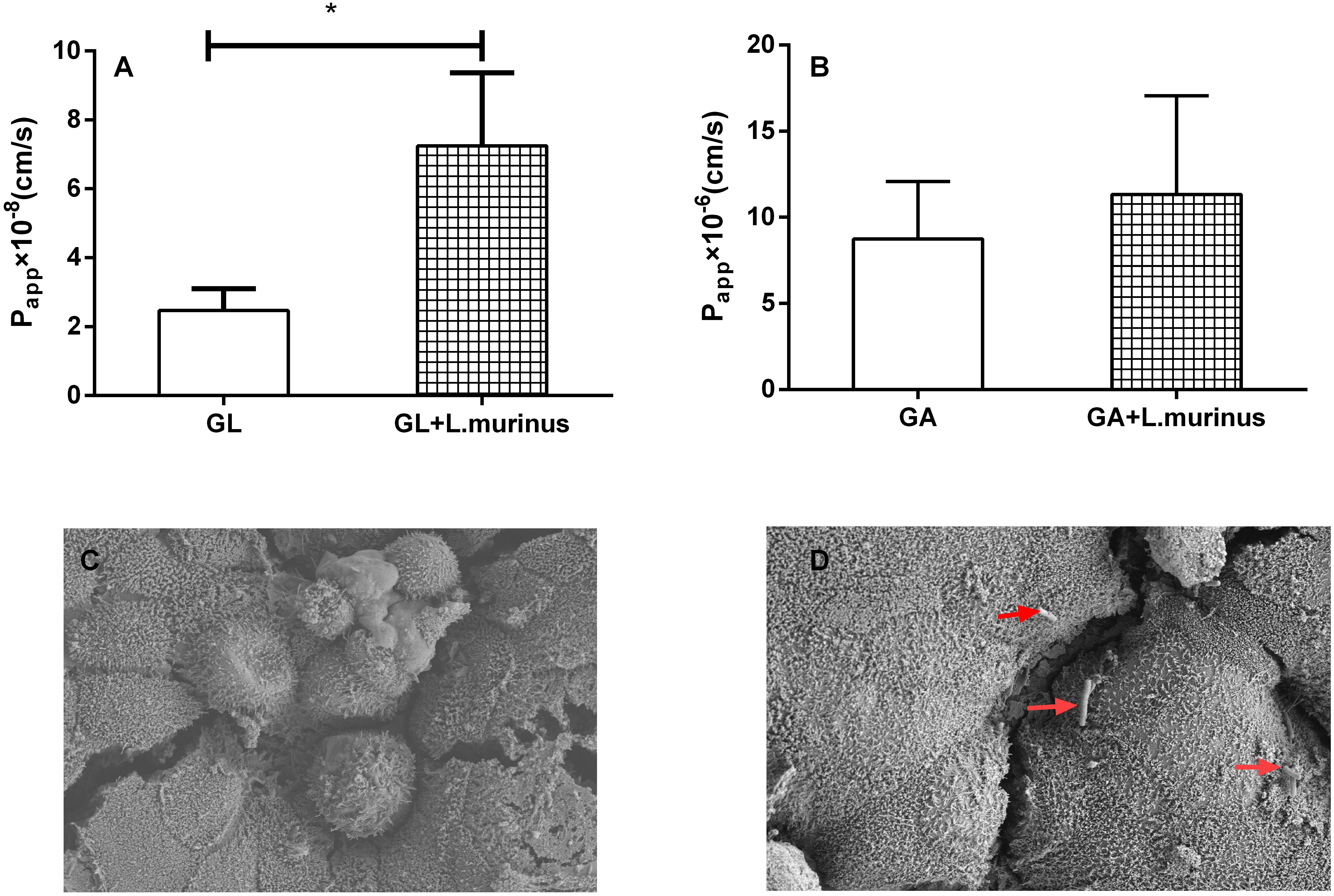
Figure 2. L. murinus promotes the translocation of GL on the Caco-2 Transwell model. (A) The effect of L. murinus on the Papp values of GL. (B) The effect of L. murinus on the Papp values of GA. (C) SEM images of the Caco-2 cells as control. (D) SEM images of L. murinus adherence to the Caco-2. Data are represented as the mean ± SD (n = 3). *P < 0.05 compared with the control group.
Adherence of L. murinus to the Caco-2 Cells
Adherence to intestinal surfaces is usually considered to be a defining activity of a probiotic. Lactobacillus was reported to possess mucin-binding proteins, which are known as one of the effector molecules involved in its adherence to the host (Kaushik et al., 2009). In our present study, SEM analysis of L. murinus adherence to Caco-2 cells was performed. Our results showed that L. murinus definitely bind to the surface of the cell line (Figures 2C,D), which suggests that L. murinus could have direct interaction with the GI cell line.
L. murinus Decreased the Expression of Transporter Genes on Caco-2 Cells
The expression of transporter genes such as P-glycoprotein, multidrug resistance protein 1/2 (MRP1/MRP2), and breast cancer resistance protein (BCRP) plays an important role in the absorption of many drugs. The adhesion of L. murinus to Caco-2 cells was supposed to have induced a variation in the mRNA expression of transporter genes. Caco-2 cells are cultured as a monolayer and spontaneously differentiate into enterocytes, which can form tight junctions between cells and express transporters. Four different transporter gene expressions on Caco-2 cells were investigated by co-culturing with L. murinus. The results showed that the addition of L. murinus to Caco-2 cells could remarkably downregulate the gene expression of MDR1 and MRP2 compared to untreated cultures (Figures 3A,B). whereas the gene expression levels of MRP4 and BCRP were not significantly changed (Figures 3C,D). Our results indicated that the downregulation of transporter genes may have contributed to the absorption of GL and GA in vivo.
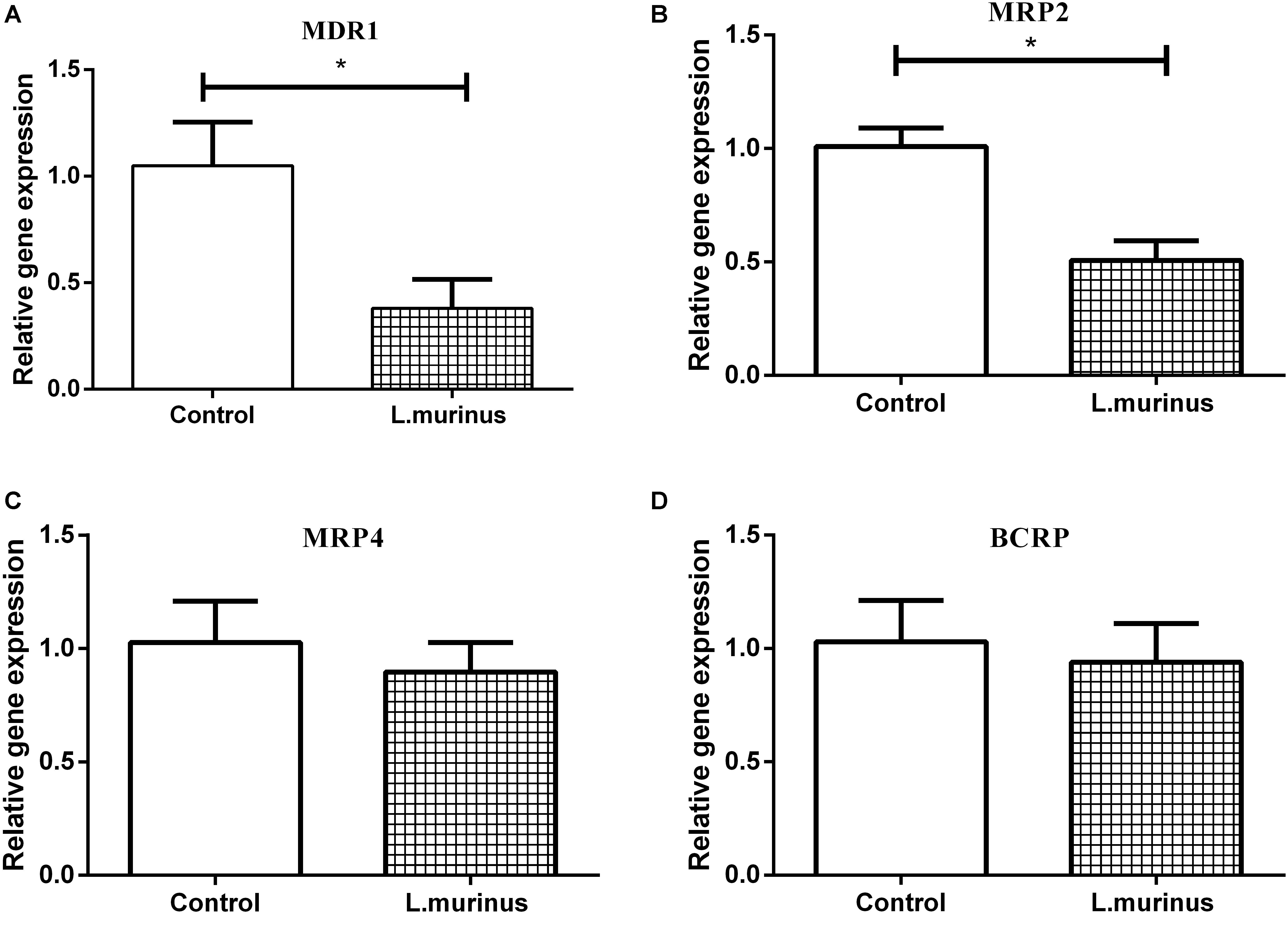
Figure 3. Effect of L. murinus on relative gene expression of transporter genes on Caco-2 cells. Only addition with GL as the control group. (A) The gene expression of MDR1 treated with L. murinus. (B) The gene expression of MRP2 treated with L. murinus. (C) The gene expression of MRP4 treated with L. murinus. (D) The gene expression of BCRP treated with L. murinus. Data are represented as the mean ± SD (n = 3). *P < 0.05 compared with the control group.
GL Oral Bioavailability Decreased Significantly in Liver Injury Rats
CCl4 was used to induce liver cirrhosis in rats; the biochemical analysis of ALT and AST revealed that CCl4 treatment induced a liver injury in the model group (Figure 4B). The plasma concentration levels of GL and GA were determined after GL oral administration. The mean plasma concentration–time profiles of GL and GA in the control and model groups are shown in Figures 4C,D, and the resultant pharmacokinetic data were shown in Table 1. The Cmax values of GL in the control group and model group were 0.29 ± 0.11 and 0.24 ± 0.08 μg/ml, respectively, while the Cmax values of GA in the control group and model group were 2.05 ± 0.48 and 1.16 ± 0.42 μg/ml, respectively. Meanwhile, the AUC value was also significantly decreased in the model group (39.83 ± 20.49 μg/ml h) compared with that in the control group (88.57 ± 25.81 μg/ml h).
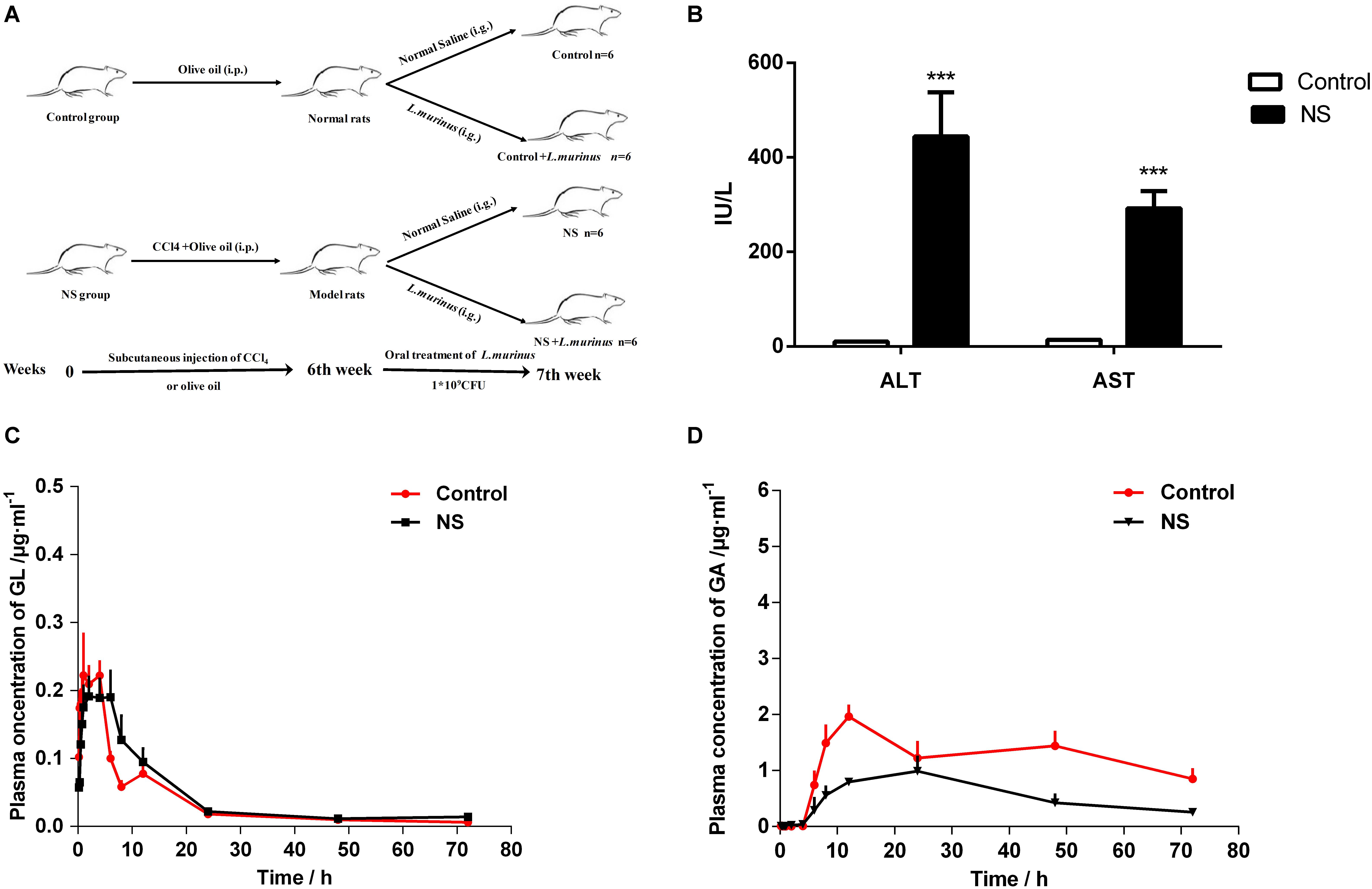
Figure 4. Bioavailability of GL was decreased by liver cirrhosis. (A) Experimental protocol of control group and liver fibrosis model group. (B) Rat serum levels of ALT and AST. (C) Plasma concentration-time curves of GL in healthy and liver cirrhosis group. (D) Plasma concentration–time curves of GA in healthy and liver cirrhosis group. Data are represented as the mean ± S.D. (n = 6). ***P < 0.001 compared with control group.
It has been reported that GL is hardly absorbed through oral administration. Our results showed that the plasma concentration of GL is quite low and that there is no difference between the two groups. However, the concentration of GA metabolized from GL was significantly decreased in the model group. These data indicated that the dysbacteriosis caused by liver injury directly influenced the bioavailability of GL.
L. murinus Improved the Bioavailability of GL Under a Pathological State
To further confirm whether the pharmacokinetics of GL was influenced by Lactobacillus in vivo, L. murinus was selected to be orally administered into the healthy and model groups by a daily gavage protocol. The plasma concentration levels of GL and GA were determined after the oral administration of L. murinus. The plasma concentration-versus-time curves for GL and GA are shown in Figure 5. The AUC value of GL was slightly increased to 3.80 ± 2.49 μg/ml h compared with that in the control group at 2.66 ± 0.56 μg/ml h (Figure 5A), while the AUC value of GA was significantly increased to 147.70 ± 58.62 μg/ml h compared with the control group 88.57 ± 25.81 μg/ml h (Figure 5B).
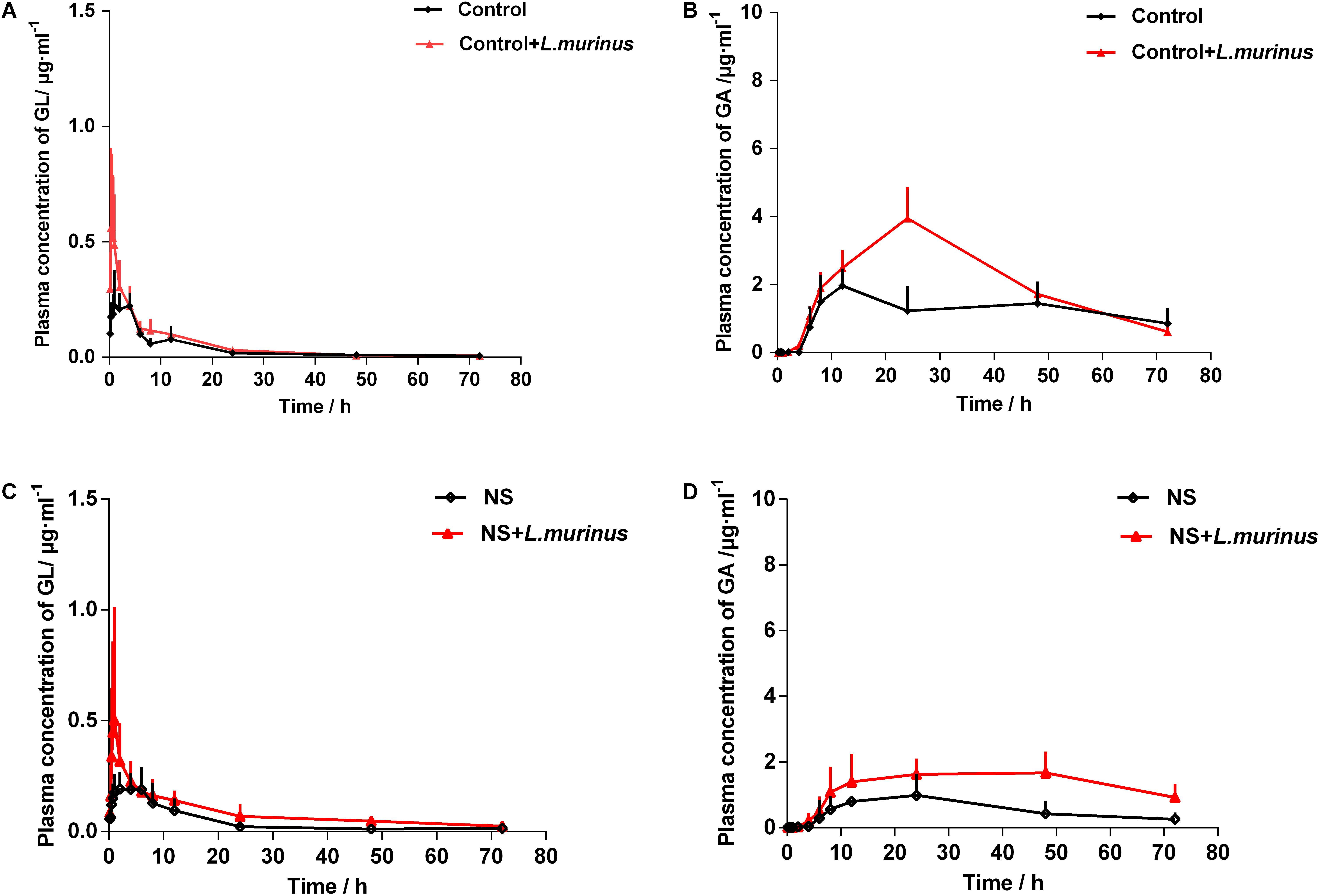
Figure 5. L. murinus promoted the bioavailability of GL under a pathological state. (A) Plasma concentration profiles of GL treated with L. murinus in the healthy group. (B) Plasma concentration profiles of GA treated with L. murinus in the healthy group. (C) Plasma concentration profiles of GL treated with L. murinus in the model group. (D) Plasma concentration profiles of GA treated with L. murinus in the model group. Data are represented as the mean ± SD (n = 6).
As mentioned above, the bioavailability of GL was remarkably decreased in the rats with liver injury. The addition of L. murinus in the model rats was investigated, and the resultant pharmacokinetic parameters were described in Table 1. Remarkably increased plasma levels of GL (AUC = 6.11 ± 0.71 μg/ml h) and GA (AUC = 96.27 ± 23.70 μg/ml h) were observed after L. murinus administration to rats in comparison with the samples from the model group with a GL AUC value of 3.22 ± 0.86 μg/ml h and GA AUC value of 39.83 ± 20.49 μg/ml h (Figures 5C,D). Not only did application of L. murinus increase the bioabsorption of GL in the normal condition, more importantly, it could significantly promote the bioavailability of GL in the liver cirrhosis models. Meanwhile, these results supported the role of gut microbiota, especially Lactobacillus, in GL bioabsorption.
Discussion
GL, isolated from the traditional Chinese medicine liquorice, is known for its detoxifying and hepatoprotective properties, as well as many other pharmacological activities (Li et al., 2014). Orally administered GL is deglycosylated to GA by gut microbiota, which is then absorbed into the blood to have a therapeutic effect. Therefore, the pharmacokinetic parameters of oral GL are hugely influenced by the fluctuating gut composition, especially in certain pathological conditions of the GI tract that might alter the gut microbial composition.
However, the current concept of bioavailability of oral drugs is usually based on the normal animal gut (Enright et al., 2016). Emerging evidences have shown that the modification of gut microbiota can drastically alter drug clinical effectiveness (Hitchings and Kelly, 2019). Recently, E. coli infection was observed to significantly reduce the absorption of orally administered enrofloxacin in broilers (Guo et al., 2014). Yan Wang reported that berberine treatment lowered blood lipids and glucose effectively only in the high-fat diet (HFD)-fed hamsters with increased levels of nitrate reductase (NR) activity in the gut microbiota (Wang et al., 2017). In our previous research work, liver injury affects the gut microbiome in rats, particularly by depleting the Lactobacillus, which is directly involved in the bioconversion of the GL. The suppression of Lactobacillus by liver injury prompted us to investigate whether supplementation of Lactobacillus improves the bioavailability of GL, especially under a pathological state.
In the current work, we isolated the beneficial bacterium from healthy rats’ feces, which was identified as L. murinus. Two other common Lactobacillus strains, namely, L. rhamnosus and L. acidophilus, were purchased from the CGMCC as a representative and screened for GL biotransformation ability in vitro; our result showed that all three Lactobacillus species can bioconvert GL to GA, which partially demonstrated the important role of Lactobacillus in GL bioabsorption. The Caco-2 cell model is a standard tool to predict in vivo intestinal absorption of various drugs (Sambuy et al., 2005). The transfer capacity of L. murinus was validated on the Caco-2 model by co-culturing with GL and GA separately. Our results showed that the supplementation of L. murinus could increase the transport concentration of GL and GA compared with the control group. Under in vitro conditions, the intestinal mucin layer was considered as a suitable model for adherence due to the presence of specific receptors (Devi et al., 2018). It was noted that lots of Lactobacillus species possess this functional property: mucin proteins are involved in the adherence of L. rhamnosus to the host (Barnett et al., 2018), and Lactobacillus plantarum 299v adheres to mannose residues on intestinal epithelial cells (IECs) (Pretzer et al., 2005). L. murinus was also found to bind with the surface of the Caco-2 cell, suggesting its role in the interaction to the host epithelial layer.
The possible mechanism of GL transfer promotion by the addition of L. murinus was further investigated. The expression of intestinal transporters that are involved in drug transport plays an important role in drug bioavailability. MDR1, MRP2/MRP4, and BCRP all belong to the ABC transporters, mainly distributed in the apical membrane of IECs (Harwood et al., 2013; Wang et al., 2019). L. murinus treatment significantly reduced the gene expression of MDR1 and MRP2, which were directly involved in drug efflux, while MRP4 and BCRP were not affected by co-culturing with L. murinus. GL was reported to be a substrate of P-gp, which can reduce the absorption of GL (Feng X. C. et al., 2015). However, P-gp expression and function under normal and inflammatory conditions were found to be significantly enhanced by Lactobacillus (Saksena et al., 2011). This opposite conclusion may result from the fact that each Lactobacillus species has its own specific properties. Our results showed that L. murinus could bind to the surface of Caco-2 cells and downregulate the transporter gene expression compared with control. However, the detailed mechanism was not investigated in this study, so further research is required to address this issue.
Our results are in agreement with the speculation in our previous studies, where rats with liver cirrhosis were observed to have a significant decrease in the absorption of GL. A single dose of GL was orally administered, and the determination of blood concentration of GL and GA showed that the Cmax of GA in rats with liver cirrhosis was about 76.7% lower than that in normal rats. Meanwhile, the AUC measurements of GA showed a similar pattern of Cmax. However, the Cmax and AUC values of GL were found to have no significant changes between the two groups, probably due to the oral administration of GL being hard to be directly absorbed into the blood whether under a healthy or pathological state. Furthermore, our results revealed that the supplementation of L. murinus promoted the bioabsorption of GL in healthy rats; more importantly, the blood concentrations of GL and GA in rats with liver cirrhosis were increased significantly under the treatment of L. murinus. A previous study has reported that the administration of Lactobacillus directly contributes to the improvement of liver injury (Shi et al., 2017). Supplementation of L. murinus could regulate the imbalance of gut microbiota caused by liver cirrhosis; more importantly, it may have directly interacted with the intense layer and promoted GL bioavailability. Our results confirmed that the supplementation of the selected probiotic during the short term is enough to improve the pharmacokinetic characteristics of GL, especially under pathological conditions.
Probiotic intervention is gaining more attention due to its potential to confer a health benefit on the host (Salazar et al., 2019). Lactobacillus is one of the genera that are most commonly used as probiotics, which has exerted various beneficial functions on the host (Druart et al., 2014). L. rhamnosus GG was observed to ameliorate dextran sulfate sodium (DSS)- and oxazolone-induced colitis through the activation of the epidermal growth factor receptor (EGFR) (Yan et al., 2011); the administration of L. rhamnosus LB102 reduced diet-induced obesity and inflammation, concomitant with the improvement of glucose tolerance and insulin sensitivity (Le Barz et al., 2019). L. murinus has also been reported to successfully colonize in rodent intestines and to possess protective properties for necrotizing enterocolitis (Isani et al., 2018). And treatment of mice with L. murinus prevented salt-induced aggravation of actively induced experimental autoimmune encephalomyelitis and salt-sensitive hypertension (Wilck et al., 2017). Recently, L. murinus has been reported to significantly increase the life span and the brood size of the nematode Caenorhabditis elegans (Pan et al., 2018). In the current study, we display the novel aspects of the beneficial property of Lactobacillus, L. murinus was observed to have the capacity to increase the bioavailability of GL for the first time.
Growing evidences have demonstrated that gut flora disequilibrium is closely related with liver cirrhosis; in the current study, our results revealed that microbiota modification definitely influences drug absorption. Combing in vitro screening and in vivo bioabsorption experiments, our data highlighted that supplements of L. murinus significantly improved the bioavailability of orally administered GL, especially under pathological conditions, which may provide a novel strategy for improving the clinical therapeutic effect of liver protective drugs.
Data Availability Statement
The datasets generated for this study can be found in the https://figshare.com/s/823ded22d8ef86a034ca.
Ethics Statement
The animal study was reviewed and approved by Animal Studies Ethics Committee of Nanjing University of Chinese Medicine.
Author Contributions
TY and JW carried out the sample collection and data analysis and drafted the manuscript. LC and JS revised the final draft of the manuscript. LD, who is the corresponding author, conceived of the study and helped to draft the manuscript. All authors read and approved the final manuscript.
Funding
This work was supported by the National Natural Science Foundation of China (no. 81774156).
Conflict of Interest
The authors declare that the research was conducted in the absence of any commercial or financial relationships that could be construed as a potential conflict of interest.
Acknowledgments
We are grateful to all the participants in the study.
Supplementary Material
The Supplementary Material for this article can be found online at: https://www.frontiersin.org/articles/10.3389/fmicb.2020.00597/full#supplementary-material
References
Akao, T. (2000). Effects of glycyrrhizin and glycyrrhetic acid on the growth, glycyrrhizin beta-D-glucuronidase and 3 beta-hydroxysteroid dehydrogenase of human intestinal bacteria. Biol. Pharm. Bull. 23, 104–107. doi: 10.1248/bpb.23.104
Armanini, D., Fiore, C., Mattarello, M. J., Bielenberg, J., and Palermo, M. (2002). History of the endocrine effects of licorice. Exp. Clin. Endocrinol. Diabetes 110, 257–261. doi: 10.1055/s-2002-34587
Barnett, A. M., Roy, N. C., Cookson, A. L., and McNabb, W. C. (2018). Metabolism of caprine milk carbohydrates by probiotic bacteria and Caco-2:HT29-MTX epithelial co-cultures and their impact on intestinal barrier integrity. Nutrients 10:949. doi: 10.3390/nu10070949
Choi, M. S., Yu, J. S., Yoo, H. H., and Kim, D. H. (2018). The role of gut microbiota in the pharmacokinetics of antihypertensive drugs. Pharmacol. Res. 130, 164–171. doi: 10.1016/j.phrs.2018.01.019
Devi, S. M., Kurrey, N. K., and Halami, P. M. (2018). In vitro anti-inflammatory activity among probiotic Lactobacillus species heck isolated from fermented foods. J. Funct. Foods 47, 19–27. doi: 10.1016/j.jff.2018.05.036
Druart, C., Alligier, M., Salazar, N., Neyrinck, A. M., and Delzenne, N. M. (2014). Modulation of the gut microbiota by nutrients with prebiotic and probiotic properties. Adv. Nutr. 5, 624s–633s. doi: 10.3945/an.114.005835
Enright, E. F., Gahan, C. G., Joyce, S. A., and Griffin, B. T. (2016). The impact of the gut microbiota on drug metabolism and clinical outcome. Yale J. Biol. Med. 89, 375–382.
Feng, R., Shou, J. W., Zhao, Z. X., He, C. Y., Ma, C., Huang, M., et al. (2015). Transforming berberine into its intestine-absorbable form by the gut microbiota. Sci. Rep. 5:12155. doi: 10.1038/srep12155
Feng, X. C., Ding, L. Q., and Qiu, F. (2015). Potential drug interactions associated with glycyrrhizin and glycyrrhetinic acid. Drug Metab. Rev. 47, 229–238. doi: 10.3109/03602532.2015.1029634
Guo, M. J., Sun, Y., Zhang, Y., Bughio, S., Dai, X. H., Ren, W. L., et al. (2014). E. coli infection modulates the pharmacokinetics of oral enrofloxacin by targeting P-Glycoprotein in small intestine and CYP450 3A in liver and kidney of broilers. PLoS One 9:e7781. doi: 10.1371/journal.pone.0087781
Han, S. W., Sun, L., He, F., and Che, H. L. (2017). Anti-allergic activity of glycyrrhizic acid on IgE-mediated allergic reaction by regulation of allergy-related immune cells. Sci. Rep. 7:7222. doi: 10.1038/s41598-017-07833-1
Harwood, M. D., Neuhoff, S., Carlson, G. L., Warhurst, G., and Rostami-Hodjegan, A. (2013). Absolute abundance and function of intestinal drug transporters: a prerequisite for fully mechanistic in vitro-in vivo extrapolation of oral drug absorption. Biopharm. Drug Disposit. 34, 2–28. doi: 10.1002/bdd.1810
Hitchings, R., and Kelly, L. (2019). Predicting and understanding the human microbiome’s impact on pharmacology. Trends Pharmacol. Sci. 40, 495–505. doi: 10.1016/j.tips.2019.04.014
Hosseinzadeh, H., and Nassiri-Asl, M. (2015). Pharmacological effects of Glycyrrhiza spp. and its bioactive constituents: update and review. Phytother. Res. 29, 1868–1886. doi: 10.1002/ptr.5487
Isani, M., Bell, B. A., Delaplain, P. T., Bowling, J. D., Golden, J. M., Elizee, M., et al. (2018). Lactobacillus murinus HF12 colonizes neonatal gut and protects rats from necrotizing enterocolitis. PLoS One 13:e0196710. doi: 10.1371/journal.pone.0196710
Kaushik, J. K., Kumar, A., Duary, R. K., Mohanty, A. K., Grover, S., and Batish, V. K. (2009). Functional and probiotic attributes of an indigenous isolate of Lactobacillus plantarum. PLoS One 4:e8099. doi: 10.1371/journal.pone.0008099
Kim, D. H. (2015). Gut microbiota-mediated drug-antibiotic interactions. Drug Metab. Dispos. 43, 1581–1589. doi: 10.1124/dmd.115.063867
Kim, D. H. (2018). Gut microbiota-mediated pharmacokinetics of ginseng saponins. J. Ginseng Res. 42, 255–263. doi: 10.1016/j.jgr.2017.04.011
Kim, J. K., Choi, M. S., Jeong, J. J., Lim, S. M., Kim, I. S., Yoo, H. H., et al. (2018). Effect of probiotics on pharmacokinetics of orally administered acetaminophen in mice. Drug Metab. Dispos. 46, 122–130. doi: 10.1124/dmd.117.077222
Le Barz, M., Daniel, N., Varin, T. V., Naimi, S., Demers-Mathieu, V., Pilon, G., et al. (2019). In vivo screening of multiple bacterial strains identifies Lactobacillus rhamnosus Lb102 and Bifidobacterium animalis ssp. lactis Bf141 as probiotics that improve metabolic disorders in a mouse model of obesity. FASEB J. 33, 4921–4935. doi: 10.1096/fj.201801672R
Li, J. Y., Cao, H. Y., Liu, P., Cheng, G. H., and Sun, M. Y. (2014). Glycyrrhizic acid in the treatment of liver diseases: literature review. Biomed. Res. Int. 2014:872139. doi: 10.1155/2014/872139
Matuskova, Z., Anzenbacherova, E., Vecera, R., Tlaskalova-Hogenova, H., Kolar, M., and Anzenbacher, P. (2014). Administration of a probiotic can change drug pharmacokinetics: effect of E. coli Nissle 1917 on amidarone absorption in rats. PLoS One 9:e87150. doi: 10.1371/journal.pone.0087150
Pan, F. W., Zhang, L. Y., Li, M., Hu, Y. X., Zeng, B. H., Yuan, H. J., et al. (2018). Predominant gut Lactobacillus murinus strain mediates anti-inflammaging effects in calorie-restricted mice. Microbiome 6:54. doi: 10.1186/s40168-018-0440-5
Pretzer, G., Snel, J., Molenaar, D., Wiersma, A., Bron, P. A., Lambert, J., et al. (2005). Biodiversity-based identification and functional characterization of the mannose-specific adhesin of Lactobacillus plantarum. J. Bacteriol. 187, 6128–6136. doi: 10.1128/Jb.187.17.6128-6136.2005
Saksena, S., Goyal, S., Raheja, G., Singh, V., Akhtar, M., Nazir, T. M., et al. (2011). Upregulation of P-glycoprotein by probiotics in intestinal epithelial cells and in the dextran sulfate sodium model of colitis in mice. Am. J. Physiol. Gastrointest. Liver Physiol. 300, G1115–G1123. doi: 10.1152/ajpgi.00027.2011
Salazar, N., Neyrinck, A. M., Bindels, L. B., Druart, C., Ruas-Madiedo, P., Coni, P. D., et al. (2019). Functional effects of EPS-producing bifidobacterium administration on energy metabolic alterations of diet-induced obese mice. Front. Microbiol. 10:1809. doi: 10.3389/fmicb.2019.01809
Sambuy, Y., Angelis, I., Ranaldi, G., Scarino, M. L., Stammati, A., and Zucco, F. (2005). The Caco-2 cell line as a model of the intestinal barrier: influence of cell and culture-related factors on Caco-2 cell functional characteristics. Cell Biol. Toxicol. 21, 1–26. doi: 10.1007/s10565-005-0085-6
Sastry, S. V., Nyshadham, J. R., and Fix, J. A. (2000). Recent technological advances in oral drug delivery - a review. Pharm. Sci. Technol. Today 3, 138–145. doi: 10.1016/s1461-5347(00)00247-9
Sekirov, I., Russell, S. L., Antunes, L. C. M., and Finlay, B. B. (2010). Gut microbiota in health and disease. Physiol. Rev. 90, 859–904. doi: 10.1152/physrev.00045.2009
Shi, D., Lv, L., Fang, D., Wu, W., Hu, C., Xu, L., et al. (2017). Administration of Lactobacillus salivarius LI01 or Pediococcus pentosaceus LI05 prevents CCl4-induced liver cirrhosis by protecting the intestinal barrier in rats. Sci. Rep. 7:6927. doi: 10.1038/s41598-017-07091-1
Stojancevic, M., Bojic, G., Al Salami, H., and Mikov, M. (2014). The influence of intestinal tract and probiotics on the fate of orally administered drugs. Curr. Issues Mol. Biol. 16, 55–67.
Theilmann, M. C., Goh, Y. J., Nielsen, K. F., Klaenhammer, T. R., Barrangou, R., and Abou Hachem, M. (2017). Lactobacillus acidophilus metabolizes dietary plant glucosides and externalizes their bioactive phytochemicals. mBio 8:e001421-17. doi: 10.1128/mBio.01421-17
Wang, Y., Tong, Q., Shou, J. W., Zhao, Z. X., Li, X. Y., Zhang, X. F., et al. (2017). Gut microbiota-mediated personalized treatment of hyperlipidemia using berberine. Theranostics 7, 2443–2451. doi: 10.7150/thno.18290
Wang, Y. F., Zuo, Y. Q., Deng, S. Y., Zhu, F., Liu, Q., Wang, R. R., et al. (2019). Using caffeine and free amino acids to enhance the transepithelial transport of catechins in Caco-2 Cells. J. Agric. Food Chem. 67, 5477–5485. doi: 10.1021/acs.jafc.9b01701
Wilck, N., Matus, M. G., Kearney, S. M., Olesen, S. W., Forslund, K., Bartolomaeus, H., et al. (2017). Salt-responsive gut commensal modulates T(H)17 axis and disease. Nature 551, 585–589. doi: 10.1038/nature24628
Wilson, I. D., and Nicholson, J. K. (2017). Gut microbiome interactions with drug metabolism, efficacy, and toxicity. Transl. Res. 179, 204–222. doi: 10.1016/j.trsl.2016.08.002
Xu, J., Chen, H. B., and Li, S. L. (2017). Understanding the molecular mechanisms of the interplay between herbal medicines and gut microbiota. Med. Res. Rev. 37, 1140–1185. doi: 10.1002/med.21431
Yan, F., Cao, H., Cover, T. L., Washington, M. K., Shi, Y., Liu, L., et al. (2011). Colon-specific delivery of a probiotic-derived soluble protein ameliorates intestinal inflammation in mice through an EGFR-dependent mechanism. J. Clin. Invest. 121, 2242–2253. doi: 10.1172/JCI44031
Yuan, T. J., Wang, J., Chen, L. T., Shan, J. J., and Di, L. Q. (2019). Glycyrrhizic acid improving the liver protective effect by restoring the composition of Lactobacillus. J. Funct. Foods 52, 219–227. doi: 10.1016/j.jff.2018.11.001
Zhang, J. H., Zhang, J. M., and Wang, R. (2018). Gut microbiota modulates drug pharmacokinetics. Drug Metab. Rev. 50, 357–368. doi: 10.1080/03602532.2018.1497647
Zhao, M., Xu, J., Qian, D. W., Guo, J. M., Jiang, S., Shang, E. X., et al. (2014). Identification of astilbin metabolites produced by human intestinal bacteria using UPLC-Q-TOF/MS. Biomed. Chromatogr. 28, 1024–1029. doi: 10.1002/bmc.3111
Zhou, W., Wang, H., Zhu, X., Shan, J., Yin, A., Cai, B., et al. (2013). Improvement of intestinal absorption of forsythoside A and chlorogenic acid by different carboxymethyl chitosan and chito-oligosaccharide, application to Flos Lonicerae-Fructus Forsythiae herb couple preparations. PLoS One 8:e63348. doi: 10.1371/journal.pone.0063348
Keywords: glycyrrhizic acid, bioavailability, Lactobacillus murinus, oral administration, intestinal microbiota
Citation: Yuan T, Wang J, Chen L, Shan J and Di L (2020) Lactobacillus murinus Improved the Bioavailability of Orally Administered Glycyrrhizic Acid in Rats. Front. Microbiol. 11:597. doi: 10.3389/fmicb.2020.00597
Received: 24 December 2019; Accepted: 18 March 2020;
Published: 24 April 2020.
Edited by:
Laurent Dufossé, Université de la Réunion, FranceReviewed by:
Daiqiong Fang, Zhejiang University, ChinaChaoxin Man, Northeast Agricultural University, China
Nevena Ivanović, University of Belgrade, Serbia
Copyright © 2020 Yuan, Wang, Chen, Shan and Di. This is an open-access article distributed under the terms of the Creative Commons Attribution License (CC BY). The use, distribution or reproduction in other forums is permitted, provided the original author(s) and the copyright owner(s) are credited and that the original publication in this journal is cited, in accordance with accepted academic practice. No use, distribution or reproduction is permitted which does not comply with these terms.
*Correspondence: Liuqing Di, ZGlsaXVxaW5nMDkyOEAxNjMuY29t